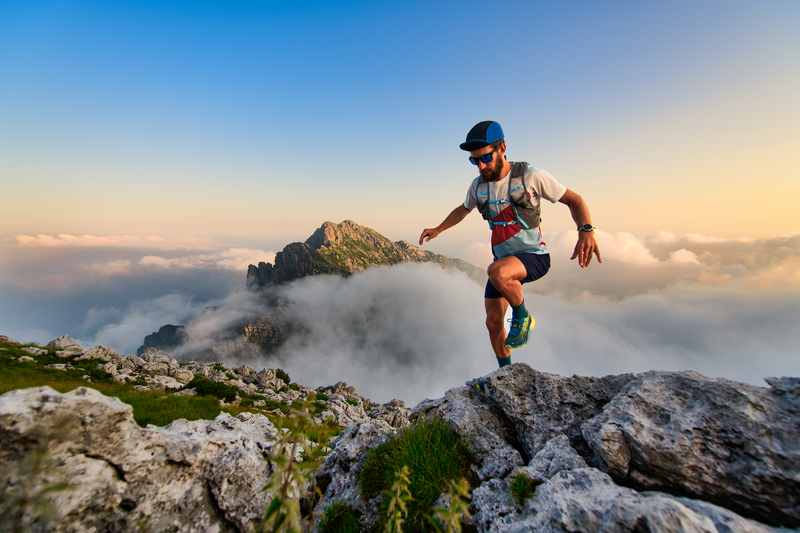
95% of researchers rate our articles as excellent or good
Learn more about the work of our research integrity team to safeguard the quality of each article we publish.
Find out more
POLICY AND PRACTICE REVIEWS article
Front. Environ. Sci. , 31 October 2024
Sec. Interdisciplinary Climate Studies
Volume 12 - 2024 | https://doi.org/10.3389/fenvs.2024.1421850
This article is part of the Research Topic Nature-based solutions for climate change adaptation View all 11 articles
One of the world’s largest “blue carbon” ecosystems, Louisiana’s tidal wetlands on the US Gulf of Mexico coast, is rapidly being lost. Louisiana’s strong legal, regulatory, and monitoring framework, developed for one of the world’s largest tidal wetland systems, provides an opportunity for a programmatic approach to blue carbon accreditation to support restoration of these ecologically and economically important tidal wetlands. Louisiana’s coastal wetlands span ∼1.4 million ha and accumulate 5.5–7.3 Tg yr−1 of blue carbon (organic carbon), ∼6%–8% of tidal marsh blue carbon accumulation globally. Louisiana has a favorable governance framework to advance blue carbon accreditation, due to centralized restoration planning, long term coastal monitoring, and strong legal and regulatory frameworks around carbon. Additional restoration efforts, planned through Louisiana’s Coastal Master Plan, over 50 years are projected to create, or avoid loss of, up to 81,000 ha of wetland. Current restoration funding, primarily from Deepwater Horizon oil spill settlements, will be fully committed by the early 2030s and additional funding sources are required. Existing accreditation methodologies have not been successfully applied to coastal Louisiana’s ecosystem restoration approaches or herbaceous tidal wetland types. Achieving financial viability for accreditation of these restoration and wetland types will require expanded application of existing blue carbon crediting methodologies. It will also require expanded approaches for predicting the future landscape without restoration, such as numerical modeling, to be validated. Additional methodologies (and/or standards) would have many common elements with those currently available but may be beneficial, depending on the goals and needs of both the state of Louisiana and potential purchasers of Louisiana tidal wetland carbon credits. This study identified twenty targeted needs that will address data and knowledge gaps to maximize financial viability of blue carbon accreditation for Louisiana’s tidal wetlands. Knowledge needs were identified in five categories: legislative and policy, accreditation methodologies and standards, soil carbon flux, methane flux, and lateral carbon flux. Due to the large spatial scale and diversity of tidal wetlands, it is expected that progress in coastal Louisiana has high potential to be generalized to similar wetland ecosystems across the northern Gulf of Mexico and globally.
Coastal blue carbon refers to the greenhouse gases (GHGs), such as carbon dioxide (CO2), methane (CH4), and nitrous oxide (N2O), that are stored, sequestered, and/or emitted by vegetated coastal tidal ecosystems amenable to management (Crooks et al., 2019; Windham-Myers et al., 2019; IPCC, 2022; Macreadie et al., 2022). Since 2000, there has been increasing interest in the carbon sequestration potential of coastal herbaceous wetlands, evidenced by an exponential increase in blue carbon-related publications (Duarte de Paula Costa and Macreadie, 2022). The recent U.S. Ocean Climate Action Plan called for advances in protection and restoration of blue carbon habitats (Ocean Policy Committee, 2023). Globally, wetland ecosystems make up only 5%–8% of Earth’s land surface, yet store between 20% and 30% of all soil carbon (Fourqurean et al., 2012; Nahlik and Fennessy, 2016). Tidal wetlands, in particular, are highly efficient at producing and accumulating organic matter and therefore have a high potential to sequester carbon (Chmura et al., 2003; Laffoley and Grimsditch, 2009; Ouyang and Lee, 2014; Hopkinson, 2020).
While the contribution of blue carbon to global-scale emissions offsetting is likely to be limited (Macreadie et al., 2022), blue carbon from tidal wetlands has the potential to make a meaningful contribution at national and sub-national scales. There are three factors driving the need to generate carbon offset credits for tidal wetland blue carbon in Louisiana. These are: 1) the state of Louisiana’s goal to meet GHG reduction targets; 2) rapid ongoing coastal land loss in the Mississippi River Delta; and 3) the need for sustainable future funding to implement coastal protection and restoration in the state of Louisiana. Approaches to supply offsets through voluntary carbon markets have been demonstrated in China, small island states, and other states in the United States (Sutton-Grier et al., 2014; Crooks et al., 2018; Wedding et al., 2021; Friess, 2023; Liu et al., 2024). In 2014, the Louisiana Coastal Protection and Restoration Authority (CPRA) developed a methodology for quantifying blue carbon sequestration resulting from wetland creation activities through the Verified Carbon Standard (VCS) (VM0024: Methodology for Coastal Wetland Creation; CPRA, 2014). At that time, the anticipated low market value of blue carbon credits, along with the high costs of project implementation, meant there was not sufficient financial return to incentivize implementation of blue carbon-oriented projects under this methodology. However, the State of Louisiana’s effort to develop that new methodology highlights their interest in developing new approaches to maximize the carbon market incentives for coastal restoration (Macreadie et al., 2022). The process of method development also identified areas where more data were needed to improve assumptions and increase accuracy in future net carbon flux quantification.
There is currently a lack of precedent when it comes to accreditation of blue carbon projects in herbaceous tidal wetlands, even though the, now retired, VM0024 was developed in Louisiana specifically for tidal wetlands. Most internationally accredited blue carbon projects have been in mangrove habitats, primarily based on large above ground biomass rather than soil carbon (Emmer et al., 2023; Verra, 2020). Therefore, mangrove projects are most analogous to the terrestrial-forest or agricultural revegetation projects that also focus on above ground biomass production. In addition, blue carbon in general makes up a small percentage of all nature-based crediting which is currently dominated by terrestrial forestry projects. Of the 35 blue carbon projects listed on registries globally (Verra, ACR, Plan Vivo; seven fully registered, 28 in development), one is focused on seagrasses, 31 include mangrove systems, and three are in tidal marshes (as of March 2023; ACR, 2023; Verra, 2023b; VIVO, 2023). Of these projects, 15 focus on conservation or avoided carbon loss, 13 on restoration or reforestation, and 7 include both conservation and restoration. In all cases, the carbon credit yield is weighted towards avoided emissions (avoided loss of habitat) rather than the potential for additional carbon stored through habitat creation or restoration (ACR, 2023; Verra, 2023b; VIVO, 2023). There are currently three U.S. blue carbon projects working towards registration (i.e., listed in the registry). Two related to restoration of water exchange in California tidal marshes using a California-specific methodology, and one in Virginia with a seagrass habitat focus under VM0033 (Verra, 2023b). The case studies for successfully crediting large blue carbon projects have also been in woody mangrove forests, such as Cispata Bay in Colombia and the Delta Blue Carbon Project in Pakistan (Kairo et al., 2020; Indus Delta Capital et al., 2021; Friess et al., 2022). No blue carbon project has been registered (issued credits) that includes any habitat equivalent to the herbaceous Spartina-dominated tidal marshes of coastal Louisiana, despite their known importance in regional carbon budgets (Baustian et al., 2020b).
There are two primary ways that restoration of tidal wetlands directly mitigates carbon release. Firstly, restoration and creation of coastal wetlands directly increases the carbon storage potential of coastal areas into the future by direct increase in wetland area (Greiner et al., 2013; Lovelock et al., 2022). Secondly, created wetlands can provide additional protection to nearby emergent wetlands, potentially reducing shoreline erosion from storms and waves. The reduced shoreline erosion has potential to be classified as avoided loss of carbon that would have occurred without the created wetland. Avoided wetland loss preserves existing carbon stocks in soils, therefore mitigating assumed release of carbon from these habitats to the atmosphere as emergent wetlands convert to open water. Transition of emergent wetland to open water is often equated with erosion and redistribution of carbon which forms an important assumption within quantification of verified carbon units (Figure 1; Pendleton et al., 2012; Sapkota and White, 2019; Moritsch et al., 2021). The state of Louisiana’s Coastal Master Plan includes risk reduction and restoration projects that are projected to create or maintain 60,000 to 81,000 ha of tidal marshes over the next 50 years (CPRA, 2023b).
Figure 1. Projection of 50 years future without restoration action, High climate change scenario (+2.5 ft of sea level rise) and 391 CRMS (Coastwide Reference Monitoring System – yellow circles) stations. Data sourced from CPRA (2023b) and CPRA 2023 Coastal Master Plan data.
Louisiana was estimated to contain 1.4 million ha of wetlands in 2016 and accumulate between 5.5 and 7.3 Tg C yr−1, accounting for 6%–8% of the global carbon accumulation in tidal marshes (Baustian et al., 2020b). The more than 700 km (linear) of Louisiana coastline includes the entire delta plain of the Mississippi River, with a watershed of ∼3.8 × 108 ha (Vörösmarty et al., 2000). The Mississippi River Delta receives the seventh-largest sediment discharge of any river globally, noting that construction of dams and a cessation of legacy land clearing have reduced sediment transport from 400 × 106 Mg yr−1 prior to the year 1900 down to a mean of 145 × 106 Mg yr−1 between 1987 and 2006 (Meade and Moody, 2009). Coastal Louisiana also includes the adjacent Chenier Plain, a separate geomorphological feature (Cheniers) with different vegetation structure that is strongly influenced by stranded beach ridges (Penland, 1990; Battaglia et al., 2012). Coastal Louisiana includes seven estuarine bays and four coastal lake systems with a variety of salinity regimes. Therefore, identifying viable pathways to credit coastal carbon across the broad range of geography, geology, and ecology within coastal Louisiana can provide knowledge and lessons that are relevant to the large number of GHG accounting mechanisms globally (Vanderklift et al., 2019). From a governance perspective, this geography has a strategic advantage since this range of ecosystems and large geography are all governed by the state of Louisiana, United States. Therefore, coastal Louisiana provides a substantial opportunity for tangible progress in pathways to apply, refine, or develop financially viable blue carbon standards and methodologies for tidal wetlands, including restoration and marsh creation actions. To assess the opportunity and progress towards realizing carbon credits from tidal wetlands, we brought together perspectives from state, federal, and international coastal managers as well as policy and scientific experts in the science and implementation of blue carbon projects through multiple verification standards. While initial conversations were conducted over many years, targeted discussions, review, and revision of this manuscript served to synthesize and clarify targeted knowledge needs that can maximize financial viability of blue carbon crediting of Louisiana’s tidal wetlands.
A first-order requirement for accreditation of tidal wetland restoration for carbon credits is a foundational principle of offset projects called additionality. Additionality maintains that an offset credit is granted only to the extent that the associated amount of emission reduction or sequestration is beyond “business-as-usual” conditions (Murray et al., 2007). Also, it must be demonstrated that the activity as well as any reductions or removals associated with the offset credits would not have occurred in the absence of the incentive created by carbon credit revenues (VCS Standard, v4.4, 2023; ICVCM, 2023). Dynamic tidal wetlands, such as those in North America, dominated by herbaceous perennials have specific challenges in terms of additionality and permanence compared to woody mangrove-dominated wetlands with regard to blue carbon credit-issuing. For wetlands projects to meet additionaility requirements and move forward they must demonstrated that the actions are not required by law, statues, or regulations (Emmer et al., 2023, VMD0052, 2021, Mack et al., 2021). Clarifying additionality requires careful analysis of initial and ongoing funding programs in collaboration with granting agencies, landowners, and carbon market standards. Another uncertainty relates to the relationships between private, state, and federal accounting mechanisms. There are multiple GHG inventory processes, not all regulatory and not all international. Therefore, in some cases, it is appropriate and acceptable to have a unit of carbon reported into an inventory process (e.g. a state / private entity inventory or commitment to reach net zero emissions) at the same time as being part of a carbon offset salable on the open market. Logically, these credits are legitimately moving both the private company and the state towards net zero at the same time, and should not pose a conflict as they are accounted for independently, however this is yet to be tested.
Permanence in carbon accounting is based on the slow cycling of carbon between the atmosphere and terrestrial or aquatic reservoirs (Joos et al., 2013; Broekhoff et al., 2019). Although the length of time required for project life and monitoring varies, 100 years is commonly considered “permanent” for the purposes of offset verification (Verra, 2014; 2020; Emmer et al., 2023). A 100-year time frame is challenging in highly dynamic coastal deltaic systems such as the Mississippi River Delta. Coastal Louisiana has one of the fastest rates of coastal land loss globally, with a mean of 42.9 km2 land lost per year between 1985 and 2010, a rate that has been slowing as less emergent tidal wetland is available to subside and erode (Figure 1; Couvillion et al., 2013, 2017). Multiple factors contribute to this land loss including hydrological changes reducing sediment supply, hurricane disturbance and storms causing erosion, eustatic sea-level rise, and high subsidence rates, being 5–8 mm yr−1 over most of Louisiana’s saline and brackish wetlands (Applied Coastal Research and Engineering, 2021; CPRA, 2023a). Thus, relative sea-level rise rates are as high as 12 ± 8 mm yr−1 in some areas of the Mississippi River Delta (Day et al., 2000; Yuill et al., 2009; Bailey et al., 2014; Khalil and Raynie, 2015; Jankowski et al., 2017; Törnqvist et al., 2020) and 9–45 mm yr−1 at the mouth of the Mississippi River (CPRA, 2023a). Two major hurricanes in Louisiana in 2005 resulted in around 560 km2 of land loss (Barras, 2006; Piazza et al., 2011). Within carbon accrediting methodologies the risk of “reversal” (loss of captured carbon back to the atmosphere) is mitigated with buffer pools, for example, VM0033 uses the “non-permanence risk tool” to reduce the number of verified carbon units to insure against future loss (Verra, 2023a). Some methodologies, such as the blue carbon accounting model of the Australian blue carbon accounting method (Lovelock et al., 2021), provide the option to calculate carbon credits using either a 100- or a 25-year horizon (noting the large penalties for 25- year projects), which may be a practical approach for highly dynamic tidal wetlands.
Current, and recently retired, methodologies for blue carbon accreditation, including Verra methodologies VM0024, VM0033, and VM0007, focus on a project footprint, have strict assumptions disallowing credit for the stable fraction of allochthonous carbon, and discount some of the carbon that leaves the project footprint based on geomorphic setting (Needelman et al., 2018). Additional carbon sequestration in dynamic and transitional coastal and deltaic systems experiencing marsh “drowning,” habitat migration, and changes in sediment supply and salinity at a coastwide scale are particularly difficult to accommodate in a project for a coastal system as complicated as the Mississippi Delta. However, in Louisiana, the state level planning capabilities and high capacity science community are in place to make progress.
In addition to the carbon attributable to the creation of tidal wetlands, the avoided loss of wetlands holds potential to yield a much higher net GHG flux benefit than direct tidal wetland creation alone. While VM0007 includes avoided loss and can be applied to dynamic tidal wetlands, it is currently challenging to quantify what would have been lost without the project and therefore quantify the avoided emissions. One approach could be to use peer-reviewed process-based numerical modeling of geomorphology and hydrodynamics to justify the baseline case of future without project against which the impacts of the project can be compared. Such an approach needs to be tested against the standard. At present, protection or conservation of intact wetlands is approved through VM0007 and restoration or creation through VM0033. It would be possible to include creation and conservation within one application (that would be under VM0007) but would be complex and there are currently no such approved projects. Verra is working on transferring tidal wetlands modules from VM0007 into an expanded VM0033 bringing conservation of intact wetlands and wetland restoration together into a single set of procedures to aid project development. An example of this approach for terrestrial forest conservation and management is the Verra methodology for improved forest management using dynamic matched baselines from national forest inventories (VM0045; Shoch et al., 2022).
In 2021, over US$1 billion worth of voluntary carbon credits were traded on international markets totaling nearly 300 Tg of carbon dioxide equivalent (Forest Trends’ Ecosystem Marketplace, 2021). The vast majority of reported voluntary carbon credit transactions in 2021 were in the agriculture, forestry, and other land use categories based on above ground biomass production. Recognizing that demand for blue carbon credits exceeds current supply (Friess et al., 2022), Louisiana’s wetland restoration projects could contribute towards blue carbon credit supply within existing voluntary markets. Current estimates are that one hectare of Louisiana tidal marsh accumulates roughly the same amount of total soil carbon as 63–84 ha of an average forest. This is based on a mean forest soil carbon accumulation of 4.6 g C m−2 yr−1 (Mcleod et al., 2011) while Louisiana tidal wetland types accumulate between 289 g C m−2 yr−1 (long term estimate - 50 years) and 382 g C m−2 yr−1 (short term estimates - 10 years; Baustian et al., 2020a, 2021; DeLaune and White, 2012). In total, Louisiana tidal wetlands are estimated to accumulate carbon at 5.5–7.3 Tg C yr−1 (Baustian et al., 2020b). This high storage potential, as well as the rapid rate of loss of emergent marsh in coastal Louisiana and assumed associated loss of carbon stock, indicate that projects for building, and avoiding loss of, critical wetland habitat could have substantive influence on net carbon balances. Rapid transition from emergent tidal wetland to shallow open water raises a specific challenge for accreditation of blue carbon in coastal Louisiana (Figure 1). However, lack of data on the fate of carbon and the specific limitations of methodologies mean that blue carbon accreditation of tidal wetlands in Louisiana cannot currently be used to help meet these supply needs.
Uncertainties in social, governance, financial, technical, and scientific aspects of blue carbon projects have also limited the number of projects resulting in verified blue carbon units that can be traded (Macreadie et al., 2022). For tidal wetlands, the high cost of constructing restoration projects and monitoring project success or failure results in challenges to blue carbon accreditation due to limited financial incentives or benefits (Mack et al., 2021, 2022). Coastal Louisiana has the governance structures, technical background, and long-term ecosystem monitoring programs to address these challenges at a large scale (∼0.5 million hectares; Couvillion et al., 2016) with the potential to demonstrate and then operationalize marketable blue carbon from tidal wetlands.
There is an increasing incentive to identify and address the economic, scientific, legal, and regulatory impediments to implementing current, or developing new, standards and methodologies to increase the flow of blue carbon credits onto the voluntary market (Mcleod et al., 2011; Lovelock and Duarte, 2019; Macreadie et al., 2019, 2022; Friess et al., 2022; Sapkota et al., 2023). Implemented and planned coastal restoration projects in Louisiana have been estimated to build or maintain over 207,200 ha of emergent wetland (CPRA, 2017), with planned but not yet funded projects estimated to build or maintain a further 60,000–81,000 ha of tidal wetlands over the next 50 years (CPRA, 2023b). The state of Louisiana has the potential to supply high value blue carbon credits and has high incentive to generate revenue in support of coastal restoration or contribute to meeting net zero emissions targets. Decades of legislative and policy progress supporting blue carbon in coastal Louisiana provide a strong potential to realize this opportunity (Figure 2).
Figure 2. Map of 2023 Louisiana Coastal Master Plan restoration projects. Data sourced from (CPRA, 2023b) and CPRA 2023 Coastal Master Plan data.
Currently, the largest revenue stream supporting coastal restoration in Louisiana is from the settlement of the 2010 Deepwater Horizon (DWH) oil spill, which allocated more than US$8 billion in one-time funds to the state of Louisiana (GCERC, 2016; DWH NRDA Trustees, 2017; Carruthers et al., 2020; Henkel and Dausman, 2020; The Water Institute, 2020). Much of this funding has, or will be, used to implement restoration projects within the current or previous iterations of the Louisiana Coastal Master Plan, but final allocation of DWH settlement funds will occur in 2031 (Henkel and Dausman, 2020; CPRA, 2023b). However, all currently known funding sources still fall more than US$20 billion short of the estimated US$50 billion required to implement the Coastal Master Plan for protection and restoration of coastal Louisiana (CPRA, 2023b). In February 2022, the Louisiana Climate Action Task Force adopted the Climate Action Plan (CAP), recommending strategies and actions to reduce GHG emissions to net zero by 2050 (Climate Initiatives Task Force, 2022). One of the strategies recommended in the CAP was the restoration and conservation of Louisiana’s tidal wetlands to maximize climate mitigation and adaptation goals (Climate Initiatives Task Force, 2022). Actions within that strategy include optimizing the carbon sequestration potential of Louisiana’s tidal wetlands through implementation of the state’s integrated coastal restoration plan, the Louisiana Coastal Master Plan.
Louisiana has developed a strong legislative and policy framework to progress accreditation and sale of blue carbon credits from coastal restoration (Table 1). Louisiana’s Coastal Protection and Restoration Trust Fund was constitutionally established in Louisiana’s state treasury to provide a dedicated, recurring source of revenue for the development and implementation of the state’s Coastal Master Plan through CPRA. Language was added to CPRA’s enabling legislation in 2009 specifically to address the potential generation of carbon credits from CPRA projects and ensure that any revenues gained from such projects were dedicated back to CPRA’s Trust Fund (Act 523, 2009 Regular Session of the Louisiana Legislature). This trust provides a mechanism for the state of Louisiana to manage funds from carbon credits and utilize them for future restoration activities, rather than those funds going into the state of Louisiana’s general revenue. Unlike most coastal areas, approximately 75% of the coastal zone in Louisiana is owned by private entities (Coreil, 1996). Ownership of the emissions reductions from blue carbon projects depend on who owns the land, the applicable regulatory scheme, and private contractual relationships. A 2010 law was enacted to provide that compensation for carbon credit is due to the landowner of the property where the sequestration occurred, with important exceptions (Table 1). First, the landowner can contractually assign revenues to another party and, second, the law exempts projects carried out and or sponsored by the Coastal Protection and Restoration Authority, in which case any revenue is the property of the state of Louisiana (Table 1). These clarifications provide a strong basis to determine ownership of revenue from blue carbon credits resulting from coastal restoration implemented by CPRA. Finally, the Louisiana Legislature authorized the Department of Agriculture and Forestry to act as the state’s primary agency for programs related to carbon sequestration or the reduction of GHG emissions from agriculture and forestry.1 This authorization did not extend to other land-use sectors and expressly reserved for CPRA the authority over “benefits, credits, or offsets derived from projects approved and undertaken” by CPRA.2 This legislation further supported the potential for the state of Louisiana to claim carbon credits for land created or preserved through coastal restoration projects (Table 1).
Table 1. Legal and regulatory framework in the state of Louisiana, United States, favorable for programmatic and large scale blue carbon accreditation from restoration of tidal wetlands. Note that VCS methodologies are constantly being updated and major revisions are to be released in 2024–2025.
The State of Louisiana’s Climate Action Plan (CAP) recommended quantifying and monitoring the potential blue carbon in Louisiana habitats and developing a blue carbon market crediting mechanism. One path to achieve this goal is the development of an entirely new Louisiana-specific blue carbon accreditation system with an underlying standard and appropriate methodologies customized for the various habitat types in the state, most importantly herbaceous tidal wetlands. One potential challenge is the uncertainty of demand for a new or unique blue carbon credit. If there is not sufficient demand within the state, the international market would need to be convinced of the validity, reliability, and therefore value, of the carbon credits. A Louisiana blue carbon crediting mechanism could also include stacked, or stapled, benefits such as restoration projects that restore ecosystems to provide biodiversity, human benefits, and increased prevention of land loss. It could also help the state track and quantify its emissions and offsets in a manner aligned with its net zero emissions goals and other ecosystem maintenance goals. Another pathway could be to work through existing carbon standard programs, such as the Verra VCS, to expand application of, update, or amend existing methodologies. VM0033 is currently being updated, and the standard is considering some of the known challenges including carbonate consumption/production for its role in producing/consuming alkalinity and dissolved CO2. Given the large spatial scale and well developed legal and regulatory framework in coastal Louisiana, it is conceivable that a special case application of an established methodology (such as VM0033) may be feasible. One of the priority knowledge needs is a thorough investigation of these different policy pathways to determine the opportunities to reach a financially viable accreditation pathway for tidal wetlands in coastal Louisiana.
1. As 75% of land is privately owned in coastal Louisiana and considering the state legislation around carbon ownership, establish potential investment pathways for purchasers of carbon credits, landowners, and opportunities to fund restoration implementation.
2. To ensure regulatory additionality for projects with multiple funding sources, investigate which of the many current funding sources allow for resultant carbon to be certified for offsets.
3. Investigate the opportunities to have a special application of current methodologies to the large scale of coastal Louisiana and/or the potential for methodologies, through regular updates, to better accommodate some of the unique challenges posed by tidal wetlands in coastal Louisiana.
4. Assess the viability and opportunity of developing a new methodology under a current standard (such as the VCS) or development of a composite methodology under a new standard.
5. Specifically consider the opportunity of a coastwide programmatic approach for blue carbon accreditation in coastal Louisiana utilizing the integrated protection and restoration planning within the state (Louisiana’s Coastal Master Plan).
To be financially viable, quantifying and monitoring of blue carbon change over time will require cost-effective, efficient, and standardized protocols, including remote assessment of carbon and GHGs (Macreadie et al., 2019, 2022). Scientific consensus is becoming established on critical knowledge and data needs to reduce uncertainty in carbon storage and flux estimates (Mcleod et al., 2011; Holmquist et al., 2018; Lovelock and Duarte, 2019; Duarte de Paula Costa and Macreadie, 2022; Williamson and Gattuso, 2022). In one example, dozens of scientists from diverse disciplines developed a list of the top 10 priority research needs for blue carbon science (Macreadie et al., 2019). Similarly, the discussion below is framed from those aspirational priorities and describes how to apply them to the current governance and scientific context in coastal Louisiana. The four categories of key scientific knowledge needs are accreditation methodologies and standards, soil carbon flux, methane flux, and lateral carbon flux. These priorities have been developed over a decade of discussions amongst many of the authors of this article and others in the research, restoration, and coastal management community of the northern Gulf of Mexico and globally. They were clarified and synthesized in the development of this manuscript.
Coastal Louisiana has a strong legal and regulatory framework and baseline scientific data to develop the necessary crediting, market, standard, methodology, or verification mechanism, a major barrier to implementing trusted blue carbon markets (Pande, 2024). This includes almost two decades of effort focused on integrated restoration planning strongly built upon integrated coastwide monitoring (e.g., Coastwide Reference Monitoring System, CRMS), resource centralization (e.g., Coastal Information Management System, CIMS), and state agency centralization in restoration planning through CPRA. Additionally, there are ongoing updates to the Louisiana Coastal Master Plan and the Integrated Compartment Model (ICM), the numerical model on which it is based (Baustian et al., 2020a; Fischbach et al., 2021; White, 2021), as well as development of basin scale Delft3D numerical models for restoration project planning and implementation (Bregman et al., 2020; The Water Institute, 2022), and the refinement of the Land Use and Carbon Scenario Simulator (LUCAS) model (Liu et al., 2021) for coastal application. Carbon process and dynamics models have also been compared with regard to applicability for use in supporting carbon accreditation (Mack et al., 2023). Data synthesis and integration will need to continue to be the basis of developing the science and crediting framework supporting a Louisiana coastal carbon crediting methodology/standard. This will require an approach (including further building upon and developing current numerical and conceptual models) to quantify carbon in an ongoing regular (i.e., annual) cycle at a hectare scale. Due to critical uncertainties (and resultant highly conservative assumptions) in baseline projections for avoided loss, most projects moving forward through Verra, for example, are focused on revegetation rather than conservation or restoration of hydrological function.
Australia has approved a blue carbon methodology for attaining offsets or credits that is based on a calculation model that has simplified site assessment requirements (Lovelock et al., 2021). However, due to its broad geographic applicability the empirical model is still highly conservative, so may not address the critical uncertainties in quantifying carbon in coastal Louisiana. To achieve a more comprehensive and accurate assessment of net carbon flux at a hectare scale and maximize potential verifiable carbon units, previous process based numerical modeling efforts for coastal Louisiana will need further development and refinement. Opportunities for improvement include greater use of remote sensing data and empirical machine learning models to quantify carbon fluxes based on process-based hydrology, hydrodynamic, and morphology numerical models. While the coastwide ICM that underlies the Louisiana Coastal Master Plan has a 30 m × 30 m habitat grid, the salinity has compartments that are tens of kilometers in scale (White et al., 2017; White, 2021). Salinity is one of the more critical determinants of GHG flux estimates, particularly for CH4 emissions which are one of the largest sources of uncertainty in US coastal wetland GHG inventories (Holmquist et al., 2018). Therefore, the current resolution of the hydrology grid in the ICM model would not be sufficiently resolved to support progress towards a financially viable carbon accreditation framework due to high levels of uncertainty in primary input variables related to net GHG flux (such as salinity). The carbon accreditation framework would require incorporation of different model types to estimate and predict key parameters affecting blue carbon storage and flux in coastal Louisiana. Combining the strengths of numerical and empirical machine learning models can improve the accuracy and computational efficiency of blue carbon assessments, reduce uncertainties, and provide a more comprehensive understanding of carbon-associated coastal ecosystem processes. In addition, numerical modeling techniques can allow the application of spatially and temporally targeted local field measurements to estimate (or validate) carbon stocks and fluxes. Both process-based numerical modeling, machine learning, and remote sensing will be essential components of estimating the rate, and uncertainty, of change in carbon storage with and without restoration as well as estimating avoided loss through preservation and restoration actions.
A scientifically desirable and equitable approach is to have a network of control plots and matching criteria that can be used to quantify baseline emissions and removals in the absence of preservation and restoration actions and for numerical model validation. Coastal Louisiana has a comprehensive network of wetland monitoring sites (391 CRMS stations: Figure 1) that have well over a decade of baseline data. Targeted addition of metrics or refining protocols, if required, could ensure that this valuable monitoring network provides control data sites to specifically inform ongoing carbon quantification. The aim of monitoring to quantify baseline emissions and removals is a recent innovation in the VCM that has been integrated into methodologies for the restoration (Shoch et al., 2023) and improved management of terrestrial forests (Shoch et al., 2022) that could be applied to restoration and preservation of coastal wetlands. The main advantage of such an approach is that external factors impacting baseline emissions or removals—such as policy, markets, and climate change—are hard to predict in advance, and are better observed on a dynamic, real-time basis in control plots. Control plots could be monitored with a combination of remote sensing and periodic field measurements. For comparison, the Forest Inventory Analysis is maintained and updated every 5 years by the U.S. Forest Service and serves as the database for matching control plots under the new improved forest management methodology (Shoch et al., 2022). The existing CRMS network covering a variety of wetland types across the entire coast of Louisiana could be similarly leveraged for coastal restoration and preservation projects. This provides another example of how the strong scientific knowledge and monitoring data availability in coastal Louisiana can support progress towards large scale blue carbon accreditation.
1. Assess carbon flux at large spatial scale (basin and watershed) useful for national GHG inventories, based on LUCAS model output.
2. Report on carbon storage potential coastwide for state of Louisiana GHG inventory, using the Louisiana Coastal Master Plan numerical model (ICM).
3. Develop high resolution numerical models, based on ground-based monitoring, remotely sensed observations, and empirical machine learning models to reduce uncertainty in GHG flux estimates at a project, or programmatic, scale by refining available high resolution models such as the basin-wide Delft3D model (Meselhe et al., 2015; Baustian et al., 2018; Bregman et al., 2020).
4. Inform carbon quantification and numerical model validation for blue carbon accreditation with historic and ongoing monitoring data collection (Hijuelos and Hemmerling, 2016; CRMS, 2023).
5. Assess the potential of current, adapted, or additional standards and methodologies to provide financially viable coastwide Louisiana-specific blue carbon accreditation that incorporates best-available science.
Despite decades of research in coastal Louisiana ecosystems, the processes of soil carbon transport (lateral transport including flux during transition of emergent marsh to open water) and open-water sediment accumulation are not well-understood (Steinmuller and Chambers, 2019; Sapkota and White, 2020). In addition, there are gaps in the scientific understanding of shifting GHG balance with restoration activity (Mack et al., 2021). In the context of blue carbon accreditation, additional understanding through data collection can reduce uncertainty in establishing a geomorphologically relevant definition of permanence which is a critical component of carbon accreditation methodologies (Verra, 2014; Emmer et al., 2023). Changes in CH4 emissions are also an important component of permanence. For example, increasing temperature with climate change will exert an important influence on net CH4 fluxes through emergent marsh transitions (McTigue et al., 2021; Noyce and Megonigal, 2021). Almost all available accretion data are for emergent land, even though an increasing area of the coastal wetlands in Louisiana are shallow open water. Therefore, a better understanding of accretion and lateral transfer processes in submerging coastal wetlands (e.g., relict tidal swamp forests), submerged aquatic vegetation, and shallow bare benthos is required (see Lovelock et al., 2017). Current, or recently retired, carbon standards (VMD0050 module within VM0007) and common CH4 inventory methods used by U.S. Environmental Protection Agency (USEPA) assume that most or all soil carbon within the top 1 m is lost to the atmosphere when an emergent wetland converts to open water (Emmer et al., 2020; Baustian et al., 2023). These standards and methodologies also assume that carbon capture and storage are immediately regained to the level of a mature wetland when open water is restored to wetland habitat. While these assumptions may be mostly valid for surficial sediments occupied by the vegetation rhizosphere (Chambers et al., 2019; Wasson et al., 2019), they are unlikely to be valid for deeper sediment layers that may contain substantial buried wetland peat deposits accumulated over decades and centuries (Artigas et al., 2015; Rogers et al., 2019). Some studies of tidal wetland restoration projects indicate that carbon storage may take multiple decades before realizing soil carbon accumulation (e.g., Herbert et al., 2016; Murphy, 2020; Osland et al., 2020; Ledford et al., 2021). While there are indications that restoration of wetlands overall is positive for GHG mitigation (Kolka et al., 2021), uncertainties in key assumptions within current methodologies reduce the accuracy and reliability of net GHG flux quantification.
1. Conduct sensitivity analyses of key assumptions in net GHG flux calculations, including time scales and effect of management actions.
2. Improve assumptions of GHG flux (in particular CH4) across tidal wetland types through quantification accounting for salinity variability.
3. Refine assumptions of soil carbon loss (currently 75% below ground carbon down to 1 m) through quantification across tidal wetland types considering relevant physical inputs (exposure etc.).
4. Improve quantification of open water, basin, lake, and channel carbon accumulation rates, using modern data and paleo evidence.
The financial viability of blue carbon projects in Louisiana’s coastal wetlands is currently limited by substantial uncertainties in the amount of CH4 emissions from these environments (Williamson and Gattuso, 2022). Depending on the time horizon used, CH4 has a global warming potential between 27.9 and 81.1 (100-year and 20-year) times higher than CO2 (Calvin et al., 2023). Because of this large climate impact, current standards for certifying blue carbon projects in wetlands (e.g., VM0033) require developers to either continuously monitor CH4 emissions or to estimate them using a verified and validated methodology (Verra, 2014). Continuous monitoring is prohibitively expensive, and several cases exist where blue carbon projects have been withdrawn from consideration due to uncertainties and knowledge gaps related to CH4 emissions (Mack et al., 2022). As such, there is a need for improved methods to accurately estimate CH4 emissions from variable salinity, tidal blue carbon ecosystems that do not rely on continuous direct monitoring.
Sulfate from seawater leads sulfate-reducing microorganisms to outcompete methanogenic microorganisms, resulting in reduced methane production. As a result, salinity has long been used as a proxy to estimate CH4 emissions in coastal ecosystems, with high rates of CH4 production at salinities <5 psu and low rates of CH4 production at salinities >20 psu (Poffenbarger et al., 2011; Holm et al., 2016; Holmquist et al., 2018). The current carbon offset verification standard for tidal wetland restoration, VM0033, allows project developers to use a default CH4 emission rate of 0.011 T CH4 ha−1 yr−1 where the average or low-point salinity is 18–20 psu, and 0.0056 T CH4 ha−1 yr−1 where salinity is ≥20 psu. However, no default emission rates are permitted for intermediate and brackish salinities (0.5–18 psu). Which characterize ∼50% of Louisiana’s coastal wetlands (Sasser et al., 2008), although CH4 emissions vary between vegetation types and are negatively correlated to salinity across ecosystem types, the overall predictive power of this relationship remains too low for the purpose of verifying blue carbon offsets at a project or sub-basin scale (Derby et al., 2022). A recent meta-analysis of 97 studies found salinity predicts only 23% of CH4 flux variance in saltmarshes at a global scale (Al-Haj and Fulweiler, 2020) although the methods used to quantify salinity varied greatly across this meta-analysis. Because of this, other environmental drivers and approaches for estimation are needed to improve predictions of CH4 emissions in coastal wetlands.
Besides salinity, both microbial CH4 production (methanogenesis) and consumption (methanotrophy) are affected by sediment porosity, the concentration and composition of organic matter, water saturation depth and duration, frequency of soil exposure at low tide, temperature, and pH. Many wetland soils produce CH4 in deep anaerobic horizons, but CH4 can be oxidized back to CO2 before being emitted (52%–81% of gross CH4 production; Megonigal and Schlesinger, 2002). Plant productivity and community composition play a significant role in wetland CH4 cycling by regulating the availability of organic carbon, oxygen, and ferric iron for soil microorganisms. Some wetland plant species drive CH4 emissions by directly transporting soil CH4 to the atmosphere through highly porous aerenchyma tissue, allowing buried CH4 to bypass methanotrophic microbes in the upper soil layers (Sutton-Grier and Megonigal, 2011; Villa et al., 2020; Derby et al., 2022).
A key approach going forward will be to develop, and utilize where possible, new approaches that combine abiotic, plant, and microbial datasets to generate predictions of wetland CH4 emissions. Previous research in terrestrial ecosystems has demonstrated that the influence of microbial community composition on carbon dynamics rivals in magnitude the influence of soil chemistry (Domeignoz-Horta et al., 2020, 2021; Raczka et al., 2021), and small-scale models of soil carbon which explicitly relate environmental data to assays of microbe-mediated processes have an improved ability to capture and predict carbon dynamics (Blagodatsky and Richter, 1998; Lawrence et al., 2009; Allison et al., 2010; McGuire and Treseder, 2010; Allison, 2012; Wieder et al., 2013; Wieder et al., 2015a; Wieder et al., 2015b; Fujita et al., 2014; Lehmann et al., 2020). Likewise, Earth system models that neglect plant-microbe interactions may be biased and underestimate modeled climate impacts (Zhu et al., 2017; Shi et al., 2019; Wang et al., 2023). Prior investigations in permafrost and peat soil ecosystems have demonstrated that CH4 flux can be accurately predicted by the quantification of gene transcripts for methyl coenzyme reductase A (mcrA) and particulate CH4 monooxygenase (pmoA), the respective key functional genes for methanogenesis and CH4 oxidation (Freitag and Prosser, 2009; Freitag et al., 2010). Others have demonstrated cases where microbial composition data provided significant explanatory power for soil CH4 emissions and other biogeochemical processes that cannot be captured by abiotic variables (i.e., temperature, redox potential, pH) alone (McCalley et al., 2014; Graham et al., 2016; Meyer et al., 2020), in one case improving statistical fit of modeled CH4 emissions by 47% (Godin et al., 2012; Graham et al., 2016).
These approaches have not been applied in tidal wetlands and have potential to reduce uncertainty in quantification of CH4 flux in tidal wetlands with variable salinity (Spivak et al., 2019). These novel methods, with their cost-effectiveness at scale compared to continuous measurement approaches, have the potential to substantially improve CH4 prediction accuracy while lowering cost. In turn, these methods would bolster the feasibility of blue carbon initiatives in wetlands that encompass brackish and intermediate salinity wetlands where using current assumptions leads to the conclusion that CH4 emissions exceed carbon sequestration benefits.
1. Develop new and refined cost-effective proxies and models for CH4 emissions that are designed to leverage technology and databases for improved spatial and temporal resolution in highly salinity variable tidal wetlands across the salinity range 0.5–18 psu.
2. Apply data analytics and machine learning tools to microbial community and abundance data, as has been done for terrestrial soil communities, to reduce uncertainty in CH4 flux estimates.
3. Refine predictions of CH4 flux in salinity variable tidal wetlands to partition fluxes across tidal wetland types and spatial scales using eddy covariance measures, flux chambers, handheld sensors, quantification of microbial communities, and quantification of aerenchymatous wetland plants.
Better quantification of particulate transport and lateral fluxes from tidal wetlands in Louisiana has high potential to reduce uncertainty in GHG flux estimates. Under current crediting mechanisms, it is assumed that 100% of the aboveground carbon is released back into the atmosphere as GHGs when wetland soils are eroded (Verra, 2014; Emmer et al., 2023). Some of the carbon, in both dissolved and particulate forms, may undergo lateral flux or be transported to adjacent estuaries, lakes, or offshore and may not be ultimately emitted back to the atmosphere. Outflow of dissolved inorganic carbon (DIC) and alkalinity into the coastal oceans surpassed burial by about 1.7 times in a subtropical mangrove ecosystem, establishing it as a substantial long-term carbon sink (Maher et al., 2018; Reithmaier et al., 2023). Quantifying the fate of particulate carbon lateral transport from emergent marshes and shallow open water areas is needed to reduce uncertainty in carbon accounting for carbon accreditation. Lateral flux of dissolved carbonate species and alkalinity to adjacent marshes and open water, especially across different salinity tidal wetlands (fresh, intermediate, brackish, and saline) still requires detailed quantification for carbon accreditation purposes.
Carbon fluxes occur laterally between wetlands and estuaries including dissolved organic carbon (DOC), particulate organic carbon (POC), and DIC which are commonly imported and exported through runoff and tidal flushing (Olefeldt and Roulet, 2014; Fouché et al., 2017; Mueller et al., 2023). A significant part of tidal wetland and estuarine carbon budgets is the lateral flux from coastal wetlands to estuaries, which is due mainly to tidal flushing. Estimates of total organic carbon (TOC; in both dissolved and particulate forms) exchange (per unit area of wetland) in coastal wetlands of the eastern United States were summarized by Herrmann et al. (2015), who calculated an overall average of 185 ± 71 g C per m−2 yr−1. Similarly, estimates of DIC exchange in eastern US coastal wetlands were summarized by Najjar et al. (2018), who calculated an overall average of 236 ± 120 g C m-2 yr-1. Lateral carbon flux has been identified as an important mechanism of carbon loss from terrestrial ecosystems (Bogard et al., 2020; Regnier et al., 2022). However, little is known about the influence of coastal wetland restoration on lateral carbon fluxes or their impact on carbon balance of the surrounding wetlands (Arias-Ortiz et al., 2021). The Verra methodology, VM0033, includes default factors for carbon preservation in depositional environments (CPDE) based upon published values, recognizing large variations within offshore locations, including along the northern Gulf of Mexico (Blair and Aller, 2012). A requirement of the Verra tidal wetland methodologies is to account for carbon that enters or leaves the project area. This creates complexity for quantifying smaller, individual projects in the landscape. This is particularly the case for projects in landscapes vulnerable to erosion, where sequestered carbon is at risk of reversal, and where carbon may be exchanged with adjacent wetlands. One possibility to address this is to consider a much larger project footprint for verification purposes, such as a basin or multiple basin scale. This could include either a single restoration or protection activity or a group of restoration and protection activities. In the case of the larger footprint, there is also the potential that emissions and removals may be accounted for more completely across the landscape as part of regional monitoring programs, reducing the cost of additional monitoring and improving the application of data from available long term monitoring efforts.
As Louisiana wetlands transition to open water, vertical fluxes from understudied ecosystems and lateral carbon transport or fluxes from all ecosystems within shallow open waters will become a larger component of quantifying estuarine carbon budgets. For example, submerged aquatic vegetation (SAV) are abundant in intermediate and fresh wetlands in Louisiana (Poirrier et al., 2017; DeMarco et al., 2018). However, this carbon pool and its contribution to net GHG flux has not been included in coastal carbon budgets for Louisiana, even though it is estimated to be as large as 6.4 ± 0.1 Tg Corg (Hillmann et al., 2020). Coastwide carbon accumulation in SAV, 0.3 Tg Corg yr−1 (Hillmann et al., 2020), is an order of magnitude lower than emergent wetland, 2.2 Tg Corg yr−1 (Hillmann et al., 2020) or 4.3–7.3 g TC yr−1 (Baustian et al., 2020b, 2021). However, in consideration of carbon accounting for accreditation at a hectare scale, SAV has potential to be a high percentage of the carbon pool in some locations (Liu et al., 2023b). The potential contribution of SAV with respect to lateral carbon flux within shallow water areas has also not been quantified. Long-term monitoring has been identified as a need to determine the role of intense, episodic forcing events such as hurricanes and tidal cycles on carbon dynamics across wetland-estuary ecosystems (Cao and Tzortziou, 2021; D’Sa et al., 2023). Advances in remote sensing techniques have a strong potential to help fill this data gap with higher spatial and temporal resolution as well as the opportunity to map the dynamics of these temporally and spatially variable carbon stocks (Mcleod et al., 2011; Liu et al., 2023a).
1. Prioritize and improve net carbon flux estimates through increased accounting of lateral as well as vertical carbon fluxes.
2. Investigate dynamic numerical modeling to track improved quantification of dissolved and particulate carbon lateral fluxes from, and into, tidal wetlands across a range of salinity regimes to refine estimates of carbon loss to the atmosphere when emergent wetlands transition to shallow open water.
3. Include submerged aquatic vegetation (SAV) into coastal carbon budgets for Louisiana, through mapping and quantification of their role in lateral carbon transport and fluxes (accumulation, repository, and source), especially at the hectare scale to inform carbon accounting relevant to accreditation.
There are economic, ecological, and community benefits of maintaining tidal wetlands in coastal Louisiana. Large-scale ecosystem restoration is planned and coordinated through the Louisiana Coastal Protection and Restoration Authority (CPRA). CPRA leads prioritization, planning, and implementation of coastal risk reduction and restoration efforts, planning that extends out past 2050, assuming sufficient funding is made available. The state of Louisiana is also committed to reach net zero GHG emissions by 2050, as are many private companies that want to purchase high-value blue carbon offsets. The convergence of these needs present an opportunity for the benefits of blue carbon as a mitigation tool to meaningfully contribute to the prioritization of restoration actions within Louisiana’s Coastal Master Plan. This has potential dual benefits both to maximize climate change mitigation and to provide an additional funding stream to support implementation of coastal risk reduction and restoration. This would increase economic, ecological, and community resiliency in coastal Louisiana. Coordination and integration to reduce known scientific uncertainties with the revision or development of legal and regulatory mechanisms will be critical for accreditation of blue carbon to provide timely solutions to the challenges of coastal adaptation and mitigation. Coastal Louisiana has a long history of wetland science as well as systematic and large-scale ecosystem monitoring in parallel with decades of legislation on how to implement carbon accreditation of tidal wetlands. Even with this base of knowledge and experience, focused effort will be required to investigate and establish viable blue carbon accreditation pathways. This work identified twenty targeted knowledge needs that will address known data and knowledge gaps and can reduce uncertainty in key assumptions for quantifying net GHG flux at multiple spatial scales for accreditation of Louisiana’s tidal wetlands. Filling these knowledge gaps will need to be iterative, assessing the potential benefit (in terms of verifiable carbon units) of reducing uncertainty in a particular metric or updating a standard methodological assumption. Due to rapid loss of tidal wetlands and limited future funding for implementation of large-scale coastal restoration, the state of Louisiana has a strong incentive to generate carbon credits from the restoration of tidal wetlands. Given the size and ecological diversity of tidal wetlands in coastal Louisiana, progress made towards financially viable tidal wetland carbon credits in Louisiana will have strong potential for transfer to similar wetlands along the northern Gulf of Mexico and globally.
TC: Conceptualization, Investigation, Methodology, Supervision, Visualization, Writing–original draft, Writing–review and editing. SJ: Conceptualization, Validation, Visualization, Writing–original draft, Writing–review and editing. MT: Conceptualization, Investigation, Methodology, Visualization, Writing–original draft, Writing–review and editing. JS: Conceptualization, Methodology, Visualization, Writing–original draft, Writing–review and editing. BP: Conceptualization, Methodology, Visualization, Writing–original draft, Writing–review and editing. VB: Investigation, Writing–original draft, Writing–review and editing. JE: Conceptualization, Investigation, Visualization, Writing–original draft. PB: Validation, Writing–review and editing. RC: Validation, Writing–review and editing. SC: Validation, Writing–review and editing. JC: Conceptualization, Writing–review and editing. KD: Writing–review and editing. AMD: Conceptualization, Writing–review and editing. ALD: Validation, Writing–review and editing. SD: Conceptualization, Writing–original draft, Writing–review and editing. CE: Writing–review and editing. DF: Validation, Writing–review and editing. JF: Validation, Writing–review and editing. IG: Conceptualization, Writing–review and editing. GG: Validation, Writing–review and editing. SH: Validation, Writing–review and editing. EH: Validation, Writing–review and editing. GH: Conceptualization, Methodology, Writing–review and editing. JH: Methodology, Validation, Writing–review and editing. HJ: Writing–original draft, Writing–review and editing. SJ: Validation, Visualization, Writing–review and editing. EK: Writing–original draft, Writing–review and editing. KK: Validation, Writing–review and editing. PL: Methodology, Validation, Writing–review and editing. BL: Writing–original draft, Writing–review and editing. CL: Validation, Writing–review and editing. SM: Methodology, Validation, Writing–review and editing. PM: Validation, Writing–review and editing. KM: Validation, Writing–review and editing. JM: Writing–review and editing, Validation. BR: Writing–review and editing. SS: Writing–review and editing. LS: Writing–review and editing. HS: Methodology, Writing–review and editing. AS-G: Validation, Writing–review and editing. JV: Methodology, Writing–review and editing. JW: Writing–review and editing. MW: Validation, Writing–review and editing.
The author(s) declare financial support was received for the research, authorship, and/or publication of this article. This work was developed from planned and ad hoc discussions, meetings, and emails amongst the co-authors over a period of more than 10 years. While this effort received no direct financial support, staff time and publication costs were supported by The Water Institute, Baton Rouge, Louisiana, United States.
James Pahl, of the Coastal Protection and Restoration Authority (CPRA) and Richard Raynie, while working at CPRA, provided comments on an early version of this manuscript. Carlos M. Duarte, King Abdullah University of Science and Technology (KAUST), Gary Kendrick, School of Biological Sciences and the Oceans Institute, The University of Western Australia, Ryan Moyer, TerraCarbon, Lydia Olander, Nicholas Institute for Energy, Environment, and Sustainability, Duke University, Stephanie Simpson, The Nature Conservancy, Tom Sevick, U.S. Army Corps of Engineers, and Jean Cowan, Scott Hemmerling, Mike Miner, Garvin Pittman, of The Water Institute, all provided critical review comments on aspects of this manuscript. Charley Cameron provided technical review of the final text and Dexter Ellis formatted figures. This is contribution #1757 from the Institute of Environment at Florida International University.
Author MT was employed by Plauché and Carr, LLP. Authors JS and BP were employed by Stantec. Author SC was employed by Silvestrum Climate Associates. Author GH was employed by Jacobs Engineering Group. Author SM was employed by Tierra Resources LLC. Author SS was employed by TerraCarbon LLC.
The remaining authors declare that the research was conducted in the absence of any commercial or financial relationships that could be construed as a potential conflict of interest.
The author(s) declared that they were an editorial board member of Frontiers, at the time of submission. This had no impact on the peer review process and the final decision.
All claims expressed in this article are solely those of the authors and do not necessarily represent those of their affiliated organizations, or those of the publisher, the editors and the reviewers. Any product that may be evaluated in this article, or claim that may be made by its manufacturer, is not guaranteed or endorsed by the publisher.
Any use of trade, firm, or product names is for descriptive purposes only and does not imply endorsement by the U.S. Government.
1La. R.S. 3:1221 in 2010
2La. R.S. 3:1221(C)
ACR (2023). American carbon registry. Available at: https://acr2.apx.com/mymodule/mypage.asp (Accessed June 21, 2023).
Al-Haj, A. N., and Fulweiler, R. W. (2020). A synthesis of methane emissions from shallow vegetated coastal ecosystems. Glob. Change Biol. 26, 2988–3005. doi:10.1111/gcb.15046
Allison, S. D. (2012). A trait-based approach for modelling microbial litter decomposition. Ecol. Lett. 15, 1058–1070. doi:10.1111/j.1461-0248.2012.01807.x
Allison, S. D., Wallenstein, M. D., and Bradford, M. A. (2010). Soil-carbon response to warming dependent on microbial physiology. Nat. Geosci. 3, 336–340. doi:10.1038/ngeo846
Applied Coastal Research and Engineering (2021). Recent subsidence trends for Southeastern Louisiana derived from static GPS measurements at primary and secondary benchmarks. Prepared by Applied Coastal Research and Engineering in Cooperation with CDM Smith for the Louisiana Coastal Protection and Restoration Authority.
Arias-Ortiz, A., Oikawa, P. Y., Carlin, J., Masqué, P., Shahan, J., Kanneg, S., et al. (2021). Tidal and nontidal marsh restoration: a trade-off between carbon sequestration, methane emissions, and soil accretion. J. Geophys Res. Biogeosci 126. doi:10.1029/2021JG006573
Artigas, F., Shin, J. Y., Hobble, C., Marti-Donati, A., Schäfer, K. V. R., and Pechmann, I. (2015). Long term carbon storage potential and CO2 sink strength of a restored salt marsh in New Jersey. Agric. For. Meteorology 200, 313–321. doi:10.1016/j.agrformet.2014.09.012
Bailey, C., Gramling, R., and Laska, S. B. (2014) “Complexities of resilience: adaptation and change within human communities of coastal Louisiana,” in Perspectives on the restoration of the Mississippi Delta, Estuaries of the World (Springer), 125–140. doi:10.1007/978-94-017-8733-8_9
Barras, J. A. (2006). Land area change in coastal Louisiana after the 2005 hurricanes: a historical perspective (from 1956). U.S. Geological Survey Open-File. Report No.: 06–1274
Battaglia, L. L., Woodrey, M. S., Peterson, M. S., Dillon, K. S., and Visser, J. M. (2012). “Wetlands of the northern Gulf coast,” in Wetland habitats of North America: ecology and conservation concerns. Editors D. P. Batzer,, and A. H. Baldwin (Berkeley, CA: University of California Press).
Baustian, M. M., Liu, B., Moss, L. C., Dausman, A., and Pahl, J. W. (2023). Climate change mitigation potential of Louisiana’s coastal area: current estimates and future projections. Ecol. Appl. 33, e2847. doi:10.1002/eap.2847
Baustian, M. M., Meselhe, E. A., Jung, H., Sadid, K., Duke-Sylvester, S. M., Visser, J. M., et al. (2018). Development of an integrated biophysical model to represent morphological and ecological processes in a changing deltaic and coastal ecosystem. Environ. Model. & Softw. 109, 402–419. doi:10.1016/j.envsoft.2018.05.019
Baustian, M. M., Reed, D. J., Visser, J. M., Duke-Sylvester, S. M., Snedden, G. A., Wang, H., et al. (2020a). 2023 Coastal master plan: ICM-wetlands, vegetation, and soils model improvement report. Baton Rouge, LA: Coastal Protection and Restoration Authority.
Baustian, M. M., Stagg, C. L., Perry, C. L., Moss, L., and Carruthers, T. (2021). Long-term carbon sinks in marsh soils of coastal Louisiana are at risk to wetland loss. AGU Biogeosciences 126. doi:10.1029/2020jg005832
Baustian, M. M., Stagg, C. L., Perry, C. L., Moss, L. C., Carruthers, T. J. B., and Allison, M. A. (2020b). Correction to: “Relationships between salinity and short-term soil carbon accumulation rates from marsh types across a landscape in the Mississippi River Delta”. Wetlands 40, 917–923. doi:10.1007/s13157-020-01283-8
Blagodatsky, S. A., and Richter, O. (1998). Microbial growth in soil and nitrogen turnover: a theoretical model considering the activity state of microorganisms. Soil Biol. Biochem. 30, 1743–1755. doi:10.1016/S0038-0717(98)00028-5
Blair, N. E., and Aller, R. C. (2012). The fate of terrestrial organic carbon in the marine environment. Ann. Rev. Mar. Sci. 4 (1), 401–423.
Bogard, M. J., Bergamaschi, B. A., Butman, D. E., Anderson, F., Knox, S. H., and Windham-Myers, L. (2020). Hydrologic export is a major component of coastal wetland carbon budgets. Glob. Biogeochem. Cycles 34, e2019GB006430. doi:10.1029/2019GB006430
Bregman, M., Messina, F., Jung, H., Yuill, B. T., Baustian, M. M., and Georgiou, I. Y. (2020). Basin wide model version 4: basin wide model for mid-Breton sediment diversion modeling. Baton Rouge, LA: The Water Institute of the Gulf. Funded by the Coastal Protection and Restoration Authority.
Broekhoff, D., Gillenwater, M., Colbert-Sangree, T., and Cage, P. (2019). Securing climate benefit: a guide to using carbon offsets. Stockholm Environment Institute & Greenhouse Gas Management Institute. Available at: https://www.offsetguide.org.
Calvin, K., Dasgupta, D., Krinner, G., Mukherji, A., Thorne, P. W., Trisos, C., et al. (2023). “IPCC, 2023: climate change 2023: synthesis report,” in Contribution of working groups I, II and III to the sixth assessment report of the intergovernmental panel on climate change [core writing team]. Editors H. Lee,, and J. Romero (Geneva, Switzerland: First. Intergovernmental Panel on Climate Change, IPCC). doi:10.59327/IPCC/AR6-9789291691647
Cao, F., and Tzortziou, M. (2021). Capturing dissolved organic carbon dynamics with landsat-8 and sentinel-2 in tidally influenced wetland–estuarine systems. Sci. Total Environ. 777, 145910. doi:10.1016/j.scitotenv.2021.145910
Carruthers, T. J. B., Raynie, R., Dausman, A., and Khalil, S. (2020). Strategies to improve implementation of adaptive management practices for restoration in coastal Louisiana. Shore & Beach 88, 83–91. doi:10.34237/10088110
Chambers, L. G., Steinmuller, H. E., and Breithaupt, J. L. (2019). Toward a mechanistic understanding of “peat collapse” and its potential contribution to coastal wetland loss. Ecology 15, e02720. doi:10.1002/ecy.2720
Chmura, G. L., Anisfeld, S. C., Cahoon, D. R., and Lynch, J. C. (2003). Global carbon sequestration in tidal, saline wetland soils. Glob. Biogeochem. Cycles 17, 1–22. doi:10.1029/2002gb001917
Climate Initiatives Task Force (2022). Louisiana climate action plan. Available at: https://gov.louisiana.gov/assets/docs/CCI-Task-force/CAP/Climate_Action_Plan_FINAL_3.pdf (Accessed February 17, 2022).
Coreil, P. D. (1996). “Landowners’ perceptions related to wetland regulatory policy in coastal Louisiana,” in 8th triennial national wildlife & fisheries extension specialists conference.
Couvillion, B. R., Beck, H., Schoolmaster, D., and Fischer, M. (2017). Land area change in coastal Louisiana from 1932 to 2016. U.S. Geological Survey Scientific Investigations Map 3381, 16. doi:10.3133/sim3381
Couvillion, B. R., Fischer, M. R., Beck, H. J., and Sleavin, W. J. (2016). Spatial configuration trends in coastal Louisiana from 1985 to 2010. Wetlands 36, 347–359. doi:10.1007/s13157-016-0744-9
Couvillion, B. R., Steyer, G. D., Wang, H., Beck, H. J., and Rybczyk, J. M. (2013). Forecasting the effects of coastal protection and restoration projects on wetland morphology in coastal Louisiana under multiple environmental uncertainty scenarios. J. Coast. Res. 67, 29–50. doi:10.2112/SI_67_3
CPRA (2012). Louisiana’s comprehensive master plan for a sustainable coast. Baton Rouge, LA: Coastal Protection and Restoration Authority. Available at: http://www.coastalmasterplan.la.gov.i1551-5036-67-sp1-16-CPRA1 (Accessed October 5, 2024).
CPRA (2014) Approved VCS methodology VM0024: methodology for coastal wetland creation. Washington D.C., United States: Version 1.0. Verified Carbon Standard.
CPRA (2017). Louisiana’s comprehensive master plan for a sustainable coast. Baton Rouge, Louisiana: Coastal Protection and Restoration Authority of Louisiana. Available at: http://coastal.la.gov/wp-content/uploads/2017/04/2017-Coastal-Master-Plan_Web-Single-Page_CFinal-with-Effective-Date-06092017.pdf (Accessed October 5, 2024).
CPRA (2023a) A brief chronology of CPRA’s approach and various studies on subsidence measurements in coastal Louisiana. Baton Rouge, LA: Coastal Protection and Restoration Authority.
CPRA (2023b). Louisiana’s comprehensive master plan for a sustainable coast. Baton Rouge, LA: Louisiana Coastal Protection and Restoration Authority, the State of Louisiana.
CRMS (2023). Coastwide reference monitoring system. Available at: https://www.lacoast.gov/crms/Home.aspx (Accessed June 20, 2023).
Crooks, S., Sutton-Grier, A. E., Troxler, T. G., Herold, N., Bernal, B., Schile-Beers, L., et al. (2018). Coastal wetland management as a contribution to the US National Greenhouse Gas Inventory. Nat. Clim. Change 8, 1109–1112. doi:10.1038/s41558-018-0345-0
Crooks, S., Windham-Myers, L., and Troxler, T. G. (2019). “Chapter 1. Defining blue carbon: the emergence of a climate context for coastal carbon dynamics,” in A blue carbon primer: the state of coastal wetland science, practice, and policy (Boca Raton: CRC Press), Chap. 2.
Day, J. W., Britsch, L. D., Hawes, S. R., Shaffer, G. P., Reed, D. J., and Cahoon, D. (2000). Pattern and process of land loss in the Mississippi delta: a spatial and temporal analysis of wetland habitat change. Estuaries 23, 425. doi:10.2307/1353136
DeLaune, R. D., and White, J. R. (2012). Will coastal wetlands continue to sequester carbon in response to an increase in global sea level? a case study of the rapidly subsiding Mississippi river deltaic plain. Clim. Change 110, 297–314. doi:10.1007/s10584-011-0089-6
DeMarco, K., Couvillion, B., Brown, S., and La Peyre, M. (2018). Submerged aquatic vegetation mapping in coastal Louisiana through development of a spatial likelihood occurrence (SLOO) model. Aquat. Bot. 151, 87–97. doi:10.1016/j.aquabot.2018.08.007
Derby, R. K., Needelman, B. A., Roden, A. A., and Megonigal, J. P. (2022). Vegetation and hydrology stratification as proxies to estimate methane emission from tidal marshes. Biogeochemistry 157, 227–243. doi:10.1007/s10533-021-00870-z
Domeignoz-Horta, L. A., Pold, G., Liu, X.-J. A., Frey, S. D., Melillo, J. M., and DeAngelis, K. M. (2020). Microbial diversity drives carbon use efficiency in a model soil. Nat. Commun. 11, 3684. doi:10.1038/s41467-020-17502-z
Domeignoz-Horta, L. A., Shinfuku, M., Junier, P., Poirier, S., Verrecchia, E., Sebag, D., et al. (2021). Direct evidence for the role of microbial community composition in the formation of soil organic matter composition and persistence. ISME Commun. 1, 64–4. doi:10.1038/s43705-021-00071-7
D’Sa, E. J., Tzortziou, M., and Liu, B. (2023). Extreme events and impacts on organic carbon cycles from ocean color remote sensing: review with case study, challenges, and future directions. Earth-Science Rev. 243, 104503. doi:10.1016/j.earscirev.2023.104503
Duarte de Paula Costa, M., and Macreadie, P. I. (2022). The evolution of blue carbon science. Wetlands 42, 109. doi:10.1007/s13157-022-01628-5
DWH NRDA Trustees (2017). Deepwater horizon oil spill natural resource damage assessment: strategic framework for bird restoration activities. National Oceanic and Atmospheric Administration. Available at: http://www.gulfspillrestoration.noaa.gov/restoration-planning/gulf-plan (Accessed June 12, 2024).
Emmer, I., Needelman, B. A., Emmet-Mattox, S., Crooks, S., Megonigal, P. J., Myers, D., et al. (2023). VM0033: methodology for tidal wetland and seagrass restoration v2.1. Verified Carbon Stand. Available at: https://verra.org/wp-content/uploads/2023/09/VM0033-Methodology-for-Tidal-Wetland-and-Seagrass-Restoration-v2.1.pdf (Accessed October 16, 2024).
Emmer, I. M., Needelman, B. A., Emmett-Mattox, S., Crooks, S., Megonigal, J., Myers, D., et al. (2020). Estimation of baseline carbon stock changes and greenhouse gas emissions in Tidal Wetland restoration and conservation Project Activities (BL-TW).
Fischbach, J. R., Johnson, D. R., Wilson, M. T., Geldner, N. B., and Stelzner, C. (2021). 2023 coastal master plan: model improvement report. Baton Rouge LA: CPRA.
Forest Trends’ Ecosystem Marketplace (2021) “Markets in motion,” in State of the voluntary carbon markets 2021. Washington DC: Forest Trends Association.
Fouché, J., Lafrenière, M. J., Rutherford, K., and Lamoureux, S. (2017). Seasonal hydrology and permafrost disturbance impacts on dissolved organic matter composition in high arctic headwater catchments. Arct. Sci. 3, 378–405. doi:10.1139/as-2016-0031
Fourqurean, J. W., Duarte, C. M., Kennedy, H., Marbà, N., Holmer, M., Mateo, M. A., et al. (2012). Seagrass ecosystems as a globally significant carbon stock. Nat. Geosci. 5, 505–509. doi:10.1038/ngeo1477
Freitag, T. E., and Prosser, J. I. (2009). Correlation of methane production and functional gene transcriptional activity in a peat soil. Appl. Environ. Microbiol. 75, 6679–6687. doi:10.1128/aem.01021-09
Freitag, T. E., Toet, S., Ineson, P., and Prosser, J. I. (2010). Links between methane flux and transcriptional activities of methanogens and methane oxidizers in a blanket peat bog. FEMS Microbiol. Ecol. 73, 157–165. doi:10.1111/j.1574-6941.2010.00871.x
Friess, D. A. (2023). The potential for mangrove and seagrass blue carbon in small island states. Curr. Opin. Environ. Sustain. 64, 101324. doi:10.1016/j.cosust.2023.101324
Friess, D. A., Howard, J., Huxham, M., Macreadie, P. I., and Ross, F. (2022). Capitalizing on the global financial interest in blue carbon. PLoS Clim. 1, e0000061. doi:10.1371/journal.pclm.0000061
Fujita, Y., Witte, J.-P. M., and van Bodegom, P. M. (2014). Incorporating microbial ecology concepts into global soil mineralization models to improve predictions of carbon and nitrogen fluxes. Glob. Biogeochem. Cycles 28, 223–238. doi:10.1002/2013GB004595
GCERC (2016). Gulf coast ecosystem restoration council comprehensive plan update 2016: restoring the Gulf coast’s ecosystem and economy. RESTORE Council.
Godin, A., McLaughlin, J. W., Webster, K. L., Packalen, M., and Basiliko, N. (2012). Methane and methanogen community dynamics across a boreal peatland nutrient gradient. Soil Biol. Biochem. 48, 96–105. doi:10.1016/j.soilbio.2012.01.018
Graham, E. B., Knelman, J. E., Schindlbacher, A., Siciliano, S., Breulmann, M., Yannarell, A., et al. (2016). Microbes as engines of ecosystem function: when does community structure enhance predictions of ecosystem processes? Front. Microbiol. 7, 214. doi:10.3389/fmicb.2016.00214
Greiner, J. T., McGlathery, K. J., Gunnell, J., and McKee, B. A. (2013). Seagrass restoration enhances “blue carbon” sequestration in coastal waters. PLoS One 8, e72469. doi:10.1371/journal.pone.0072469
Henkel, J., and Dausman, A. (2020). A short history of funding and accomplishments post-deepwater horizon. Shore & Beach, 11–16. doi:10.34237/1008811
Herbert, E. R., Marton, J. M., and Craft, C. B. (2016). “Tidal wetland restoration,” in Wetland soils: genesis, hydrology, landscapes, and classification. Editors M. J. Vepraskas, and C. B. Craft (Boca Raton, FL: CRC Press).
Herrmann, M., Najjar, R. G., Kemp, W. M., Alexander, R. B., Boyer, E. W., Cai, W.-J., et al. (2015). Net ecosystem production and organic carbon balance of U.S. East coast estuaries: a synthesis approach. Glob. Biogeochem. Cycles 29, 96–111. doi:10.1002/2013GB004736
Hijuelos, A. C., and Hemmerling, S. A. (2016). Coast wide and basin wide monitoring plans for Louisiana’s system wide and monitoring program (SWAMP) version III. Baton Rouge, LA: The Water Institute of the Gulf. Contract no. 2503-12-58 6. Prepared for and funded by the Coastal Protection and Restoration Authority under Task Order.
Hillmann, E. R., Rivera-Monroy, V. H., Nyman, J. A., and La Peyre, M. K. (2020). Estuarine submerged aquatic vegetation habitat provides organic carbon storage across a shifting landscape. Sci. Total Environ. 717, 137217. doi:10.1016/j.scitotenv.2020.137217
Holm, G. O., Perez, B. C., McWhorter, D. E., Krauss, K. W., Johnson, D. J., Raynie, R. C., et al. (2016). Ecosystem level methane fluxes from tidal freshwater and brackish marshes of the Mississippi River Delta: implications for coastal wetland carbon projects. Wetlands 36, 401–413. doi:10.1007/s13157-016-0746-7
Holmquist, J. R., Windham-Myers, L., Bernal, B., Byrd, K. B., Crooks, S., Gonneea, M. E., et al. (2018). Uncertainty in United States coastal wetland greenhouse gas inventorying. Environ. Res. Lett. 13, 115005. doi:10.1088/1748-9326/aae157
Hopkinson, C. S. (2020). “Chapter 5. Net ecosystem carbon balance of coastal wetland-dominated estuaries,” in A blue carbon primer: the state of coastal wetland science, practice, and policy. Editors S. Crooks, L. Windham-Myers, and T. G. Troxler (Boca Raton: CRC Press), 15.
ICVCM (2023). Core carbon principles, assessment framework and assessment procedure. Available at: https://icvcm.org/core-carbon-principles/ (Accessed May 3, 2024).
Indus Delta Capital, The Government of Sindh, and Silvestrum Climate Associates (2021). Delta blue carbon - 1. The Indus delta mangrove restoration project phase 1.
IPCC (2022). The Ocean and cryosphere in a changing climate: special report of the intergovernmental panel on climate change. 1st Edn. Cambridge University Press. doi:10.1017/9781009157964
Jankowski, K. L., Törnqvist, T. E., and Fernandes, A. M. (2017). Vulnerability of Louisiana’s coastal wetlands to present-day rates of relative seasea-level rise. Nat. Commun. 8, 14792. doi:10.1038/ncomms14792
Joos, F., Roth, R., Fuglestvedt, J. S., Peters, G. P., Enting, I. G., Von Bloh, W., et al. (2013). Carbon dioxide and climate impulse response functions for the computation of greenhouse gas metrics: a multi-model analysis. Atmos. Chem. Phys. 13, 2793–2825. doi:10.5194/acp-13-2793-2013
Kairo, J. G., Hamza, A. J., and Wanjiru, C. (2020). “Chapter 24. Mikoko pamoja: a demonstrably effective community-based blue carbon project in Kenya,” in A blue carbon primer: the state of coastal wetland science, practice, and policy. Editors S. Crooks, L. Windham-Myers, and T. G. Troxler (Boca Raton: CRC Press), 10.
Khalil, S. M., and Raynie, R. C. (2015). “An integrated system-based approach to restoration of Louisiana’s barrier islands,” in The proceedings of the coastal sediments 2015 (San Diego, USA: World Scientific). doi:10.1142/9789814689977_0189
Kolka, R., Trettin, C., and Windham-Myers, L. (2021). “24. The importance of wetland carbon dynamics to society: insight from the second state of the carbon cycle science report,” in Wetland carbon and environmental management. Editors K. W. Krauss, Z. Zhu, and C. L. Stagg (Wiley). doi:10.1002/9781119639305.fmatter
Laffoley, D., and Grimsditch, G. D. (2009). The management of natural coastal carbon sinks. Gland, Switzerland: IUCN.
Lawrence, C. R., Neff, J. C., and Schimel, J. P. (2009). Does adding microbial mechanisms of decomposition improve soil organic matter models? A comparison of four models using data from a pulsed rewetting experiment. Soil Biol. Biochem. 41, 1923–1934. doi:10.1016/j.soilbio.2009.06.016
Ledford, T. C., Mortazavi, B., Tatariw, C., Starr, S. F., Smyth, E., Wood, A. G., et al. (2021). Ecosystem carbon exchange and nitrogen removal rates in two 33-year-old constructed salt marshes are similar to those in a nearby natural marsh. Restor. Ecol. 29. doi:10.1111/rec.13439
Lehmann, J., Hansel, C. M., Kaiser, C., Kleber, M., Maher, K., Manzoni, S., et al. (2020). Persistence of soil organic carbon caused by functional complexity. Nat. Geosci. 13, 529–534. doi:10.1038/s41561-020-0612-3
Liu, B., D’Sa, E. J., Messina, F., Baustian, M. M., Maiti, K., Rivera-Monroy, V. H., et al. (2023a). Dissolved organic carbon dynamics and fluxes in Mississippi-Atchafalaya deltaic system impacted by an extreme flood event and hurricanes: a multi-satellite approach using Sentinel-2/3 and Landsat-8/9 data. Front. Mar. Sci. 10, 1159367. doi:10.3389/fmars.2023.1159367
Liu, B., Sevick, T., Jung, H., Kiskaddon, E., and Carruthers, T. (2023b). Quantifying the potential contribution of submerged aquatic vegetation to coastal carbon capture in a delta system from field and landsat 8/9-operational land imager (OLI) data with deep convolutional neural network. Remote Sens. 15, 3765. doi:10.3390/rs15153765
Liu, J., Failler, P., and Ramrattan, D. (2024). Blue carbon accounting to monitor coastal blue carbon ecosystems. J. Environ. Manag. 352, 120008. doi:10.1016/j.jenvman.2023.120008
Liu, J., Sleeter, B., Selmants, P. C., Diao, J., Zhou, Q., Worstell, B., et al. (2021). Modeling watershed carbon dynamics as affected by land cover change and soil erosion. Ecol. Model. 459, 109724. doi:10.1016/j.ecolmodel.2021.109724
Lovelock, C. E., Adame, M. F., Bradley, J., Dittmann, S., Hagger, V., Hickey, S. M., et al. (2022). An Australian blue carbon method to estimate climate change mitigation benefits of coastal wetland restoration. Restoration Ecol. n/a 31, e13739. doi:10.1111/rec.13739
Lovelock, C. E., and Duarte, C. M. (2019). Dimensions of blue carbon and emerging perspectives. Biol. Lett. 15, 20180781. doi:10.1098/rsbl.2018.0781
Lovelock, C. E., Fourqurean, J. W., and Morris, J. T. (2017). Modeled CO2 emissions from coastal wetland transitions to other land uses: tidal marshes, mangrove forests, and seagrass beds. Front. Mar. Sci. 4, 143. doi:10.3389/fmars.2017.00143
Lovelock, C. E., Sippo, J., Adame, M. F., Dittmann, S., Hickey, S., Hutley, L., et al. (2021). Blue carbon accounting model (BlueCAM) technical overview. Prepared for the Clean Energy Regulator.
Mack, S. K., Lane, R. R., Cowan, R., and Cole, J. W. (2021). “23. Status and challenges of wetlands in carbon markets,” in Wetland carbon and environmental management. Editors K. W. Krauss, Z. Zhu, and C. L. Stagg (Wiley). doi:10.1002/9781119639305.fmatter
Mack, S. K., Lane, R. R., Deng, J., Morris, J. T., and Bauer, J. J. (2023). Wetland carbon models: applications for wetland carbon commercialization. Ecol. Model. 476, 110228. doi:10.1016/j.ecolmodel.2022.110228
Mack, S. K., Lane, R. R., Holland, K., Bauer, J., Cole, J., and Cowan, R. (2022). A blue carbon pilot project: lessons learned. Carbon Manag. 13, 420–434. doi:10.1080/17583004.2022.2112292
Macreadie, P. I., Anton, A., Raven, J. A., Beaumont, N., Connolly, R. M., Friess, D. A., et al. (2019). The future of blue carbon science. Nat. Commun. 10, 3998. doi:10.1038/s41467-019-11693-w
Macreadie, P. I., Robertson, A. I., Spinks, B., Adams, M. P., Atchison, J. M., Bell-James, J., et al. (2022). Operationalizing marketable blue carbon. One Earth 5, 485–492. doi:10.1016/j.oneear.2022.04.005
Maher, D. T., Call, M., Santos, I. R., and Sanders, C. J. (2018). Beyond burial: lateral exchange is a significant atmospheric carbon sink in mangrove forests. Biol. Lett. 14, 20180200. doi:10.1098/rsbl.2018.0200
McCalley, C. K., Woodcroft, B. J., Hodgkins, S. B., Wehr, R. A., Kim, E.-H., Mondav, R., et al. (2014). Methane dynamics regulated by microbial community response to permafrost thaw. Nature 514, 478–481. doi:10.1038/nature13798
McGuire, K. L., and Treseder, K. K. (2010). Microbial communities and their relevance for ecosystem models: decomposition as a case study. Soil Biol. Biochem. 42, 529–535. doi:10.1016/j.soilbio.2009.11.016
Mcleod, E., Chmura, G. L., Bouillon, S., Salm, R., Björk, M., Duarte, C. M., et al. (2011). A blueprint for blue carbon: toward an improved understanding of the role of vegetated coastal habitats in sequestering CO2. Front. Ecol. Environ. 9, 552–560. doi:10.1890/110004
McTigue, N. D., Walker, Q. A., and Currin, C. A. (2021). Refining estimates of greenhouse gas emissions from salt marsh “blue carbon” erosion and decomposition. Front. Mar. Sci. 8, 661442. doi:10.3389/fmars.2021.661442
Meade, R. H., and Moody, J. A. (2009). Causes for the decline of suspended-sediment discharge in the Mississippi River system, 1940-2007. Hydrol. Process. 24, 35–49. doi:10.1002/hyp.7477
Megonigal, J. P., and Schlesinger, W. H. (2002). Methane-limited methanotrophy in tidal freshwater swamps. Glob. Biogeochem. Cycles 16, 35-1–35-10. doi:10.1029/2001GB001594
Meselhe, E., Baustian, M. M., Khadka, A., Jung, H., Allison, M., Duke-Sylvester, S. M., et al. (2015) “Basinwide model development for the LCA Mississippi river hydrodynamic and delta management study,” in Report 1: Delft3D model setup and development. Baton Rouge, LA: The Water Institute of the Gulf. Funded by the Coastal Protection and Restoration Authority under Task Order 27.1.
Meyer, K. M., Morris, A. H., Webster, K., Klein, A. M., Kroeger, M. E., Meredith, L. K., et al. (2020). Belowground changes to community structure alter methane-cycling dynamics in amazonia. Environ. Int. 145, 106131. doi:10.1016/j.envint.2020.106131
Moritsch, M. M., Young, M., Carnell, P., Macreadie, P. I., Lovelock, C., Nicholson, E., et al. (2021). Estimating blue carbon sequestration under coastal management scenarios. Sci. Total Environ. 777, 145962. doi:10.1016/j.scitotenv.2021.145962
Mueller, P., Kutzbach, L., Mozdzer, T. J., Jespersen, E., Barber, D. C., and Eller, F. (2023). Minerogenic salt marshes can function as important inorganic carbon stores. Limnol. & Oceanogr. 68, 942–952. doi:10.1002/lno.12322
Murphy, N. R. (2020). Vegetative community and health assessment of a constructed juncus-dominated salt marsh in the northern Gulf of Mexico.
Murray, B. C., Sohngen, B., and Ross, M. T. (2007). Economic consequences of consideration of permanence, leakage and additionality for soil carbon sequestration projects. Clim. Change 80, 127–143. doi:10.1007/s10584-006-9169-4
Nahlik, A. M., and Fennessy, M. S. (2016). Carbon storage in US wetlands. Nat. Commun. 7, 13835. doi:10.1038/ncomms13835
Najjar, R. G., Herrmann, M., Alexander, R., Boyer, E. W., Burdige, D. J., Butman, D., et al. (2018). Carbon budget of tidal wetlands, estuaries, and shelf waters of eastern North America. Glob. Biogeochem. Cycles 32, 389–416. doi:10.1002/2017GB005790
Needelman, B. A., Emmer, I. M., Emmett-Mattox, S., Crooks, S., Megonigal, J. P., Myers, D., et al. (2018). The science and policy of the verified carbon standard methodology for tidal wetland and seagrass restoration. Estuaries Coasts 41, 2159–2171. doi:10.1007/s12237-018-0429-0
Noyce, G. L., and Megonigal, J. P. (2021). Biogeochemical and plant trait mechanisms drive enhanced methane emissions in response to whole-ecosystem warming. Biogeosciences 18, 2449–2463. doi:10.5194/bg-18-2449-2021
Olefeldt, D., and Roulet, N. T. (2014). Permafrost conditions in peatlands regulate magnitude, timing, and chemical composition of catchment dissolved organic carbon export. Glob. Change Biol. 20, 3122–3136. doi:10.1111/gcb.12607
Osland, M. J., Feher, L. C., Spivak, A. C., Nestlerode, J. A., Almario, A. E., Cormier, N., et al. (2020). Rapid peat development beneath created, maturing mangrove forests: ecosystem changes across a 25-yr chronosequence. Ecol. Appl. 30, e02085. doi:10.1002/eap.2085
Ouyang, X., and Lee, S. Y. (2014). Updated estimates of carbon accumulation rates in coastal marsh sediments. Biogeosciences 11, 5057–5071. doi:10.5194/bg-11-5057-2014
Pendleton, L., Donato, D. C., Murray, B. C., Crooks, S., Jenkins, W. A., Sifleet, S., et al. (2012). Estimating global ‘“Blue carbon”’ emissions from conversion and degradation of vegetated coastal ecosystems. PLOS ONE 7, e43542. doi:10.1371/journal.pone.0043542
Penland, P. S. (1990). Barrier island evolution, delta plain development, and Chenier Plain formation in Louisiana. Louisiana: Louisiana State University and Agricultural and Mechanical College.
Piazza, S. C., Steyer, G. D., Cretini, K. F., Sasser, C. E., Visser, J. M., Holm, G. O., et al. (2011). Geomorphic and ecological effects of hurricanes katrina and rita on coastal Louisiana marsh communities. Reston, Virginia: U.S. Geological Survey.
Poffenbarger, H. J., Needelman, B. A., and Megonigal, J. P. (2011). Salinity influence on methane emissions from tidal marshes. Wetlands 31, 831–842. doi:10.1007/s13157-011-0197-0
Poirrier, M. A., Caputo, C. E., and Franze, C. D. (2017). Biogeography of submerged aquatic vegetation (SAV) in the Pontchartrain Basin: species salinity zonation and 1953–2016 Lake Pontchartrain trends. Southeast. Geogr. 57, 273–293. doi:10.1353/sgo.2017.0025
Raczka, N. C., Piñeiro, J., Tfaily, M. M., Chu, R. K., Lipton, M. S., Pasa-Tolic, L., et al. (2021). Interactions between microbial diversity and substrate chemistry determine the fate of carbon in soil. Sci. Rep. 11, 19320. doi:10.1038/s41598-021-97942-9
Regnier, P., Resplandy, L., Najjar, R. G., and Ciais, P. (2022). The land-to-ocean loops of the global carbon cycle. Nature 603, 401–410. doi:10.1038/s41586-021-04339-9
Reithmaier, G. M. S., Cabral, A., Akhand, A., Bogard, M. J., Borges, A. V., Bouillon, S., et al. (2023). Carbonate chemistry and carbon sequestration driven by inorganic carbon outwelling from mangroves and saltmarshes. Nat. Commun. 14, 8196. doi:10.1038/s41467-023-44037-w
Rogers, K., Kelleway, J. J., Saintilan, N., Megonigal, J. P., Adams, J. B., Holmquist, J. R., et al. (2019). Wetland carbon storage controlled by millennial-scale variation in relative seasea-level rise. Nature 567, 91–95. doi:10.1038/s41586-019-0951-7
Sapkota, Y., and White, J. R. (2019). Marsh edge erosion and associated carbon dynamics in coastal Louisiana: a proxy for future wetland-dominated coastlines world-wide. Estuar. Coast Shelf Sci. 226, 106289. doi:10.1016/j.ecss.2019.106289
Sapkota, Y., and White, J. R. (2020). Carbon offset market methodologies applicable for coastal wetland restoration and conservation in the United States: a review. Sci. Total Environ. 701, 134497. doi:10.1016/j.scitotenv.2019.134497
Sapkota, Y., Xu, K., Maiti, K., Inglett, P., and White, J. R. (2023). Temporal variability in soil organic matter accretion rates in coastal deltaic wetlands under changing depositional environments. Soil Sci. Soc. Am. J. 87, 390–403. doi:10.1002/saj2.20515
Sasser, C. E., Visser, J. M., Mouton, E., Linscombe, J., and Hartley, S. B. (2008). Vegetation types in coastal Louisiana in 2007. U.S. Geol. Surv. Available at: http://sabdata.cr.usgs.gov/sabnet/priv/net_pub_products/DATA_SPA/2012-16-0001.ZIP (Accessed July 16, 2015).
Shi, M., Fisher, J. B., Phillips, R. P., and Brzostek, E. R. (2019). Neglecting plant–microbe symbioses leads to underestimation of modeled climate impacts. Biogeosciences 16, 457–465. doi:10.5194/bg-16-457-2019
Shoch, D., Settelmeyer, S., Dickson, R., Ericksen, D., and Emmer, I. (2023). Methodology for afforestation, reforestation, and revegetation projects. Verra Standard VM0047 v1.0.
Shoch, D., Swais, E., Sonne Hall, E., Rifkin, B., Griscom, B., and Latta, G. (2022). Methodology for improved forest management using dynamic matched baselines from national forest inventories. Verra Methodology VM0045.
Spivak, A. C., Sanderman, J., Bowen, J. L., Canuel, E. A., and Hopkinson, C. S. (2019). Global-change controls on soil-carbon accumulation and loss in coastal vegetated ecosystems. Nat. Geosci. 12, 685–692. doi:10.1038/s41561-019-0435-2
Steinmuller, H. E., and Chambers, L. G. (2019). Characterization of coastal wetland soil organic matter: implications for wetland submergence. Sci. Total Environ. 677, 648–659. doi:10.1016/j.scitotenv.2019.04.405
Sutton-Grier, A. E., and Megonigal, J. P. (2011). Plant species traits regulate methane production in freshwater wetland soils. Soil Biol. Biochem. 43, 413–420. doi:10.1016/j.soilbio.2010.11.009
Sutton-Grier, A. E., Moore, A. K., Wiley, P. C., and Edwards, P. E. T. (2014). Incorporating ecosystem services into the implementation of existing U.S. Natural resource management regulations: operationalizing carbon sequestration and storage. Mar. Policy 43, 246–253. doi:10.1016/j.marpol.2013.06.003
The Water Institute (2020) Louisiana adaptive management status and improvement report: vision and recommendations. Baton Rouge, Louisiana: The Water Institute. Prepared for the coastal protection and restoration authority (CPRA) and the Louisiana trustee implementation group (LA TIG), funded by the LA TIG.
The Water Institute (2022). Partnership for our working coast: a community-informed transdisciplinary approach to maximizing benefits of dredged sediment for wetland restoration planning at port Fourchon, Louisiana. Baton Rouge, LA: The Water Institute of the Gulf. Prepared for and funded by the national fish and wildlife foundation, Shell, Chevron, Danos, and the greater Lafourche port commission.
Törnqvist, T. E., Jankowski, K. L., Li, Y.-X., and González, J. L. (2020). Tipping points of Mississippi Delta marshes due to accelerated sea-level rise. Sci. Adv. 6, eaaz5512. doi:10.1126/sciadv.aaz5512
Vanderklift, M. A., Marcos-Martinez, R., Butler, J. R. A., Coleman, M., Lawrence, A., Prislan, H., et al. (2019). Constraints and opportunities for market-based finance for the restoration and protection of blue carbon ecosystems. Mar. Policy 107, 103429. doi:10.1016/j.marpol.2019.02.001
Verra (2014). VM0024 methodology for coastal wetland creation. Available at: https://verra.org/methodologies/vm0024-methodology-for-coastal-wetland-creation-v1-0/ (Accessed October 5, 2024).
Verra (2020). VCS methodology VM0007 REDD+ methodology framework (REFF+ MF) v1.6. Available at: https://verra.org/wp-content/uploads/2020/09/VM0007-REDDMF_v1.6.pdf (Accessed November 17, 2022).
Verra (2023b). Verra search page. Available at: https://registry.verra.org/app/search/VCS/All%20Projects (Accessed June 21, 2023).
Villa, J. A., Ju, Y., Stephen, T., Rey-Sanchez, C., Wrighton, K. C., and Bohrer, G. (2020). Plant-mediated methane transport in emergent and floating-leaved species of a temperate freshwater mineral-soil wetland. Limnol. Oceanogr. 65, 1635–1650. doi:10.1002/lno.11467
VIVO (2023). Plan Vivo foundation – for nature, climate and communities. Plan Vivo Foundation. Available at: https://www.planvivo.org/ (Accessed June 20, 2023).
VMD0052 (2021) Demonstration of additionality of tidal wetland restoration and conservation project activities (ADD-AM), v2.0. Verified Carbon Stand. Available at: https://verra.org/methodologies/vmd0052-demonstration-of-additionality-of-tidal-wetland-restoration-and-conservation-project-activities-add-am-v1-0/ (Accessed October 16, 2024).
Vörösmarty, C. J., Fekete, B. M., Meybeck, M., and Lammers, R. B. (2000). Geomorphometric attributes of the global system of rivers at 30-minute spatial resolution. J. Hydrology 237, 17–39. doi:10.1016/S0022-1694(00)00282-1
Wang, H., Dai, Z., Krauss, K. W., Trettin, C. C., Noe, G. B., Burton, A. J., et al. (2023). Modeling impacts of saltwater intrusion on methane and nitrous oxide emissions in tidal forested wetlands. Ecol. Appl. 33, e2858. doi:10.1002/eap.2858
Wasson, K., Ganju, N. K., Defne, Z., Endris, C., Elsey-Quirk, T., Thorne, K. M., et al. (2019). Understanding tidal marsh trajectories: evaluation of multiple indicators of marsh persistence. Environ. Res. Lett. 14, 124073. doi:10.1088/1748-9326/ab5a94
Wedding, L. M., Moritsch, M., Verutes, G., Arkema, K., Hartge, E., Reiblich, J., et al. (2021). Incorporating blue carbon sequestration benefits into sub-national climate policies. Glob. Environ. Change 69, 102206. doi:10.1016/j.gloenvcha.2020.102206
White, E. (2021). 2023 coastal master plan, landscape modeling: final model improvements and scenario settings. Available at: https://coastal.la.gov/wp-content/uploads/2021/03/ICM-Landscape-Modeling_Part2_Jan2021_ppt.pdf (Accessed October 5, 2024).
White, E., Meselhe, E., McCorquodale, A., Couvillion, B., Dong, Z., Duke-Sylvester, S., et al. (2017). 2017 coastal master plan: attachment C3-22 – integrated compartment model (ICM) development. Available at: https://coastal.la.gov/wp-content/uploads/2017/04/Attachment-C3-22_FINAL_03.07.2017.pdf.
Wieder, W. R., Allison, S. D., Davidson, E. A., Georgiou, K., Hararuk, O., He, Y., et al. (2015a). Explicitly representing soil microbial processes in Earth system models. Glob. Biogeochem. Cycles 29, 1782–1800. doi:10.1002/2015GB005188
Wieder, W. R., Bonan, G. B., and Allison, S. D. (2013). Global soil carbon projections are improved by modelling microbial processes. Nat. Clim. Change 3, 909–912. doi:10.1038/nclimate1951
Wieder, W. R., Grandy, A. S., Kallenbach, C. M., Taylor, P. G., and Bonan, G. B. (2015b). Representing life in the Earth system with soil microbial functional traits in the MIMICS model. Biogeosciences. doi:10.5194/gmdd-8-2011-2015
Williamson, P., and Gattuso, J.-P. (2022). Carbon removal using coastal blue carbon ecosystems is uncertain and unreliable, with questionable climatic cost-effectiveness. Front. Clim. 4, 853666. doi:10.3389/fclim.2022.853666
Windham-Myers, L., Crooks, S., and Troxler, T. (2019). A blue carbon primer: the state of coastal wetland carbon science, practice and policy (Boca Raton, FL: CRC Press). Available at: https://www.routledge.com/A-Blue-Carbon-Primer-The-State-of-Coastal-Wetland-Carbon-Science-Practice/Windham-Myers-Crooks-Troxler/p/book/9780367893521 (Accessed May 19, 2021).
Yuill, B., Lavoie, D., and Reed, D. J. (2009). Understanding subsidence processes in coastal Louisiana. J. Coast Res. 54, 23–36. doi:10.2112/SI54-012.1
Keywords: blue carbon, tidal marsh, mitigation, adaptation, Louisiana, carbon credits, offsets, restoration
Citation: Carruthers TJB, Jones SB, Terrell MK, Scheibly JF, Player BJ, Black VA, Ehrenwerth JR, Biber PD, Connolly RM, Crooks S, Curole JP, Darnell KM, Dausman AM, DeJong AL, Doyle SM, Esposito CR, Friess DA, Fourqurean JW, Georgiou IY, Grimsditch GD, He S, Hillmann ER, Holm GO Jr, Howard J, Jung H, Jupiter SD, Kiskaddon E, Krauss KW, Lavery PS, Liu B, Lovelock CE, Mack SK, Macreadie PI, McGlathery KJ, Megonigal JP, Roberts BJ, Settelmyer S, Staver LW, Stevens HJ, Sutton-Grier AE, Villa JA, White JR and Waycott M (2024) Identifying and filling critical knowledge gaps can optimize financial viability of blue carbon projects in tidal wetlands. Front. Environ. Sci. 12:1421850. doi: 10.3389/fenvs.2024.1421850
Received: 23 April 2024; Accepted: 30 September 2024;
Published: 31 October 2024.
Edited by:
Nalini Rao, Electric Power Research Institute (EPRI), United StatesReviewed by:
Varghese Rani, Kerala University of Fisheries and Ocean Studies, IndiaCopyright © 2024 Carruthers, Jones, Terrell, Scheibly, Player, Black, Ehrenwerth, Biber, Connolly, Crooks, Curole, Darnell, Dausman, DeJong, Doyle, Esposito, Friess, Fourqurean, Georgiou, Grimsditch, He, Hillmann, Holm, Howard, Jung, Jupiter, Kiskaddon, Krauss, Lavery, Liu, Lovelock, Mack, Macreadie, McGlathery, Megonigal, Roberts, Settelmyer, Staver, Stevens, Sutton-Grier, Villa, White and Waycott. This is an open-access article distributed under the terms of the Creative Commons Attribution License (CC BY). The use, distribution or reproduction in other forums is permitted, provided the original author(s) and the copyright owner(s) are credited and that the original publication in this journal is cited, in accordance with accepted academic practice. No use, distribution or reproduction is permitted which does not comply with these terms.
*Correspondence: Tim J. B. Carruthers, dGNhcnJ1dGhlcnNAdGhld2F0ZXJpbnN0aXR1dGUub3Jn
†ORCID: Tim J. B. Carruthers, orcid.org/0000-0002-7772-0570; James W. Fourqurean, orcid.org/0000-0002-0811-8500; Ioannis Y. Georgiou, orcid.org/0000-0002-4384-8517; Songjie He, orcid.org/0000-0003-4814-8453; Catherine E. Lovelock, orcid.org/0000-0002-2219-6855; Karen J. McGlathery, orcid.org/0000-0003-2810-8198; J. Patrick Megonigal, orcid.org/0000-0002-2018-7883; Brian J. Roberts, orcid.org/0000-0002-6366-3165; Lorie W. Staver, orcid.org/0000-0002-1443-4008; Jorge A. Villa, orcid.org/0000-0003-1130-9401; John R. White, orcid.org/0000-0002-9967-013X
Disclaimer: All claims expressed in this article are solely those of the authors and do not necessarily represent those of their affiliated organizations, or those of the publisher, the editors and the reviewers. Any product that may be evaluated in this article or claim that may be made by its manufacturer is not guaranteed or endorsed by the publisher.
Research integrity at Frontiers
Learn more about the work of our research integrity team to safeguard the quality of each article we publish.