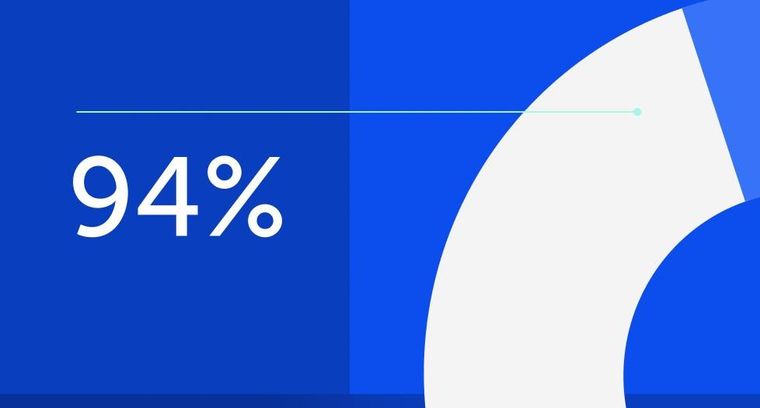
94% of researchers rate our articles as excellent or good
Learn more about the work of our research integrity team to safeguard the quality of each article we publish.
Find out more
ORIGINAL RESEARCH article
Front. Environ. Sci., 06 December 2024
Sec. Interdisciplinary Climate Studies
Volume 12 - 2024 | https://doi.org/10.3389/fenvs.2024.1421237
This article is part of the Research TopicNature-based solutions for climate change adaptationView all 11 articles
Constructed levees are designed to protect anthropogenic developments from destructive flooding events, but their construction has reduced groundwater recharge, increased flood risk severity under levee failure, increased the incision of river channels, and deteriorated riparian habitat. To reverse these impacts, levee setbacks are often designed to reduce flood risk and provide the opportunity to restore ecohydrological function, while groundwater recharge is rarely considered because it may require relatively detailed groundwater system analysis. In this study, we evaluated 100 heterogeneous hydrogeology realizations to estimate recharge with high-conductivity pathways (HCPs) under varying flood flows for a range of levee setback distances to identify the trade-offs in groundwater recharge and floodplain habitat. We find that on a regional scale, total recharge potential increases with setback distance, with the largest gains up to 1,400 m where there are outcropping HCPs and sufficient flow to inundate more of the setback area. In contrast, the recharge per unit area (i.e., the average daily recharge divided by setback area) generally decreases as levee setback increases, but there are local increases in the recharge per unit area at 1,400 m where HCP recharge may sufficiently offset the larger area. There is a median 10%–40% reduction in peak streamflow with increasing setback distance, which would aid flood risk reduction, but the increased area leads to decreasing depth due to flow losses and increased spreading of flood water. Ultimately, the decision for levee setback distance will depend on local conditions and management goals, as we find that increasing recharge will reduce the floodplain depth necessary for ecosystem function. Our results highlight the opportunity to consider groundwater recharge benefits in levee setback feasibility studies in semi-arid regions impacted by floods and groundwater overdrafts so that setback distance designs can achieve integration of flood risk reduction, riparian habitat, and groundwater recharge.
Levees are anthropogenic embankments constructed along a water course or river for flood risk reduction to prevent overflow of the river onto adjacent lands. Most levees constructed to date were designed to confine floods within a narrow, engineered floodway that blocked river access to its natural floodplain, resulting in deepening and accelerated flow through the leveed reach and decreased groundwater recharge, increased channel erosion, and reduced native species habitat (Chambers et al., 2023; Knox et al., 2022; Opperman et al., 2017). Many of these floodplain and water cycle functions have been further impacted by climate change in recent decades as the severity of floods and droughts has increased, which also reduces the effectiveness of traditional water management (e.g., canals and reservoirs) to capture, transfer, and store these extreme flows (Cayan et al., 2005; Swain et al., 2018). To reduce flood risk under an increasingly uncertain climate and to provide environmental benefits for riverine ecosystems, levee setback is a valuable tool that is increasingly adopted by flood managers, yet groundwater recharge benefits are seldom considered in the design stage of levee setback because designs are often guided by ecosystem and hydro-geomorphic functionality (Serra-Llobet et al., 2022; Smith et al., 2017).
While traditional flood risk reduction strategies have mainly focused on engineered control structures of river systems such as levees and reservoirs that were built based on a short streamflow record (less than 100 years), levee setbacks provide a more nature-based solution to flood risk reduction and in some ways a form of managed infrastructure retreat (Chambers et al., 2023; Klijn et al., 2018; Van Rees et al., 2024). Levees and reservoirs function best under the conditions of the design flow, but the extreme streamflows occurring today due to climate change are increasing the risk of infrastructure failure (Swain et al., 2018) and require the implementation of alternative flood management strategies such as levee setbacks. Levee setbacks can offer greater protection services by reconnecting the river with the floodplain, which offers greater floodwater conveyance on the floodplain, lower flood stages, and a reduced likelihood of overtopping levees or erosive failures (Dahl et al., 2017; National Research Council, 2013; Smith et al., 2017). A widened leveed reach can improve levee reliability and level of protection against climate change uncertainties and land use-driven changes in flood regimes (Chambers et al., 2024; Dierauer et al., 2012; Klijn et al., 2018). Levee setbacks reduce the exposure of levees (especially aging levees) and landward communities to flood hazards (e.g., extended and repeated periods of hydraulic loading with high river stages and high flow velocities) and are therefore considered an adaptive strategy for at-risk communities (Allan James and Singer, 2008; Cayan et al., 2005; Hui et al., 2018). The expanded floodplain created by levee setback also offers opportunities to rehabilitate levee-stressed aquatic and floodplain ecosystems that provide multiple benefits such as trapping suspended sediment, providing waterfowl habitat, or storing nutrients (Dahl et al., 2017; Gordon et al., 2020; Newcomer Johnson et al., 2016). Levee setback is typically done to increase flood capacity and to reduce risk at key points once levee failure, or a breach, has occurred at a river such that the design of levee setback for flood risk reduction depends on the required flood capacity increase necessary to significantly lower risk of levee failure (Dahl et al., 2017). However, opening the river corridor to allow more widespread flooding during extreme events can also create groundwater recharge benefits that are often neglected in the planning and design phase of levee setbacks.
In many arid and semi-arid regions, river seepage is the main recharge source for alluvial groundwater systems (Blasch et al., 2004; Jasechko et al., 2021; Morin et al., 2009; Watson et al., 2021). The same is true of rivers traversing aquifer systems that have been depleted due to overpumping. Widening the inundated floodplain through levee setback could, therefore, result in additional groundwater recharge (Dahl et al., 2017; Smith et al., 2017), which can provide both natural and human ecosystem benefits (Sommer et al., 2001). Levee setback allows opening the straightened, leveed river (which is often also reduced in length) to a larger area and frequent flooding, a process that is one type of flood managed aquifer recharge (Flood-MAR), where river flood waters are managed and directed to recharge opportunities such as dedicated recharge basins, agricultural fields, or floodplains (Dahlke et al., 2018; Levintal et al., 2023; Niswonger et al., 2017). However, more research is needed to understand the amount of groundwater recharge that can be achieved through levee setback, particularly the levee setback distance needed to unlock areas of high recharge potential, including historic river channels of the meandering river within the former river floodplain that could be filled with coarse sediments (Marchand et al., 2022). This is particularly relevant because floodplains, even when overlying major aquifer systems, tend to be relatively low in permeability as primarily finer-grain sediments are deposited overbank in the lower energy reaches of rivers except for river channel deposits from previous meanders of the river before levees were added. As shown in previous work (Fleckenstein et al., 2006; Niswonger et al., 2008), however, aquifer heterogeneity can result in locally high recharge rates beneath floodplains that need to be accounted for in estimating potential recharge benefits.
To address both rising flood risk and growing groundwater overdraft, levee setbacks and intentional groundwater recharge could provide valuable water management options to both increase water security and reduce damage from floods. Groundwater recharge from levee setbacks may be an important mechanism to address chronic groundwater overdrafts, especially in groundwater-dependent regions experiencing unprecedented drought conditions where users increasingly rely on already-burdened aquifers (Taylor et al., 2013). Flood-MAR is one method to increase recharge that has been rising in popularity because of more extreme streamflow events expected with climate change that, if diverted, would reduce downstream flood risk and provide large volumes of water for recharge (Kocis and Dahlke, 2017). Intentional recharge would help prevent overdraft in recharge-limited basins, but implementation is currently limited by the availability of high recharge potential sites, access to flood waters, and proof of other benefits, including ecosystem services, to name a few (Fuentes and Vervoort, 2020; O’Geen et al., 2015; Perrone and Rohde, 2016). Flood-MAR has been successfully demonstrated to increase groundwater storage and reduce depth to groundwater for urban and agricultural groundwater pumpers, but ecosystem return-flows may take decades to appear (Kourakos et al., 2019), and any seasonal habitat formed will be relatively homogeneous (floodplain with minimal vegetation or topographic variability) (Opperman et al., 2017). Limited research on floodplain restoration has estimated how levee setbacks could influence groundwater recharge in the widened floodplain. Yoder (2018) found an upward shift in the relationship between groundwater recharge and discharge, indicating that levee removal increased recharge, but results were limited by a lack of groundwater data from pre-restoration conditions (Yoder, 2018).
In recent years, research on groundwater recharge has shifted to include the effect of subsurface heterogeneity on recharge potential (Gottschalk et al., 2017; Maples et al., 2019; 2020; Parsekian et al., 2014; Rodriguez et al., 2021). However, little research exists on the effect that subsurface heterogeneity has on the floodplain recharge potential in levee setback areas (Serra-Llobet et al., 2022; Smith et al., 2017). Aquifer heterogeneity and specifically the existence of high conductivity pathways in the subsurface (Fogg 1986) can result in higher recharge rates at specific locations and faster transport of recharge water through the aquifer system (Maples et al., 2019); thus, if present in a levee setback area, they would increase recharge. A high conductivity pathway can be visualized as a coarse grain sediment (e.g., sand and gravel) deposit surrounded by lower conductivity sediments where the sand and gravel deposits may interconnect, thus supporting fast paths for water flow underground. These high conductivity pathways can be identified with geophysical surveying and well driller logs to delineate their boundaries and may have outcrops at the land surface that increase the likelihood of recharge from floodplain inundation to reach the saturated zone below (Goebel and Knight, 2021; Gottschalk et al., 2017). Thus, levee setbacks designed to maximize groundwater recharge should include some of these surface outcrops to be effective (Maples et al., 2019; Rodriguez et al., 2021).
Levee setback may be one of the solutions for joint flood risk reduction and drought resilience if the groundwater recharge benefits can be quantified. However, the effectiveness of groundwater recharge from levee setbacks has not yet been quantified in detail because it depends on the combination of setback distance and subsurface geology, among other hydraulic and hydrologic site characteristics. Most previous studies have estimated the levee setback distance based on flood risk (e.g., after a levee breach occurred) due to changing channel geometry and associated flood damage cost, with Zhu et al. using true optimization to estimate the costs and benefits of flood control decisions over long periods under changing economic and climatic conditions (Dierauer et al., 2012; Guida et al., 2016; U.S. Army Corps of Engineers USACE, 2012; Zhu et al., 2007). In this study, we quantify the floodplain inundation depth and recharge potential from high conductivity pathways (HCP) connecting the land surface to the aquifer for a range of levee setback distances and subsurface geologic representations to determine the setback distance that provides an optimal compromise. Specifically, this study explores the trade-offs between groundwater recharge potential and floodplain inundation depth for 100 realizations of the subsurface hydrogeology, three flood types, and varying levee setback distances that range from 200 m to 3,200 m.
This study is conducted along the lower Cosumnes River in California, United States. The Cosumnes River (watershed area of 2,460 km2, with elevations between −1 m near the confluence with the Mokelumne River and elevations up to 2,400 m above mean sea level [amsl] at its source in the Sierra Nevada Mountain range) is one of the few unregulated rivers remaining in California and of high importance to maintaining environmental flows and natural habitat for native anadromous fish such as trout and salmon (Whipple, 2018). The river channel is fairly incised, as it is 3–6 m below the adjacent ground surface elevations on the landward side of the levees and naturally high banks that confine the river on both sides. The farmland on both sides of the river is generally leveled for agricultural production, such as vineyards, alfalfa, miscellaneous grain and hay, and corn, but locations where the levees breached in recent history may have slight mounds in the fields. In the middle reaches of the model domain to the south of the river is the town of Wilton, which consists of low-density housing mixed with lands in agricultural production.
As the river is unregulated, it is dependent on winter precipitation and limited snowfall (2002–2022 average total annual precipitation in the watershed was 603.7 mm), which is converted to runoff and limited baseflow in the upper watershed to supply streamflow in the dry season, as the majority of the lower Cosumnes River is losing flow to the groundwater system. The river is a flashy system during precipitation events, which can lead to extreme streamflows (the 5-year flood is ∼640 m3/s, and the 20-year flood is ∼1,100 m3/s), but the dry season tends to see flows between 1 and 10 m3/s at the Michigan Bar stream gauge with the lower reaches drying out entirely in dry years. Deer Creek is a tributary that parallels the Cosumnes River and joins it approximately halfway down the modeling domain. It is estimated to produce flood flows at approximately 14.7% of the stream gauge at Michigan Bar (Whipple, 2018, p. 20). Deer Creek is not represented independently, as it is encompassed by the larger levee setbacks such that it would be part of the main conveyance with levees removed. For this study, a 20 km by 46 km area along the lower Cosumnes River was chosen to study the groundwater recharge potential for different levee setback distances to align with the extent of a geologic model developed for the area (Figure 1).
Figure 1. Map overview of the model domain (rectangle) used for regional analysis showing the maximum levee setback distance of 3,200 m. Black lines show cross-sectional areas established every 2 km along the river that identify 2 km stream reaches used for estimating floodplain inundation extents and depths.
The hydrogeology underlying the Cosumnes River is primarily an alluvial aquifer consisting of the Laguna Formation, which comprises a heterogeneous mix of silt, clay, sand, and gravel that extends approximately 100 m below ground (Bulletin No. 118–3 Evaluation of Groundwater Resources Sacramento County, 1974). The Laguna Formation is pumped by both domestic well users and agricultural producers with wells in the study area that range in depth from approximately 50–100 m (Well Completion Reports, 2020). There are several other lower water-yielding geologic formations underlying the Laguna Formation that dip westward from the Sierra Nevada Mountain range. Directly under the Laguna Formation is the Mehrten Formation, which consists of interwoven tuff-breccia and andesitic sand and clay layers that extend to a depth of 450 m below ground and are pumped by municipalities and limited agricultural producers (Bulletin No. 118–3 Evaluation of Groundwater Resources Sacramento County, 1974).
The lower Cosumnes River area was chosen because it is a river that has been narrowly confined by levees, resulting in disconnected floodplains that historically would have provided groundwater recharge. Now, the aquifer system needs to be recharged due to the loss of floodplain recharge and increased groundwater pumping. Previous research on river restoration, river habitat, and groundwater modeling conducted along the river have highlighted the importance of groundwater management and floodplain restoration (Fleckenstein, 2004; Frei et al., 2009; Jeffres et al., 2008; Moyle et al., 2003; Whipple et al., 2017). In addition, Rodriguez et al. (2021) developed a high-resolution geostatistical model of the hydrogeology of the lower Cosumnes River alluvial aquifer that is used in this study to explore locations of high recharge pathways (Figure 2).
Figure 2. Example realizations of the geostatistical model on the left with local representations of the coarse facies that create high conductivity pathways.
TPROGS (Transition Probability Geostatistical Software) (Carle, 1999; Carle and Fogg, 1996) was used to create 100 equally probable heterogeneous realizations of the geology within the lower Cosumnes River Corridor as shown in Figure 2 (see Rodriguez et al., 2021 for details on geostatistical model inputs and outputs). The geostatistical model used in this article is a spatially extended version of the model developed by Rodriguez et al. (2021) and covers an area of 20 km × 46 km, ranging in vertical depth from 80 m above to −80 m below mean sea level. The geologic model was built based on geologic data from 1,249 well completion reports that were categorized into four hydrofacies, aquifer materials with distinct characteristics: mud, sandy mud, sand, and gravel. TPROGS was used to calculate the transition probabilities between facies (i.e., the probability that a cell will be a different facies given a distance from the current cell) for the model domain, and mean lengths (i.e., the typical length of a connected hydrofacies unit that is not the background unit) were supplied as geologic model input from a previous geologic analysis (Table 1). The geologic model was not constrained to the local geologic data to avoid creating zones of artificially high or low conductivity that are specific to local conditions. This variability among realizations presents a wider range of geologic possibilities to review under levee setback conditions and creates realizations that have equal chances of having HCPs in all river reaches.
Table 1. Hydrofacies definitions with their corresponding physical attributes that make the aquifer materials distinct. Mean lengths are used to inform the geologic model, and the vertical conductivity is used in the recharge calculation.
The horizontal hydraulic conductivity for the four hydrofacies was taken from a previous numerical modeling study of stream–aquifer interactions along the Cosumnes River listed in Table 1 (Fleckenstein et al., 2006). The recharge potential calculation in this study is conducted at the land surface using the first 4 m of geology so that the interconnected coarse facies, with a mean length of 4 m, should remain high conductivity. Meanwhile, the disconnected coarse facies are more likely to include a mud or sandy mud, which will decrease the hydraulic conductivity. A vertical anisotropy, the ratio of horizontal to vertical conductivity of 100, was applied to the horizontal conductivity near the land surface to account for the clogging of the soil zone and for the structural anisotropy introduced by the meandering sand and gravel units that act as high conductivity pathways (HCPs) for water into the aquifer. The hydraulic conductivity values with a vertical anisotropy aligned with previous estimates of streambed conductivity and were found to range from 0.008 m/day to 1.74 m/day for reaches with lengths ranging from 500 to 1,500 m (Niswonger et al., 2008) (Table 1).
The 100 geostatistical realizations were applied to understand the regional variability in HCPs, composed of the sand and gravel hydrofacies, connecting from the ground surface to the aquifer below within each levee setback distance. The location of HCPs was determined by applying a connectivity software (CONNEC3D) to the 100 hydrogeologic realizations, which identifies the cell faces of the coarse facies (i.e., sand and gravel) that connect to each other to identify the unique connected bodies that form high conductivity pathways (Pardo-Igúzquiza and Dowd, 2003). The connected cells with sand and gravel facies were then filtered to select those that connected vertically from the ground surface to the bottom of the model boundary (80 m below mean sea level). The maximum depth to the water table for the upper aquifer (i.e., Laguna formation) in the Cosumnes River basin typically varies at approximately 30 m; hence, choosing pathways that connect the land surface to the bottom of the model domain would ensure a connection from the land surface to the water table in the first aquifer. As such, the connectivity analysis identified the continuous pathways of the high conductivity facies that are likely preferentially used for the flow of water during groundwater recharge (Maples et al., 2019). The numbers of connected HCP cells at the ground surface within a given levee setback distance were then counted to calculate the available area of HCP cells for each stream reach and setback distance scenario (e.g., 200 m–3,200 m) (Supplementary Figure S1).
For this study, levee setback distances ranging from 200 m to 3,200 m in increments of 200 m were chosen to represent the setback possibilities between the current river location (and existing levees) and a wider floodplain. A levee setback distance is measured with a perpendicular line from the edge of a stream cell outward in 200 m steps to match the discretization of the geologic model. These setback distances were chosen to test different floodplain widths and associated floodplain inundation depths and their potential overlap with high conductivity pathways that could promote increased groundwater recharge. While levee setbacks may increase the probability of inundating outcrops of high recharge pathways within the floodplain, these outcrops must be inundated during flood events to transfer flood waters from the surface to the aquifers below. River channel cross sections were sampled from a 10 m USGS digital elevation (DEM) from the channel center to a distance of 3,200 m in both directions perpendicular to the channel center line. The cross sections were sampled at a 200-m longitudinal interval to align with the model grid and were subset to every two river kilometers to avoid overlap (shown in Figure 1). The USGS DEM data were collected between 2017-12-01 and 2018-04-24 when the average daily flow was 24 m3/s; thus, the primary channel was covered with water, and the cross sections do not include the inset river channel, which will result in an overestimate of flood inundation and depth. However, this overestimate will be diminished during large flood events (e.g., 597 m3/s).
To estimate floodplain inundation extents and depths, we estimated the effective inundation depths for each levee setback distance using flood hydrographs based on a flood regime typology study that was conducted for the Cosumnes River by Whipple et al. (2017). Whipple et al. (2017) identified seven characteristic flood regime types for the lower Cosumnes River, using a 107-year-long daily flow time series, to characterize the median peak discharge, event duration, time to peak flow, and flood frequency as criteria. In this study, we use the metrics developed by Whipple et al. (2017) to create triangular synthetic hydrographs on a daily scale as input for the floodplain inundation simulations of the different levee setback distances (Whipple et al., 2017). The flood types used in this study for the inundation simulations are Very Large floods (Type 1), Large and Long floods (Type 2), and Long and Late floods (Type 3) (Figure 3). These flood types were chosen because the Very Large, Large and Long, and Long and Late floods were associated with multiple winter storms producing a major streamflow event, and all Very Large storms were connected to an atmospheric river event over the period of record. Daily peak flows for the three flood types were 597 m3/s, 300 m3/s, and 57 m3/s (15%, 36%, and 53% of the annual expected probability, respectively), and their durations were 90 days, 35 days, and 15 days, respectively. The flood types used in this analysis required peak flows to rise above the average winter baseflow of 23 m3/s to ensure sufficient saturation of the main channel streambed and adjacent floodplain (Whipple et al., 2017). In addition, this study assumed that levees have sufficient height and channels are wide enough to prevent overtopping.
Figure 3. Characteristics of the three flood regime types (Whipple et al., 2017) used in this study to estimate the potential groundwater recharge under different levee setback distances (A). Example stream channel cross section showing the three flood types and corresponding flood elevations to demonstrate how the inundated area is impacted by flow (B).
The effective inundation depth was estimated along each cross section using Manning’s equation, whereby the channel slope, cross-sectional area, wetted perimeter, and hydraulic radius were informed with the cross-sectional elevation data (Equation 1). The longitudinal slope of each 2-km river section was used as Manning’s slope, while Manning’s roughness coefficient was estimated from literature values for a sandy channel with moderate vegetation, as can be observed in the floodplain of the lower Cosumnes River.
where
The flood elevations calculated for each cross section were applied to the 200 m × 200 m grid cells in the groundwater recharge model surrounding the lower Cosumnes River to create flood elevation maps that were then compared against the terrain representations in the geology model to determine the percentage of a cell that would be inundated during a flood event. The average flood depths (i.e., inundation depths) estimated for each of the three flood regime types were used to calculate the vertical hydraulic gradient for the groundwater recharge potential calculation done with Equation 2.
The floodplain recharge potential was calculated on the model grid cell level as the inundation depths varied on the cell-by-cell level due to variations in land surface elevation and the vertical hydraulic conductivity of that model cell. The groundwater recharge potential was calculated for the 100 hydrogeology realizations to evaluate the impact of a range of high conductivity pathway outcroppings in the floodplain on groundwater recharge potential. The focus of the groundwater recharge potential is to identify the trade-offs in total recharge and recharge per unit area (i.e., recharge efficiency) with levee setback distance. Thus, a simplified groundwater system is assumed, which allows comparing recharge amounts under different levee setback distances assuming a thick unsaturated zone where a rising groundwater table does not impede vertical recharge.
The floodplain inundation depths were used to identify cells with >10 cm of inundation depth, that is, cells with substantial inundation beyond the local topographical variability, and these cells were used to calculate recharge potential (Equation 1). The flux of water into the aquifer depends on the vertical hydraulic conductivity of the hydrogeology, the inundation depth (i.e., hydraulic gradient), and the thickness of the clogging layer in the streambed. This relationship is important as a larger setback distance might increase the number of HCP cells activated for recharge but reduce the hydraulic gradient because of shallower inundation, such that the total recharge might be reduced. Groundwater recharge rates were estimated based on the inundation depth estimated for each day of the triangular hydrographs of the three flood types (90 days [Very Large floods], 35 days [Large and Long floods], and 15 days [Long and Late floods]) and then summed up for the event using the 1D vertical Darcy equation, assuming saturation of a near-surface clogging layer, that is, soil zone (Equation 2).
where
For each setback distance and hydrogeology realization, Figure 4 summarizes the HCP areas available for recharge, assuming there are no limitations on water availability; that is, the entire floodplain area within the levees is inundated. The box plots show the variability in HCP area for the 100 hydrogeology realizations for a given levee setback distance.
Figure 4. Box-whisker plots of high-conductivity pathway area added by each setback distance to show the range of results for the 100 hydrogeologic realizations. Blue boxes indicate the interquartile range as defined by the 25th and 75th percentiles. Green lines show the median, and black horizontal bars show the outlier limits, which are 1.5 times the interquartile range beyond the 25th and 75th percentiles of the HCP area across all 100 realizations.
The HCP area added by each levee setback versus the setback presented in Figure 4 shows that there are local maxima (e.g., 600 m and 1,200 m) and minima (e.g., 800 m and 1,400 m). It indicates a steadily increasing relationship between total HCP area and setback distance. As suggested by the interquartile range of each box plot, the HCP area is largest at the 600 m and 1,200 m setback distances, which correspond to the mean length of the coarse facies in the transverse and parallel directions of the river when accounting for the 200 m grid cell size. The local maxima are setback distances where the most gains in HCP area per setback distance occur. Therefore, at these distances, the groundwater recharge potential would be maximized. However, the existence of HCP areas does not guarantee inundation of these areas during flood events because peak flows during the three tested flood types (Type 1, Very Large; Type 2, Large and Long; and Type 3, Long and Late) need to sufficiently spread into the floodplain to inundate HCP areas for longer periods of time to induce recharge (Figures 5A–E). Next, we looked at the inundation of the floodplain within each levee setback distance and for each hydrogeology realization to estimate the inundated HCP areas and the recharge per unit area resulting from the three flood types.
Figure 5. Vertical hydraulic conductivity for one of the 100 hydrogeologic realizations for a levee setback distance of 1,200 m (A). Inundation depth of the floodplain simulated for the Large and Long flood type (Type 2) for a levee setback distance of 600 m (B) and 1,200 m (C). High conductivity pathways activated by the floodplain inundation and their total recharge amounts for a levee setback distance of 600 m (D) and 1,200 m (E). Inundated fraction of the setback area by levee setback distance for the median of the hydrogeologic realizations. Inundated fraction of the setback area by levee setback distance for the median of the hydrogeologic realizations (F). Maps of flood depths at peak flow averaged across the 100 realizations. Results are shown for the two remaining flood types, Type 1 Very Large floods and Type 3 Long and Late floods, for the 600 m (G, H) and 1,200 m (I, J) setback distances, respectively.
For the 600 m setback distance, the total HCP area was 21% of the total setback area, but the inundated HCP area only accounted for 6% of the total setback area under the Large and Long flood type (Figures 5A–E). However, the HCPs accounted for 77% of the median 79 million cubic meters (MCM) of total recharge potential within the setback area. For the 1,200 m setback distance, HCP areas likewise covered 21% of the total setback area, but the inundation fraction was only 4% likely because many of the HCPs outcropped at farther distances from the channel and higher elevations that would require extreme flows to inundate. The median total recharge increased to 124 MCM as more land was accessible within the 1,200 m setback distance for recharge to occur, albeit at slightly reduced inundation depths. The recharge fraction that occurred through HCPs decreased slightly to 73%, which is consistent with the decrease in inundated HCP area for the 1,200 m setback distance. For the upper reaches of the Cosumnes River, the floodplain inundation is mostly limited to areas adjacent to the river channel, while the middle and lower reaches show inundation further away from the channel with larger inundation depths. As shown in Figures 5A–E, most of the inundated HCP areas occur in the lower reaches of the Cosumnes, leading to greater recharge rates.
Inundation simulations were conducted for the three flood types (Type 1, Very Large; Type 2, Large and Long; and Type 3, Long and Late). The 1,200 m setback distance had median inundation fractions of 18%, 11%, and 3%, respectively, while under the shorter setback distance of 600 m, there was a slight increase to 23%, 14%, and 4% (Figures 5B, C, G–J). The smaller setbacks have greater inundation fractions because the flow volume is spread over a smaller setback area.
The majority of floodplain inundation occurs in the lower third of the domain where the river has historically connected to the floodplains due to an absence of levees or levees with lower heights, which reduced channel scour and enabled sediment exchange between the channel and floodplain (Florsheim and Mount, 2002). The spatial distribution of floodplain inundation is consistent between the Very Large and Large and Long flood types, while the Long and Late flood type shows inundation in zones where the depth was already deep for the larger flood types. Figures 5B, C, G–J also show major inundation areas in the lower reaches where historically there have been levee breaches during extreme storm events such as a 58-year flood event in 2017, which reached a peak instantaneous flow of 1,407 m3/s, and in areas where floodplain restoration allows for connection of the Cosumnes River with the floodplain during smaller flood events (Whipple, 2018). This larger spatial extent of inundation in the lower reaches leads to the larger inundated HCPs shown in Figures 5B, C, G–J, where most of the recharge occurs. The greater occurrence of inundated HCPs in the lower Cosumnes River is supported by historical records as groundwater elevations are higher in regions of the lower Cosumnes River where more floodplain inundation occurs, although it has not been previously demonstrated that recharge is driven by HCPs.
However, HCP inundation and the amount of recharge that occurs vary depending on the setback distance. In some cases, the variation can result in an asymmetric distribution of recharge within the floodplain (Figure 5F). The impacts of increased setback distance on flood depth are most noticeable in the lower reaches where the 600 m setback distance results in floodplain inundation on both sides of the channel, but the 1,200 m setback distance is no longer able to inundate parts of the left bank floodplain as there are lower elevation floodplains on the right bank that take most of the flow.
The inundated fraction of the floodplain decreases with increasing setback distance because the setback area increases with each setback distance, and the inundated area decreases as flow is lost to recharge. As a result, higher elevation areas are excluded from inundation. The inundated fraction of the setback areas for all three flood types has a consistent declining pattern but shows a clear offset at the starting point (zero setback distance) as the larger flood flows result in the greater initial inundated area due to their larger overall flow volumes and peak flows. For the three flood types, the inundated fraction varies between 56% and 27% for the 0 m setback distance (i.e., current stream channel bound between the levees), after which it declines steeply to between 19% and 3% at a setback distance of 600 m. This decline can be explained by the fact that the first 200 m and 400 m setbacks expand out of the leveed, incised channel into the historic, larger floodplain of the river. After 600 m, there is a slower decline in the inundated fraction. However, at a setback distance of 1,400 m, there is an increase in inundated fraction likely because the floodplain has a low elevation but not incised region (e.g., backfilled river arm) that is readily inundated without requiring large inundation depths.
Comparing the three flood types, we observe a similar pattern of total recharge potential for each setback distance, although the magnitude of the recharge achieved decreases from Type 1 to Type 3 as the flow volume available for recharge is largest for Type 1 and smallest for Type 3 floods. The total recharge potential under the Type 1 and 2 floods shows an increasing trend for levee setback distances ranging between 0 m and 800 m, followed by an increase in slope for setback distances ranging between 800 m and 1,400 m before leveling off (Figure 6). The Type 3 flood recharge increases more moderately with an increase in levee setback distance. The most pronounced increase in recharge for flood Type 3 can be observed for the levee setback distances ranging between 800 m and 1800 m before leveling off. The fact that the maximum recharge occurs at larger setback distances, despite diminishing inundation fraction, is further illustrated in Supplementary Figure S4, which shows a histogram of the maximum recharge achieved under the different setback distances and flood types. However, the optimal setback distance to maximize recharge also depends on flood type, whereby for smaller floods, maximum recharge is achieved at smaller setback distances because the smaller floods lack the flow to inundate the floodplain under the larger setback distances (Figure 6).
Figure 6. Total groundwater recharge potential under each levee setback distance scenario and flood type. Results are shown for the 100 hydrogeology realizations and the three flood types (Type 1 - Very Large, Type 2 - Large and Long, and Type 3 - Long and Late). Note the difference in the scale of the y-axes.
The recharge per unit area (i.e., recharge efficiency) is the average volumetric recharge rate divided by the total area within the setback distance. Not surprisingly, the recharge efficiency is greatest for a setback distance of 0 m (Figure 7). This is because it has the smallest setback area, so the total recharge with respect to the setback area is large. Because a levee setback project will go beyond 0 m to reduce flood risk and create riparian habitat, it is reasonable to evaluate the benefits among the larger setback distances. When disregarding the smaller setback distances, recharge efficiency peaks at 1,400 m across all flood types and at 800 m for the Type 3 Long and Late flood types (Figure 7). These maxima in recharge efficiency at greater setback distances are likely influenced by the fact that HCPs tend to outcrop more frequently at larger setbacks (e.g., 600 m and 1,200 m) in our 100 hydrogeology realizations such that there may be larger increases in recharge to offset the greater setback area.
Figure 7. Recharge per unit area (m/day), which is the total recharge divided by the total area within each setback distance. Please note the log scale on the y-axis.
Inundating more HCPs during flood events not only promotes greater recharge but also leads to a reduction in streamflow along the course of the river. For the Cosumnes River, we observe that streamflow is significantly reduced in the downstream sections of the river, where most of the floodplain inundation and recharge through HCP areas occur (Figure 8). Loss of streamflow to recharge is further increased at larger setback distances due to less topography in the lower Cosumnes reaches, allowing more HCP areas to inundate and recharge.
Figure 8. The column on the left has a subplot of the peak flow for each cross section for each flood type, and the gray lines represent the median for the 100 realizations for setbacks 0–3,200 m. On the right-hand side, the average depth during the flow event is plotted for the median across realizations.
The recharge to the aquifer demonstrably reduces the channel discharge that is routed to downstream river reaches, also resulting in a reduction in stream stage and maximum inundation depth in downstream riparian areas. Under the 1,400 m setback distance, the average flow reduction in peak flow across the 100 hydrogeologic realizations is 24.4% (±7.4%) for the Type 1, Very Large; 30.8% (±9.7%) for the Type 2, Large and Long; and 34.3% (±10.1%) for the Type 3, Long and Late flood events (Figure 9). Meanwhile, under the 800 m setback distance, peak flow is reduced by 16.7% (±5.7%) for the Very Large, 21% (±7.6%) for the Large and Long, and 29% (±13.3%) for the Long and Late flood type (Figure 9).
Figure 9. Streamflow discharge and recharge achieved at peak flow for each flood type across all 100 realizations under the 1,400 m levee setback.
The HCP analysis demonstrates a periodicity in the relationship of HCP areas available under each levee setback distance that appears to align with the mean lengths of the coarse facies on a regional scale (i.e., the typical length of a connected hydrofacies unit that is not the background unit). Through transition probability geostatistical analysis, Rodriguez et al. (2021) estimated the mean lengths of the coarse facies to be 450 m in the x-direction and 1,100 m for sand and 1,300 m for gravel in the y-direction (Rodriguez et al., 2021), which align with the largest HCP areas achieved under the 600 m and 1,200 m setback distances (Figure 4). This suggests geologic information such as mean lengths can better inform recharge projects as they are indicators of the outcropping of HCPs. Further study of other alluvial aquifers with different mean lengths or geological models where mean lengths are artificially adjusted using similar analysis procedures as presented here could be useful to identify the strength of the influence of the mean lengths on the relationship between HCP area and setback.
In terms of recharge management, the volume of flood flow available will be a limiting factor in achieving recharge in HCP locations located at a greater distance from the channel. Often, elevation increases away from stream channels, requiring greater flow volumes to reach and inundate HCP areas further away from channels, making them unlikely to flood. These effects may be more pronounced in zones with steep topography as outcrops of coarse facies may be located on hills where they are unlikely to be inundated except under the largest flood flows.
The setback distances with the maximum recharge by realization tend to occur at 1,400 m, 2000–2,200 m, and 3,000 m for the Type 1 and 2 floods, with the addition of 800–1,000 m for the Type 3 flood (Supplementary Figure S4). The range of higher setback distance suggests that the increases in the inundated area due to setbacks bring in more recharge despite reduced flood depth. However, the occurrence of maxima at 800–1,000 m for flood Type 3 shows that in some realizations, a lack of flow availability means that fewer HCPs are activated at the greater setback distances. Unlike the maximum total recharge, the maximum recharge per unit area of floodplain is largest at a setback distance of 0 m but also has local maxima at 800 m and 1,400 m setback distances. This contrast in total recharge and recharge per unit area is a result of decreasing recharge efficiency with setbacks due to less flood depth and a smaller inundated area (Figures 5B, C, G–J). Therefore, determining the optimal levee setback distance for recharge and floodplain inundation will require a balance of recharge efficiency and total flood volume available for floodplain inundation. Additionally, as the levee setback distance increases, there is often a significant flood risk reduction benefit, and the cost of rebuilding the levee is reduced as it can be built with reduced sinuosity; thus, the increased land cost may be offset. In our study, the 800 m or 1,400 m setbacks may be optimal due to maxima in recharge at those distances indicating local increases in efficiency.
The proposed levee setback distances are on the higher end of setbacks that are typically implemented due to land availability and cost but fall within the range of setbacks identified by literature when considering multiple benefits. A comparison of levee setback alternatives in Hungary found setbacks of 1,500 m and 2000 m were the best practical alternatives to balance benefits to flood risk reduction, floodplain habitat, project cost, and population impacts (Guida et al., 2016), while a study of flood risk reduction on the Mississippi identified distances of 1,000 m and 1,500 m when combined with property buyouts within the floodplain (Dierauer et al., 2012). These setback ranges align with the scale of the groundwater recharge setback results, but the flood risk reduction and population impacts (e.g., buyouts) will vary by regional characteristics. For example, the Cosumnes River area has a large fraction of agricultural land that might be more reasonably purchased to create levee setbacks and potentially smaller flood events, which would require smaller setbacks to achieve flood risk reduction. Therefore, the Cosumnes River setback results suggest that groundwater recharge benefits require greater setback distances than those for flood risk reduction. Evaluating recharge potential under different flood types further allows assessing the trade-offs between the area needed to access HCPs and the availability of flow for natural floodplain processes; thus, the decision between setbacks with maximum recharge benefits will depend on the needs of the local riparian ecosystem for inundation depths and frequencies (Yarnell et al., 2022).
Floodplain inundation and recharge were simulated for the entire lower Cosumnes River as the geologic model was designed for larger scale analyses, but floodplain inundation occurred predominantly in the lower portion of the model domain where smaller elevation changes dominate the floodplain, thus leading to larger inundated areas (Figures 5B, C, G–J). These lower reaches of the Cosumnes River also have higher groundwater elevations (e.g., 5–15 m below ground) due to more frequent flooding. The recharge potential estimated in this hypothetical study assumed a thick unsaturated zone that did not account for rising groundwater levels; thus, in reality, elevated groundwater levels would reduce recharge in wet years.
Total recharge potential steadily increases with setback distance because it enlarges the area for recharge and access to HCPs. However, the increase in recharge potential varies significantly with setback distance, as the inundated floodplain fraction depends on the elevations within each setback and the HCP area (Figure 6). The Long and Late flood type shows less recharge potential increases with greater setback distance as there is smaller peak streamflow that has a smaller inundated floodplain fraction. While there is more flow availability under the Very Large and Large and Late flood types, these flood types have a 15% and 36% probability of occurrence in the Cosumnes watershed and, therefore, a lower probability of resulting in substantial recharge. Because of their low occurrence frequency (every 6.7 years or 2.8 years), the Type 1 - Very Large and Type 2 - Large and Long floods could also lead to a loss of riparian ecosystem function under greater setback distances, as some species might require more frequent flooding for their survival. The benefit of the Long and Late flood, which occurs every 1.9 years, is that one can expect the floodplain habitat to be active every other year, which would create consistent habitat availability for riparian ecosystem species, specifically the Fall-run Chinook salmon that would greatly benefit from increased food availability (Jeffres et al., 2008; Takata et al., 2017). Focusing on smaller but more frequently occurring flood events for selection of a setback distance would reduce the recharge of the larger flood types but would bring benefits such as increased flow availability for fluvial geomorphological process within the expanded channel (Florsheim and Mount, 2002), increased variability in habitat types created between years (e.g., greater depths when larger flows are available and smaller depths in average years), and leave more flow in the channel for managed aquifer recharge projects that divert flow from the floods.
This study also highlights that floodplain activation by flood waters and inundation depth depends on the overall floodplain topography and that large elevation differences between the river channel and the setback area can inhibit access to HCP areas in the floodplain (Figure 9). A 2D hydrodynamic surface water model would be needed to fully assess the issue of floodplain topographical connectivity with setback distance on a local scale (10–100 m), but with the 1D approximation used in this study, we represented larger dynamics (1–10 km) involving the interplay of inundation depth and loss of streamflow due to recharge. The uniform flow approximation in this study may overestimate the inundation depth of floodplain areas far from the main channel by assuming instantaneous connection when it takes longer for flow to arrive at far areas. A 2D flow model would better represent inundation depths at further distances from the main channel where flows may come from overbank flooding, resulting in smaller initial stages that persist longer due to potential confinement. Inundation depth decreases with increasing setback distance for all three flood types because the flood volumes are spread over larger areas. However, floodplain topography, in combination with a larger setback distance, can, in some cases, increase access to lower floodplain elevations, as shown in Figure 9. For example, the 800 m setback has greater inundation depths than the 1,400 m setback for most stream reaches, with one exception occurring at an upstream distance of 10 km under the Large and Long flood type, likely because the additional setback to 1,400 m opened access to lower elevations (Figure 9). A water manager reviewing these options would need to balance the cost of increasing setback area to increase recharge and floodplain habitat such that if the main goal of the project was to maximize recharge and restoration, then the 1,400 m setback distance would be the more optimal choice.
Rivers in Mediterranean climates have winter flood flows that vary interannually and tend to have native species adapted to survive extreme flood events, while non-native species are more likely to see population declines (Kiernan et al., 2012). The larger levee setback distances, which have greater recharge rates but also greater peak flow reductions, could decrease the severity of winter storms for riparian ecosystems and may ultimately benefit non-native species that depend on more static conditions. In this study, we observed that the mean reduction in peak flow was most pronounced in the most frequent flood type, with an increase in streamflow reduction from 17% to 29% for the 0 m–1,400 m setback distances (Figure 9). These smaller flow events typically drive the winter baseflow in Mediterranean climates (Yarnell et al., 2022), so the reduction in peak flow is beneficial if the loss to recharge leads to locally elevated riparian groundwater elevations that increase baseflow through winter and spring.
The setback distances identified for the lower Cosumnes River in this study that balance groundwater recharge potential, efficiency, and floodplain inundation were 800 m and 1,400 m, respectively. However, these setback distances are larger than distances previously implemented in setback studies focused on flood risk reduction and habitat restoration (Smith et al., 2017; Stofleth et al., 2007), suggesting that studies looking to incorporate recharge need to consider a larger floodplain area. The median total recharge potential predicted with an 800 m setback under the Large and Long flood type, 99 MCM, would be sufficient to offset 84% of the groundwater pumping for irrigation (118 MCM) in the groundwater basin to the north of the Cosumnes River (South American Subbasin Groundwater Sustainability Plan, 2021; Section 2), highlighting that regional scale levee setback would reduce groundwater overdraft. The scale of potential recharge to regional groundwater pumping and to peak streamflow supports the inclusion of groundwater recharge benefits into levee setback feasibility studies that previously ignored recharge or considered recharge an ancillary benefit (Smith et al., 2017; Van Rees et al., 2024).
The goal of this research was to understand the interplay of floodplain recharge and inundation with levee setback distance in heterogeneous aquifers under geologic uncertainty. We acknowledge that our study is, in large part, a theoretical exercise that compares 100 different hydrogeologic realizations of the subsurface to investigate access to high recharge potential zones in the floodplain of levee setbacks. However, it is unknown which of the 100 hydrogeologic realizations is a true representation of the subsurface heterogeneity of the lower Cosumnes River alluvial aquifer. The setback analysis was completed on a regional scale because the hydrogeologic realizations created with a geostatistical model (TProGs) represent aquifer pathways and heterogeneity on a regional scale due to the spatial density of geologic data. As high-resolution geophysical surveying data become more readily available, it could be applied to a proposed levee setback site to identify local aquifer pathways and heterogeneity that are necessary to identify the optimal setback distance for groundwater recharge. Existing geophysical methods such as SkyTEM (i.e., equipment flown by helicopter) could provide reference on a regional scale for where to begin investigating, but it is tow-TEM (i.e., equipment towed behind an all-terrain vehicle) that records high-resolution data in the horizontal and vertical directions that would be ideal for identifying local HCPs (Goebel and Knight, 2021; Pepin et al., 2022). In addition, the extent of levee setback may be limited by infrastructure or high land costs; the impact of these factors will depend on local conditions, such as the value of land in agricultural production. Future work should perform an economic analysis of the joint flood risk reduction, ecological, and groundwater recharge benefits while considering the costs of restoration, such as land value and channel reconstruction.
In conclusion, our modeled results suggest that the location of high conductivity pathways in the floodplain influences the setback distance of maximum groundwater recharge potential due to the interplay of setback distance, opened floodplain area, and floodplain inundation depth. For the lower Cosumnes River, California, we found that 800 m and 1,400 m levee setback distances maximize groundwater recharge while providing floodplain areas that decrease flood depths and increase spatial flood extent with increasing setback distance. Total groundwater recharge under the optimal setback distances (800 m and 1,400 m) sufficiently reduces peak flow under the median of the 100 hydrogeologic realizations tested in this study, such that there are likely beneficial flood risk reductions for neighboring lands. The decision of the optimal setback will depend on the outcomes desired by local water managers, such as larger inundation depths to create floodplain habitat for aquatic species like Chinook salmon or increased spatial extent of floodplains for migratory birds with increased recharge. The groundwater recharge and peak flow reduction due to levee setback are large enough that they should be incorporated in levee setback design to optimize recharge benefits when feasible.
The datasets presented in this study can be found in online repositories. The names of the repository/repositories and accession number(s) can be found below: http://www.hydroshare.org/resource/1d4ff37fbf964efc97937fabef81f145, (Calderwood et al. 2024).
AC: conceptualization, data curation, formal analysis, investigation, methodology, software, visualization, writing–original draft, and writing–review and editing. AR: data curation, formal analysis, and writing–review and editing. LF: funding acquisition and writing–review and editing. HD: visualization, conceptualization, funding acquisition, resources, supervision, and writing–review and editing.
The author(s) declare that financial support was received for the research, authorship, and/or publication of this article. This work was partially funded by the University of California Research Initiatives (Grant LFR-18-548316), the Gordon and Betty Moore Foundation (Grant 7975) , and the National Institute for Food and Agriculture (Grant 2021-69012-35916).
Thank you to the previous graduate students from the Hydrologic Sciences Graduate Group and beyond who performed research on the Cosumnes River and paved the way for this work. Special thanks to Alison Whipple, whose research on flood typology provided the basis for the floods analyzed and for providing the flood hydrograph characteristics. Thanks also to Graham Fogg, who provided feedback on geologic model development and on the early research questions that led to this paper. Thank you to the reviewers for their feedback on the manuscript and insight on the relation to flood modeling and management.
The authors declare that the research was conducted in the absence of any commercial or financial relationships that could be construed as a potential conflict of interest.
All claims expressed in this article are solely those of the authors and do not necessarily represent those of their affiliated organizations, or those of the publisher, the editors, and the reviewers. Any product that may be evaluated in this article, or claim that may be made by its manufacturer, is not guaranteed or endorsed by the publisher.
The Supplementary Material for this article can be found online at: https://www.frontiersin.org/articles/10.3389/fenvs.2024.1421237/full#supplementary-material
Allan James, L., and Singer, M. B. (2008). Development of the lower Sacramento valley flood-control system: historical perspective. Nat. Hazards Rev. 9 (3), 125–135. doi:10.1061/(asce)1527-6988(2008)9:3(125)
Blasch, K., Ferré, T. P. A., Hoffmann, J., Pool, D., Bailey, M., and Cordova, J. (2004) “Processes controlling recharge beneath ephemeral streams in southern Arizona,” in Groundwater recharge in a desert environment, 9. Hoboken, New Jersey: Wiley Online Library, 69–76.
Bulletin No. 118-3 Evaluation of Groundwater Resources Sacramento County (1974). Sacramento, California: CA Department of Water Resources.
Calderwood, A., Rodriguez, A., Foglia, L., and Dahlke, H. (2024). Levee setback distance analysis of groundwater recharge input and output. HydroShare. Available at: http://www.hydroshare.org/resource/1d4ff37fbf964efc97937fabef81f145.
Carle, S. F. (1999). T-PROGS: transition probability geostatistical software, version 2.1. Department of Land, Air and Water Resources. Davis: University of California.
Carle, S. F., and Fogg, G. E. (1996). Transition probability-based indicator geostatistics. Math. Geol. 28, 453–476. doi:10.1007/bf02083656
Cayan, D., Dettinger, M., Stewart, I., and Knowles, N. (2005). Recent changes toward earlier springs—early signs of climate warming in western North America. Watershed Manag. Counc. Networker, 3–7.
Chambers, M., Lammers, R., Gupta, A., Vernon Bilskie, M., and Bledsoe, B. (2024). Modeling the flood protection services of levee setbacks, a nature-based solution. J. Hydrology 634, 131106. doi:10.1016/j.jhydrol.2024.131106
Chambers, M., Van Rees, C. B., Bledsoe, B. P., Crane, D., Ferreira, S., Hall, D. M., et al. (2023). Nature-based solutions for leveed river corridors. Anthropocene 44, 100417. doi:10.1016/j.ancene.2023.100417
Dahl, T. A., Theiling, C. H., and Echevarria, W. (2017). Overview of levee setback projects and benefits. Vicksburg, Mississippi: July, 1–9. doi:10.21079/11681/22767
Dahlke, H. E., LaHue, G. T., Mautner, M. R. L., Murphy, N. P., Patterson, N. K., Waterhouse, H., et al. (2018) “Managed aquifer recharge as a tool to enhance sustainable groundwater management in California: examples from field and modeling studies,” in Advances in chemical pollution, environmental management and protection, 3. Elsevier, 215–275. doi:10.1016/bs.apmp.2018.07.003
Dierauer, J., Pinter, N., and Remo, J. W. F. (2012). Evaluation of levee setbacks for flood-loss reduction, Middle Mississippi River, USA. J. Hydrology 450–451, 1–8. doi:10.1016/j.jhydrol.2012.05.044
Fleckenstein, J. H. (2004). Modeling river-aquifer interactions and geologic heterogeneity in an alluvial fan system, Cosumnes River, California. Davis, California: University of California. Available at: http://search.proquest.com/pqdt/docview/305213487/abstract/141E7D7DD1C579C4129/3?accountid=14505%5Cnhttp://media.proquest.com/media/pq/classic/doc/862919131/fmt/ai/rep/SPDF?hl=fleckenstein.
Fleckenstein, J. H., Niswonger, R. G., and Fogg, G. E. (2006). River-aquifer interactions, geologic heterogeneity, and low-flow management. Ground Water 44 (6), 837–852. doi:10.1111/j.1745-6584.2006.00190.x
Florsheim, J. L., and Mount, J. F. (2002). Restoration of floodplain topography by sand-splay complex formation in response to intentional levee breaches, Lower Cosumnes River, California. Geomorphology 44 (1–2), 67–94. doi:10.1016/s0169-555x(01)00146-5
Fogg, G. E. (1986). Groundwater flow and sand body interconnectedness in a thick, multiple-aquifer system. Water Resour. Res. 22 (5), 679–694. doi:10.1029/wr022i005p00679
Frei, S., Fleckenstein, J. H., Kollet, S. J., and Maxwell, R. M. (2009). Patterns and dynamics of river-aquifer exchange with variably-saturated flow using a fully-coupled model. J. Hydrology 375 (3–4), 383–393. doi:10.1016/j.jhydrol.2009.06.038
Fuentes, I., and Vervoort, R. W. (2020). Site suitability and water availability for a managed aquifer recharge project in the Namoi basin, Australia. J. Hydrology Regional Stud. 27 (December 2019), 100657. doi:10.1016/j.ejrh.2019.100657
Goebel, M., and Knight, R. (2021). Recharge site assessment through the integration of surface geophysics and cone penetrometer testing. Vadose Zone J. 20 (4), e20131. doi:10.1002/vzj2.20131
Gordon, B. A., Dorothy, O., and Lenhart, C. F. (2020). Nutrient retention in ecologically functional floodplains: a review. Water 12 (10), 2762. doi:10.3390/w12102762
Gottschalk, I. P., Hermans, T., Knight, R., Caers, J., Cameron, D. A., Regnery, J., et al. (2017). Integrating non-colocated well and geophysical data to capture subsurface heterogeneity at an aquifer recharge and recovery site. J. Hydrology 555, 407–419. doi:10.1016/j.jhydrol.2017.10.028
Guida, R. J., Remo, J. W. F., and Secchi, S. (2016). Tradeoffs of strategically reconnecting rivers to their floodplains: the case of the Lower Illinois River (USA). Sci. Total Environ. 572, 43–55. doi:10.1016/j.scitotenv.2016.07.190
Hui, R., Herman, J., Lund, J., and Madani, K. (2018). Adaptive water infrastructure planning for nonstationary hydrology. Adv. Water Resour. 118 (October 2017), 83–94. doi:10.1016/j.advwatres.2018.05.009
Jasechko, S., Seybold, H., Perrone, D., Fan, Y., and Kirchner, J. W. (2021). Widespread potential loss of streamflow into underlying aquifers across the USA. Nature 591 (7850), 391–395. doi:10.1038/s41586-021-03311-x
Jeffres, C. A., Opperman, J. J., and Moyle, P. B. (2008). Ephemeral floodplain habitats provide best growth conditions for juvenile Chinook salmon in a California river. Environ. Biol. Fishes 83, 449–458. doi:10.1007/s10641-008-9367-1
Kiernan, J. D., Moyle, P. B., and Crain, P. K. (2012). Restoring native fish assemblages to a regulated California stream using the natural flow regime concept. Ecol. Appl. 22 (5), 1472–1482. doi:10.1890/11-0480.1
Klijn, F., Asselman, N., and Wagenaar, D. (2018). Room for rivers: risk reduction by enhancing the flood conveyance capacity of The Netherlands’ large rivers. Geosciences 8 (6), 224. doi:10.3390/geosciences8060224
Knox, R. L., Wohl, E. E., and Morrison, R. R. (2022). Levees don’t protect, they disconnect: a critical review of how artificial levees impact floodplain functions. Sci. Total Environ. 837, 155773. doi:10.1016/j.scitotenv.2022.155773
Kocis, T. N., and Dahlke, H. E. (2017). Availability of high-magnitude streamflow for groundwater banking in the Central Valley, California. Environ. Res. Lett. 12 (8), 084009. doi:10.1088/1748-9326/aa7b1b
Kourakos, G., Dahlke, H. E., and Harter, T. (2019). Increasing groundwater availability and seasonal base flow through agricultural managed aquifer recharge in an irrigated basin. Water Resour. Res. 55 (9), 7464–7492. doi:10.1029/2018wr024019
Levintal, E., Kniffin, M. L., Ganot, Y., Marwaha, N., Murphy, N. P., and Dahlke, H. E. (2023). Agricultural managed aquifer recharge (Ag-MAR)—a method for sustainable groundwater management: a review. Crit. Rev. Environ. Sci. Technol. 53 (3), 291–314. doi:10.1080/10643389.2022.2050160
Maples, S. R., Fogg, G. E., and Maxwell, R. M. (2019). Modeling managed aquifer recharge processes in a highly heterogeneous, semi-confined aquifer system. Hydrogeology J. 27 (8), 2869–2888. doi:10.1007/s10040-019-02033-9
Maples, S. R., Foglia, L., Fogg, G. E., and Maxwell, R. M. (2020). Sensitivity of hydrologic and geologic parameters on recharge processes in a highly heterogeneous, semi-confined aquifer system. Hydrology Earth Syst. Sci. 24 (5), 2437–2456. doi:10.5194/hess-24-2437-2020
Marchand, J.-P., Biron, P., Buffin-Bélanger, T., and Larocque, M. (2022). High-resolution spatiotemporal analysis of hydrologic connectivity in the historical floodplain of straightened lowland agricultural streams. River Res. Appl. 38 (6), 1061–1079. doi:10.1002/rra.3990
Morin, E., Grodek, T., Dahan, O., Benito, G., Kulls, C., Jacoby, Y., et al. (2009). Flood routing and alluvial aquifer recharge along the ephemeral arid Kuiseb River, Namibia. J. Hydrology 368 (1–4), 262–275. doi:10.1016/j.jhydrol.2009.02.015
Moyle, P. B., Crain, P. K., Whitener, K., and Mount, J. F. (2003). Alien fishes in natural streams: fish distribution, assemblage structure, and conservation in the Cosumnes River, California, USA. Environ. Biol. Fishes 68, 143–162. doi:10.1023/b:ebfi.0000003846.54826.a6
National Research Council (2013). Levees and the national flood insurance program: improving policies and practices. Washington, DC: National Academies Press.
Newcomer Johnson, T., Kaushal, S., Mayer, P., Smith, R., and Sivirichi, G. (2016). Nutrient retention in restored streams and rivers: a global review and synthesis. Water 8 (4), 116. doi:10.3390/w8040116
Niswonger, R. G., Morway, E. D., Triana, E., and Huntington, J. L. (2017). Managed aquifer recharge through off-season irrigation in agricultural regions. Water Resour. Res. 53 (8), 6970–6992. doi:10.1002/2017wr020458
Niswonger, R. G., Prudic, D. E., Fogg, G. E., Stonestrom, D. A., and Buckland, E. M. (2008). Method for estimating spatially variable seepage loss and hydraulic conductivity in intermittent and ephemeral streams. Water Resour. Res. 44 (5), 1–14. doi:10.1029/2007WR006626
O’Geen, A. T., Saal, M. B., Dahlke, H. E., Doll, D. A., Elkins, R., Fulton, A., et al. (2015). Soil suitability index identifies potential areas for groundwater banking on agricultural lands. Calif. Agr 69 (2), 75–84. doi:10.3733/ca.v069n02p75
Opperman, J. J., Moyle, P. B., Larsen, E. W., Florsheim, J. L., and Manfree, A. D. (2017). Floodplains: processes and management for ecosystem services. Oakland, California: Univ of California Press.
Pardo-Igúzquiza, E., and Dowd, P. A. (2003). CONNEC3D: a computer program for connectivity analysis of 3D random set models. Comput. and Geosciences 29 (6), 775–785. doi:10.1016/s0098-3004(03)00028-1
Parsekian, A. D., Regnery, J., Wing, A. D., Knight, R., and Drewes, J. E. (2014). Geophysical and hydrochemical identification of flow paths with implications for water quality at an ARR site. Groundw. Monit. and Remediat. 34 (3), 105–116. doi:10.1111/gwmr.12071
Pepin, K., Knight, R., Goebel-Szenher, M., and Kang, S. (2022). Managed aquifer recharge site assessment with electromagnetic imaging: identification of recharge flow paths. Vadose Zone J. 21 (3), e20192. doi:10.1002/vzj2.20192
Perrone, D., and Rohde, M. M. (2016). Benefits and economic costs of managed aquifer recharge in California. San Franc. Estuary Watershed Sci. 14 (2), 0–13. doi:10.15447/sfews.2016v14iss2art4
Rodriguez, A., Calderwood, A., Gooch, B. T., Kniffin, M., and Foglia, L. (2021). Determining initial viability of local scale managed aquifer recharge projects in alluvial deposition systems. Acque Sotterranee-Italian J. Groundw. 10 (2), 7–18. doi:10.7343/as-2021-500
Serra-Llobet, A., Jähnig, S. C., Geist, J., Kondolf, G. M., Damm, C., Scholz, M., et al. (2022). Restoring rivers and floodplains for habitat and flood risk reduction: experiences in multi-benefit floodplain management from California and Germany. Front. Environ. Sci. 9, 778568. doi:10.3389/fenvs.2021.778568
Smith, D., Miner, S., Theiling, C., Behm, R., and Nestler, J. (2017). Levee setbacks: an innovative, cost-effective, and sustainable solution for improved flood risk management. Environ. Lab. (U.S.). doi:10.21079/11681/22736
Sommer, T., Harrell, B., Nobriga, M., Brown, R., Moyle, P., Kimmerer, W., et al. (2001). California’s Yolo Bypass: evidence that flood control can be compatible with fisheries, wetlands, wildlife, and agriculture. Fisheries 26 (8), 6–16. doi:10.1577/1548-8446(2001)026<0006:cyb>2.0.co;2
South American Subbasin Groundwater Sustainability Plan (2021) South American Subbasin groundwater sustainability agency board, 2. Sacramento Valley, California: Section, 176. Available at: http://www.sasbgroundwater.org/resources.html.
Stofleth, J., Collison, A., Bowles, C., and Andrews, E. (2007). Restoring the floodplain activation flow to the bear river. World Environ. Water Resour. Congr. 2007, 1–34. doi:10.1061/40927(243)367
Swain, D. L., Langenbrunner, B., Neelin, J. D., and Hall, A. (2018). Increasing precipitation volatility in twenty-first-century California. Nat. Clim. Change 8 (5), 427–433. doi:10.1038/s41558-018-0140-y
Takata, L., Sommer, T. R., Louise Conrad, J., and Schreier, B. M. (2017). Rearing and migration of juvenile Chinook salmon (Oncorhynchus tshawytscha) in a large river floodplain. Environ. Biol. Fishes 100 (9), 1105–1120. doi:10.1007/s10641-017-0631-0
Taylor, R. G., Scanlon, B., Döll, P., Rodell, M., van Beek, R., Wada, Y., et al. (2013). Ground water and climate change. Nat. Clim. Change 3, 322–329. doi:10.1038/nclimate1744
U.S. Army Corps of Engineers (USACE). (2012). Assessment of conceptual nonstructural alternative levee setbacks along the Missouri river (lower L-575/upper L-550 and lower L-550) final report.
Van Rees, C. B., Chambers, M. L., Catalano, A. J., Buhr, D. X., Mansur, A. V., Hall, D. M., et al. (2024). An interdisciplinary overview of levee setback benefits: supporting spatial planning and implementation of riverine nature-based solutions. WIREs Water 11, e1750. doi:10.1002/wat2.1750
Watson, A., Kralisch, S., van Rooyen, J., and Miller, J. (2021). Quantifying and understanding the source of recharge for alluvial systems in arid environments through the development of a seepage model. J. Hydrology 601, 126650. doi:10.1016/j.jhydrol.2021.126650
Well Completion Reports (2020). Calif. Nat. Resour. Agency. Available at: https://data.cnra.ca.gov/dataset/647afc02-8954-426d-aabd-eff418d2652c/resource/8da7b93b-4e69-495d-9caa-335691a1896b/download/wellcompletionreports.csv.
Whipple, A. A. (2018) “Managing flow regimes and landscapes together: hydrospatial analysis for evaluating spatiotemporal floodplain inundation patterns with restoration and climate change implications,”. [University of California Davis]. Available at: https://www.alumni.ucsd.edu/s/1170/rd18/home.aspx.
Whipple, A. A., Viers, J. H., and Dahlke, H. E. (2017). Flood regime typology for floodplain ecosystem management as applied to the unregulated Cosumnes River of California, United States. Ecohydrology 10 (5), 1–18. doi:10.1002/eco.1817
Yarnell, S. M., Willis, A., Obester, A., Peek, R. A., Lusardi, R. A., Zimmerman, J., et al. (2022). Functional flows in groundwater-influenced streams: application of the California environmental flows framework to determine ecological flow needs. Front. Environ. Sci. 9. doi:10.3389/fenvs.2021.788295
Yoder, A. M. (2018) “Effects of levee-breach restoration on groundwater recharge,” in Cosumnes River floodplain, California. Davis: University of California.
Keywords: levee setback, groundwater recharge, aquifer heterogeneity, floodplain inundation, ecosystem function, flood management
Citation: Calderwood AJ, Rodriguez A, Foglia L and Dahlke HE (2024) Evaluating levee setback distance for the co-benefits of groundwater recharge and riparian ecosystem function. Front. Environ. Sci. 12:1421237. doi: 10.3389/fenvs.2024.1421237
Received: 22 April 2024; Accepted: 11 November 2024;
Published: 06 December 2024.
Edited by:
Andrea Ghermandi, University of Haifa, IsraelReviewed by:
Anitra Pawley, California Department of Water Resources, United StatesCopyright © 2024 Calderwood, Rodriguez, Foglia and Dahlke. This is an open-access article distributed under the terms of the Creative Commons Attribution License (CC BY). The use, distribution or reproduction in other forums is permitted, provided the original author(s) and the copyright owner(s) are credited and that the original publication in this journal is cited, in accordance with accepted academic practice. No use, distribution or reproduction is permitted which does not comply with these terms.
*Correspondence: Andrew J. Calderwood, Y2FsZGVyd29vZGFqQGdtYWlsLmNvbQ==
Disclaimer: All claims expressed in this article are solely those of the authors and do not necessarily represent those of their affiliated organizations, or those of the publisher, the editors and the reviewers. Any product that may be evaluated in this article or claim that may be made by its manufacturer is not guaranteed or endorsed by the publisher.
Research integrity at Frontiers
Learn more about the work of our research integrity team to safeguard the quality of each article we publish.