- 1Agricultural Innovation and Technology Transfer Center (AITTC), College of Agriculture and Environmental Sciences (CAES), University Mohammed VI Polytechnic (UM6P), Benguerir, Morocco
- 2Research Institute of Organic Agriculture (FIBL), Frick, Switzerland
- 3Centre Régional de la Recherche Agronomique de Settat (CRRA-Settat), Institut National de la Recherche Agronomique, Settat, Morocco
- 4Département de Production, Protection et Biotechnologie Végétales, Institut Agronomique et Vétérinaire Hassan II (IAV Hassan II), Rabat, Morocco
- 5Centre Régional de la Recherche Agronomique de Rabat (CRRA-Rabat), Institut National de la Recherche Agronomique, Rabat, Morocco
- 6Société L’Ouest Marocain, Meknes, Morocco
Few studies have investigated how one-time targeted tillage of long-term no-till fields impacts topsoil properties and weed dynamics. An on-farm trial was implemented in 2020 to test the effects of occasional tillage (OT) in Morocco with a long-term no-tillage (NT) system and rainfed field crops: durum wheat (Triticum durum), faba bean (Vicia faba minor), and chickpea (Cicer arietinum). Four treatments were established, namely, continuous NT with crop residues maintained (“NT + residue”); continuous NT with crop residues not maintained (“NT-residue”); shallow inversion tillage (“shallow OT”); and deep non-inversion tillage (“deep OT”). We assessed the effect of these treatments on soil physical and chemical properties in 0–10 and 10–20 cm soil depths after crop harvest of the 2020–2021 (year 1) and 2021–2022 (year 2) growing seasons corresponding to 1 and 2 years after OT, respectively. In addition, we evaluated the effect of the treatments on weed populations and the effect of the legume crop rotated with wheat on soil nitrogen (N) and weed density. In year 1, deep OT reduced the water content at field capacity and available water capacity at 0–10 cm compared to continuous NT; the cation-exchange capacity (CEC) under deep OT was lower than in NT-residue and NT + residue at 0–10 cm and 10–20 cm, respectively. Furthermore, deep OT increased ammonium-N (NH4-N) at 0–10 and 10–20 cm compared to NT + residue but reduced exchangeable potassium (K) at 10–20 cm depth compared to NT-residue. In year 2, shallow OT had lower total porosity at 10–20 cm than NT + residue, while shallow and deep OT recorded higher water-stable aggregates at 0–10 cm than NT + residue; at 10–20 cm, deep OT recorded lower CEC than NT + residue. However, deep OT had higher nitrate-N (NO3-N) and available sulfur (S) than NT-residue at 10–20 cm. Occasional tillage did not significantly affect 10 out of 19 of the soil properties evaluated, including soil organic matter (SOM), in all the years and did not help reduce the stratification of soil nutrients in NT. In year 1, 50 days after OT, deep OT reduced the weed density by 46% compared to NT + residue, while in year 2, 406 days after OT, shallow OT reduced weed density by 53% compared to NT-residue. Regarding the effect of the legume rotated with wheat, faba bean appeared to be the better preceding or following wheat crop as it resulted in higher residual soil mineral N and lower weed infestation than chickpea.
1 Introduction
Occasional tillage (OT), also known as strategic tillage, is an innovative practice within conservation agriculture (CA) that aims to address some biophysical constraints in long-term no-tillage (NT) systems such as weed management, soil compaction, water repellency, stratification of soil organic matter (SOM) and nutrients, and increased incidence of soil- and crop residue-borne diseases (Radford and Thornton, 2011; Dang et al., 2015; Crawford et al., 2018; Liu et al., 2020; Wortmann and Dang, 2020; McPheeters et al., 2022). For instance, it has been found in a meta-analysis study that NT farming increased soil bulk density (i.e., higher risk of soil compaction) by 2.3% compared to conventional tillage (Li et al., 2020). The soil type and nature of NT problems to be overcome will determine whether OT involves shallow or deep cultivation (Hall et al., 2020).
Generally, OT is applied once as tillage is not strictly a principle of CA but a lever that can correct soil or weed problems in long-term NT systems. To the best of our knowledge, in the research community, OT has been rarely applied more than once in consecutive years. However, OT can be performed periodically in NT systems (Pierce and Fortin, 1996; Grandy et al., 2006). For instance, in the United States, periodic OT has been used for a long time as part of a crop rotation or to manage pest or soil problems (Pierce and Fortin, 1996).
However, tillage practices are known to affect soil health attributes potentially. A major effect of tillage on the soil system is the disruption of soil aggregates (Karunatilake and Van Es, 2002; Olchin et al., 2008; Triplett and Dick, 2008). By breaking soil aggregates, tillage disrupts the physical protection of SOM and favors its microbial decomposition (Olchin et al., 2008; Gozubuyuk et al., 2014; Issah et al., 2021). Compared to NT, tillage causes a redistribution of SOM and plant nutrients in the soil (Asefa, 2001). However, tillage can be a useful practice for reducing soil mechanical resistance and bulk density, hence alleviating soil compaction (McConkey et al., 2012), increasing soil macroporosity (Pierce and Fortin, 1996), increasing infiltration, and improving saturated hydraulic conductivity in the short term (Haruna et al., 2018). Nevertheless, tillage breaks the continuity of the soil pore network (Jin et al., 2017). As for NT, it contributes to improving soil health, mainly by increasing SOM, soil microbial biomass, and soil structure and hydraulic properties, especially when associated with the retention of crop residues (Mrabet, 2008; Li et al., 2018; 2020).
Consequently, after a long period of continuous NT, soil tillage could impact the soil ecosystem, including soil microbiome, SOM, nutrients and water, and soil particle arrangement. Hence, it is crucial to evaluate the effects of OT on soil properties. A key question in the research community is whether one-time tillage in a long-term NT system reduces the soil health benefits accumulated over many years, especially regarding SOM, soil biology, soil aggregation, and nutrient content. Many uncertainties exist regarding the effects of one-time OT in long-term NT lands (McPheeters et al., 2022). Wortmann and Dang (2020) reported that OT has very often had minor or no short-term beneficial or harmful effects on soil properties. They reported that OT often reduces bulk density and soil compaction, weed density, microbial biomass, or activity and increases water infiltration, soil erosion, nutrient availability (particularly for low-mobile nutrients such as phosphorus), and grain yield. Tilling soil under long-term NT could decrease soil aggregation and accelerate SOM, soil carbon (C), and nitrogen (N) losses and then undo the years of soil restoration through NT (Grandy et al., 2006). Furthermore, OT may cause disturbance of soil life (Melero et al., 2011). However, the effects of OT on soil properties might vary depending on the NT age, tillage implementation, soil type, and climate (Blanco-Canqui and Ruis, 2018; Conyers et al., 2019). Chisel and disk harrow are two tillage implements commonly used to conduct OT (Blanco-Canqui and Wortmann, 2020).
The effects of OT may also diminish over time. For instance, Fidalski et al. (2015) found that the effect of OT with plowing and harrowing on the reduction of soil bulk density had an ephemeral duration of 6 months. Pierce et al. (1994) found that the effects of OT with moldboard plowing on soil physical and chemical properties were evident 1 year after tillage, but most soil properties had returned to levels of NT 4–5 years after tillage. It has been reported that OT can be done without causing a decrease in soil organic carbon (SOC) or soil aggregate stability during the 2 or 3 years following tillage application (Garcia et al., 2007).
In the literature, the evaluation time of the effects of OT on soil physical, chemical, and biological properties varies from a few weeks or months to 1–5 years after tillage implementation (Blanco-Canqui and Wortmann, 2020; Wortmann and Dang, 2020). For instance, Liu et al. (2016a) and Çelik et al. (2019) evaluated soil microbiological properties and soil physical properties, respectively, both 1 year after OT implementation. Some researchers, including Stockfisch et al. (1999) and López-Garrido et al. (2011), assessed the effects of OT on SOC 1, 2, and 3 years after OT implementation. Wortmann et al. (2010) evaluated soil properties and crop yield in eastern Nebraska 5 years after the implementation of one-time OT with a moldboard plow.
Many studies have reported an increase in weed abundance and a higher reliance on herbicides in CA than those in conventional tillage (MacLaren et al., 2021). In addition, tillage reduction may cause weed communities to shift from annual dicots to grassy annuals and perennials (Nichols et al., 2015). Despite the availability of herbicides with new active ingredients, weed issues continue to be one of the major barriers to farmers using NT systems (Derrouch et al., 2020). Weed control is a major reason for the implementation of OT in otherwise NT farming systems (Kettler et al., 2000; Dang et al., 2015; Crawford et al., 2018; MacLaren et al., 2021). To manage weeds that are difficult to control with herbicides, weeds that are too advanced to control with herbicides, and herbicide-resistant weeds, OT may be required in NT systems (Radford and Thornton, 2011). Occasional tillage has been suggested as an option for integrated weed management (IWM) through the integration of tillage, crop diversification (rotations and cover crops), and crop residue retention with chemical herbicides for weed control (McPheeters et al., 2022).
In Morocco, weeds have been reported to cause serious problems for crop production in NT systems mainly for cereal crops, favoring some grassy (e.g., Bromus rigidus and Lolium rigidum) and perennial weeds (Hajjaj et al., 2016). Weed management in NT systems is mainly based on the use of chemical herbicides and crop rotations (Mrabet et al., 2012). To the best of our knowledge, in Morocco, no study has been done so far on the potentiality of using OT to manage weeds in medium-to-long-term NT systems. Beyond weed management, OT is considered an option that could minimize the risk of soil compaction in NT systems in North African regions, where grazing at high stocking densities and soil moisture can cause soil compaction (Diop et al., 2024). No study has been done on the effects of OT on soil conditions and the dynamics of weeds in long-term NT systems in North Africa, unlike other Mediterranean countries such as France, Spain, or Türkiye. In addition, worldwide, there is limited research on the effect of OT on soil properties in the short and long terms (Stavi et al., 2011; Crawford et al., 2014; Crawford et al., 2018). This study, which is the first to be carried out in North Africa, would provide information on the expected effects of using OT in NT systems for a specific purpose.
The present study aims to investigate the effects of OT on soil physical and chemical properties, including nutrient stratification, and weed populations in three crops, namely, wheat, faba bean, and chickpea, in a long-term (10 years) continuous NT system under rainfed conditions. We hypothesized that OT would
- lead to better soil physical conditions, mainly through lower bulk density (i.e., lower risk of soil compaction) and higher soil total porosity and plant-available water,
- reduce the stratification of soil nutrients, mainly phosphorus (P) and potassium (K), relative to continuous NT,
- cause changes in SOM and other soil chemical properties, and
- decrease weed densities in the short term.
Another objective of our study was comparing the effect of the legume crop (faba bean vs. chickpea) used in rotation with wheat (the main crop of the experiment) on soil properties, mainly the forms and levels on soil N (total, NO3-, NH4-, and mineral N) and weed density. We hypothesized that the growing of faba bean vs. chickpea as precedent or following crops for wheat leads to different levels of soil N and weed population.
2 Materials and methods
2.1 Study area
The on-farm trial was implemented in a long-term NT system, which was installed in 2010 in the Meknes region (Morocco) (33°72 N, 5°69 W, and 702 m altitude) (Figure 1). The trial site has a semi-arid Mediterranean climate with hot and dry summers and wet winters, classified as Csa (warm-temperate summer or dry-hot summer), according to the Köppen climate classification system (Hadria et al., 2019). The trial was implemented on a flat slope and clayed soil (52% of clay + 21% of silt + 27% of sand in the 0–40-cm soil layer). The soil was classified as Luvisol (IUSS Working Group WRB, 2022). The description of the soil profile of the experiment is shown in Supplementary Table S1. The meteorological data (monthly temperature and rainfall) of the trial site during the 2020–2021 (year 1) and 2021–2022 (year 2) growing seasons are presented in Figure 2. Cumulative rainfall in the trial site from October to June in years 1 and 2 is 344.6 mm and 376 mm, respectively. Further details on the trial site regarding the history of the land used for the experiment and soil characterization at the trial establishment are given by Diop et al. (2024).
2.2 Experimental design
The trial investigated the effect of four tillage treatments applied once in November 2020 in a 10-year continuous no-till field with cereal–legume rotations: continuous NT with crop residues maintained “NT + residue,” continuous NT with crop residues not maintained “NT-residue,” shallow inversion tillage with an offset disk harrow at a depth of 10 cm (first occasional tillage option) “shallow OT,” and deep non-inversion tillage with a chisel at a depth of 25 cm (second occasional tillage option) “deep OT.” Three crops were sown in the trial: durum wheat (Triticum durum), faba bean (Vicia faba minor), and chickpea (Cicer arietinum). OT treatments were applied 2 days before sowing, which was carried out on 12 November 2020 for all crops. The experimental design is a split plot with crops in the main plots and tillage treatments in the subplots, with three blocks or replicates. Details on crop management and crop phenology appearance in years 1 and 2 are presented in Supplementary Tables S2, S3, respectively. The plot size at the trial establishment was 30 m × 36 m for durum wheat and 15 m × 36 m for faba bean and chickpea. During the 2021–2022 season, crop rotations were conducted according to the following pattern:
-Wheat (2020–2021) is followed by faba bean and chickpea (2021–2022)
-Faba bean (2020–2021) is followed by wheat (2021–2022)
-Chickpea (2020–2021) is followed by wheat (2021–2022)
In the following sections, “wheat/faba bean,” “wheat/chickpea,” “faba bean/wheat,” and “chickpea/wheat” indicate wheat sown after faba bean, wheat sown after chickpea, faba bean sown after wheat, and chickpea sown after wheat during the growing season 2021–2022 (year 2), respectively. In 2021–2022, all the wheat plots initially with a size of 30 m × 36 m in 2020–2021 were split into 15 m × 36-m plots occupied by faba bean and chickpea.
2.3 Soil sampling and assessment
The soil physical and chemical properties evaluated in this study included soil bulk density (BD), total porosity (TP), water-stable aggregates (WSAs), field capacity (FC), permanent wilting point (PWP), available water capacity (AWC), SOM, soil pH, soil electrical conductivity (EC), cation exchange capacity (CEC), total nitrogen (N), nitrate-N (NO3-N), ammonium-N (NH4-N), mineral N, available phosphorus (P), exchangeable potassium (K), available sulfur (S), exchangeable calcium (Ca), and exchangeable magnesium (Mg). The soil samples were collected after crop harvest in year 1 (November 2021, first soil evaluation) and year 2 (November 2022, second soil evaluation) in all treatments and crops in two soil layers, 0–10 and 10–20 cm, using an auger. Furthermore, we took undisturbed soil samples in cores to determine the bulk density in the same soil depths.
Soil BD was determined by the core method and calculated as the ratio of the mass of soil dry solids to the soil bulk volume (Blake and Hartge, 1986a). The mass was determined after drying soil samples to a constant weight at 105°C, and the volume of the soil sample corresponds to the core volume.
Soil TP (%) was calculated using Equation 1 (Yang et al., 2021):
Soil PD was determined as the ratio of the total mass of the solid particles to their total volume using the volumetric method (Blake and Hartge, 1986b). A weight of 20 g of 2-mm-sieved and dry soil was transferred into a 50-cm3 flask, and then, ethyl alcohol was added. The soil volume was calculated as the difference between the flask volume and the volume of alcohol added to reach the flask volume.
Aggregate stability, expressed as the percentage of WSAs, was determined by the wet sieving method described by Kemper and Rosenau (1986) and using the Eijkelkamp wet sieving apparatus (Eijkelkamp Soil and Water, 2018). This method is based on the principle that when soil aggregates are submerged in water, unstable aggregates break down more quickly than stable aggregates. To determine the WSAs (in % w/w), eight sieves with a 60-mesh screen were filled with 1–2 mm of air-dried soil (4 g). These sieves were placed within a first set of water-filled cans that moved up and downward for a fixed time. Unstable aggregates disintegrated, passed through the sieve, and were collected in the water-filled cans underneath the sieve. The soil remaining on the sieve was then immersed in a second set of cans containing a dispersing solution (2 g l–1 sodium hexametaphosphate). The dispersion of aggregates was carried out until only sand particles (and root fragments) were left on the sieve. The weight of unstable and stable aggregates was determined after drying both sets of cans and subtracting the empty weight of the cans. In the dispersing solution, the weight of the dispersing solute (0.2 g) was subtracted to obtain the soil weight. The percentage of water-stable aggregates was calculated using Equation 2 (Outbakat et al., 2022).
where (A–0.2) is the dry weight of water-stable aggregates and B is the dry weight of water-unstable aggregates.
Soil FC and PWP were determined by the pressure plate method at matric potentials of −33 and −1,500 kPa, respectively, using 5 and 15-bar pressure plate extractors, as described by Beniaich et al. (2023). First, soil samples were saturated for 24 h by capillarity. Then, they were drained to 0.33 and 15 bar on ceramic pressure plate extractors (SoilMoisture Equipment Corp.). Finally, we determined the gravimetric water content at FC and PWP by drying at 105°C for 24 h and weighing the samples. Soil AWC was calculated using Equation 3:
Soil organic matter content was measured according to the NF ISO 14235 standard. The method consists of the determination of the content of SOC content by spectrocolorimetry after its oxidation by a quantity of potassium dichromate in excess in a sulfochromic reaction medium at 135°C. The soil organic matter content was calculated from SOC content by multiplying by a coefficient of 1.724.
Soil EC was determined according to the NF ISO 11265 standard. A conductivity meter was used to measure the specific EC of an aqueous soil extract prepared at an extraction ratio of 1/5 (w/v) with water at 20°C ± 1°C. The result of the measurement of the specific EC of the filtered extract was corrected to a temperature of 25°C. Soil pH was determined according to the NF ISO 10390 standard. A pH meter equipped with a glass electrode immersed in a suspension of soil diluted to 1/5 (v/v) in water (i.e., water pH) was used to measure pH.
Soil CEC was measured according to the NF X 31-130 standard. The soil CEC was determined using cobaltihexamine chloride. After the exchange of soil cations with the [Co(NH3)6]3+ ion, the estimation of the CEC is based on the determination of the remaining Co in the solution. Soil total nitrogen (TN) was determined according to the Kjeldahl method. The soil sample was mineralized in a sulfuric acid medium in the presence of copper (II) and a catalyst (titanium oxide). Soil NO3-N, NH4-N, and available S (SO42-) content of the soil were determined according to the Skalar protocol using an extraction ratio of 1/5 (w/v). Soil mineral N was calculated as the sum of soil NO3-N and NH4-N.
Available P (P2O5) was determined according to the NF ISO 11263 standard. The available P was measured based on the extraction of soluble P from the soil with a sodium hydrogen carbonate solution and the formation of a colored complex that allows its determination by spectrocolorimetry. Exchangeable K (K2O), Ca (CaO), and Mg (MgO) contents were determined according to the NF X 31-108 standard. The exchangeable K, Ca, and Mg are extracted (extraction ratio of 1/20 (m/v)) using a solution of ammonium acetate at 1 mol/L adjusted to a neutral pH (=7) and measured by atomic absorption spectrometry.
For each of the soil nutrients studied, including mineral N, available P and S, and exchangeable K, Ca, and Mg, we evaluated their vertical distribution or their degree of mixing in the soil through the stratification ratio using Equation 4 (Franzluebbers, 2002):
2.4 Weed density evaluation
Weed assessment was carried out using the methodology described by Tanji and Boutfirass (2018) and Tanji and El Brahli (2018). Weeds were collected using randomly placed 0.5-m2 (1 m × 0.5 m) quadrats in triplicate in each plot. All the weeds within a quadrat were clipped at ground level and bagged. The fresh weed samples were brought to the laboratory, counted, and identified. The weed species were identified using the “Flora of Morocco” by Fennane et al. (1999); Fennane et al. (2007); and Fennane et al. (2014), while their scientific names were determined according to the recommendations of Dobignard and Chatelain (2010); Dobignard and Chatelain (2011a); Dobignard and Chatelain (2011b); Dobignard and Chatelain (2012); and Dobignard and Chatelain (2013). Weed density (number of weeds per m2) was assessed at three dates for all crops during the growing season 2020–2021: 48, 111, and 132 days after sowing (DAS). During the growing season 2021–2022, weed density was evaluated at four dates: 19, 43, 91, and 123 days after wheat sowing, corresponding to 18, 42, 90, and 122 days after sowing for both faba bean and chickpea. In the first year of the experiment, the weed samplings showed the presence of five major weeds: bur clover (Medicago polymorpha), foxtail restharrow (Ononis alopecuroides), knotted hedge-parsley (Torilis nodosa), common chicory (Cichorium intybus), and common poppy (Papaver rhoeas). In the second year, the major weeds in the field trial were T. nodosa, M. polymorpha, P. rhoeas, C. intybus, friar’s cowl (Arisarum vulgare), great brome (Anisantha diandra), field marigold (Calendula arvensis), and foxtail restharrow (O. alopecuroides).
2.5 Statistical analysis of data
All statistical analyses were performed using R software (R version 4.2.1.) (R Core Team, 2023). To carry out an analysis of variance (ANOVA) on the studied variables, we first tested their normal distribution. The normality of the variables was tested using the Shapiro–Wilk test (Shapiro and Wilk, 1965). For the variables that did not follow normality, the adequate transformation was tested using Log10, arcsinh, square root, cube root, square, and cube transformations and the Shapiro–Wilk test after transformation. Furthermore, we used Levene’s test (Levene, 1960) to check the homogeneity of variance. The details and results regarding Shapiro–Wilk and Levene’s tests and variable transformation are given in Supplementary Tables S4–S7. Variables respecting the criteria of normality and homogeneity of variance were subjected to ANOVA, and the means were compared by Šidák’s test (Šidák, 1967). The linear mixed effect (lme) function of the package nlme (Pinheiro et al., 2024) was used to determine the effects of tillage and crop on soil properties and weed density.
When we did not find an adequate transformation for a variable, the concerned variable was subject to the Scheirer–Ray–Hare test (Scheirer et al., 1976), a two-way non-parametric ANOVA (Peng et al., 2023), using the R package rcompanion (Mangiafico, 2024). When the Scheirer–Ray–Hare test resulted in significant differences, the Dunn test (Dunn, 1965) with Holm corrections was conducted using the R package FSA (Ogle et al., 2023). The significance level of all statistical tests was set at 0.05.
3 Results
3.1 Effect of tillage on soil physical properties
The effect of tillage on the soil physical properties is shown in Figures 3, 4; in the soil evaluation in year 1 (November 2021), only FC and AWC were significantly (p ≤ 0.05) influenced by tillage. FC was significantly higher in continuous NT treatments (NT + residue and NT-residue) than in deep OT at 0–10 and 10–20 cm soil depths in year 1 (Figure 3). As for AWC, it was significantly lower under deep OT than that in NT + residue and NT-residue at 0–10 cm. At 10–20 cm, deep OT had a significantly lower AWC than NT-residue, while shallow OT and NT + residue had intermediate values between those of deep OT and NT-residue. Two years after OT implementation (November 2022), TP at 10–20 cm soil depth was significantly lower under shallow OT than that in NT + residue, while WSAs at 0–10 cm were significantly higher in both shallow and deep OT than NT + residue (Figure 4). For all the years of soil evaluation, BD and PWP were not significantly affected by tillage treatments (Supplementary Table S8).
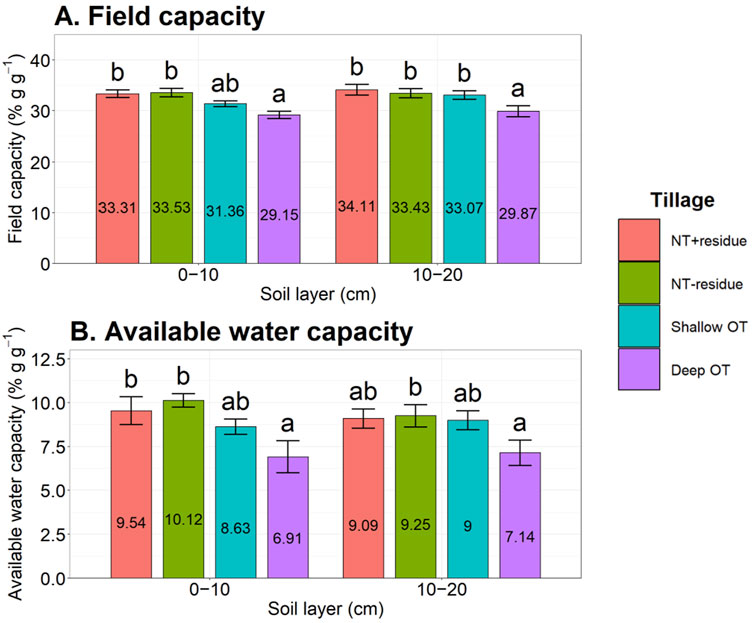
Figure 3. Significant (p ≤ 0.05) effect of tillage treatments on field capacity (A) and available water capacity (B) at 0–10 and/or 10–20 cm depths 1 year after OT application. Error bars represent the standard errors. Within the same subgraph (A,B) and soil depth, lower-case letters indicate whether the means of tillage treatments are significantly different (different letters) or similar (at least one letter in common) according to Sidak’s test.
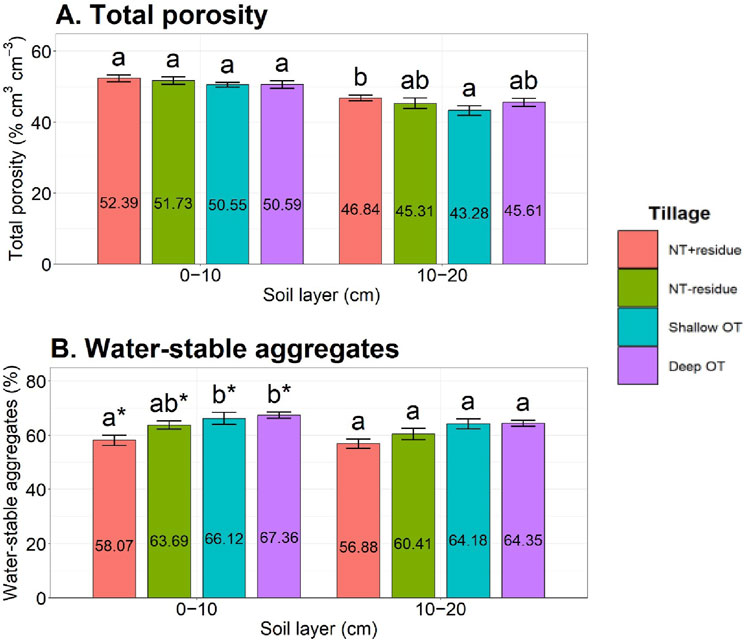
Figure 4. Significant (p ≤ 0.05) effect of tillage treatments on total porosity (A) and water-stable aggregates (B) at 0–10 and/or 10–20 cm depths 2 years after OT application. Error bars represent the standard errors. Within the same subgraph (A,B) and soil depth, lower-case letters without * indicate whether the means of tillage treatments are significantly different (different letters) or similar (at least one letter in common) according to Sidak’s test; lower-case letters with * indicate whether the means of tillage treatments are significantly different (different letters) or similar (at least one letter in common) according to Dunn’s test, following the non-parametric Scheirer–Ray–Hare test.
3.2 Effect of tillage on soil chemical properties
In year 1, CEC, NH4-N, and exchangeable K were the only soil chemical properties significantly affected by tillage (Figure 5). CEC under deep OT was significantly lower than in NT-residue and NT + residue at 0–10-cm and 10–20 cm soil depths, respectively. Soil NH4-N at both 0–10 and 10–20 cm soil depths was significantly higher in deep OT than that in NT + residue. Exchangeable K was significantly lower in deep OT than that in NT-residue at 10–20 cm depth, while it was slightly lower in OT treatments (shallow and deep OT) than in continuous NT treatments (NT + residue and NT-residue) at 0–10 cm.
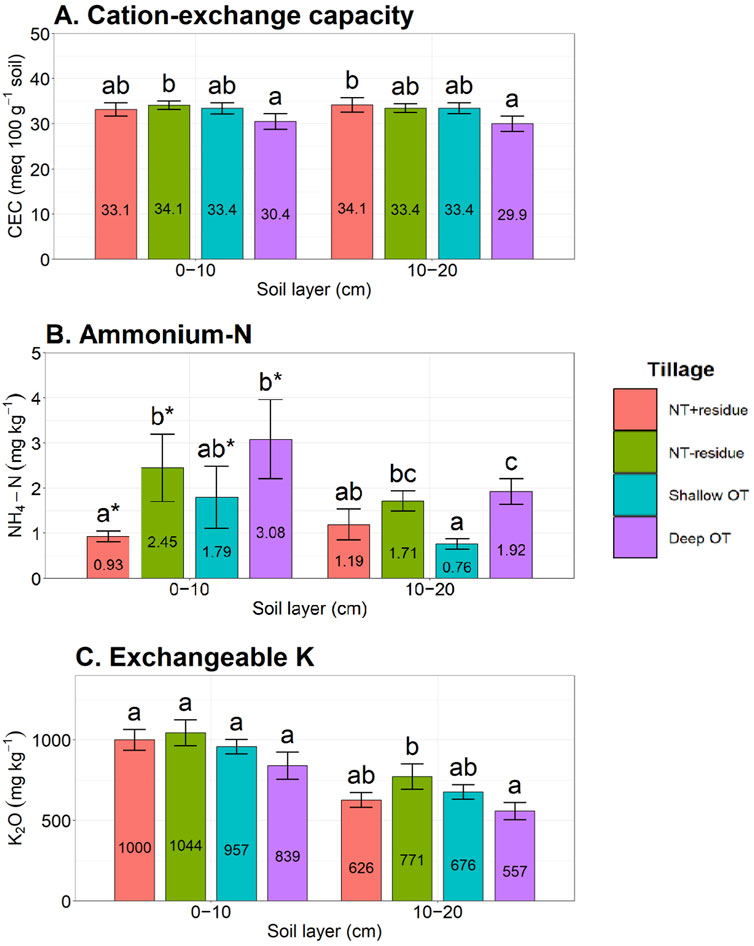
Figure 5. Significant (p ≤ 0.05) effect of tillage treatments on cation-exchange capacity (A), ammonium–nitrogen (B), and exchangeable potassium (C) at 0–10 and/or 10–20 cm depths 1 year after OT application. Error bars represent the standard errors. Within the same subgraph (A–C) and soil depth, lower-case letters without * indicate whether the means of tillage treatments are significantly different (different letters) or similar (at least one letter in common) according to Sidak’s test; lower-case letters with * indicate whether the means of tillage treatments are significantly different (different letters) or similar (at least one letter in common) according to Dunn’s test, following the non-parametric Scheirer–Ray–Hare test.
The soil evaluation results in year 2 showed that CEC, NO3-N, and available S were the sole soil chemical properties affected by tillage (Figure 6). In year 2, soil CEC at 10–20 cm soil depth was still significantly lower under deep OT (and NT-residue) than in NT + residue. Both NO3-N and available S at 10–20 cm soil depth were significantly higher in deep OT than in NT-residue 2 years post-tillage.
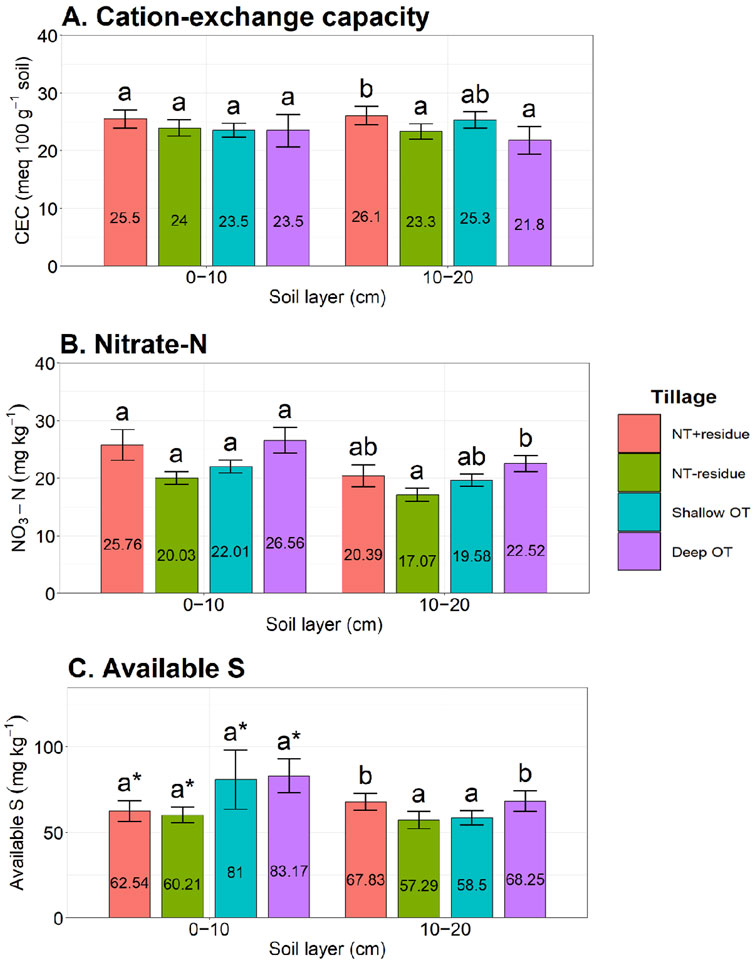
Figure 6. Significant (p ≤ 0.05) effect of tillage treatments on cation-exchange capacity (A), nitrate–nitrogen (B), and available sulfur (C) at 0–10 and/or 10–20 cm depths 2 years after OT application. Error bars represent the standard errors. Within the same subgraph (A–C) and soil depth, lower-case letters without * indicate whether the means of tillage treatments are significantly different (different letters) or similar (at least one letter in common) according to Sidak’s test; lower-case letters with * indicate whether the means of tillage treatments are significantly different (different letters) or similar (at least one letter in common) according to Dunn’s test, following the non-parametric Scheirer–Ray–Hare test.
For all the years, SOM (i.e., the pivot of soil health), pH, EC, total N, mineral N, available P, and exchangeable Ca and Mg were not significantly affected by tillage treatments (Supplementary Table S9). However, in the evaluation in year 1 and 0–10 cm soil depth, available P and exchangeable K, Ca, and Mg were slightly higher in continuous NT treatments (NT + residue and NT-residue) than in OT treatments (shallow and deep OT), while SOM was slightly higher in NT + residue than in OT treatments (Supplementary Table S9). The stratification ratio of all soil nutrients studied did not vary significantly between OT treatments (shallow and deep OT) and continuous NT treatments (NT + residue and NT-residue), irrespective of the year of soil evaluation (Supplementary Table S9). The stratification ratio of available P and exchangeable K was high for all the years of soil evaluation.
3.3 Effect of tillage on weed density
In year 1, tillage had a significant effect on weed density measured at 48 DAS, but no significant effect was observed at 111 and 132 DAS (Table 1). Weed density under deep OT was significantly lower than in NT + residue at 48 DAS (i.e., 50 days after OT implementation) and slightly lower than in NT + residue at 111 and 132 DAS. On the other hand, weed density at 48, 111, and 132 DAS was slightly lower under shallow OT and NT-residue than in NT + residue. Chemical and manual weed control operations conducted between 81 and 122 DAS in the crops of the experiment (Supplementary Table S2) contributed to reducing weed densities to a certain extent, as revealed mainly by the decrease in weed density at 132 DAS.
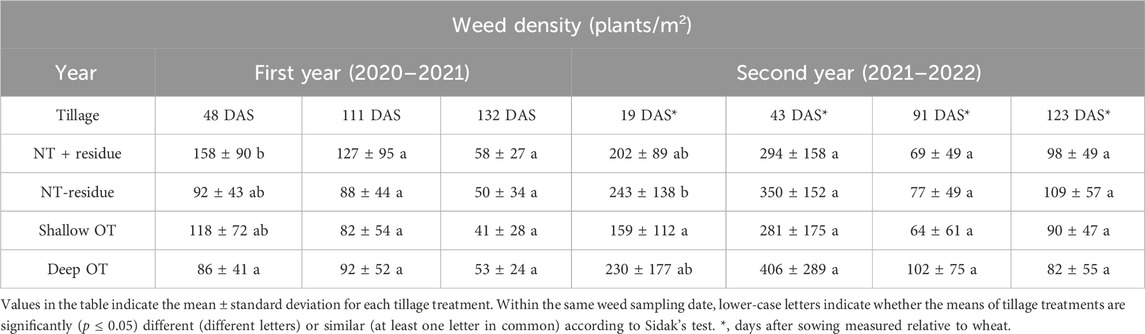
Table 1. Variation in weed density between tillage treatments at different weed sampling dates during the growing seasons 2020–2021 (year 1) and 2021–2022 (year 2).
In year 2, at 19 days after wheat sowing (i.e., 406 days after OT implementation), weed density under shallow OT was significantly lower than in NT-residue and slightly lower than in NT + residue (Table 1). At 43, 91, and 123 days after (wheat) sowing, weed density did not significantly vary between tillage treatments despite shallow OT having slightly lower weed density than in NT treatments. For all weed sampling dates in year 2, weed density in deep OT was slightly higher than in NT + residue. The decrease in weed density in all tillage treatments between 43 and 91 days after wheat sowing was due to the chemical and manual weed control operations carried out between 46 and 90 days after wheat sowing in all crops (Supplementary Table S2).
The results of weed density in years 1 and 2 show that weed density under shallow OT was slightly lower than in NT + residue for all years and weed sampling dates, while deep OT had lower weed density than in NT + residue only in year 1.
3.4 Effect of faba bean vs. chickpea on soil nitrogen, other soil properties, and weed infestation
The soil evaluation in year 1 shows that both soil NO3-N and mineral N were significantly higher in faba bean plots than in chickpea plots at both 0–10 and 10–20 cm soil depths (Figure 7), while total N and NH4-N were unaffected by the crop type. Furthermore, the soil sampling in year 2 shows that wheat grown after faba bean (wheat/faba bean) recorded higher soil residual NO3-N and mineral N at 10–20 cm than wheat grown after chickpea (wheat/chickpea) (Figure 8). Similarly, in year 2, faba bean following wheat (faba bean/wheat) led to slightly higher (+16%) mineral N at 10–20 cm than chickpea following wheat (Figure 8).
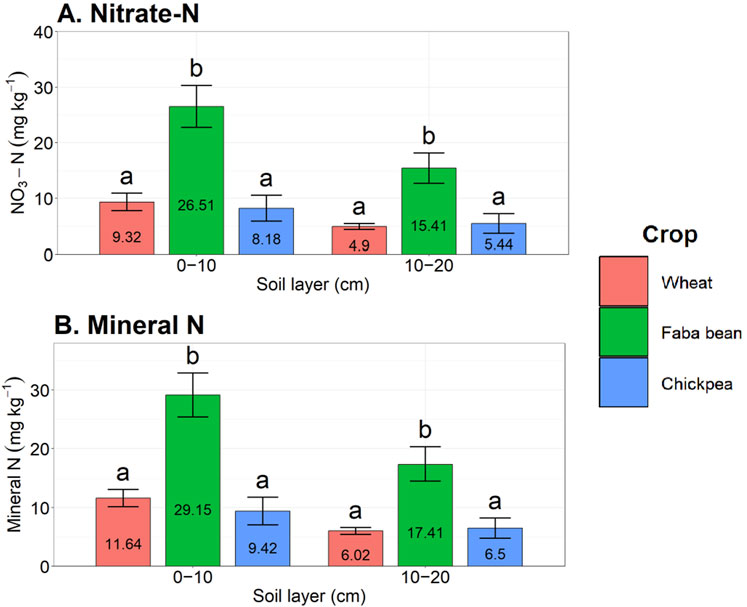
Figure 7. Significant (p ≤ 0.05) effect of crop types on nitrate–nitrogen (A) and mineral nitrogen (B) at 0–10 and/or 10–20 cm depths after the growing season 2020–2021. Error bars represent the standard errors. Within the same subgraph (A,B) and soil depth, lower-case letters indicate whether the means of crop types are significantly different (different letters) or similar (at least one letter in common) according to Sidak’s test.
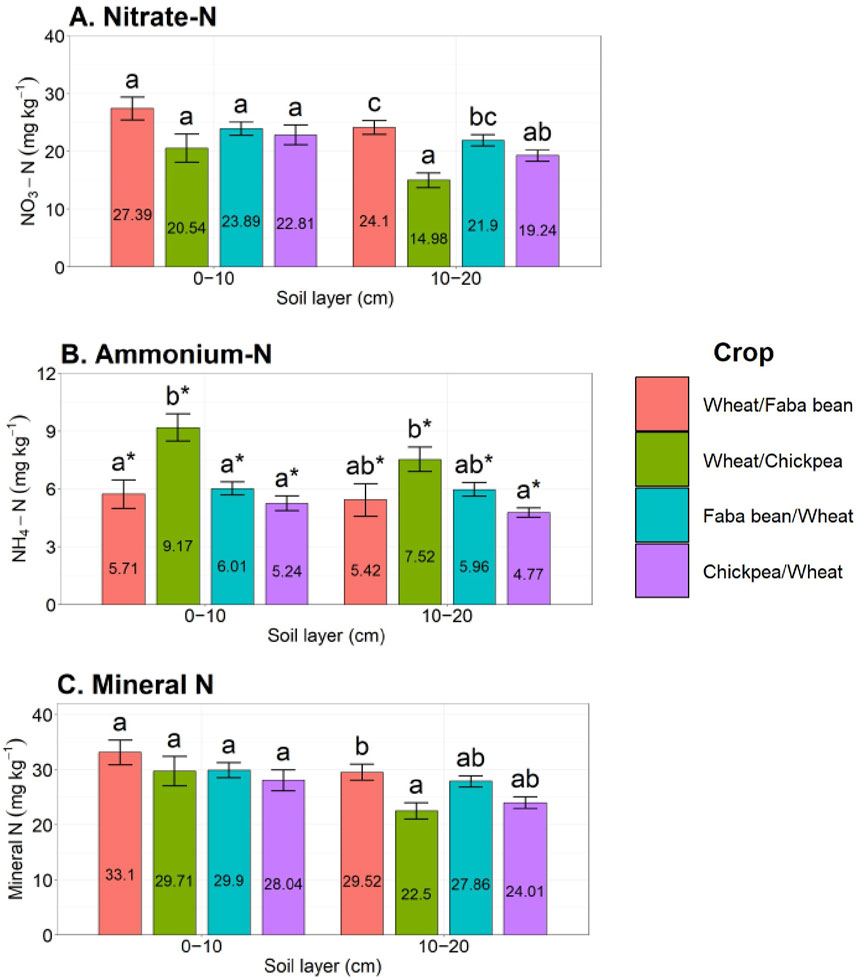
Figure 8. Significant (p ≤ 0.05) effect of crop types on nitrate–nitrogen (A), ammonium–nitrogen (B), and mineral nitrogen (C) at 0–10 and/or 10–20 cm depths after the growing season 2021–2022. Error bars represent the standard errors. Within the same subgraph (A–C) and soil depth, lower-case letters without * indicate whether the means of crop types are significantly different (different letters) or similar (at least one letter in common) according to Sidak’s test; lower-case letters with * indicate whether the means of tillage treatments are significantly different (different letters) or similar (at least one letter in common) according to Dunn’s test, following the non-parametric Scheirer–Ray–Hare test.
However, the soil CEC measured at both 0–10 and 10–20 cm in year 1 was significantly higher in chickpea plots than in faba bean plots (Supplementary Table S11). Similarly, in year 2, wheat/faba bean plots had lower CEC and lower available S at both 0–10 and 10–20 cm than wheat/chickpea plots (Supplementary Table S11). In year 1, the crop type did not have a significant effect on the soil physical properties studied at all soil depths (Supplementary Table S12). In year 2, soil physical properties did not vary significantly between faba bean/wheat and chickpea/wheat on one hand and between wheat/faba bean and wheat/chickpea on the other hand (Supplementary Tables S11, S12). The stratification ratio of soil nutrients did not significantly vary between crop types for all the years (Supplementary Table S13).
In year 1, for all weed density measurement dates, the crop type did not have a significant effect on weed density despite the fact that weed density was slightly lower in faba bean than in chickpea at 48 and 132 DAS (Table 2). In year 2, crop rotation had a significant effect on weed density at all weed sampling dates (Table 2). At 19 days after wheat sowing, weed density in wheat/faba bean was significantly lower than in wheat/chickpea and slightly lower in faba bean/wheat than in chickpea/wheat. The same trend was observed at 43, 91, and 123 days after wheat sowing during year 2.
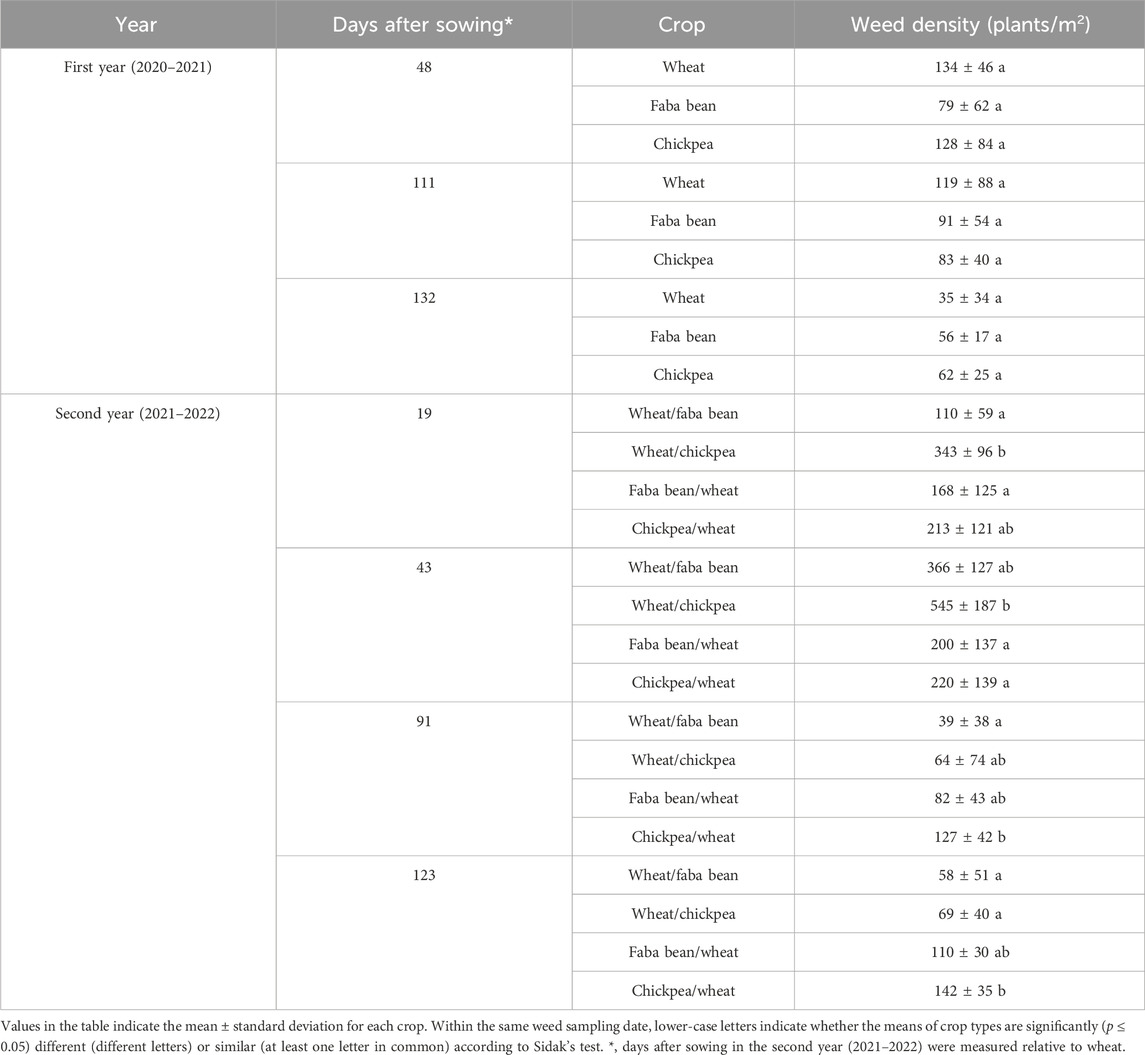
Table 2. Variation in weed density between crop types at different weed sampling dates during the growing seasons 2020–2021 (year 1) and 2021–2022 (year 2).
4 Discussion
4.1 Effect of occasional tillage on soil physical and chemical properties
Our results regarding the negative effects of deep OT on field capacity and AWC in year 1 are in agreement with those obtained by Çelik et al. (2019), who found under Mediterranean conditions (Türkiye) that moldboard-based OT significantly reduced water content at field capacity and plant available water at 0–20 cm soil depth compared to NT. Soil water-retention characteristics, including FC and PWP, are reported to be influenced by soil parameters like soil texture, soil organic matter, and soil structure (Saxton and Rawls, 2006). Continuous NT generally contributes to increasing water content at FC and AWC through enhanced SOM content and soil biological activity (Blanchy et al., 2023).
Knowing that there was no significant difference in SOM content between tillage treatments, the lower FC in deep OT than in continuous NT treatments could be attributed to a difference in pore-size distribution between these treatments even though the total porosity was not significantly affected by the tillage mode in year 1. Meso- and micro-porosity are considered the main drivers of water retention at FC (Çelik et al., 2019). Mesopores and micropores (0.5–50 μm) are defined as storage pores because they retain soil water, which is made available for plant roots and soil organisms (Salvo et al., 2014). No-till can increase the volume of smaller pores and decrease the volume of bigger pores, whereas tillage may favor the opposite behavior (Reichert et al., 2017). Pierce and Fortin (1996) found that OT had higher macroporosity than NT, while NT soil had higher microporosity. Díaz-Zorita et al. (2004) reported in a wheat crop that the percentage of mesopores was 23% higher in NT soils than that in soils tilled once every 2 years, which resulted in better water storage in NT.
In year 2, the lower total porosity at 10–20 cm under shallow OT (10 cm depth only) than in NT + residue after crop harvest can be attributed to a slight increase in BD at 10–20 cm in shallow OT compared to NT + residue (Supplementary Table S8). The increase in WSAs at the 0–10 cm depth in the OT treatments compared to NT + residue in year 2 could be caused by the action of the cementing agents to bind the soil particles. The cementing agents are plant roots, SOM, clay and biological agents such as fungal hyphae (Abbas et al., 2021). Knowing that the soil in our trial has an increasing gradient of clays content with soil depth, OT could have brought some clay particles from sub-surface soil layers to bind with SOM deposited on the topsoil during the evaluated seasons. In particular, deep OT could have brought clay particles from the B horizon situated at a depth of 10 cm (Supplementary Table S1). Several studies demonstrated the importance of primary soil particles (clay, silt, and sand) as the first factor controlling micro-aggregate formation in hierarchical aggregate formation (Six et al., 2004; Wagner et al., 2007). The soil content in glomalin, which contributes to stabilizing soil aggregates (Wright and Upadhyaya, 1998; Rillig, 2004; Vlček and Pohanka, 2020), could also explain the difference in WSAs between OT treatments and NT + residue. Labuschagne et al. (2020) found that OT with deep tine significantly increased glomalin content compared to continuous NT.
There is a huge gap in the literature regarding the effect of OT on soil CEC even though tillage is known as a factor that can influence soil CEC (Salinas-Garcia et al., 1997; Ma et al., 2024). Clay (amount and type), SOM, and pH are the major soil parameters affecting CEC (Bortoluzzi et al., 2006; Emamgolizadeh et al., 2015; Purnamasari et al., 2021). The lower CEC under deep OT at 0–10 and 10–20 cm in year 1 could be attributed to the effect of pH mainly. In year 1, CEC and pH were significantly and positively correlated at both 0–10 cm (r = 0.77) and 10–20 cm (r = 0.80) soil depths (Supplementary Figures S1, S2); soil pH under deep OT was slightly lower than under NT-residue and NT + residue at 0–10 and 10–20 cm, respectively (Supplementary Table S9). Increased pH can increase soil CEC through the increase in pH-dependent charges from both organic and inorganic origins (Curtin and Smillie, 1979; Foth, 1991). Many authors (Krogh et al., 2000; Zhang et al., 2015; Ibrahim et al., 2022) have found a significant and positive correlation between soil pH and CEC.
In year 2, soil CEC at 10–20 cm, which was lower under deep OT than in NT + residue, was significantly and positively correlated to both pH (r = 0.32) and SOM (r = 0.32) (Supplementary Figure S4); soil pH and SOM were slightly lower in deep OT than in NT + residue at 10–20 cm soil depth (Supplementary Table S9). Soil organic matter is a well-known major determinant of soil CEC through the presence of negatively charged groups (e.g., carboxyl and phenolic groups) providing 30%–60% of the adsorption sites for cations (Yang et al., 2024).
The significantly (p ≤ 0.05) lower content of exchangeable K in deep OT than in NT-residue and the slightly lower contents of available P and exchangeable Ca and Mg at 0–10 cm in OT treatments than in continuous NT treatments 1 year after OT could be due to a certain dilution of these nutrients in the tilled zone. Another hypothesis is that OT could have led to greater bioavailability and uptake of these nutrients by the crops. Regarding the higher soil ammonium-N (NH4-N) at 0–10 and 10–20 cm depths in deep OT than those in NT + residue 1 year after OT, it can be explained by two hypotheses: (1) higher mineralization of soil organic N in deep OT than in NT + residue and (2) a higher biological N fixation (i.e., conversion by diazotrophs of N2 gas from the atmosphere into NH4+ (Zhu et al., 2023)) by the legume crops (faba bean and chickpea) in deep OT than in NT + residue through better development and activity of legume nodules and/or N-fixing bacteria in deep OT. The higher content of soil NO3-N and available S at 10–20 cm depth under deep OT than under NT-residue 2 years after OT, beyond the hypotheses of higher mineralization of organic N and S in deep OT or higher N fixation by faba bean and chickpea in deep OT for the case of NO3-N, may be due to an increased downward movement of NO3-N and available S under deep OT through an increased macroporosity in the topsoil.
The absence of any significant effect of OT on BD, PWP, SOM, pH, EC, total N, mineral N, available P, and exchangeable Ca and Mg and soil nutrient stratification in all dates of soil evaluation can be explained by the low aggressiveness of the tillage tools used in our experiment (offset disk and chisel) compared with the most common tillage tool, i.e., the moldboard plow (Acir et al., 2020). These results are consistent with those obtained by Liu et al. (2016b), who found that BD, pH, EC, and available P were not statistically affected by OT, regardless of the time of OT application. Díaz-Zorita et al. (2004) observed that regardless of soil depth, soil BD, total SOC, pH, total N, and soil test P were similar between NT and OT, both 8 and 20 months after the tillage operation. Dang et al. (2018) found that BD in the topsoil was not significantly affected by OT both 3 and 24 months after implementing a single OT operation and no significant effect of OT on total SOC after 3, 12, and 24 months after tillage operation. The insignificant effect of OT on SOM and soil pH is also in line with the results obtained by Baan et al. (2009) and Obour et al. (2021). Our result regarding the insignificant effect of OT on the stratification of soil nutrients is consistent with the findings obtained by Garcia et al. (2007), who observed that one-time OT using a chisel or disk did not contribute to effectively redistributing soil nutrients.
4.2 Effect of occasional tillage on weeds
In years 1 and 2, weed population at the early crop growth stages (48 DAS for year 1 and 19 days after wheat sowing for year 2) shows that deep OT and shallow OT contributed to reducing weed density compared to NT + residue and NT-residue, respectively (Table 1). These results can be attributed to an alteration in the spatial distribution of the soil weed seedbank by OT treatments. In continuous NT, weed seeds tend to concentrate on the soil surface due to low soil disturbance (Chauhan et al., 2006), while tillage affects the vertical and horizontal distribution of weed seeds in the soil (Chauhan et al., 2012). OT treatments (shallow and deep OT) may have reduced weed germination and emergence through burial in the soil of weed seeds initially concentrated on the soil surface. The deeper tillage contributes to burying the weed seeds, initially present on the first centimeters of the soil, and the more effective it is in preventing their further development (Douglas and Peltzer, 2004). This could explain the better performance of deep OT than that of shallow OT in terms of reducing weed density compared to NT + residue at 48 DAS in year 1.
Our results on the reduction in weed density by OT at early crop growth in years 1 and 2 are compatible with those obtained by Crawford et al. (2015), who found a significant reduction in weed populations 3 months after the implementation of a single tillage including chisel tine, offset disk, and prickle/disk chain at 20 cm depth in NT. Dang et al. (2018) reported that introducing OT reduced weed populations in the first year after tillage. Obour et al. (2021) found that weed density was significantly lower in OT than in long-term NT and that OT significantly contributed to controlling herbicide-resistant weeds in their study.
As for the slight weed density reduction in NT-residue compared to NT + residue in year 1 (Table 1), it is possible that removing straw in NT (NT-residue) increased the rate of weed seed decay and seed predation, while in NT + residue, weed seeds may be protected by crop residues and less prone to desiccation. No-till can lead to low weed-seedling establishment due to the desiccation of seeds or predation activity of insects on or near the soil surface (Chauhan et al., 2006). Weed seed granivory, whether it is carried out by vertebrate or invertebrate organisms, is reported to play a significant role in the population dynamics of weed species (Law et al., 2023).
The slightly higher weed density in deep OT than in NT + residue in year 2 is probably due to the short duration (≤ 1 year) of the effects of deep OT (chisel) on weed density. The persistent effect of shallow OT (offset disk harrow) on weed density till the second year could be attributed to the fact that it inverted the soil, burying weed seeds more homogeneously in the soil. Nevertheless, in terms of burying weed seeds, OT using both an offset disk harrow and chisel is reported to be less effective than moldboard-OT (Blanco-Canqui and Wortmann, 2020), which yields inversion tillage >30 cm that buries weed seeds at an unfavorable depth for emergence after germination (Kettler et al., 2000; Douglas and Peltzer, 2004; Renton and Flower, 2015). The comparable levels of weed between NT and OT treatments at some weed density sampling dates could be explained, beyond the low aggressiveness of shallow and deep OT, by the fact that the concentration of weed seeds on the soil surface in NT can lead to unfavorable weed seed germination due to poor weed seed and soil contact (Cordeau et al., 2020), which may limit weed development under NT.
4.3 Effect of faba bean vs. chickpea on soil nitrogen and weed infestation
The higher soil NO3-N and mineral N following the growth of faba bean than that of chickpea could be explained by the better performance of faba bean in terms of biological N fixation. Faba bean is reported to fix more N, have a higher dependence on N2 fixation for growth, and substantially use a lower amount of soil N than chickpea under the same soil N supply (Turpin et al., 2002; Jensen et al., 2010). The % N derived from the atmosphere for faba bean and chickpea is reported to be 68–75 and 63–65, respectively. As for the lower weed density under faba bean, it can be attributed to the higher competitiveness against weeds of this crop than that of chickpea. Morphological traits of faba bean, such as the higher height and more vigorous early growth of the plant, contribute to higher shading capacity and weed suppression than those of chickpea (Frenda et al., 2013), which develops slowly and has an open and short canopy architecture, reducing its competitiveness against weeds (Avola et al., 2008).
5 Conclusion and perspectives
The present study shows that the implementation of occasional tillage in a long-term no-till system affected FC, AWC, CEC, exchangeable K, and NH4-N 1 year after OT and TP, WSAs, NO3-N, and available S 2 years after OT. However, the majority (10 out of 19) of soil properties evaluated in our study were not significantly influenced by OT, which was attributed to the low severity of the tillage implements used.
One year after OT application, deep OT significantly reduced the FC and AWC of the soil at 0–10 cm soil depth, compared with continuous NT treatments (NT + residue and NT-residue). At 10-20 cm depth, FC was significantly lower in deep OT compared to continuous NT treatments while AWC in deep OT was significantly and slightly lower than in NT-residue and NT + residue, respectively. Under deep OT, the soil CEC was lower than in NT-residue and NT + residue at 0–10 cm and 10–20 cm soil depths, respectively. Deep OT reduced exchangeable K compared to NT-residue at 10–20 cm soil depth and slightly decreased available P and exchangeable Ca and Mg at 0–10 cm. However, still 1 year after OT, deep OT increased soil NH4-N at both 0–10 and 10–20 cm depths compared to NT + residue.
Two years after OT application, TP at 10–20 cm under shallow OT (10 cm depth only) was lower than that in NT + residue. Furthermore, both shallow and deep OT had higher WSAs at 0–10 cm relative to NT + residue. In year 2, soil CEC at 10–20 cm was lower under deep OT than in NT + residue. Two years after OT, deep OT increased soil NO3-N and available sulfur (S) at 10–20 cm compared to NT-residue. However, in terms of nutrient management, OT did not reduce the stratification ratio of soil nutrients under continuous NT, which was high for available P and exchangeable K at both 1 and 2 years after OT implementation.
On the other hand, OT treatments helped reduce weed densities compared with NT + residue through the burial of weed seeds in the soil. These results could suggest that the OT using a chisel/an offset disk harrow can be associated with traditional chemical (or manual) weed control methods in NT systems into an integrated weed management (IWM) approach. However, our study revealed that the positive effect of deep OT using a chisel to reduce weed density compared to NT was limited to the first year following the application of OT, while the effect of shallow OT using an offset disk harrow on weed density was persistent till the second year.
Furthermore, our study revealed that faba bean appeared to be the better preceding or following crop of wheat for higher residual soil NO3-N and mineral N mainly at 10–20 cm depth and lower weed infestation than chickpea. This is a major finding in terms of nitrogen and weed management in wheat-based cropping systems where faba bean and chickpea are used in rotation with wheat.
The results of this study suggest a low but non-negligible effect of OT on soil properties in the short term and the potential of integrating OT in long-term NT as a lever of weed management. Farmers willing to mitigate soil or weed problems related to long-term NT systems should consider whether tillage will cause soil water depletion, especially in drylands, where water is the main factor limiting crop yield and biomass production. This study is the first investigation of occasional tillage conducted in North Africa, contributing to a better understanding of its effects on soil properties and weed dynamics. However, further studies are needed in other pedoclimatic conditions.
Data availability statement
The raw data supporting the conclusion of this article will be made available by the authors, without undue reservation.
Author contributions
MD: writing–original draft, writing–review and editing, investigation, methodology, software, and visualization. ADB: writing–review and editing, funding acquisition, investigation, methodology, resources, supervision, and validation. HC: writing–review and editing, conceptualization, funding acquisition, investigation, methodology, project administration, resources, supervision, and validation. HO: writing–review and editing, conceptualization, investigation, methodology, resources, supervision, and validation. OE: writing–review and editing, conceptualization, investigation, methodology, resources, supervision, and validation. AT: writing–review and editing, conceptualization, investigation, methodology, resources, supervision, and validation. AHB: writing–review and editing, conceptualization, investigation, methodology, resources, supervision, and validation. RD: writing–review and editing, conceptualization, investigation, methodology, resources, supervision, and validation. AZ: writing–review and editing, conceptualization, investigation, methodology, resources, and validation. ME: writing–review and editing, funding acquisition, investigation, methodology, resources, supervision, and validation. KE: writing–review and editing, funding acquisition, investigation, methodology, resources, supervision, and validation.
Funding
The author(s) declare that financial support was received for the research, authorship, and/or publication of this article. This research was funded by the Agricultural Innovation and Technology Transfer Center (AITTC), University Mohammed VI Polytechnic (UM6P), and the ConServeTerra project (a Research and Innovation project financed by PRIMA (prima-med.org) under Grant No. 1913).
Acknowledgments
The authors acknowledge the “Société L’Ouest Marocain” and thank the Zine El Abidine family, the Agriculture, Environment, and Development for the Avenir (“AGENDA”) research team, and the AITTC researchers and staff.
Conflict of interest
The authors declare that the research was conducted in the absence of any commercial or financial relationships that could be construed as a potential conflict of interest.
Publisher’s note
All claims expressed in this article are solely those of the authors and do not necessarily represent those of their affiliated organizations, or those of the publisher, the editors, and the reviewers. Any product that may be evaluated in this article, or claim that may be made by its manufacturer, is not guaranteed or endorsed by the publisher.
Supplementary material
The Supplementary Material for this article can be found online at: https://www.frontiersin.org/articles/10.3389/fenvs.2024.1431822/full#supplementary-material
References
Abbas, F., Zhu, Z., and An, S. (2021). Evaluating aggregate stability of soils under different plant species in Ziwuling Mountain area using three renowned methods. Catena 207, 105616. doi:10.1016/j.catena.2021.105616
Acir, N., Günal, H., Çelik, İ., Barut, Z. B., Budak, M., and Kılıç, Ş. (2020). Effects of long-term conventional and conservational tillage systems on biochemical soil health indicators in the Mediterranean region. Arch. Agron. Soil Sci. 68, 795–808. doi:10.1080/03650340.2020.1855327
Asefa, T. W. (2001). Effects of stubble management, tillage and cropping sequence on sustainable wheat production in the south-eastern highlands of Ethiopia. Ph.D. Thesis. Bloemfontein, South Africa: University of the Free State.
Avola, G., Tuttobene, R., Gresta, F., and Abbate, V. (2008). Weed control strategies for grain legumes. Agron. Sustain. Dev. 28, 389–395. doi:10.1051/agro:2008019
Baan, C. D., Grevers, M. C. J., and Schoenau, J. J. (2009). Effects of a single cycle of tillage on long-term no-till prairie soils. Can. J. Soil Sci. 89, 521–530. doi:10.4141/cjss08041
Beniaich, A., Otten, W., Shin, H. C., Cooper, H. V., Rickson, J., Soulaimani, A., et al. (2023). Evaluation of pedotransfer functions to estimate some of soil hydraulic characteristics in North Africa: a case study from Morocco. Front. Environ. Sci. 11, 1–17. doi:10.3389/fenvs.2023.1090688
Blake, G. R., and Hartge, K. H. (1986a). “Bulk density,” in Methods of soil analysis. Part I: physical and mineralogical method. Editor A. Klute (USA: American Society of Agronomy, Inc. Soil Science Society of America, Inc.), 363–375. (South Segoe Road, Madison, WI 53711.
Blake, G. R., and Hartge, K. H. (1986b). “Particle density,” in Methods of soil analysis. Part I: physical and mineralogical method. Editor A. Klute (USA: American Society of Agronomy, Inc. Soil Science Society of America, Inc.), 377–382. (South Segoe Road, Madison, WI 53711.
Blanchy, G., Bragato, G., Di Bene, C., Jarvis, N., Larsbo, M., Meurer, K., et al. (2023). Soil and crop management practices and the water regulation functions of soils: a qualitative synthesis of meta-analyses relevant to European agriculture. Soil 9, 1–20. doi:10.5194/soil-9-1-2023
Blanco-Canqui, H., and Ruis, S. J. (2018). No-tillage and soil physical environment. Geoderma 326, 164–200. doi:10.1016/j.geoderma.2018.03.011
Blanco-Canqui, H., and Wortmann, C. S. (2020). Does occasional tillage undo the ecosystem services gained with no-till? A review. Soil Tillage Res. 198, 104534. doi:10.1016/j.still.2019.104534
Bortoluzzi, E. C., Tessier, D., Rheinheimer, D. S., and Julien, J. L. (2006). The cation exchange capacity of a sandy soil in southern Brazil: an estimation of permanent and pH-dependent charges. Eur. J. Soil Sci. 57, 356–364. doi:10.1111/j.1365-2389.2005.00746.x
Çelik, İ., Günal, H., Acar, M., Acir, N., Bereket Barut, Z., and Budak, M. (2019). Strategic tillage may sustain the benefits of long-term no-till in a Vertisol under Mediterranean climate. Soil Tillage Res. 185, 17–28. doi:10.1016/j.still.2018.08.015
Chauhan, B. S., Gill, G., and Preston, C. (2006). Seedling recruitment pattern and depth of recruitment of 10 weed species in minimum tillage and no-till seeding systems. Weed Sci. 54, 658–668. doi:10.1614/ws-05-135r.1
Chauhan, B. S., Singh, R. G., and Mahajan, G. (2012). Ecology and management of weeds under conservation agriculture: a review. Crop Prot. 38, 57–65. doi:10.1016/j.cropro.2012.03.010
Conyers, M., van der Rijt, V., Oates, A., Poile, G., Kirkegaard, J., and Kirkby, C. (2019). The strategic use of minimum tillage within conservation agriculture in southern New South Wales, Australia. Soil Tillage Res. 193, 17–26. doi:10.1016/j.still.2019.05.021
Cordeau, S., Baudron, A., and Adeux, G. (2020). Is tillage a suitable option for weed management in conservation agriculture? Agronomy 10, 1746. doi:10.3390/agronomy10111746
R Core Team (2023). R: a language and environment for statistical computing. Available at: https://www.r-project.org/(Accessed June 15, 2024).
Crawford, M., Dang, Y., Balzer, A., Rincon-Florez, V., and Carvalhais, L. (2014). “Strategic Tillage in Conservation farming systems; its impact on soil health and productivity,” in Soil change matters, international workshop 24-27 march 2014, bendigo, victoria, Australia (East Melbourne, Australia: Department of Environment and Primary Industries), 33–38.
Crawford, M. H., Bell, K., Kodur, S., and Dang, Y. (2018). The influence of tillage frequency on crop productivity in sub-tropical to semi-arid climates. J. Crop Sci. Biotechnol. 21, 13–22. doi:10.1007/s12892-017-0044-0
Crawford, M. H., Rincon-Florez, V., Balzer, A., Dang, Y. P., Carvalhais, L. C., Liu, H., et al. (2015). Changes in the soil quality attributes of continuous no-till farming systems following a strategic tillage. Soil Res. 53, 263–273. doi:10.1071/SR14216
Curtin, D., and Smillie, G. W. (1979). Origin of the pH-dependent cation exchange capacities of Irish soil clays. Geoderma 22, 213–224. doi:10.1016/0016-7061(79)90020-x
Dang, Y. P., Balzer, A., Crawford, M., Rincon-Florez, V., Liu, H., Melland, A. R., et al. (2018). Strategic tillage in conservation agricultural systems of north-eastern Australia: why, where, when and how? Environ. Sci. Pollut. Res. 25, 1000–1015. doi:10.1007/s11356-017-8937-1
Dang, Y. P., Seymour, N. P., Walker, S. R., Bell, M. J., and Freebairn, D. M. (2015). Strategic tillage in no-till farming systems in Australia’s northern grains-growing regions: I. Drivers and implementation. Soil Tillage Res. 152, 104–114. doi:10.1016/j.still.2015.03.009
Derrouch, D., Chauvel, B., Felten, E., and Dessaint, F. (2020). Weed management in the transition to conservation agriculture: farmers’ response. Agronomy 10, 843. doi:10.3390/agronomy10060843
Díaz-Zorita, M., Grove, J. H., Murdock, L., Herbeck, J., and Perfect, E. (2004). Soil structural disturbance effects on crop yields and soil properties in a No-till production system. Agron. J. 96, 1651–1659. doi:10.2134/agronj2004.1651
Diop, M., Beniaich, A., Cicek, H., Ouabbou, H., Bamouh, A., El Gharras, O., et al. (2024). Short-term residual effects of occasional tillage on crop performance, soil water, and water-use efficiency in a 10-year no-till system under a dry Mediterranean climate. Front. Sustain. Food Syst. 8. doi:10.3389/fsufs.2024.1375666
Dobignard, A., and Chatelain, C. (2010). Index Synonymique de la Flore d’Afrique du Nord, Volume 1: Pteridophyta, Gymnospermae, Monocotyledoneae. Geneva, Switzerland: Conservatoire et jardin botaniques de la ville de Genève.
Dobignard, A., and Chatelain, C. (2011a). Index Synonymique de la Flore d’Afrique du Nord, Volume 2: Dicotyledoneae : Acanthaceae - Asteraceae Geneva, Switzerland: Conservatoire et jardin botaniques de la ville de Genève.
Dobignard, A., and Chatelain, C. (2011b). Index Synonymique de la Flore d’Afrique du Nord, Volume 3: Dicotyledoneae : Balsaminaceae - Euphorbiaceae Geneva, Switzerland: Conservatoire et jardin botaniques de la ville de Genève.
Dobignard, A., and Chatelain, C. (2012). Index Synonymique de la Flore d’Afrique du Nord, Volume 4: Dicotyledoneae : Fabaceae – Nymphaeaceae Geneva, Switzerland: Conservatoire et jardin botaniques de la ville de Genève.
Dobignard, A., and Chatelain, C. (2013). Index Synonymique de la Flore d’Afrique du Nord, Volume 5: Dicotyledoneae : Oleaceae – Zygophyllaceae Geneva, Switzerland: Conservatoire et jardin botaniques de la ville de Genève.
Douglas, A., and Peltzer, S. (2004). “Managing herbicide resistant annual ryegrass (Lolium rigidum Gaud.) in no-till systems in Western Australia using occasional inversion ploughing,” in Weed management — balancing people, planet. Fourteenth Australian weeds conference, 300–303. Available at: http://caws.org.au/awc/2004/awc200413001.pdf.
Dunn, O. J. (1965). Multiple comparisons using rank sums. Technometrics 6, 241–252. doi:10.1080/00401706.1964.10490181
Eijkelkamp Soil and Water (2018). Wet sieving apparatus. Manuel. Available at: https://geomor.com.pl/wp-content/uploads/2019/08/0813_Manual.pdf (Accessed February 1, 2024).1–6
Emamgolizadeh, S., Bateni, S. M., Shahsavani, D., Ashrafi, T., and Ghorbani, H. (2015). Estimation of soil cation exchange capacity using genetic expression programming (GEP) and multivariate adaptive regression splines (MARS). J. Hydrol. 529, 1590–1600. doi:10.1016/j.jhydrol.2015.08.025
Fennane, M., IbnTattou, M., Mathez, J., and El Oualidi, J. (2014). Flore pratique du Maroc, manuel de détermination des plantes vasculaires, Volume 3: Dicotyledones (p.p.), Monocotyledones Rabat, Morocco: Institut Scientifique, Université Mohammed V.
Fennane, M., IbnTattou, M., Mathez, J., Ouyahya, A., and El Oualidi, J. (1999). Flore pratique du Maroc, manuel de détermination des plantes vasculaires, Volume 1: Pteridophyta, Gymnospermae, Angiospermae (Lauraceae – Neuradaceae) Rabat, Morocco: Institut Scientifique, Université Mohammed V.
Fennane, M., IbnTattou, M., Mathez, J., Ouyahya, A., and El Oualidi, J. (2007). Flore pratique du Maroc, manuel de détermination des plantes vasculaires, Volume 2: Angiospermae (Leguminosae - Lentibulariaceae) Rabat, Morocco: Institut Scientifique, Université Mohammed V.
Fidalski, J., Yagi, R., and Tormena, C. A. (2015). Revolvimento Ocasional e Calagem em Latossolo Muito Argiloso em Sistema Plantio Direto Consolidado. Rev. Bras. Cienc. do Solo 39, 1483–1489. doi:10.1590/01000683rbcs20140428
Foth, H. D. (1991). Fundamentals of soil science. 8th edition. Hoboken, New Jersey, USA: John Wiley and Sons, Inc.
Franzluebbers, A. J. (2002). Soil organic matter stratification ratio as an indicator of soil quality. Soil Tillage Res. 66, 95–106. doi:10.1016/S0167-1987(02)00018-1
Frenda, A. S., Ruisi, P., Saia, S., Frangipane, B., Di Miceli, G., Amato, G., et al. (2013). The critical period of weed control in faba bean and chickpea in mediterranean areas. Weed Sci. 61, 452–459. doi:10.1614/ws-d-12-00137.1
Garcia, J. P., Wortmann, C. S., Mamo, M., Drijber, R., and Tarkalson, D. (2007). One-time tillage of no-till: effects on nutrients, mycorrhizae, and phosphorus uptake. Agron. J. 99, 1093–1103. doi:10.2134/agronj2006.0261
Gozubuyuk, Z., Sahin, U., Ozturk, I., Celik, A., and Adiguzel, M. C. (2014). Tillage effects on certain physical and hydraulic properties of a loamy soil under a crop rotation in a semi-arid region with a cool climate. Catena 118, 195–205. doi:10.1016/j.catena.2014.01.006
Grandy, A. S., Robertson, G. P., and Thelen, K. D. (2006). Do productivity and environmental trade-offs justify periodically cultivating no-till cropping systems? Agron. J. 98, 1377–1383. doi:10.2134/agronj2006.0137
Hadria, R., Benabdelouahab, T., Elmansouri, L., Gadouali, F., Ouatiki, H., Lebrini, Y., et al. (2019). Derivation of air temperature of agricultural areas of Morocco from remotely land surface temperature based on the updated Köppen-Geiger climate classification. Model. Earth Syst. Environ. 5, 1883–1892. doi:10.1007/s40808-019-00645-4
Hajjaj, B., Bouhache, M., Mrabet, R., Taleb, A., and Douaik, A. (2016). Efficacité de quelques herbicides des céréales dans une culture du blé tendre conduite en semis direct. Rev. Marocaine Des. Sci. Agron. Vétérinaires 4, 48–56.
Hall, D. J. M., Davies, S. L., Bell, R. W., and Edwards, T. J. (2020). Soil management systems to overcome multiple constraints for dryland crops on deep sands in a water limited environment on the south coast of western Australia. Agronomy 10, 1881. doi:10.3390/agronomy10121881
Haruna, S. I., Anderson, S. H., Nkongolo, N. V., and Zaibon, S. (2018). Soil hydraulic properties: influence of tillage and cover crops. Pedosphere 28, 430–442. doi:10.1016/s1002-0160(17)60387-4
Ibrahim, O. M., El-Gamal, E. H., Darwish, K. M., and Kianfar, N. (2022). Modeling main and interactional effects of some physiochemical properties of Egyptian soils on cation exchange capacity via artificial neural networks. Eurasian Soil Sci. 55, 1052–1063. doi:10.1134/S1064229322080051
Issah, G., Schoenau, J., and Knight, J. D. (2021). Landscape position, sampling time, and tillage, but not legume species, affect labile carbon and nitrogen fractions in a 4-yr-old rejuvenated grazed pasture. Can. J. Soil Sci. 101, 641–653. doi:10.1139/cjss-2021-0052
IUSS Working Group WRB (2022). World Reference Base for Soil Resources. International soil classification system for naming soils and creating legends for soil maps. 4th edition. Vienna, Austria: International Union of Soil Sciences IUSS.
Jensen, E. S., Peoples, M. B., and Hauggaard-Nielsen, H. (2010). Faba bean in cropping systems. F. Crop. Res. 115, 203–216. doi:10.1016/j.fcr.2009.10.008
Jin, K., White, P. J., Whalley, W. R., Shen, J., and Shi, L. (2017). Shaping an optimal soil by root–soil interaction. Trends Plant Sci. 22, 823–829. doi:10.1016/j.tplants.2017.07.008
Karunatilake, U. P., and Van Es, H. M. (2002). Rainfall and tillage effects on soil structure after alfalfa conversion to maize on a clay loam soil in New York. Soil Tillage Res. 67, 135–146. doi:10.1016/S0167-1987(02)00056-9
Kemper, W. D., and Rosenau, R. C. (1986). “Aggregate stability and size distribution,” in Methods of soil analysis. Part I: physical and mineralogical method. 2nd edition (American Society of Agronomy), 317–328. Chap. 17. doi:10.2136/sssabookser5.4.c14
Kettler, T. A., Lyon, D. J., Doran, J. W., Powers, W. L., and Stroup, W. W. (2000). Soil quality assessment after weed-control tillage in a No-till wheat-fallow cropping system. Soil Sci. Soc. Am. J. 64, 339–346. doi:10.2136/sssaj2000.641339x
Krogh, L., Breuning-Madsen, H., and Greve, M. H. (2000). Cation-exchange capacity pedotransfer functions for Danish soils. Acta Agric. Scand. - Sect. B Soil Plant Sci. 50, 1–12. doi:10.1080/090647100750014358
Labuschagne, J., van Zyl, J., Agenbag, G. A., Crous, I. R., and Hoffman, J. E. (2020). Effect of once-off tillage on soil water, inorganic nitrogen and glomalin contents under conservation agriculture in the Swartland sub-region of the Western Cape. South Afr. J. Plant Soil 37, 273–282. doi:10.1080/02571862.2020.1750069
Law, J. J., Gallagher, R. S., Leslie, T. W., and Weber, J. B. (2023). Enhanced invertebrate activity-densities and weed seed predation in an integrated cropping system. Basic Appl. Ecol. 68, 46–56. doi:10.1016/j.baae.2023.03.005
Levene, H. (1960). “Robust testes for equality of variances,” in Contributions to probability and statistics: essays in honor of harold hotelling. Editor I. Olkin (Redwood City, California, USA: Stanford University Press), 278–292.
Li, Y., Chang, S. X., Tian, L., and Zhang, Q. (2018). Conservation agriculture practices increase soil microbial biomass carbon and nitrogen in agricultural soils: a global meta-analysis. Soil Biol. biochem. 121, 50–58. doi:10.1016/j.soilbio.2018.02.024
Li, Y., Li, Z., Cui, S., and Zhang, Q. (2020). Trade-off between soil pH, bulk density and other soil physical properties under global no-tillage agriculture. Geoderma 361, 114099. doi:10.1016/j.geoderma.2019.114099
Liu, H., Carvalhais, L. C., Rincon-Florez, V., Crawford, M., Dang, Y. P., Dennis, P. G., et al. (2016a). One-time strategic tillage does not cause major impacts on soil microbial properties in a no-till Calcisol. Soil Tillage Res. 158, 91–99. doi:10.1016/j.still.2015.12.007
Liu, H., Crawford, M., Carvalhais, L. C., Dang, Y. P., Dennis, P. G., and Schenk, P. M. (2016b). Strategic tillage on a Grey Vertosol after fifteen years of no-till management had no short-term impact on soil properties and agronomic productivity. Geoderma 267, 146–155. doi:10.1016/j.geoderma.2016.01.002
Liu, Q., Kan, Z., He, C., and Zhang, H. (2020). Effects of strategic tillage on soil physicochemical properties and grain yield in the north China plain. Agronomy 10, 1167. doi:10.3390/agronomy10081167
López-Garrido, R., Madejón, E., Murillo, J. M., and Moreno, F. (2011). Soil quality alteration by mouldboard ploughing in a commercial farm devoted to no-tillage under Mediterranean conditions. Agric. Ecosyst. Environ. 140, 182–190. doi:10.1016/j.agee.2010.12.001
Ma, D., He, Z., Zhao, W., Li, R., Sun, W., Wang, W., et al. (2024). Long-term effects of conventional cultivation on soil cation exchange capacity and base saturation in an arid desert region. Sci. Total Environ. 949, 175075. doi:10.1016/j.scitotenv.2024.175075
MacLaren, C., Labuschagne, J., and Swanepoel, P. A. (2021). Tillage practices affect weeds differently in monoculture vs. crop rotation. Soil Tillage Res. 205, 104795. doi:10.1016/j.still.2020.104795
Mangiafico, S. (2024). Package‘rcompanion. Available at: https://cran.r-project.org/web/packages/rcompanion/index.html (Accessed July 31, 2024).
McConkey, T., Bulmer, C., and Sanborn, P. (2012). Effectiveness of five soil reclamation and reforestation techniques on oil and gas well sites in northeastern British Columbia. Can. J. Soil Sci. 92, 165–177. doi:10.4141/CJSS2010-019
McPheeters, D., Bruns, M. A., Karsten, H. D., and Dell, C. J. (2022). Integrated weed management with strategic tillage can maintain soil quality in continuous living cover systems. Front. Sustain. Food Syst. 6, 1–16. doi:10.3389/fsufs.2022.907590
Melero, S., Panettieri, M., Madejón, E., Macpherson, H. G., Moreno, F., and Murillo, J. M. (2011). Implementation of chiselling and mouldboard ploughing in soil after 8 years of no-till management in SW, Spain: effect on soil quality. Soil Tillage Res. 112, 107–113. doi:10.1016/j.still.2010.12.001
Mrabet, R. (2008). No-tillage systems for sustainable dryland agriculture in Morocco. Rabat, Morocco: INRA.
Mrabet, R., Moussadek, R., Fadlaoui, A., and van Ranst, E. (2012). Conservation agriculture in dry areas of Morocco. F. Crop. Res. 132, 84–94. doi:10.1016/j.fcr.2011.11.017
Nichols, V., Verhulst, N., Cox, R., and Govaerts, B. (2015). Weed dynamics and conservation agriculture principles: a review. F. Crop. Res. 183, 56–68. doi:10.1016/j.fcr.2015.07.012
Obour, A. K., Holman, J. D., Simon, L. M., and Schlegel, A. J. (2021). Strategic tillage effects on crop yields, soil properties, and weeds in dryland no-tillage systems. Agronomy 11, 662. doi:10.3390/agronomy11040662
Ogle, D. H., Doll, J. C., Wheeler, A. P., and Dinno, A. (2023). Package ‘FSA.’ R package version 0.9.4. Available at: https://cran.r-project.org/web/packages/FSA/index.html (Accessed July 31, 2024).
Olchin, G. P., Ogle, S., Frey, S. D., Filley, T. R., Paustian, K., and Six, J. (2008). Residue carbon stabilization in soil aggregates of No-till and tillage management of dryland cropping systems. Soil Sci. Soc. Am. J. 72, 507–513. doi:10.2136/sssaj2006.0417
Outbakat, M. B., Mejahed, K.El, Omari, K.El, and Beniaich, A. (2022) Effect of phosphogypsum on soil physical properties in Moroccan salt-affected soils, 14, 1–16.
Peng, M., He, H., Wang, X., Wang, Z., and Zhuang, L. (2023). Comparison of network connectivity and environmental driving factors of root-associated fungal communities of desert ephemeral plants in two habitat soils. J. Environ. Manage. 332, 117375. doi:10.1016/j.jenvman.2023.117375
Pierce, F. J., and Fortin, M.-C. (1996). “Long-term tillage and periodic plowing of a No-tilled soil in Michigan: impacts, yield, and soil organic matter,” in Soil organic matter in temperate agroecosystems long term experiments in North America. Editors E. A. Paul, K. H. Paustian, E. T. Elliott, and C. V. Cole (Boca Raton, Florida: CRC Press), 141–149. 33487, US). doi:10.1201/9780367811693-9
Pierce, F. J., Fortin, M.-C., and Staton, M. J. (1994). Periodic plowing effects on soil properties in a No-till farming system. Soil Sci. Soc. Am. J. 58, 1782–1787. doi:10.2136/sssaj1994.03615995005800060029x
Pinheiro, J., Bates, D., DebRoy, S., Sarkar, D., EISPACK authors, , and Heisterkamp, S. (2024). Package “nlme.”. doi:10.32614/CRAN.package.nlme
Purnamasari, L., Rostaman, T., Widowati, L. R., and Anggria, L. (2021). Comparison of appropriate cation exchange capacity (CEC) extraction methods for soils from several regions of Indonesia. IOP Conf. Ser. Earth Environ. Sci. 648, 012209. doi:10.1088/1755-1315/648/1/012209
Radford, B. J., and Thornton, C. M. (2011). Effects of 27 years of reduced tillage practices on soil properties and crop performance in the semi-arid subtropics of Australia. IJEEE 19, 1–22.
Reichert, J. M., Brandt, A. A., Rodrigues, M. F., da Veiga, M., and Reinert, D. J. (2017). Is chiseling or inverting tillage required to improve mechanical and hydraulic properties of sandy clay loam soil under long-term no-tillage? Geoderma 301, 72–79. doi:10.1016/j.geoderma.2017.04.012
Renton, M., and Flower, K. C. (2015). Occasional mouldboard ploughing slows evolution of resistance and reduces long-term weed populations in no-till systems. Agric. Syst. 139, 66–75. doi:10.1016/j.agsy.2015.06.005
Rillig, M. C. (2004). Arbuscular mycorrhizae, glomalin, and soil aggregation. Can. J. Soil Sci. 84, 355–363. doi:10.4141/S04-003
Salinas-Garcia, J. R., Matocha, J. E., and Hons, F. M. (1997). Long-term tillage and nitrogen fertilization effects on soil properties of an alfisol under dryland corn/cotton production. Soil Tillage Res. 42, 79–93. doi:10.1016/S0167-1987(96)01092-6
Salvo, L., Hernández, J., and Ernst, O. (2014). Soil organic carbon dynamics under different tillage systems in rotations with perennial pastures. Soil Tillage Res. 135, 41–48. doi:10.1016/j.still.2013.08.014
Saxton, K. E., and Rawls, W. J. (2006). Soil water characteristic estimates by texture and organic matter for hydrologic solutions. Soil Sci. Soc. Am. J. 70, 1569–1578. doi:10.2136/sssaj2005.0117
Scheirer, C. J., Ray, W. S., and Hare, N. (1976). The analysis of ranked data derived from completely randomized factorial designs. Biometrics 32, 429. doi:10.2307/2529511
Shapiro, S. S., and Wilk, M. B. (1965). An analysis of variance test for normality (complete samples). Biometrika 52, 591–611. doi:10.2307/2333709
Šidák, Z. (1967). Rectangular confidence regions for the means of multivariate normal distributions. J. Am. Stat. Assoc. 62, 626–633. doi:10.1080/01621459.1967.10482935
Six, J., Bossuyt, H., Degryze, S., and Denef, K. (2004). A history of research on the link between (micro)aggregates, soil biota, and soil organic matter dynamics. Soil Tillage Res. 79, 7–31. doi:10.1016/j.still.2004.03.008
Stavi, I., Lal, R., and Owens, L. B. (2011). On-farm effects of no-till versus occasional tillage on soil quality and crop yields in eastern Ohio. Agron. Sustain. Dev. 31, 475–482. doi:10.1007/s13593-011-0006-4
Stockfisch, N., Forstreuter, T., and Ehlers, W. (1999). Ploughing effects on soil organic matter after twenty years of conservation tillage in Lower Saxony, Germany. Soil Tillage Res. 52, 91–101. doi:10.1016/S0167-1987(99)00063-X
Tanji, A., and Boutfirass, M. (2018). Effective preemergence herbicides for rigid ryegrass (Lolium rigidum gaud.) control in irrigated bread wheat (Triticum aestivum L.). J. Agric. Sci. 10, 79–85. doi:10.5539/jas.v10n4p79
Tanji, A., and El Brahli, A. (2018). Weed surveys in rainfed canola (Brassica napus l.) fields in Chaouia, Morocco. Eur. J. Agric. For. Res. 6, 43–56. Available at: https://www.eajournals.org/wp-content/uploads/Weed-Surveys-in-Rainfed-Canola-Brassica-Napus-L.-Fields-in-Chaouia-Morocco.pdf.
Triplett, G. B., and Dick, W. A. (2008). No-tillage crop production: a revolution in agriculture. Agron. J. 100, 153–165. doi:10.2134/agronj2007.0005c
Turpin, J. E., Herridge, D. F., and Robertson, M. J. (2002). Nitrogen fixation and soil nitrate interactions in field-grown chickpea (Cicer arietinum) and fababean (Vicia faba). Aust. J. Agric. Res. 53, 599–608. doi:10.1071/AR01136
Vlček, V., and Pohanka, M. (2020). Glomalin – an interesting protein part of the soil organic matter. Soil Water Res. 15, 67–74. doi:10.17221/29/2019-SWR
Wagner, S., Cattle, S. R., and Scholten, T. (2007). Soil-aggregate formation as influenced by clay content and organic-matter amendment. J. Plant Nutr. Soil Sci. 170, 173–180. doi:10.1002/jpln.200521732
Wortmann, C. S., and Dang, Y. P. (2020). “Strategic tillage for the improvement of No-till farming systems,” in No-Till farming systems for sustainable agriculture. Editors Y. Dang, R. Dalal, and N. Menzies (Cham.: Springer), 155–171. doi:10.1007/978-3-030-46409-7_10
Wortmann, C. S., Drijber, R. A., and Franti, T. G. (2010). One-time tillage of no-till crop land five years post-tillage. Agron. J. 102, 1302–1307. doi:10.2134/agronj2010.0051
Wright, S. F., and Upadhyaya, A. (1998). A survey of soils for aggregate stability and glomalin, a glycoprotein produced by hyphae of arbuscular mycorrhizal fungi. Plant Soil 198, 97–107. doi:10.1023/a:1004347701584Available at: https://sci-hub.tw/10.2307/24122646.
Yang, M., Zhou, D., Hang, H., Chen, S., Liu, H., Su, J., et al. (2024). Effects of balancing exchangeable cations Ca, Mg, and K on the growth of tomato seedlings (Solanum lycopersicum L.) based on increased soil cation exchange capacity. Agronomy 14, 629. doi:10.3390/agronomy14030629
Yang, Y., Wu, J., Zhao, S., Mao, Y., Zhang, J., Pan, X., et al. (2021). Impact of long-term sub-soiling tillage on soil porosity and soil physical properties in the soil profile. L. Degrad. Dev. 32, 2892–2905. doi:10.1002/ldr.3874
Zhang, Y. G., Yang, S., Fu, M. M., Cai, J. P., Zhang, Y. Y., Wang, R. Z., et al. (2015). Sheep manure application increases soil exchangeable base cations in a semi-arid steppe of Inner Mongolia. J. Arid. Land 7, 361–369. doi:10.1007/s40333-015-0004-5
Keywords: conservation agriculture, crop residue management, crop rotation, soil health, soil nitrogen, strategic tillage, weed populations, wheat
Citation: Diop M, Beniaich A, Cicek H, Ouabbou H, El Gharras O, Tanji A, Bamouh A, Dahan R, Zine El Abidine A, El Gharous M and El Mejahed K (2024) Effects of occasional tillage on soil physical and chemical properties and weed infestation in a 10-year no-till system. Front. Environ. Sci. 12:1431822. doi: 10.3389/fenvs.2024.1431822
Received: 13 May 2024; Accepted: 21 August 2024;
Published: 10 September 2024.
Edited by:
Rosa Francaviglia, Council for Agricultural Research and Agricultural Economy Analysis CREA, ItalyReviewed by:
Wooiklee Paye, United States Department of Agriculture, United StatesYuan Li, Lanzhou University, China
Copyright © 2024 Diop, Beniaich, Cicek, Ouabbou, El Gharras, Tanji, Bamouh, Dahan, Zine El Abidine, El Gharous and El Mejahed. This is an open-access article distributed under the terms of the Creative Commons Attribution License (CC BY). The use, distribution or reproduction in other forums is permitted, provided the original author(s) and the copyright owner(s) are credited and that the original publication in this journal is cited, in accordance with accepted academic practice. No use, distribution or reproduction is permitted which does not comply with these terms.
*Correspondence: Khalil El Mejahed, Khalil.Elmejahed@um6p.ma