- 1Iowa Geological Survey, University of Iowa, Iowa City, IA, United States
- 2Department of Civil and Environmental Engineering, University of Iowa, Iowa City, IA, United States
- 3Department of Civil, Architectural, and Environmental Engineering, Missouri University of Science and Technology, Rolla, MO, United States
Constructed wetlands are standard conservation practices used to reduce nitrate loads in agricultural watersheds. Many studies have examined the efficiency of denitrification in wetlands under various scenarios, but quantifying the watershed-scale impact of wetlands on downstream nitrate levels is rarely done using field observations. In this study, we estimated nitrate removal in a constructed wetland in the headwaters of Mud Creek, a HUC12 watershed in eastern Iowa, from May–September 2022 and May–September 2023 (a ten-month period). We also measured nitrate loads at four successive downstream sites, three along Mud Creek and one below its confluence with the larger Cedar River. The wetland removed 6,200 kg of nitrate (74% of total inputs). At the three downstream locations in Mud Creek, the percentage of each site’s total nitrate load removed by the wetland decreased to 19, 8.6, and 4.1%—this latter value represents the wetland’s influence on nitrate removal in the entire Mud Creek basin. The wetland’s impact of nitrate loads in the Cedar River was negligible (reduction of 0.02%). The percentage of a site’s drainage area treated by the wetland approximately followed a 1:1 relationship to that site’s percent reduction in nitrate. Profiles of nitrate concentrations in Mud Creek notably varied pre- and post-wetland. Concentrations before the installation steadily decreased along the waterway, while post-wetland concentrations rapidly decreased directly downstream of the wetland and steadily increased at each succeeding site. Our results demonstrate that while the wetland successfully lowered local nitrate levels, its effect on the basin’s overall nitrate loads was minimal. Achieving nutrient reduction goals at the watershed scale solely using constructed wetlands appears infeasible given that the required number of practices greatly exceeds current efforts.
1 Introduction
Waterborne nitrate-nitrogen (nitrate) is a major pollutant of concern in the U.S. Corn Belt (Chambers et al., 2008; Schilling et al., 2012). Its presence drives stream and lake eutrophication at local and regional scales (Smith and Schindler, 2009), and downstream export has contributed to widespread hypoxic conditions in the Gulf of Mexico (Burkart and James, 1999; Rabalais et al., 2002). These eutrophic conditions have detrimental impacts on aquatic ecological health (Singh et al., 2022) and episodically spur harmful algal blooms, which present acute risks for humans and animals upon contact (Hallegraeff, 2003). Nitrate concentrations in drinking water also present an acute threat to human health (Fewtrell, 2004), and they may be carcinogenic under prolonged periods of exposure (Ward et al., 2018).
Nitrate delivery to waterbodies has been linked to agricultural activities (Syswerda et al., 2012), especially intensive row crop production (Randall and Mulla, 2001). Nitrate loss in the U.S. Corn Belt is among the highest per unit area in the nation (Aulenbach et al., 2007; Schilling and Libra, 2000). Specific states, such as Iowa (Jones et al., 2018b), contribute disproportionate amounts of nitrate to downstream waterbodies (Goolsby et al., 2001). In many watersheds, stream nitrate concentrations repeatedly exceed drinking water standards and threaten water suppliers using surface intakes (Jha et al., 2010; Jones et al., 2020). Municipal water suppliers must ensure nitrate levels in their finished drinking water are below the maximum contaminant level of 10 mg/L, and the expenses and labor associated with these treatment processes place financial burdens on numerous Midwest communities (Christianson et al., 2013; Vedachalam et al., 2019). Furthermore, surface water nitrate concentrations are often far greater than levels suggested by nutrient criteria meant to benefit ecological health (Dodds and Welch, 2000; Jones et al., 2018c).
In light of this situation, considerable effort has gone into lowering nitrate levels in Midwestern rivers and streams. Many states have initiatives with explicit nutrient reduction goals (Anderson et al., 2016; David et al., 2013; Scavia et al., 2017). In Iowa, efforts are guided by the Iowa Nutrient Reduction Strategy (Iowa State University, 2012). Additionally, specific waterbodies often have limits on allowable nutrient levels, which are defined through the creation of total maximum daily loads (TMDLs). Iowa has approximately 20 TMDLs for nutrients. Some of the most prominent TMDLs for nitrate include those created for the Cedar (Hutchinson and Christiansen, 2013), Des Moines (Schilling and Wolter, 2009), and Raccoon Rivers (Jha et al., 2010).
Conservation plays a large role in all of these reduction efforts, and numerous conservation strategies have been developed to reduce nitrate loads (Butt and Brown, 2000; Conley, 1999). Constructed wetlands have been shown to be among the most effective nitrate reduction practices (Crumpton, 2001; Haas et al., 2017), especially in strategies utilizing conservation planning tools (Scavia et al., 2017; Weber et al., 2018). Constructed wetlands are engineered systems designed and built to take advantage of natural processes typically found within the vegetation, soils, and microbiomes of the wetlands (Vymazal, 2007). Herein, all mention of wetlands refers specifically to constructed wetlands built for the purpose of improving water quality.
Although wetlands can treat a variety of waterborne pollutants, most have proven effective at fostering denitrification and removing large quantities of nitrate (Spieles and Mitsch, 1999). Not all nitrate removal in constructed wetlands occurs via denitrification (Martin and Reddy, 1997). Some nitrate is assimilated into dissolved or particulate organic nitrogen, which can be discharged downstream or retained in the wetland (Lee et al., 2009).
In agricultural landscapes, wetlands are usually located along the main channel of a stream or at major tile drainage outlets draining farmland catchments (Crumpton et al., 2006). Inflow water is slowed within the wetland, and the submerged vegetation and soils produce a subaqueous environment conducive to denitrification (Vymazal, 2007). The specific rate at which denitrification occurs depends on many factors, including incoming nitrate concentrations, water temperatures, flow rates, vegetation species or maturity, and residence time (Collins and Gillies, 2014; Lee et al., 2009; Lin et al., 2008).
A great deal of study has been conducted on maximizing wetland removal efficiency (Cheng et al., 2020; Crumpton et al., 2020; Singh et al., 2019). In many instances, wetlands have removed substantial portions (>50%) of their nitrate inputs (Crumpton et al., 2006; Hunt et al., 1999; Maxwell et al., 2017), leading to large reductions in local nitrate concentrations (Drake et al., 2018). While these nitrate reductions are a positive outcome, the benefits of a particular wetland on downstream water quality become increasingly hard to measure as more untreated water reaches the stream from larger drainage areas and dilutes the treated water containing fewer nitrates (Ikenberry et al., 2014; Kovacic et al., 2000). Loads removed by a wetland often become minuscule compared to loads in larger downstream waterbodies. For example, Drake et al. (2018) reported nitrate removal of 66,800 kg from 2014–2016 (a reduction of 49%) in a constructed wetland near the headwaters of the Cedar River in eastern Iowa. Compared to the nitrate flux of the entire Cedar River basin across the same timeframe (120 Gg; (Jones et al., 2018b)), this value represents a reduction of only 0.05%.
The downstream impacts of wetlands on river water quality have been estimated using load calculations (Drake et al., 2018) or watershed-scale models (Böhlke et al., 2009; Conan et al., 2003; Mitsch et al., 2005), but rarely have impacts been verified with field observations. Quantifying the spatial rate at which nitrate removal from a wetland diminishes with increasing watershed area remains largely unexplored. In this study, we evaluated the downstream impacts of a newly constructed wetland on nitrate levels in the highly agricultural Mud Creek watershed in east-central Iowa. After quantifying nitrate removal in the constructed wetland, we utilized high-resolution nitrate sensors in four downstream monitoring locations and in the larger receiving river system to document how the impacts of the wetland diminish with watershed scale. Specifically, our objectives were to: 1) calculate the nitrate removed by the wetland during May–Sep in 2022 and May–September 2023; 2) quantify nitrate loads in Mud Creek at four successive downstream locations to assess the impact of the wetland on downstream water quality, and 3) evaluate how the longitudinal profile of nitrate concentrations along Mud Creek varied before and after wetland installation.
2 Methods
2.1 Site description and setting
Mud Creek is a perennial stream draining a 115 km2 watershed (HUC12 basin 070802051104) in Benton County, Iowa (Figure 1). Mud Creek outfalls into the Cedar River—a much larger waterway with 15,800 km2 of drainage area at its confluence with Mud Creek (∼five HUC08 basins). Mud Creek is located in the Iowan Surface landform region (Prior, 1991), an area marked by low-relief topography and poorly drained glacial till plains overlying shallow sedimentary bedrock (mainly limestone, dolomite, and shale (Witzke, 1998)). The Cedar River basin also predominantly lies within the Iowan Surface and contains hydrologic and geologic behavior similar to that of Mud Creek.
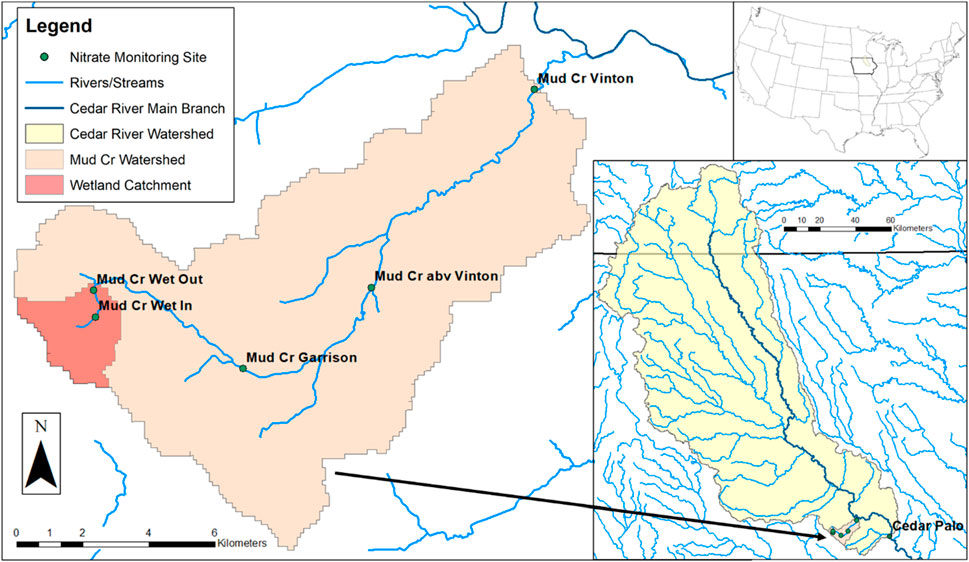
Figure 1. The nitrate monitoring sites and watersheds for the Cedar River, Mud Creek, and wetland. The main stem of the Cedar River is displayed in dark blue.
Land use in Mud Creek watershed is agricultural, consisting of approximately 80% of the land area in row crop production (corn and soybean), with small amounts (<5% of the total watershed) of urban land use near Mud Creek’s outfall in the town of Vinton, IA (Iowa DNR NRGIS, 2009). Annual precipitation in Benton County is approximately 850 mm (annual average from 1895–2023), with a majority of rainfall (∼2/3) occurring during the spring and summer (NOAA, 2024). Artificial tile drainage is widespread and contributes, along with groundwater movement, to perennial flows at Mud Creek’s outlet. The combination of high agricultural intensity and artificial drainage has resulted in impairments to surface water quality in the region (Brendel and Soupir, 2017; Kalkhoff et al., 2000; Squillace and Engberg, 1988), with nitrate being a particular pollutant of concern (Jones et al., 2018c; Schilling and Libra, 2000). Hence, the processes driving nitrate transport in Mud Creek are found throughout the overall Cedar River watershed, and the eutrophication challenges and remediation strategies conducted in Mud Creek are likewise taking place throughout the Cedar River basin (Weber et al., 2018).
2.2 Wetland construction
In 2021, a wetland was constructed near the headwaters of Mud Creek (Figure 1). The wetland is located along Mud Creek’s main channel and contains a surface area of 5.1 ha. The design and installation of the wetland was completed as part of the Iowa Watershed Approach, a large-scale initiative to improve water quality and reduce flooding in nine major watersheds (Weber et al., 2018). Funding for the Mud Creek wetland was provided by both the Iowa Watershed Approach (https://iowawatershedapproach.org/) and the Environmental Protection Agency (https://www.epa.gov/), with the goal of lowering local nitrate concentrations and reducing flood peaks.
A local engineering firm completed the wetland’s technical design. The wetland was constructed in-stream, with the main channel of Mud Creek acting as its primary inlet and outlet. It was designed to have a maximum depth of 2.1 m and a storage capacity of 160,000 m3. Primary and auxiliary spillways were constructed to contain the 24-h design storms for return periods of 25 and 50 years, respectively. With the cooperation of the local landowner, row crop lands adjacent to the Mud Creek channel were converted to grass and native vegetation and incorporated into the wetland’s storage area. The wetland became fully operational in August 2021—requiring a few months to fill with water post-construction. The total cost of the wetland, including its design, construction, and permitting efforts, was approximately $600,000.
Compared to the larger Mud Creek watershed, the tributary area of the wetland is overwhelmingly agricultural (>95%). Approximately 3.37 km2 of catchment area drains to the wetland via Mud Creek’s main channel, whereas an additional 1.76 km2 of adjacent farmland drains to the wetland via nine tile lines and one ephemeral creek. In total, the wetland treats an area of 5.18 km2, which is 4.58% of the Mud Creek watershed. Mud Creek produces consistent flow at the wetland’s inlet and outlet under normal hydrologic conditions. However, flow can cease during extended dry periods, resulting in stagnant water in the main channel. Tile lines typically flow during the spring and early summer but can also dry out in the absence of wet weather. After discharging from the wetland’s outlet, flow continues unimpounded along Mud Creek for 21.9 km until it reaches the Cedar River. Overall, the wetland was typical (in terms of its dimensions and location) of those commonly constructed along stream channels in agricultural watersheds (Crumpton et al., 2020; Lemke et al., 2022; Messer et al., 2021).
2.3 Monitoring and data collection
Streamflow and nitrate concentrations were monitored at five locations along Mud Creek to assess the wetland’s impact on stream conditions. Table 1 contains each location’s relevant metadata, including their abbreviated names. Two of the monitoring locations included the wetland inlet (Mud Cr Wet In) and outlet (Mud Cr Wet Out). The remaining three sites were evenly spaced along Mud Creek, with the final location (Mud Cr Vinton) 1.6 km upstream of its confluence with the Cedar River.
Nitrate concentrations were monitored using in situ probes (Hach Nitratax Plus SC, 5 mm path length). When deployed, these sensors record nitrate concentrations every 15 min using ultraviolet absorption. This sensor model has proven proficient in providing continuous unbiased measurements of riverine nitrate in Iowa (Cao et al., 2023; Drake et al., 2018; Jones et al., 2017; Jones et al., 2020). In early 2020, nitrate sensors were deployed at each site except Mud Cr Wet Out, which was installed in July 2021 following wetland construction. Several sensors were installed in 2020 to gauge Mud Creek’s longitudinal nitrate profile pre-wetland. The nitrate sensors were removed during winter and thus followed a deployment schedule from approximately roughly May to November each year from 2020–2023. Supplementary Figure S1 summarizes the sensors’ operational timeframes at each location.
Nitrate concentrations measured using the probes were validated by collecting 30 grab samples at four sensor locations during 2020 (R2 = 0.91). Nitrate concentrations in the grab samples ranged from 2.1–17.6 mg/L and were measured by the State Hygienic Laboratory at the University of Iowa. A Bland-Altman analysis confirmed agreement between measurement techniques at all sites (Dewitte et al., 2002). Furthermore, all probes were shipped to their parent manufacturer following their retrieval each winter. The manufacturer inspected and recalibrated the equipment to ensure continued functionality before redeployment in the spring. Mud Creek’s continuous nitrate concentrations are publicly available through the Iowa Water Quality Information System (IWQIS: https://iwqis.iowawis.org/app/), a web portal containing continuous, in situ water quality data across Iowa. We have also included the specific nitrate data from our analysis within this study’s Supplementary Material.
Streamflow values were estimated using stage-discharge relationships at the five sites (Quintero et al., 2021). Topographic surveys were conducted to determine the channel’s cross-sectional geometries. Stream stages were monitored at four locations using stream sensors developed by the Iowa Flood Center (IFC); these sensors were installed on roadway bridges and used ultrasonic technology to measure river levels every 15 min (Kruger et al., 2016). No overhead bridge is present near the Mud Cr Wet Out site, so a water level transducer (In-Situ Level TROLL 400 Data Logger) was used to monitor stage elevations.
During a preliminary analysis in 2022, we noticed biases occurring in the values reported by IFC sensors, whereby stage was overestimated during low-flow conditions. We subsequently installed water level transducers at three Mud Creek sites (Mud Cr Wet In, Mud Cr Garrison, and Mud Cr Vinton) in early 2023. The transducers recorded stage elevations alongside the IFC sensors in 2023. These new stage data were used to correct the biases reported by the IFC sensors in 2022. Consequently, 15-min stage records were obtained for the 2022–2023 measurement period. Stages were converted to corresponding streamflow values using the continuous slope-area method (Lee et al., 2017). Streamflow estimates were validated using an acoustic Doppler velocimeter (SonTek FlowTracker2 Handheld-ADV) to measure flow on-site 10–12 times at each monitoring location in 2020 and 2023. Measured streamflow values ranged between 0.02 and 4.4 m3/s, and all on-site measurements were within 15% of estimated discharges. All flow values are publicly available through the Iowa Flood Information System (https://ifis.iowafloodcenter.org/ifis/app/), a web portal that contains continuous flow measurements collected by the IFC sensors (Krajewski et al., 2017). The specific water depth and streamflow datasets used in this study have also been included in the Supplementary Material.
The United States Geological Survey (USGS) also operates a stream gauge along the Cedar River 34.9 km downstream of Mud Creek’s outfall near Palo (USGS gauge 05464420). Both streamflow and nitrate have been continuously monitored at this site since 2012 using standard USGS protocols (Lins and Slack, 1999; Pellerin et al., 2014). To estimate the wetland’s nitrate inputs not captured by the main inlet, tile lines and a creek draining directly to the wetland were monitored throughout May–October 2023 using grab samples collected every 2 weeks. Flow rates were estimated during sample collection by simply timing the rate at which discharge filled a 19-L bucket. Upon collection, grab samples were returned to a laboratory and analyzed for nitrate using a probe identical to those deployed in the field.
2.4 Nitrate load calculations
Nitrate loads were calculated using nitrate concentrations and corresponding flow values at all six monitoring locations. Load calculations presented in this study describe five-month periods (May–September) in 2022 and 2023. This timeframe was constrained by the deployment schedules of the nitrate sensors, but it broadly aligns with typical seasonal patterns of nitrate loads in Iowa (Zhang and Schilling, 2005). Most wetland denitrification also occurs during this period due to the higher nitrate concentrations, greater streamflow, and warmer temperatures (Crumpton et al., 2020; Stein and Hook, 2005).
Daily values were calculated for nitrate concentrations and streamflow by taking arithmetic means of the 15-min data. Nitrate concentrations were interpolated during occasional instances of sensor fouling (<5% of days), as linear interpolation has proven effective for stream nitrate (Schilling et al., 2017). Daily loads were then found at each site by multiplying mean nitrate concentrations and flow values. This daily load calculation method was used due to short-term variabilities of river stage measurement documented in our bridge sensor equipment. Bridge sensor readings can occasionally contain aberrant values, but these largely balance out on a daily scale (Kruger et al., 2016; Quintero et al., 2021). Other researchers have successfully utilized this method when estimating nitrate loads over months or years (Jones et al., 2018a; Pellerin et al., 2014; Zimmer et al., 2019). During days with no streamflow (i.e., stagnant water), loads were set to 0. Loads were aggregated across the entire analysis period (Table 2; May–September 2022 and May–September 2023) and for each individual month (Table 3). Yields were also calculated by dividing a site’s nitrate load by its drainage area.
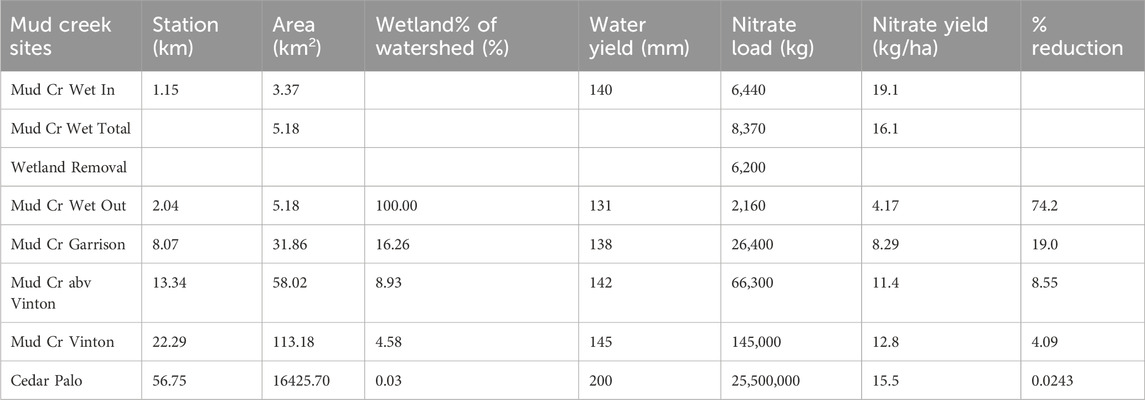
Table 2. Nitrate loads (from May - September 2022 and May - September 2023) and the corresponding reductions caused by the wetland. “Wetland% of Watershed” column refers to the percentage of the site’s drainage area that is treated by the wetland.
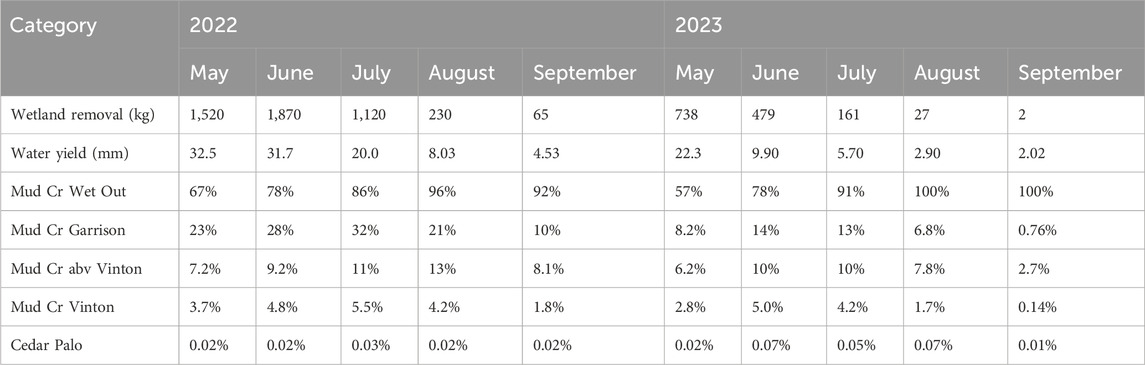
Table 3. % Reduction of nitrate loads by month. Wetland removal refers to the nitrate retained by the wetland during a given month, while the water yield refers to the monthly streamflow yield at Mud Cr Vinton.
Nitrate loads entering the wetland through the peripheral tile lines were correlated to those entering the wetland through the main channel. This relationship suggested that tile nitrate consistently accounted for 20% of the nitrate inflow from the main channel (Supplementary Figure S6). We thus multiplied the daily loads at Mud Cr Wet In by 1.2 to estimate the total nitrate load entering the wetland (Mud Cr Wet Total). The mass of nitrate removed by the wetland (Wetland Removal) was calculated by taking the difference between the total nitrate entering it (Mud Cr Wet Total) and the nitrate discharged at the outlet (Mud Cr Wet Out). More precisely, the Wetland Removal term refers to all nitrate transformed or retained within the wetland; therefore, this calculation represents a simple mass balance of the wetland’s nitrate inputs and outputs. To contextualize the mass of nitrate removed by the wetland, we defined the metric % Reduction, which was calculated as
where Nitrate Load is a site’s nitrate flux across the analysis period. This percentage is bounded between 0% and 100% and is the nitrate removed by the wetland divided by the sum of this removal and a location’s observed nitrate load. % Reduction thus quantifies the wetland’s nitrate loss in terms of a site’s overall nitrate load.
We also calculated another metric termed Wetland% of Watershed (Table 2). This term refers to the percentage of a site’s drainage area treated by the wetland. For instance, the Mud Cr Garrison site has a tributary area of 31.9 km2. The wetland’s tributary area is 5.18 km2, which comprises 16.3% of the total drainage area of Mud Cr Garrison.
3 Results
3.1 Observed nitrate concentrations
Figure 2 displays the daily nitrate concentrations estimated at each monitoring. Continuous daily records of nitrate concentrations were available from May to Nov each year at most sites. Retrieval and storage of the equipment was necessitated during the winter and early spring, and occasional short-term gaps in the nitrate records occurred due to sensor fouling (4.3% of daily nitrate estimates) or low water levels (6.3% of daily nitrate estimates). In particular, low water levels observed during the July–September 2023 period at the Mud Cr Wet Out resulted in missing nitrate values.
Concentrations were highest at the Mud Cr Wet In (median of 11.5 mg/L) and lowest at the Mud Cr Wet Out (median of 1.94 mg/L). Of the remaining sites, Cedar Palo generally had lower nitrate levels (median of 4.06 mg/L) compared to Mud Cr Vinton (7.55 mg/L) and Mud Cr Garrison (5.56 mg/L). A wide range of nitrate concentrations were observed throughout Mud Creek, with minimum values near 0.10 mg/L and a maximum value measured at Mud Cr Wet In (19.5 mg/L). Maximum concentrations at the remaining sites ranged from 12.3 to 15.1 mg/L. Nitrate concentrations typically peaked in May and June before steadily declining throughout the rest of the summer. The lowest concentrations (0.10 mg/L) were routinely measured in Aug and Sep, followed by a rise in concentrations in late Sep and October (Figure 2). Average annual nitrate concentrations were highest in 2020—the only year with above-average precipitation.
We also utilized the monitoring data to investigate the spatial pattern of nitrate concentrations in Mud Creek before and after the wetland installation. Assessing the wetland’s impact on local nitrate concentrations is of great interest to stakeholders, as nutrient concentrations portend eutrophic conditions. Lower nitrate concentrations in Mud Creek improve the water’s drinkability and reduce the occurrence of algal blooms. The arithmetic mean of daily nitrate concentrations at each site in 2020 (pre-wetland) and 2022 (post-wetland) during the five-month analysis period (May–September) was evaluated. [Note that other years were not included in this analysis due to confounding factors: 2021 was the year the wetland was constructed, and 2023 was confounded by drought.] While 2020 and 2022 provide concise comparison points of the pre- and post-wetland conditions, this comparison does not capture differences in hydrologic conditions between these 2 years. These longitudinal profiles display local nutrient levels but do not describe the mass flux during these periods.
The longitudinal profiles of mean nitrate concentrations in Mud Creek in 2020 and 2022 show marked differences between these 2 years. In the pre-wetland condition, nitrate concentrations monotonically declined as water moved downstream. A steady decrease in stream nitrate concentrations is consistent with Peterson and Benning (2013) and Schilling et al. (2012), who reported nitrate concentrations highest in the headwaters of agricultural streams that gradually declined in downstream catchments with larger drainage areas. In contrast, the 2022 longitudinal profile shows a sharp drop in nitrate concentrations directly downgradient from the wetland—a clear sign of its efficacy in nitrate reduction. Following the sizable drop, concentrations rose at each subsequent site along Mud Creek, reaching levels near those measured in the Cedar River at Palo. This behavior was similar to the nitrate yields shown in Supplementary Figure S5.
3.2 Wetlands loads and nitrate removal
Daily nitrate loads were calculated at all six monitoring sites during the May–September timeframe in 2022 and 2023 (Figure 3). However, it is important to note that Eastern Iowa experienced significant drought conditions across the two-year monitoring period. Indeed, Palmer Drought Severity Index (PDSI) values dropped below −2 in 2022 and −4 in 2023 (Supplementary Figure S2), indicating moderate to severe drought periods. Nearby USGS gauges recorded annual streamflow totals far below average (Supplementary Figure S3). Consequently, the results from this study should not be viewed as representing the full range of hydrologic conditions but as a period marked by drier-than-average streamflow.
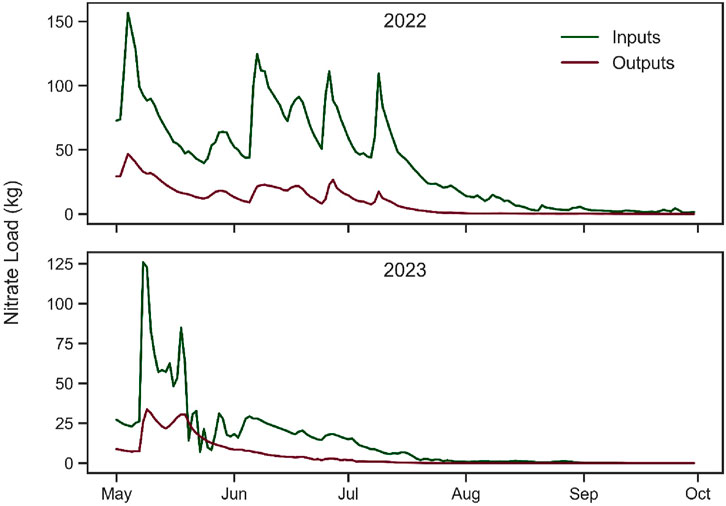
Figure 3. Estimated daily nitrate loads into and out of the wetland for 2022 (top) and 2023 (bottom).
Daily nitrate loads into the wetland ranged from 0 to 151 kg (Figure 3). Loads exported from the wetland ranged from 0 to 48 kg. The days with the highest loads corresponded to the highest flow days, most of which took place in May, Jun, and Jul in 2022. Following a final wet weather event in July 2022, export loads gradually decreased through the remainder of the year. Two wet weather events occurred in May 2023, followed by the onset of significant drought conditions and diminished loads in Jun and Jul. By Aug, flows into and out of the wetland had completely dried up, resulting in days with 0 kg of nitrate load.
We estimated that 6,440 kg entered the wetland through the main channel across this ten-month period. When incorporating nitrate flux from the surrounding tile lines, estimated nitrate inputs to the wetland totaled 8,370 kg. Nitrate loads at the wetland outlet were 2,160 kg, indicating that the wetland removed 6,200 kg. This implied a % Reduction of 74.2%—meaning 74.2% of nitrate inputs were removed by the wetland. Nitrate yields, defined as a site’s nitrate load from the ten-month analysis period divided by its tributary area, varied dramatically upstream (16.1 kg/ha) and downstream (4.17 kg/ha) of the wetland (Supplementary Figure S5).
3.3 Downstream loads and wetland impacts
Nitrate loads downstream of the wetland were much larger than the influx into the wetland (Supplementary Figure S4), which was expected given the increased drainage area for each successive monitoring location. Loads at the Mud Cr Garrison, Mud Cr abv Vinton, and Mud Cr Vinton sites were 26,400, 66,300, and 145,000 kg, respectively. The % Reduction declined monotonically from upstream to downstream (Figure 4), as the sites had reductions of 19.0% (Mud Cr Garrison), 8.55% (Mud Cr abv Vinton), and 4.09% (Mud Cr Vinton). This latter value effectively means the wetland reduced the nitrate load at the Mud Creek outlet by 4.09%. Loads at the Cedar Palo site were more than two orders of magnitude (25,500,000 kg) larger than Mud Creek, and the % Reduction due to the wetland was essentially negligible at the scale of the Cedar River (0.0243%).
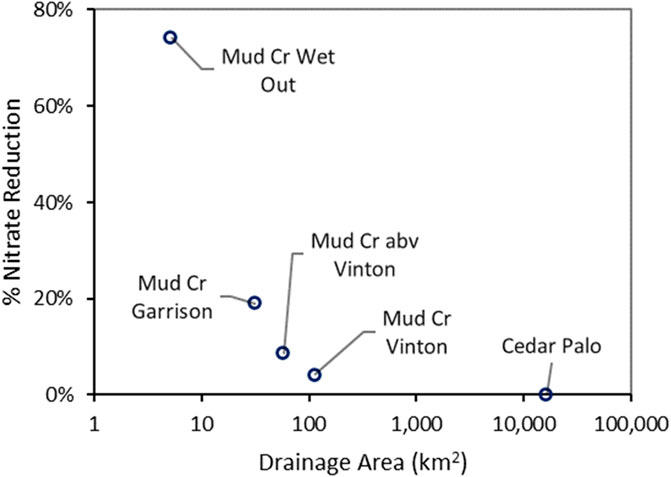
Figure 4. Percent nitrate reduction versus drainage area for the five nitrate sensor sites downstream of the wetland.
Furthermore, we plotted each site’s % Reduction value against its Wetland% of Watershed (Figure 5). The relation of % Reduction to Wetland% of Watershed was essentially linear and followed a 1:1 ratio, suggesting that the percentage of nitrate mass removed by a wetland was generally equivalent to the amount of tributary area treated by a wetland.
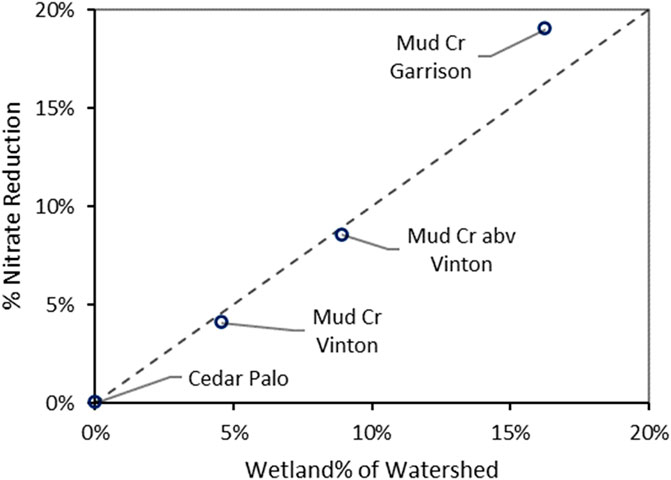
Figure 5. Percent nitrate reduction versus percentage of a site’s drainage area treated by the wetland.
Nitrate yields spanning the ten-month analysis period were marked by a sharp decrease directly downstream of the wetland, followed by a steady rise at each successive monitoring location (Table 2). Downstream of the wetland, values were 4.20 (Mud Cr Wet Out), but they increased to 15.5 kg/ha at the Cedar Palo site. The yields within Mud Cr (4.20, 8.30, 11.4, and 12.8) were notably lower than those upstream of the wetland (16.1 kg/ha), but by the time the water had reached Cedar Palo, the yields were quite similar (15.5 kg/ha).
3.4 Monthly variations
Table 3 summarizes the nitrate load removed by the wetland and the subsequent % Reduction values for each of the 10 months included in our two-year analysis period. It also contains the monthly water yield at Mud Cr Vinton, which demonstrates Mud Creek’s overall streamflow in that month. The 10 months encompass a range of hydrologic conditions ranging from 2.02 mm (September 2023) to 32.5 mm (May 2022). The nitrate load removed by the wetland was also highly variable, spanning 2 kg (September 2023) to 1,520 kg (May 2022).
The % Reduction values measured in the study experienced high variability by month. In the drier months (August–September 2023), the wetland removed 100% of the inflow nitrate. The % Reduction values were lower in wetter months, with a minimum % Reduction of 57% in May 2023. However, the % Reduction at the wetland was not well correlated with the % Reduction at downstream sites. Values ranged from 0.76%–32% at Mud Cr Garrison and 2.7%–14% at Mud Cr abv Vinton. The reduction for Mud Cr Vinton, which characterizes the entirety of the Mud Creek watershed, was lowest during the driest months (1.7% in August 2023 and 0.14% in September 2023). The maximum value was 5.5% in July 2022. In contrast, the reduction at Cedar Palo was never greater than 0.07%.
4 Discussion
4.1 Nitrate reduction in the wetland
Study results indicate that the constructed wetland in the upper Mud Creek watershed removed 6,200 kg of nitrate over a ten-month period occurring in 2022 and 2023, corresponding to a 74% reduction of input nitrate load. This nitrate load reduction is consistent with many studies documenting nitrate removal rates between 30% and 80% (Allred et al., 2014; Crumpton et al., 2006; Drake et al., 2018; Groh et al., 2015; Lemke et al., 2022). These results further suggest that this wetland is typical of those installed in Midwestern landscapes dominated by row crops. Although our monitoring data was insufficient to discern the processes responsible for nitrate removal in the wetland, denitrification, and assimilation are considered the dominant mechanisms at similar sites (Collins and Gillies, 2014; Crumpton, 2008; Crumpton et al., 2020; Drake et al., 2018; Lee et al., 2009; Lin et al., 2008). Overall, our results align with numerous studies highlighting constructed wetlands as an effective conservation practice for nitrate load reduction (Christianson et al., 2014; Crumpton et al., 2020; Gleason et al., 2011; Whigham, 1999).
Short-term variations in nitrate reductions were evident (Table 3) in the monitoring data, particularly related to streamflow and seasonality. Nitrate is mobilized following wet weather periods and delivered to the wetland through groundwater or tile line discharge (Schilling and Helmers, 2008; Spalding et al., 2001). During spring or summer wet weather events in agricultural basins, nitrate concentrations are typically diluted during peak flows but rise as streamflow recedes (i.e., during the falling limb of a hydrograph) due to the increased contributions of groundwater and tile drainage, which lag behind the surface runoff component (Speir et al., 2021; Tanner et al., 1999). Removal rates are often largest in the aftermath of rainfall events in the spring and summer when concentrations are the highest (Crumpton et al., 2020). These streamflow patterns influence the wetland residence time, complicating the relationship between flow rates and nitrate removal. Annual and monthly nitrate removal in wetlands typically decreases as the hydraulic loading rate increases because water residence times decrease with more water flux into the wetland (Crumpton, 2008; Drake et al., 2018). Longer residence time for water in a wetland means more time for nitrate reductions to occur via denitrification (Collins and Gillies, 2014; C; Lee et al., 2009; Lin et al., 2008). On the other hand, higher inflow rates lead to faster water flow through the wetland and less time for interactions between nitrate and the soil and plant media (Drake et al., 2018; Kadlec, 2010). For the Mud Creek wetland, the driest months contained the largest nitrate removal rates because of long residence times. In the months with severe drought conditions, nitrate removal reached 100% efficiency since no water exited the wetland. However, while the dry conditions resulted in high % Reduction values, nitrate mass removal was minimal due to low nitrate inputs into the wetland.
Furthermore, the location of the wetland in the headwater region of the Mud Creek watershed made it more susceptible to conditions with zero inflow during the drought. While the upper reaches of Mud Creek dried out during the later summer of 2023, perennial flow persisted in the downstream portions of the main channel due to the increased groundwater (baseflow) contributions present in a larger drainage area. Flow was perennial at the Mud Cr Garrison site and all downriver locations. During the 2023 dry period, the wetland was hydraulically disconnected from Mud Creek’s main channel and did not provide measurable nitrate loss, but nitrate loads were still discharged into the Cedar River.
4.2 Downstream impacts of the wetland
While it is clear that the constructed wetland reduced nitrate loading at the scale of the wetland, our study’s main objective was contextualizing the nitrate removal within the scale of a watershed. Calculating wetland nitrate removal efficiency is standard practice for wetlands, but rarely is it possible to measure the impact of the wetland on downstream nitrate loads. Most attempts at quantifying these impacts have used a model-based approach—simulating nitrate concentrations and loads at successive downstream locations (Arheimer and Wittgren, 2002; Böhlke et al., 2009; Conan et al., 2003) and exploring the difference in nitrate levels before and after the wetland installation (Chavan and Dennett, 2008; Ham et al., 2010).
In our analysis, we used continuous monitoring of nitrate and streamflow at four downriver sites to quantify downstream loads using field measurements. Results showed the diminishing impact of the wetland at successive downstream monitoring locations and validated the general patterns described by nitrate-based watershed models (Arheimer and Wittgren, 2002; Böhlke et al., 2009; Conan et al., 2003). Empirical observations from our study demonstrated the spatial rate at which the wetland’s impact on downstream water quality becomes indiscernible.
The landscape of the Mud Creek basin and much of the U.S. Corn Belt is dominated by intensive row crop agriculture, and measuring the impact of a singular practice on downstream water quality is often overwhelmed by untreated water from this landscape. Nitrate concentrations in Iowa rivers are significantly related to land cover; one can estimate mean annual nitrate concentrations in Iowa rivers by simply multiplying the row crop percentage in a watershed by 0.1 (Schilling and Libra, 2000). In this study, we showed that there is also a relationship between % Reduction and percentage of a watershed treated by a wetland (approximately 1:1). The portion of a watershed treated by a wetland was linearly related to the percentage of that watershed nitrate load removed by the wetland (Figure 5). This relationship presents a convenient rule of thumb for estimating the number of practices needed to achieve reduction goals. For example, if stakeholders in Mud Creek sought to reduce nitrate export by 20%, this could likely be achieved by installing four additional wetlands of similar volume and surface area. [6,200 kg * 5 = 31,000 kg, which is ∼20% of 145,000 kg (the nitrate load at Mud Cr Vinton)] While many nuances surround wetland design and effectiveness, this calculation provides a first-order approximation of the investment level in conservation practices needed to achieve a nutrient reduction goal.
The nitrate concentrations in 2020 (pre-wetland) and 2022 (post-wetland) also revealed the wetland’s impact on local nutrient levels (Figure 6). Although the difference between these profiles highlights the impacts of the wetland on local water quality conditions immediately below the outlet, this analysis is subject to a few limitations. First, this comparison of concentrations does not incorporate the overall nitrate mass removed by the wetland. It summarizes local nitrate conditions rather than explicitly denoting the wetland’s impact on nitrate removal. Second, nitrate is also inherently variable and tied to recent hydrologic behavior, so these longitudinal profiles may vary considerably when examining short timeframes.
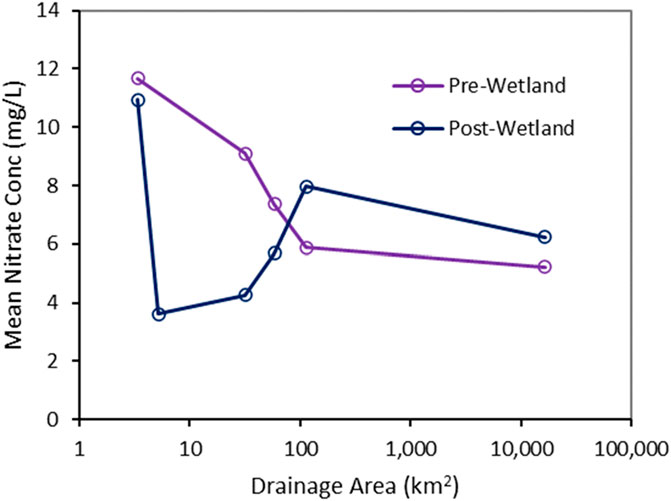
Figure 6. Mean nitrate concentrations (May - September) in Mud Creek pre-wetland (2020) and post-wetland (2022).
Figure 6 demonstrates how the wetland’s influence predictably diminishes at downstream locations with greater tributary area. Nitrate concentrations increased in Mud Creek as more water from untreated landscapes entered the channel. By the time surface water in Mud Creek reached the confluence with the Cedar River, the wetland’s influence was negligible. Hence, it is important to measure the impact of a conservation practice within a watershed at the appropriate scale. In a study of large-scale prairie restoration on watershed nitrate concentrations, Schilling and Spooner (2006) reported that the influence of headwater row crop areas on stream nitrate values overwhelmed the low-nitrate signal contributed from the restored prairie areas located in the core of the watershed, making it difficult to detect trends in the watershed over time. In both cases, water and nitrate derived from non-impacted areas confound the ability to measure conservation impacts at a watershed scale.
Although mean concentrations were higher at Mud Cr Vinton in the post-wetland condition than before its installation, this should not be viewed as a failure of the wetland since nitrate concentrations and loads can vary tremendously from year to year (Jones et al., 2018c), mainly due to hydrologic fluctuations (Ayers et al., 2021; Raymond et al., 2012). Sites often require several years (10+) of data to discern statistically significant differences in nitrate levels (Burt et al., 2010; Sprague et al., 2011), and many other factors may be at play, such as shifting conservation practices or land use, that could affect nitrate transport (Poor and McDonnell, 2007; Schilling and Libra, 2000).
4.3 Implications for nutrient reduction goals
The wetland’s trivial impact on nitrate loads in the Cedar River also provides valuable context for meeting large-scale nutrient reduction goals. The State of Iowa explicitly aims to reduce its riverine nitrate export by 45%, which is guided by the Iowa Nutrient Reduction Strategy (Iowa State University, 2012). Many states in the U.S. Corn Belt draining to the Mississippi River and Gulf of Mexico have similar nutrient reduction goals (R. Christianson et al., 2018).
The Mud Creek wetland example sheds light on the degree of conservation practice implementation needed to achieve specific goals. This singular practice removed 0.02% of the nitrate at the Cedar Palo site. By extrapolating this removal rate, we estimate that over 1,850 constructed wetlands (with sizes similar to this study’s wetland) would be needed to meet the 45% reduction goal in the Cedar River alone. It should be noted that this scaling exercise assumes a similar size, placement, and efficiency of the constructed practices. The footprint of these wetlands would comprise about 0.6% of the watershed’s total area—a value aligned with what other studies have noted on wetland land use (Cheng et al., 2020; Crumpton, 2008). Installing 1,850 wetlands across five HUC08 basins (i.e., the Cedar watershed) is a level of investment far greater than any current water quality improvement efforts in the U.S. Corn Belt. Economic assessments have found the average design and construction cost for similar wetlands to be approximately $25,000/ha (Tyndall and Bowman, 2016); extrapolating this cost to 1,850 wetlands of similar size yields a dollar amount of $235 million. This value should be considered a minimum bound on wetland installation at this scale, as many local factors can further drive expenses. For instance, the cost of the Mud Creek wetland totaled $600,000—much greater than the simple estimate of $127,000 [5.1 ha * $25,000/ha]. Even the most successful initiatives have operated at a scale many orders of magnitude smaller (R. Christianson et al., 2018; Gleason et al., 2011; Weber et al., 2018). Given the political will in an agricultural state like Iowa, installing enough wetlands to meet the 45% reduction goal appears impractical and infeasible.
Consequently, it is essential to consider the issue of scale when evaluating the impact of any specific conservation practice. A constructed wetland can provide substantial water quality benefits in the waters directly downstream of their outlets, yet its influence wanes at the HUC12 scale once water from untreated landscapes dominates a stream. At the HUC08 or state scale, wetlands alone are unlikely to curb eutrophication given the exorbitant number and cost needed. Nitrate pollution in the U.S. Corn Belt is so widespread that achieving the desired reductions will require a multi-faceted approach extending beyond implementing isolated conservation practices. Indeed, nearly all large-scale nutrient reduction efforts include components on limiting nitrate inputs and improving on-field retention on the landscape in addition to installing edge-of-field practices (Anderson et al., 2016; Hart et al., 1997; Scavia et al., 2017).
The wetland installed in Mud Creek was, by many metrics, a success; partnerships between funding agencies and landowners resulted in a practice that substantially reduced local nitrate concentrations and provided many ancillary benefits, including improved ecological health, recreation opportunities, and aesthetics. However, even successful practices have a downstream influence that quickly diminishes, and the impact of a single 5.1 ha wetland on nitrate load reduction was minor (<5%) at the HUC12 scale.
5 Conclusion
In this study, we evaluated the downstream impacts of a newly constructed wetland on nitrate levels during a ten-month period in 2022 and 2023 in the highly agricultural Mud Creek watershed located in east-central Iowa. High-resolution nitrate sensors deployed in four downstream monitoring locations and in the larger receiving river system documented the impacts of the wetland on watershed scale nitrate loads. Consistent with other wetland monitoring studies, the Mud Creek wetland was found to be an effective conservation practice, removing 74% of the nitrate that entered it. However, the impacts of the wetland on downstream water quality decreased considerably at downstream monitoring locations. At the outfall of Mud Creek into the Cedar River, the wetland was found to have reduced nitrate loads by 4.1%. Within the larger Cedar River basin, the single Mud Creek wetland reduced nitrate loads by 0.02%. Some variations in nitrate load reductions occurred primarily due to seasonal nitrate patterns and fluctuating streamflow levels. Study results suggest that the impact of any single conservation practice may be difficult to discern at the watershed scale and that a substantial number of practices will be needed to address nutrient reduction goals in larger watersheds.
Data availability statement
The data presented in this study are deposited in the IFIS (https://ifis.iowafloodcenter.org/ifis/), IWQIS (https://iwqis.iowawis.org), and USGS (https://waterdata.usgs.gov/nwis) repositories. Accession numbers can be found in the article/supplementary material. Further inquiries can be directed to the corresponding author.
Author contributions
EA: Conceptualization, Data curation, Formal Analysis, Investigation, Methodology, Writing–original draft, Writing–review and editing. KS: Conceptualization, Formal Analysis, Methodology, Writing–original draft. CJ: Conceptualization, Funding acquisition, Supervision, Writing–review and editing. B-CS: Investigation, Methodology, Writing–review and editing.
Funding
The author(s) declare that financial support was received for the research, authorship, and/or publication of this article. Funding for the project was provided by the Iowa Watershed Approach and the Environmental Protection Agency, Cooperative Agreement 00D86819.
Acknowledgments
The authors wish to thank Erin Elshoff, Cullen McDermott, Matthew Streeter, Thomas Stoeffler, and Jordan Barnett for their assistance in collecting field samples and maintaining in-situ equipment. We also thank the landowner for his willingness to construct this study’s wetland on his property and his cooperation during the monitoring process.
Conflict of interest
The authors declare that the research was conducted in the absence of any commercial or financial relationships that could be construed as a potential conflict of interest.
Publisher’s note
All claims expressed in this article are solely those of the authors and do not necessarily represent those of their affiliated organizations, or those of the publisher, the editors and the reviewers. Any product that may be evaluated in this article, or claim that may be made by its manufacturer, is not guaranteed or endorsed by the publisher.
Supplementary material
The Supplementary Material for this article can be found online at: https://www.frontiersin.org/articles/10.3389/fenvs.2024.1416018/full#supplementary-material
SUPPLEMENTAY FIGURE S1 | Days on which nitrate measurements were collected at the Mud Creek monitoring sites.
SUPPLEMENTAY FIGURE S2 | Weekly Palmer Drought Severity Index (PDSI) at the wetland.
SUPPLEMENTAY FIGURE S3 | Annual water yield at three USGS gauges near Mud Creek. (Cedar Vinton: 05464315; Salt Cr: 05452000; Wolf Cr Dysart: 05464220).
SUPPLEMENTAY FIGURE S4 | Nitrate loads versus drainage area at the six monitoring sites (May - Sep; 2022 and 2023).
SUPPLEMENTAY FIGURE S5 | Nitrate yields versus drainage area at the six monitoring sites (May - Sep; 2022 and 2023).
SUPPLEMENTAY FIGURE S6 | Comparison of nitrate loads entering the wetland through the peripheral tile lines (y-axis) and main channel (x-axis). The simple linear regression suggested a consistent relationship where tile loads were 20% of those in the main channel. Therefore, daily nitrate loads entering the wetland estimated as 1.2 times those measured at the main channel.
References
Allred, B. J., Gamble, D. L., Levison, P. W., Scarborough, R. L., Brown, L. C., and Fausey, N. R. (2014). Field test results for nitrogen removal by the constructed wetland component of an agricultural water recycling system. Appl. Eng. Agric. 30 (2), 163–177. doi:10.13031/aea.30.10061
Anderson, W. P., Wall, D., and Olson, J. L. (2016). “Minnesota nutrient reduction strategy,” in Paper presented at the 2016 10th International Drainage Symposium Conference, Minneapolis, Minnesota, 6–9 September 2016, 1–9. doi:10.13031/ids.20162492032
Arheimer, B., and Wittgren, H. B. (2002). Modelling nitrogen removal in potential wetlands at the catchment scale. Ecol. Eng. 19 (1), 63–80. doi:10.1016/S0925-8574(02)00034-4
Aulenbach, B. T., Buxton, H. T., Battaglin, W. A., and Coupe, R. H. (2007). Streamflow and nutrient fluxes of the Mississippi-Atchafalaya River Basin and subbasins for the period of record through 2005 (2331-1258). Available at: https://pubs.usgs.gov/publication/ofr20071080 (March 20, 2024).
Ayers, J. R., Villarini, G., Schilling, K. E., and Jones, C. S. (2021). Development of statistical models for estimating daily nitrate load in Iowa. Sci. Total Environ. 782, 146643. doi:10.1016/j.scitotenv.2021.146643
Böhlke, J. K., Antweiler, R. C., Harvey, J. W., Laursen, A. E., Smith, L. K., Smith, R. L., et al. (2009). Multi-scale measurements and modeling of denitrification in streams with varying flow and nitrate concentration in the upper Mississippi River basin, USA. Biogeochemistry 93, 117–141. doi:10.1007/s10533-008-9282-8
Brendel, C., and Soupir, M. L. (2017). Relating watershed characteristics to elevated stream Escherichia coli levels in agriculturally dominated landscapes: an Iowa case study. Water 9 (3), 154. doi:10.3390/w9030154
Burkart, M. R., and James, D. E. (1999). Agricultural-nitrogen contributions to hypoxia in the Gulf of Mexico. J. Environ. Qual. 28 (3), 850–859. doi:10.2134/jeq1999.00472425002800030016x
Burt, T., Howden, N., Worrall, F., and Whelan, M. (2010). Long-term monitoring of river water nitrate: how much data do we need? J. Environ. Monit. 12 (1), 71–79. doi:10.1039/b913003a
Butt, A. J., and Brown, B. L. (2000). The cost of nutrient reduction: a case study of Chesapeake Bay. Coast. Manag. 28 (2), 175–185. doi:10.1080/089207500263585
Cao, P., Lu, C., Crumpton, W., Helmers, M., Green, D., and Stenback, G. (2023). Improving model capability in simulating spatiotemporal variations and flow contributions of nitrate export in tile-drained catchments. Water Res. 244, 120489. doi:10.1016/j.watres.2023.120489
Chambers, P. A., Vis, C., Brua, R. B., Guy, M., Culp, J. M., and Benoy, G. A. (2008). Eutrophication of agricultural streams: defining nutrient concentrations to protect ecological condition. Water Sci. Technol. 58 (11), 2203–2210. doi:10.2166/wst.2008.815
Chavan, P. V., and Dennett, K. E. (2008). Wetland simulation model for nitrogen, phosphorus, and sediments retention in constructed wetlands. Water, air, soil Pollut. 187, 109–118. doi:10.1007/s11270-007-9501-2
Cheng, F., Van Meter, K., Byrnes, D., and Basu, N. (2020). Maximizing US nitrate removal through wetland protection and restoration. Nature 588 (7839), 625–630. doi:10.1038/s41586-020-03042-5
Christianson, L., Knoot, T., Larsen, D., Tyndall, J., and Helmers, M. (2014). Adoption potential of nitrate mitigation practices: an ecosystem services approach. Int. J. Agric. Sustain. 12 (4), 407–424. doi:10.1080/14735903.2013.835604
Christianson, L., Tyndall, J., and Helmers, M. (2013). Financial comparison of seven nitrate reduction strategies for Midwestern agricultural drainage. Water Resour. Econ. 2, 30–56. doi:10.1016/j.wre.2013.09.001
Christianson, R., Christianson, L., Wong, C., Helmers, M., McIsaac, G., Mulla, D., et al. (2018). Beyond the nutrient strategies: common ground to accelerate agricultural water quality improvement in the upper Midwest. J. Environ. Manag. 206, 1072–1080. doi:10.1016/j.jenvman.2017.11.051
Collins, A. R., and Gillies, N. (2014). Constructed wetland treatment of nitrates: removal effectiveness and cost efficiency. JAWRA J. Am. Water Resour. Assoc. 50 (4), 898–908. doi:10.1111/jawr.12145
Conan, C., Bouraoui, F., Turpin, N., de Marsily, G., and Bidoglio, G. (2003). Modeling flow and nitrate fate at catchment scale in Brittany (France). J. Environ. Qual. 32 (6), 2026–2032. doi:10.2134/jeq2003.2026
Conley, D. J. (1999). Biogeochemical nutrient cycles and nutrient management strategies. Man River Syst. Funct. River Syst. at Basin Scale, 87–96. doi:10.1007/978-94-017-2163-9_10
Crumpton, W. G. (2001). Using wetlands for water quality improvement in agricultural watersheds; the importance of a watershed scale approach. Water Sci. Technol. 44 (11-12), 559–564. doi:10.2166/wst.2001.0880
Crumpton, W. G. (2008). 3. Potential of Restored and Constructed Wetlands to Reduce Nutrient Export from Agricultural Watersheds in the Corn Belt. ASABE. doi:10.13031/2013.24242
Crumpton, W. G., Stenback, G., Helmers, M., and Miller, B. (2006). Potential benefits of wetland filters for tile drainage systems: impact on nitrate loads to Mississippi River subbasins. Available at: https://www.fsa.usda.gov/Assets/USDA-FSA-Public/usdafiles/EPAS/PDF/fsa_final_report_crumpton_rhd.pdf (March 20, 2024).
Crumpton, W. G., Stenback, G. A., Fisher, S. W., Stenback, J. Z., and Green, D. I. S. (2020). Water quality performance of wetlands receiving nonpoint-source nitrogen loads: nitrate and total nitrogen removal efficiency and controlling factors. J. Environ. Qual. 49 (3), 735–744. doi:10.1002/jeq2.20061
David, M., McIsaac, G., Czapar, G., Schnitkey, G., and Mitchell, C. (2013). Science assessment to support an Illinois nutrient loss reduction strategy. Urbana-Champaign, Coll. Agric. Consumer, Environ. Sci., 33–45.
Dewitte, K., Fierens, C., Stockl, D., and Thienpont, L. M. (2002). Application of the Bland–Altman plot for interpretation of method-comparison studies: a critical investigation of its practice. Clin. Chem. 48 (5), 799–801. doi:10.1093/clinchem/48.5.799
Dodds, W. K., and Welch, E. B. (2000). Establishing nutrient criteria in streams. J. North Am. Benthol. Soc. 19 (1), 186–196. doi:10.2307/1468291
Drake, C. W., Jones, C. S., Schilling, K. E., Amado, A. A., and Weber, L. J. (2018). Estimating nitrate-nitrogen retention in a large constructed wetland using high-frequency, continuous monitoring and hydrologic modeling. Ecol. Eng. 117, 69–83. doi:10.1016/j.ecoleng.2018.03.014
Fewtrell, L. (2004). Drinking-water nitrate, methemoglobinemia, and global burden of disease: a discussion. Environ. health Perspect. 112 (14), 1371–1374. doi:10.1289/ehp.7216
Gleason, R. A., Euliss Jr, N., Tangen, B., Laubhan, M., and Browne, B. (2011). USDA conservation program and practice effects on wetland ecosystem services in the Prairie Pothole Region. Ecol. Appl. 21 (sp1), S65–S81. doi:10.1890/09-0216.1
Goolsby, D. A., Battaglin, W. A., Aulenbach, B. T., and Hooper, R. P. (2001). Nitrogen input to the Gulf of Mexico. J. Environ. Qual. 30 (2), 329–336. doi:10.2134/jeq2001.302329x
Groh, T. A., Gentry, L. E., and David, M. B. (2015). Nitrogen removal and greenhouse gas emissions from constructed wetlands receiving tile drainage water. J. Environ. Qual. 44 (3), 1001–1010. doi:10.2134/jeq2014.10.0415
Haas, M. B., Guse, B., and Fohrer, N. (2017). Assessing the impacts of Best Management Practices on nitrate pollution in an agricultural dominated lowland catchment considering environmental protection versus economic development. J. Environ. Manag. 196, 347–364. doi:10.1016/j.jenvman.2017.02.060
Hallegraeff, G. (2003). Harmful algal blooms: a global overview. Man. harmful Mar. microalgae 33, 1–22.
Ham, J., Yoon, C. G., Kim, H.-J., and Kim, H.-C. (2010). Modeling the effects of constructed wetland on nonpoint source pollution control and reservoir water quality improvement. J. Environ. Sci. 22 (6), 834–839. doi:10.1016/s1001-0742(09)60185-6
Hart, J., Marx, E., Christensen, N., and Moore, J. (1997). Nutrient management strategies. J. Dairy Sci. 80 (10), 2659–2666. doi:10.3168/jds.s0022-0302(97)76225-8
Hunt, P. G., Stone, K. C., Humenik, F. J., Matheny, T. A., and Johnson, M. H. (1999). In-stream wetland mitigation of nitrogen contamination in a USA coastal plain stream. J. Environ. Qual. 28 (1), 249–256. doi:10.2134/jeq1999.00472425002800010030x
Hutchinson, K. J., and Christiansen, D. E. (2013). Use of the soil and water assessment tool (SWAT) for simulating hydrology and water quality in the Cedar River basin, Iowa 2000–10. Sci. Investig. Rep. 2013–5002, 36.
Ikenberry, C. D., Soupir, M. L., Schilling, K. E., Jones, C. S., and Seeman, A. (2014). Nitrate-nitrogen export: magnitude and patterns from drainage districts to downstream river basins. J. Environ. Qual. 43 (6), 2024–2033. doi:10.2134/jeq2014.05.0242
Iowa DNR NRGIS. (2009). High Resolution Land Cover Web Service. Iowa Geospatial Data Clearinghouse. Available at: https://geodata.iowa.gov/maps/05c7740a09304883bdae3b3945966c1a/about.
Iowa State University (2012). Iowa nutrient reduction strategy. Available at: https://www.nutrientstrategy.iastate.edu/ (March 20, 2024).
Jha, M. K., Wolter, C. F., Schilling, K. E., and Gassman, P. W. (2010). Assessment of total maximum daily load implementation strategies for nitrate impairment of the Raccoon River, Iowa. J. Environ. Qual. 39 (4), 1317–1327. doi:10.2134/jeq2009.0392
Jones, C. S., Davis, C. A., Drake, C. W., Schilling, K. E., Debionne, S. H., Gilles, D. W., et al. (2018a). Iowa statewide stream nitrate load calculated using in situ sensor network. JAWRA J. Am. Water Resour. Assoc. 54 (2), 471–486. doi:10.1111/1752-1688.12618
Jones, C. S., Kim, S.-W., and Schilling, K. (2017). Use of continuous monitoring to assess stream nitrate flux and transformation patterns. Environ. Monit. Assess. 189, 35–12. doi:10.1007/s10661-016-5749-6
Jones, C. S., Li, T., Sukalski, A., Thompson, D. A., and Cwiertny, D. M. (2020). Use of real-time sensors for compliance monitoring of nitrate in finished drinking water. Water Sci. Technol. 82 (12), 2725–2736. doi:10.2166/wst.2020.365
Jones, C. S., Nielsen, J. K., Schilling, K. E., and Weber, L. J. (2018b). Iowa stream nitrate and the Gulf of Mexico. PloS one 13 (4), e0195930. doi:10.1371/journal.pone.0195930
Jones, C. S., Schilling, K. E., Simpson, I. M., and Wolter, C. F. (2018c). Iowa stream nitrate, discharge and precipitation: 30-year perspective. Environ. Manag. 62 (4), 709–720. doi:10.1007/s00267-018-1074-x
Kadlec, R. H. (2010). Nitrate dynamics in event-driven wetlands. Ecol. Eng. 36 (4), 503–516. doi:10.1016/j.ecoleng.2009.11.020
Kalkhoff, S. J., Barnes, K. K., Becher, K., Savoca, M. E., Schnoebelen, D. J., Sadorf, E. M., et al. (2000). Water quality in the eastern Iowa basins, Iowa and Minnesota, 1996–98. Available at: https://pubs.usgs.gov/publication/cir1210.
Kovacic, D. A., David, M. B., Gentry, L. E., Starks, K. M., and Cooke, R. A. (2000). Effectiveness of constructed wetlands in reducing nitrogen and phosphorus export from agricultural tile drainage. J. Environ. Qual. 29 (4), 1262–1274. doi:10.2134/jeq2000.00472425002900040033x
Krajewski, W. F., Ceynar, D., Demir, I., Goska, R., Kruger, A., Langel, C., et al. (2017). Real-time flood forecasting and information system for the state of Iowa. Bull. Am. Meteorological Soc. 98 (3), 539–554. doi:10.1175/bams-d-15-00243.1
Kruger, A., Krajewski, W. F., Niemeier, J. J., Ceynar, D. L., and Goska, R. (2016). Bridge-mounted river stage sensors (BMRSS). IEEE Access 4, 8948–8966. doi:10.1109/access.2016.2631172
Lee, C., Fletcher, T. D., and Sun, G. (2009). Nitrogen removal in constructed wetland systems. Eng. life Sci. 9 (1), 11–22. doi:10.1002/elsc.200800049
Lee, K., Firoozfar, A. R., and Muste, M. (2017). Technical Note: monitoring of unsteady open channel flows using the continuous slope-area method. Hydrology Earth Syst. Sci. 21 (3), 1863–1874. doi:10.5194/hess-21-1863-2017
Lemke, A. M., Kirkham, K. G., Wallace, M. P., VanZomeren, C. M., Berkowitz, J. F., and Kovacic, D. A. (2022). Nitrogen and phosphorus removal using tile-treatment wetlands: a 12-year study from the midwestern United States. J. Environ. Qual. 51 (5), 797–810. doi:10.1002/jeq2.20316
Lin, Y.-F., Jing, S.-R., Lee, D.-Y., Chang, Y.-F., and Shih, K.-C. (2008). Nitrate removal from groundwater using constructed wetlands under various hydraulic loading rates. Bioresour. Technol. 99 (16), 7504–7513. doi:10.1016/j.biortech.2008.02.017
Lins, H. F., and Slack, J. R. (1999). Streamflow trends in the United States. Geophys. Res. Lett. 26 (2), 227–230. doi:10.1029/1998gl900291
Martin, J. F., and Reddy, K. (1997). Interaction and spatial distribution of wetland nitrogen processes. Ecol. Model. 105 (1), 1–21. doi:10.1016/s0304-3800(97)00122-1
Maxwell, E., Peterson, E. W., and O’Reilly, C. M. (2017). Enhanced nitrate reduction within a constructed wetland system: nitrate removal within groundwater flow. Wetlands 37, 413–422. doi:10.1007/s13157-017-0877-5
Messer, T. L., Trisha, L., Nelson, N., Ahiablame, L., Bean, E. Z., Boles, C., et al. (2021). Constructed wetlands for water quality improvement: a synthesis on nutrient reduction from agricultural effluents. Trans. ASABE 64 (2), 625–639. doi:10.13031/trans.13976
Mitsch, W. J., Day, J. W., Zhang, L., and Lane, R. R. (2005). Nitrate-nitrogen retention in wetlands in the Mississippi River basin. Ecol. Eng. 24 (4), 267–278. doi:10.1016/j.ecoleng.2005.02.005
NOAA. (2024). Precipitation Access Tools. National Centers for Environmental Information (NCEI). Available at: https://www.ncei.noaa.gov/products/precipitation.
Pellerin, B. A., Bergamaschi, B. A., Gilliom, R. J., Crawford, C. G., Saraceno, J., Frederick, C. P., et al. (2014). Mississippi River nitrate loads from high frequency sensor measurements and regression-based load estimation. Environ. Sci. and Technol. 48 (21), 12612–12619. doi:10.1021/es504029c
Peterson, E. W., and Benning, C. (2013). Factors influencing nitrate within a low-gradient agricultural stream. Environ. Earth Sci. 68, 1233–1245. doi:10.1007/s12665-012-1821-x
Poor, C. J., and McDonnell, J. J. (2007). The effects of land use on stream nitrate dynamics. J. Hydrology 332 (1-2), 54–68. doi:10.1016/j.jhydrol.2006.06.022
Quintero, F., Rojas, M., Muste, M., Krajewski, W. F., Perez, G., Johnson, S., et al. (2021). Development of synthetic rating curves: case study in Iowa. J. hydrologic Eng. 26 (1), 05020046. doi:10.1061/(asce)he.1943-5584.0002022
Rabalais, N. N., Turner, R. E., and Wiseman Jr, W. J. (2002). Gulf of Mexico hypoxia, aka “The dead zone”. Annu. Rev. Ecol. Syst. 33 (1), 235–263. doi:10.1146/annurev.ecolsys.33.010802.150513
Randall, G. W., and Mulla, D. J. (2001). Nitrate nitrogen in surface waters as influenced by climatic conditions and agricultural practices. J. Environ. Qual. 30 (2), 337–344. doi:10.2134/jeq2001.302337x
Raymond, P. A., David, M. B., and Saiers, J. E. (2012). The impact of fertilization and hydrology on nitrate fluxes from Mississippi watersheds. Curr. Opin. Environ. Sustain. 4 (2), 212–218. doi:10.1016/j.cosust.2012.04.001
Scavia, D., Kalcic, M., Muenich, R. L., Read, J., Aloysius, N., Bertani, I., et al. (2017). Multiple models guide strategies for agricultural nutrient reductions. Front. Ecol. Environ. 15 (3), 126–132. doi:10.1002/fee.1472
Schilling, K. E., and Helmers, M. (2008). Effects of subsurface drainage tiles on streamflow in Iowa agricultural watersheds: exploratory hydrograph analysis. Hydrological Process. An Int. J. 22 (23), 4497–4506. doi:10.1002/hyp.7052
Schilling, K. E., Jones, C., Wolter, C., Liang, X., Zhang, Y.-K., Seeman, A., et al. (2017). Variability of nitrate-nitrogen load estimation results will make quantifying load reduction strategies difficult in Iowa. J. Soil Water Conservation 72 (4), 317–325. doi:10.2489/jswc.72.4.317
Schilling, K. E., Jones, C. S., Seeman, A., Bader, E., and Filipiak, J. (2012). Nitrate-nitrogen patterns in engineered catchments in the upper Mississippi River basin. Ecol. Eng. 42, 1–9. doi:10.1016/j.ecoleng.2012.01.026
Schilling, K. E., and Libra, R. D. (2000). The relationship of nitrate concentrations in streams to row crop land use in Iowa. J. Environ. Qual. 29 (6), 1846–1851. doi:10.2134/jeq2000.00472425002900060016x
Schilling, K. E., and Spooner, J. (2006). Effects of watershed-scale land use change on stream nitrate concentrations. J. Environ. Qual. 35 (6), 2132–2145. doi:10.2134/jeq2006.0157
Schilling, K. E., and Wolter, C. F. (2009). Modeling nitrate-nitrogen load reduction strategies for the Des Moines River, Iowa using SWAT. Environ. Manag. 44 (4), 671–682. doi:10.1007/s00267-009-9364-y
Singh, N. K., Gourevitch, J. D., Wemple, B. C., Watson, K. B., Rizzo, D. M., Polasky, S., et al. (2019). Optimizing wetland restoration to improve water quality at a regional scale. Environ. Res. Lett. 14 (6), 064006. doi:10.1088/1748-9326/ab1827
Singh, S., Anil, A. G., Kumar, V., Kapoor, D., Subramanian, S., Singh, J., et al. (2022). Nitrates in the environment: a critical review of their distribution, sensing techniques, ecological effects and remediation. Chemosphere 287, 131996. doi:10.1016/j.chemosphere.2021.131996
Smith, V. H., and Schindler, D. W. (2009). Eutrophication science: where do we go from here? Trends Ecol. and Evol. 24 (4), 201–207. doi:10.1016/j.tree.2008.11.009
Spalding, R. F., Watts, D. G., Schepers, J. S., Burbach, M. E., Exner, M. E., Poreda, R. J., et al. (2001). Controlling nitrate leaching in irrigated agriculture. J. Environ. Qual. 30 (4), 1184–1194. doi:10.2134/jeq2001.3041184x
Speir, S. L., Tank, J. L., Bieroza, M., Mahl, U. H., and Royer, T. V. (2021). Storm size and hydrologic modification influence nitrate mobilization and transport in agricultural watersheds. Biogeochemistry 156 (3), 319–334. doi:10.1007/s10533-021-00847-y
Spieles, D. J., and Mitsch, W. J. (1999). The effects of season and hydrologic and chemical loading on nitrate retention in constructed wetlands: a comparison of low-and high-nutrient riverine systems. Ecol. Eng. 14 (1-2), 77–91. doi:10.1016/s0925-8574(99)00021-x
Sprague, L. A., Hirsch, R. M., and Aulenbach, B. T. (2011). Nitrate in the Mississippi River and its tributaries, 1980 to 2008: are we making progress? Environ. Sci. and Technol. 45 (17), 7209–7216. doi:10.1021/es201221s
Squillace, P. J., and Engberg, R. A. (1988). Surface-water quality of the Cedar River basin, Iowa-Minnesota, with emphasis on the occurrence and transport of herbicides, may 1984 through No vember 1985. Dep. Interior, US. Geol. Surv. 88.
Stein, O. R., and Hook, P. B. (2005). Temperature, plants, and oxygen: how does season affect constructed wetland performance? J. Environ. Sci. Health 40 (6-7), 1331–1342. doi:10.1081/ese-200055840
Syswerda, S. P., Basso, B., Hamilton, S. K., Tausig, J. B., and Robertson, G. P. (2012). Long-term nitrate loss along an agricultural intensity gradient in the Upper Midwest USA. Agric. Ecosyst. and Environ. 149, 10–19. doi:10.1016/j.agee.2011.12.007
Tanner, C. C., D'Eugenio, J., McBride, G. B., Sukias, J. P. S., and Thompson, K. (1999). Effect of water level fluctuation on nitrogen removal from constructed wetland mesocosms. Ecol. Eng. 12 (1), 67–92. doi:10.1016/S0925-8574(98)00055-X
Tyndall, J., and Bowman, T. (2016). Iowa nutrient reduction strategy best management practice cost overview series: constructed wetlands. Ames, IA, USA: Department of Ecology and Natural Resource Management, Iowa State University.
Vedachalam, S., Mandelia, A. J., and Heath, E. A. (2019). The impact of source water quality on the cost of nitrate treatment. AWWA Water Sci. 1 (1), e1011. doi:10.1002/aws2.1011
Vymazal, J. (2007). Removal of nutrients in various types of constructed wetlands. Sci. Total Environ. 380 (1), 48–65. doi:10.1016/j.scitotenv.2006.09.014
Ward, M. H., Jones, R. R., Brender, J. D., De Kok, T. M., Weyer, P. J., Nolan, B. T., et al. (2018). Drinking water nitrate and human health: an updated review. Int. J. Environ. Res. public health 15 (7), 1557. doi:10.3390/ijerph15071557
Weber, L. J., Muste, M., Bradley, A. A., Amado, A. A., Demir, I., Drake, C. W., et al. (2018). The Iowa Watersheds Project: Iowa's prototype for engaging communities and professionals in watershed hazard mitigation. Int. J. river basin Manag. 16 (3), 315–328. doi:10.1080/15715124.2017.1387127
Whigham, D. F. (1999). Ecological issues related to wetland preservation, restoration, creation and assessment. Sci. Total Environ. 240 (1-3), 31–40. doi:10.1016/s0048-9697(99)00321-6
Witzke, B. (1998). Bedrock geologic map of northeast Iowa. Trowbridge Hall Iowa City: Iowa DNR Geological Survey Bureau. Iowa Department of Natural Resources Iowa.
Zhang, Y.-K., and Schilling, K. E. (2005). Temporal variations and scaling of streamflow and baseflow and their nitrate-nitrogen concentrations and loads. Adv. water Resour. 28 (7), 701–710. doi:10.1016/j.advwatres.2004.12.014
Keywords: nitrate, wetland, scaling, remediation, monitoring, eutrophication
Citation: Anderson E, Schilling KE, Just CL and Seo BC (2024) Quantifying the impact of a constructed wetland on downstream nitrate concentrations and loads in the U.S. Midwest. Front. Environ. Sci. 12:1416018. doi: 10.3389/fenvs.2024.1416018
Received: 11 April 2024; Accepted: 07 October 2024;
Published: 21 October 2024.
Edited by:
Carl Mitchell, University of Toronto Scarborough, CanadaReviewed by:
Joseph Delesantro, The Pennsylvania State University (PSU), United StatesTim Duval, University of Toronto Mississauga, Canada
Copyright © 2024 Anderson, Schilling, Just and Seo. This is an open-access article distributed under the terms of the Creative Commons Attribution License (CC BY). The use, distribution or reproduction in other forums is permitted, provided the original author(s) and the copyright owner(s) are credited and that the original publication in this journal is cited, in accordance with accepted academic practice. No use, distribution or reproduction is permitted which does not comply with these terms.
*Correspondence: Keith E. Schilling, a2VpdGgtc2NoaWxsaW5nQHVpb3dhLmVkdQ==