- 1Laboratory of Environmental Health, Health Systems and Population Studies Division, International Centre for Diarrhoeal Disease Research, Bangladesh (icddr,b), Dhaka, Bangladesh
- 2Department of Microbiology, University of Manitoba, Winnipeg, MB, Canada
- 3Department of Pathobiological Sciences, School of Veterinary Medicine, Louisiana State University, Baton Rouge, LA, United States
- 4BCSIR Rajshahi Laboratories, Bangladesh Council of Scientific and Industrial Research, Dhaka, Bangladesh
- 5Infectious Diseases Division, International Centre for Diarrhoeal Disease Research, Bangladesh (icddr,b), Dhaka, Bangladesh
- 6Department of Chemical Engineering, Bangladesh University of Engineering and Technology (BUET), Dhaka, Bangladesh
- 7Institute of Water and Flood Management, Bangladesh University of Engineering and Technology (BUET), Dhaka, Bangladesh
- 8Centre for Water Supply, Sanitation and Waste Management, ITN-BUET, Dhaka, Bangladesh
- 9WASH Section, United Nations Children’s Fund, Dhaka, Bangladesh
Introduction: Efficient treatment of fecal sludge in densely populated settings is essential as it has a direct impact on public health and the environment. This study presents a comprehensive assessment of fecal sludge treatment technologies in Rohingya camps at Cox’s Bazar, Bangladesh, focusing on removal efficiencies and compliance with regulatory standards.
Methods: Seventeen treatment plants of five different technologies were evaluated based on removal efficiency and standard discharge guidelines for various physicochemical and microbiological parameters.
Results: Waste Stabilization Pond (WSP) was the top performer compared to four other different treatment technologies evaluated, achieving notable removal rates: 97.3% reduction in E. coli, 100% in helminth eggs, 98.3% for COD, 97.8% for BOD, 98.7% for TSS, 92.1% for TS, 82.8% for phosphate, and 93.3% for total nitrogen. Lime Stabilization Ponds showed lower removal rates, except for E. coli (98.9%), with reductions of 99.7% for helminth eggs, 81.6% for COD, 80.9% for BOD, 86.3% for TSS, 68.6% for TS, and 49.2% for phosphate. Upflow Filters demonstrated good removal efficiencies for E. coli (99.7%), TSS (95.9%), COD (91.7%), BOD (93.5%), and helminth eggs (93.7%). WSP consistently outperformed other technologies across all seasons. Despite these, none of the technologies fully met discharge standards.
Discussion: These findings highlight the need for a comprehensive approach, the combination of physicochemical and biological processes, to enhance efficacy. Promoting improved fecal sludge management technologies through awareness campaigns and technical support can mitigate environmental health risks in densely populated humanitarian settings.
1 Introduction
Humanitarian emergencies are occurring at increasing rates and affecting a growing number of people worldwide. One of such emergencies is the refugee crisis needing humanitarian assistance. Most of the refugees are hosted in low- and middle-income countries. Bangladesh has seen an influx of refugees on several occasions since 1991 (Wijnroks et al., 1993). During the years 1991–1992, more than 250,000 refugees migrated into the country from Myanmar. On 25th August 2017, approximately 671,500 refugees migrated to Bangladesh following continued violence in the Rakhine state of Myanmar. They were housed in refugee camps and operational costs were supported primarily through funding provided by the United Nations (UN), the Government of Bangladesh and various Non-governmental Organizations (NGOs) (Islam et al., 2019). Bangladesh has continued to support and provide shelter to refugees from ethnic, linguistic and religious abolishing despite being overpopulated and resource limited (Mahmood et al., 2017). Until 15th March 2018, more than 584,000 refugees have been settled into the Kutupalong expansion site, 187,000 in various other refugee camps and 113,000 within surrounding host communities. This considerable inflow of individuals within such a small area of the Cox’s Bazar district of Bangladesh combined with a lack of adequate resources to support such an influx has created a critical humanitarian emergency that overwhelmed the local communities and systems (World Health Organization, 2018).
The overall health and hygienic conditions remain very poor in these camps and are the leading cause of infectious disease outbreaks (Altare et al., 2019). The public health risks are further worsened by congested housing, inadequate access to safe drinking water and malnutrition. In humanitarian settings, the crisis of effective fecal sludge management remains a pressing concern, posing significant challenges to public health and environmental sustainability (Ngakane, 2021). According to WHO, insufficient treatment of wastewater and fecal sludge can cause disease and drive the development of antimicrobial resistance (Moe and Rheingans, 2006). As a result, public concern has shifted to inadequate waste management and pollution problems that result from vast quantities of waste generation. Chemical contaminants in fecal sludge, such as nitrogen, phosphorus, heavy metals, and various organic pollutants, pose additional risks to human health and the environment. Excessive nitrogen and phosphorus can lead to eutrophication in nearby water bodies, causing harmful algal blooms and dead zones that devastate aquatic ecosystems (Smith et al., 1999). Heavy metals like lead, mercury, and cadmium, often present in untreated sludge, can accumulate in the food chain, leading to toxic effects on humans and wildlife (Nicholson et al., 2003). Organic pollutants, including pharmaceuticals and personal care products, persist in the environment and disrupt endocrine systems in both humans and animals (Richardson and Ternes, 2014). Effective chemical management and treatment of fecal sludge (FS) are crucial to mitigating these risks and ensuring sustainable and safe living conditions in humanitarian settings.
Access to a functional sanitation service is considered a requirement for basic human health and wellbeing. The current strategy focuses on providing clean water, installing latrines and improving overall hygienic conditions. For this instance, more than 45 thousand latrines have been installed by different NGOs to fill up the sanitation needs at the camps (Wash Sector Cox’s Bazar, 2023). Around 40 different organizations have been working with WASH programs under different UN agencies like UNICEF, IOM and UNHCR (Inter Sector Coordination Group, 2018; Inter Sector Coordination Group and Cluster, 2018). The requirements of interventions for health and environmental protection ascertained focus on the containment transportation, treatment and disposal of FS (UNICEF, 2018).
The management and treatment of FS can be complex and often require fecal sludge treatment technologies for processing. The population of these camps is estimated to serve 931,447 beneficiaries as of June 2023, and each day an average of 1.1 L of fecal sludge per person is generated, leading to a total waste generation of 1,025,000 L or 1,025 m3 (Wash Sector Cox’s Bazar, 2023). During the first phase of emergency responses (2017–2020), various on-site sanitation system (OSS) fecal sludge treatment solutions were utilized to cope with the ever-increasing demand. As a result, 197 fecal sludge treatment plants (FSTPs) of different treatment technologies have been installed to be operated by 15 different NGOs (Inter Sector Coordination Group, 2022). Among them, waste stabilization ponds (WSP), upflow filter (UPF), anaerobic baffled reactor (ABR), decentralized wastewater treatment systems (DEWATS), lime stabilization pond (LSP), solid separation unit (SSU) are the most common (UNICEF, 2020). The existing facilities have a total treatment capacity of 879 m3/day, resulting in an inadequacy and overburden of these treatment facilities (Wash Sector Cox’s Bazar, 2023). The storage and generation of fecal sludge within pit latrines requires daily transport of fecal material to these treatment facilities either through housing within large tanks and transport via vehicles or through manual desludging and transport (OXFAM, 2022). Following treatment within the FSTPs, the liquids are discharged into the environment through nearby waterbodies (i.e., canal, river) or dried to generate solid wastes. The solid wastes are then either transported to incinerators to be processed for use in agriculture or stored within landfills with no further plan for use at the moment (OXFAM, 2022).
Although fecal sludge can be stored long term within landfills and even discharged within the environment, there are certain criteria and guidelines that need to be met for safe disposal. Biological and physicochemical parameters can be considered to characterize inlet and outlet to evaluate the efficiency of treatment technology (Strande et al., 2018). The parameters include solids concentration (total suspended solids (TSS), total solids (TS), dissolved solids and volatile solids), chemical oxygen demand (COD), biochemical oxygen demand (BOD), nutrients (nitrogen, phosphorous), ammonium, pathogens (bacteria, virus and parasites), and heavy metals (Klingel et al., 2002). The removal rate of these parameters is required for determining the efficiency of treatment technology. To improve the health quality of Rohingya residents, it is imperative to implement a rigorous and ongoing assessment of the efficacy of fecal sludge management (FSM) technologies in Rohingya camps. This will ensure the promotion of optimal sanitation and hygiene practices, directly contributing to the reduction of infectious diseases and enhancing overall community health. Besides performance evaluation, regular monitoring of FS treatment plants is an essential part of a sustainable FSM and environment, particularly in highly dense humanitarian settings like Rohingya camps. Therefore, this study aimed to characterize the FS (inlet and outlet) samples of the selected FS treatment technologies (WSP, UPF, ABR, DEWATS and LSP) and to evaluate the technology performance in terms of physicochemical and microbiological parameters at Rohingya camps in Cox’s Bazar, Bangladesh.
2 Materials and methods
2.1 Sampling site selection
The study was carried out at Rohingya camps in Cox’s Bazar, Bangladesh. Details locations of the sampling sites and points are depicted in Figure 1. The focus of this study was on the FSM technologies of the Rohingya camps, and the site selection was completed based on the location of five different FSM technologies in different camps under different NGOs and WASH partners of UNICEF. The selected technologies were UPF, ABR, LSP, WSP and DEWATS. Schematic diagrams of the technologies are depicted in (Supplementary Figures S1–S5). Samples were collected from 17 different plants (4 from each of ABR and UPF, three from each of LSP, DEWATS and WSP) of five different technologies throughout the year from both the inlet (raw sludge) and the outlet (treated sludge) of the plants. Treatment plants were symbolized from P1 through P17. Details information on the FS treatment technologies and plants along with basic structural characteristics based on the data collected by instant interviewing the respective responsible personnel of the plants are provided in Table 1. This study was carried out following a 12-round of sampling for 12 months to cover seasonal variation, so the total number of samples was 408 (17 × 2 × 12).
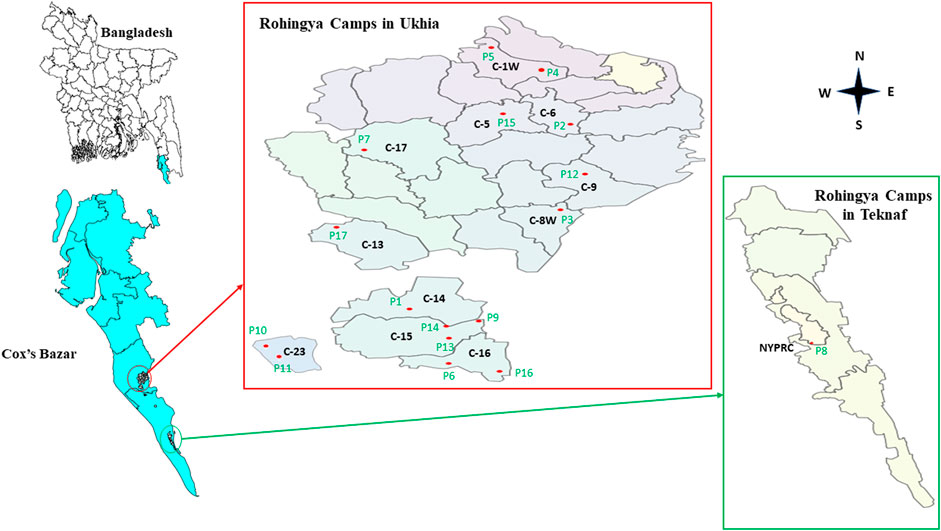
Figure 1. Geographical locations of the fecal sludge treatment plants where P1-P17 symbolised plant locations and C stands for Camp.
2.2 Sample collection and transportation
The samples were collected from December 2019 to April 2021. The composite sampling method was followed for collecting samples from both the inlet and outlet of individual treatment plant. Inlet samples were collected from the vacuum-truck during pouring into the inlet chamber at different time intervals-initial, midpoint and last stage. For the outlets, in the case of LSP and WSP, samples were collected from different corners and depth of the polishing ponds, and mixed in a large container for the pupose of composite sampling. For rest of the plants, outlet samples were collected from the discharge pipeline at different time intervals. The samples were taken into high-density polyethylene (HDPE) plastic bottles (Nalgene, Rochester, USA). For microbiological analysis, samples were collected in pre-sterilized bottles. In the case of total nitrogen (TN), and COD, samples were collected in pre-acidified acid-washed bottles. Samples for testing BOD, TS, TSS and phosphate (PO4) were collected in sterile HDPE bottles. The pH and conductivity samples were measured onsite via a portable pH/conductivity meter (Hanna Instruments, HI98130, USA). To ensure data accuracy and representativeness, duplicate samples were collected from both the inlet and outlet of each treatment plant at each sampling round. In addition, a field blank was also included in each sampling round to monitor potential contamination during sample collection. After collection, the samples were kept in a cool box with a sufficient amount of ice packs to maintain a temperature between 4°C and 10°C. Then, the samples were transported to the Laboratory of Environmental Health, icddr,b, Dhaka via air shipment. Analysis of physicochemical and microbiological parameters was done within 24 h of the collection of samples.
2.3 Physicochemical and microbiological analysis
2.3.1 Physicochemical parameters
The physicochemical parameters of both inlet and outlet samples were analyzed according to the standard methods for the examination of water and wastewater (Rice et al., 2017). The pH and temperature were determined at the field using a portable pH meter (Hanna Instrument, USA) following standard calibration and quality control procedures. TSS was determined using the gravimetric method where a glass fiber filter with a pore size of 1.50 µm (Merck- Millipore, Germany) was used (Rice et al., 2017). Phosphate was analyzed following the standard ascorbic acid method. COD was determined following the closed reflux digestion and spectrophotometric method using a spectrophotometer (Model: DR-6000, HACH, USA). BOD was determined by a respirometric BOD analysis procedure using a closed system maintaining 20°C ± 1°C and a direct reading analyzer (Model: BOD Trak II, HACH, USA). TS was determined by gravimetric method and quantified as the material remaining after evaporation and drying a suitable amount of sample in an oven at 103°C–105°C. Total nitrogen was determined by the summation of TKN from the semi-micro-Kjeldahl method (Rice et al., 2017) and nitrate-nitrogen (NO3-N) plus nitrite-nitrogen (NO2-N). NO3-N and NO2-N were determined by the ion chromatography technique (Pfaff, 1993). Commercially available standards were used to calibrate instruments and ensure the accuracy of measurements. Quality control standards, spiked samples, procedural blanks, and duplicate samples were also analyzed to ensure the accuracy and precision of the results.
2.3.2 Microbiological parameters
2.3.2.1 Enumeration of Escherichia coli
Samples were processed within 24 h after collection, maintaining standard procedure. For analysis, fecal sludge samples were subjected to serial dilutions using autoclaved normal saline (0.85% NaCl). A hundred milliliters of serial decimal dilutions (1/10, 1/100, 1/1000, 1/10000, 1/100000, 1/1000000) of the samples were filtered through a 0.22 μm membrane filter (Sartorius Stedim, Goettingen, Germany) in a Millipore filter unit (Millipore, Darmstadt, Germany). The membrane filters were then firmly placed on a modified thermotolerant E. coli (mTEC) agar (BD Difco, NJ, USA) plate. Subsequently, at 35°C ± 0.5°C, the culture plate was incubated for 2 h, followed by another episode of incubation at 44.5°C ± 0.2°C for 22 ± 2 h. After incubation, colonies with red to magenta color on mTEC media were considered as presumptive E. coli. It’s a quantitative experiment and the E. coli enumeration result was presented as CFU/100 mL of the sample (Hossain et al., 2021). Laboratory blank and E. coli ATCC 13706 were used as controls.
2.3.2.2 Enumeration of helminth eggs
The total helminth eggs were enumerated from both inlets and outlets of the FSTPs following the modified Bailenger method (Ayres et al., 1996). Briefly, a known volume of the sample was allowed for sedimentation and about 90% of the supernatant was removed carefully without disturbing the sediment. The sediment was transferred, subjected to centrifugation at 1000 g for 15 min and the supernatant was removed. Depending on the volume of the pellet, acetoacetic buffer and diethyl ether were added to the pellet, mixed well and centrifuged. The buffer maintains the pH which in turn increases the egg recovery by controlling the hydrophilic-lipophilic balance which makes the method most suitable and diethyl ether solubilizes the fatty matters present in the sample. Then, the pellets were mixed with concentrated ZnSO4 that helps to float the eggs followed by the enumeration of helminth eggs in the McMaster slide under a light microscope. Since it’s a quantitative test, the helminth eggs enumeration result was presented as the number of eggs per liter of samples.
2.4 Statistical analysis and technology efficiency evaluation
Data analysis was performed using Statistical Programming Language R (version 4.1.2) and Microsoft Excel 2019 (R Core Team, 2021; Microsoft Corporation, 2019). Due to the skewed distribution of the parameters, their summary statistics among different FS treatment technologies (inlet/outlet) were expressed in terms of the minimum, maximum, median, and interquartile range (IQR) (Schmid and Huber, 2014). To compare the efficiency of the technologies, the removal efficiency in percentage was calculated using the following formula (Otaka et al., 2019) presented in the bar diagram.
In addition to this, a bar diagram was also used to present the percentage of plants in a respective technology where the parameters were within the guidelines. Violin plots were used to illustrate the influent and effluent characteristics across different parameters, providing a clear depiction of data distribution and dispersion. Finally, the correlations between the parameters for different treatment technologies in the case of both inlet and outlet were calculated using the Spearman rank correlation method which is suitable for skewed data. Here, only the significant (at a 5% level of significance) correlation coefficients are presented in a correlation plot with a color gradient (deep blue-highly positive, deep red-highly negative). A scale (Table 2), applied to both positive and negative relationships was used to interpret the strength and direction of the relationship shown by the values inside the squares (Evans, 1996).
2.5 Comparison of outlet characteristics to discharge guidelines
The outlet characteristics were compared with the Bangladesh Department of Environment (DoE) standards for sewage discharge to the receiving water bodies and land. In the case of some parameters, Bangladesh does not have standard guidelines, therefore, the parameters were compared with EU, FAO and WHO outlet discharge guidelines. For pH, BOD, COD, TSS, E. coli and phosphate, the Environment Conservation Rules 2023 guideline was followed (The Environment Conservation Rules, 2023, 2023). For TN, the outlet values were compared with the European Union discharge guidelines (Lu et al., 2016). And lastly, for helminth and E. coli the WHO guidelines for the safe use of wastewater excreta and greywater were used (World Health Organization, 2006). Standard disccharge guideline of different parameters that were used to compare the current results from outlet (effluent) sample is provided in Table 3.
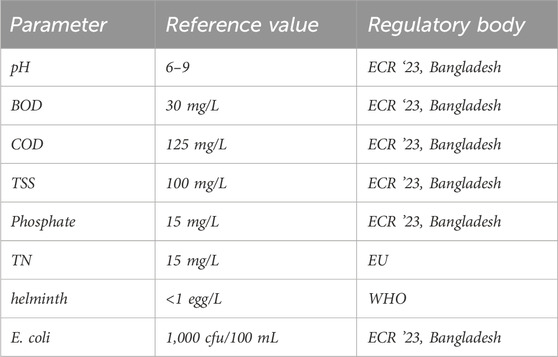
Table 3. Effluent discharge guidelines for different physicochemical and microbiological parameters.
3 Results
3.1 Microbiological and physicochemical characteristics of the inlet and outlet of each plant
The results of various physicochemical and microbiological parameters for the inlets and outlets of the selected ABR plants are presented in Supplementary Figure S6 (plants P1, P2, P7, and P16). In the ABR system, E. coli counts in the inlet consistently exceeded 20,000 cfu/100 mL across all plants. For the outlet, none of the plants met the discharge guidelines for E. coli throughout the year, except for P16 in round 5, P7 in rounds three and 5, P2 in round 11, and P1 in rounds 2 and 5. No significant trend was observed in the helminth counts for the inlets of all four ABR plants, which consistently showed high counts. However, outlet helminth counts were within the guidelines for P1 (round 3), P2 (rounds 2, 7, 10, and 11), P7 (rounds 2, 8, and 9), and P16 (rounds 3-7 and 9–12). Notably, P16 exhibited significantly lower helminth counts in all rounds except round 8. Regarding physicochemical parameters, the inlet concentrations of COD and BOD exceeded 400 mg/L and 145 mg/L, respectively, across all plants. Outlet values were within guidelines only for P7 in round 5, P1 for COD in round 5, and P2 for COD in round 5. Total nitrogen levels consistently exceeded 400 mg/L and failed to meet the guidelines. TSS levels were below the guideline for P1 (round 6), P2 (rounds 1 and 5), P7 (round 5), and P16 (rounds 5–7). Phosphate levels were within guideline limits for P1 (rounds 2–4), P2 (rounds 1–5), P7 (rounds three and 5), and P16 (round 5).
Data from DEWATS plants (P10, P11, P12, Supplementary Figure S10) showed no clear trends for inlet and outlet parameters. E. coli consistently surpassed 20,000 cfu/100 mL at the inlet. The outlet adhered to E. coli guidelines in a few plants and rounds (Plant P10: rounds 4 and 7; Plant P11: rounds 1–10; Plant P12: rounds 3 and 11). Helminth counts exceeded the guideline in P12 consistently, while P10 met the guideline in some rounds (rounds 2, 8–12) and P11 in others (rounds 1–5, 7–9, 11–12). Similar to ABR plants, inlet concentrations for COD and BOD exceeded 400 mg/L and 145 mg/L, respectively. outlet met COD and BOD guidelines in a limited number of plants and rounds (COD: P10: round 4; P11: rounds 3 and 5; BOD: P10: round 7; P12: round 9). Total nitrogen levels remained consistently high throughout all DEWATS plants. TSS levels complied with the guideline in specific plants and rounds (Plant P10: rounds 4, 7, 11; Plant P11: rounds 2–12; Plant P16: rounds 9–11). Phosphate levels adhered to regulations in some plants and rounds (Plant P10: rounds 7–12; Plant P11: rounds 3–12; Plant P12: rounds 3 and 9). Notably, pH levels generally remained within the acceptable range for both inlet and outlet samples.
The results for the LSP plants, depicted in Supplementary Figure S8 (Plants P4, P5, and P15) revealed varied patterns. For E. coli, counts were consistently high, except for the outlet in P4, which met the guideline with 0 cfu/100 mL in rounds 4 and 6. Helminth egg counts were within guidelines for P4 (round 10), P5 (round 12), and P15 (rounds 7 and 12). Outlet COD and BOD concentrations exceeded guidelines across all plants, except for the inlet BOD in P4 during round 5. Total nitrogen levels consistently remained above guidelines. TSS levels were within guidelines for P5 (rounds 8 and 11) and P15 (rounds 6 and 7). Phosphate levels were compliant in P4 (round 6), P5 (rounds 3–5), and P15 (rounds 1 and 5). pH levels were generally within guidelines, except for P4 (rounds 4 and 6).
UPF plants (plants P3, P6, P8, and P17, Supplementary Figure S7) also exhibited no clear trends in the data. Escherichia coli counts in the outlet met the guideline in several plants and rounds (Plant P3: rounds 1, 3, 9–12; Plant 6: round 4). Notably, helminth counts in the outlet were consistently below 150 eggs/L and adhered to the guidelines for specific plants and rounds (Plant P3: rounds 2, 6–8; Plant P6: round 6; Plant P17: rounds 5, 7, 9, 11). Outlet concentrations for COD and BOD met the guidelines in limited cases (COD: Plant P3: round 6; Plant P17: rounds 5 and 6; BOD: Plant P17: round 7). Total nitrogen levels remained persistently above the guideline across all UPF plants. TSS levels were within the limit for P3 (rounds 1 and 5–12), P6 (rounds 5 and 6), and P17 (rounds 5–12). Phosphate levels adhered to regulations in some plants and rounds (Plant P3: rounds 2, 4, 6; Plant P8: rounds 3–5; Plant P17: rounds 5–7; Plant P6: rounds 5 and 6). Generally, pH levels were compliant, except for some instances (Plant P1: round 2; Plant P7: round 3; Plant P6: round 12).
The results for the WSP plants, shown in Supplementary Figure S9 (plants P9, P13, and P14) exhibited similar inlet patterns. E. coli outlet counts met guidelines for P9 in rounds 4 and 7, and for P13 in rounds 4, 6, 7, 9, and 10. Helminth counts were within guidelines for P9 (rounds 2 and 8–12), P13 (rounds 1, 3, and 5–12), and P14 (rounds 7 and 12). Outlet COD and BOD concentrations were within guidelines for P9 in round 4, and for BOD only in round 5. P13 showed compliant levels for both COD and BOD in rounds 5–8. Total nitrogen levels were consistently above guidelines across all plants. TSS levels were below the guideline for P9 (rounds 4, 7, and 8) and P13 (rounds 1, 3, 5–8, and 10–12). Phosphate levels were compliant for P9 (rounds 7–9 and 11), P13 (rounds 3–8 and 10–12), and P14 (rounds 1, 5, and 12). pH levels were generally within guidelines, except for P13 in round 6.
3.2 Concentration distribution of different parameters
The overall data distribution, including minimum, maximum, and quartiles, is presented in Table 4. For inlet samples, the highest median E. coli count was observed in WSP (4.15 × 10^6 cfu/100 mL), while the lowest was in ABR (9 × 10^5 cfu/100 mL). The interquartile range (IQR) revealed the greatest spread in UPF (1.18 × 10^7 cfu/100 mL) and the least in LSP (4.8 × 10^5 cfu/100 mL). In outlet samples, LSP exhibited the highest median value, whereas DEWATS showed the lowest (3,000 cfu/100 mL). The IQR was highest in UPF (2.6 × 10^5 cfu/100 mL) and lowest in WSP (22,375 cfu/100 mL). Violin plots (Figure 2) indicated that inlet E. coli concentrations were concentrated around 450,000 cfu/100 mL across all technologies, while outlet concentrations were around 20,000 cfu/100 mL for ABR, LSP, and UPF, and 3,000 cfu/100 mL for DEWATS and WSP.
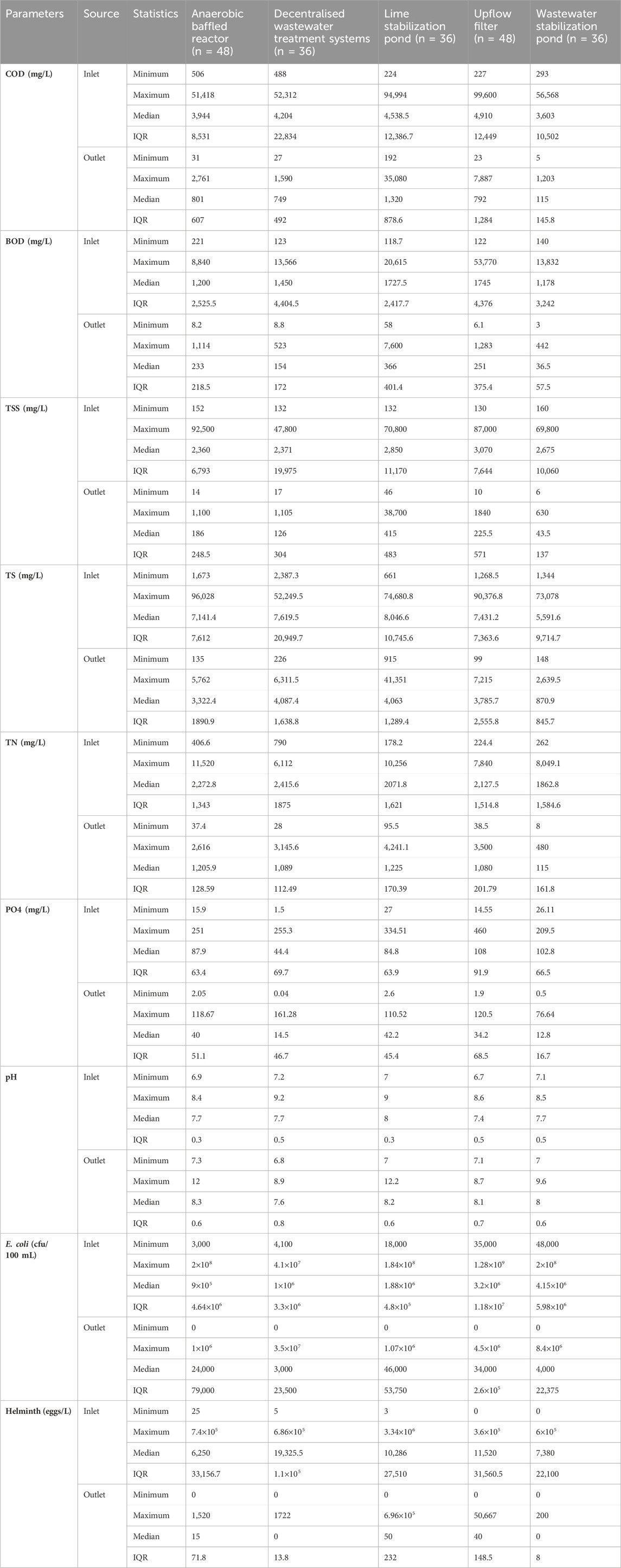
Table 4. Microbiological and physicochemical characterization of different fecal sludge treatment plants (inlet and outlet) determined over a 12-month period.
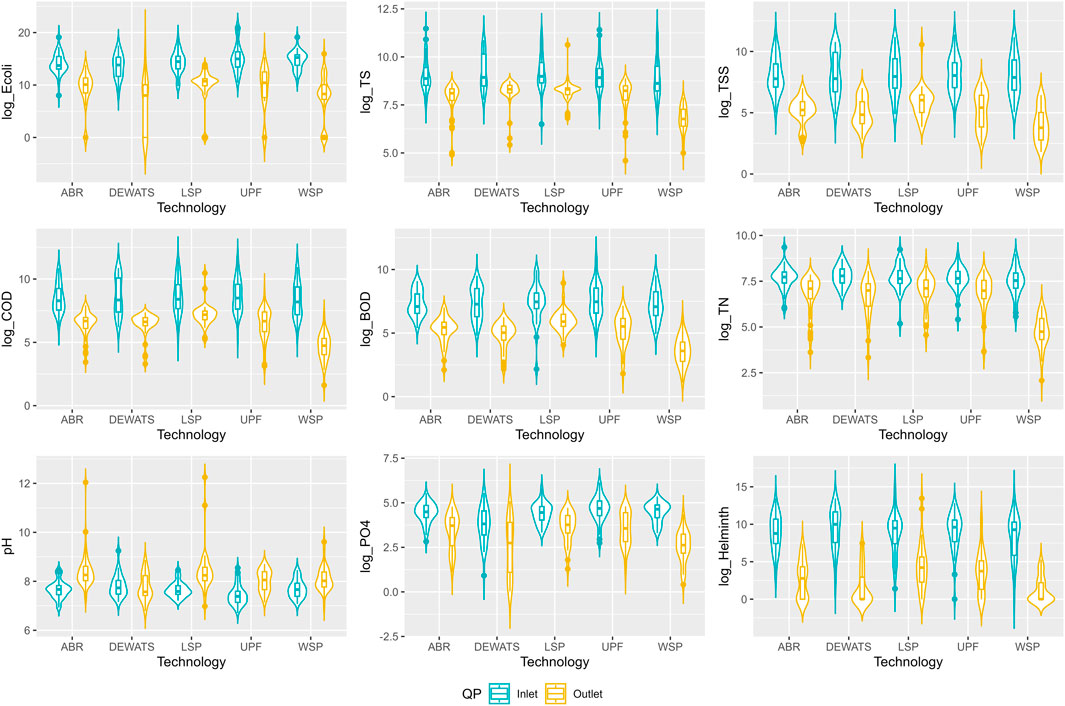
Figure 2. Violin plots illustrating comparative distributions of physicochemical and microbiological parameters across different plants of five treatment technologies.
For helminth eggs in inlet samples, DEWATS had the highest median value (19,325.5 eggs/L), while ABR had the lowest (6,250 eggs/L). The IQR was highest in DEWATS (1.1 × 10^5 eggs/L) and lowest in WSP (22,100 eggs/L). For outlet samples, WSP and DEWATS had the lowest median values (0 eggs/L), whereas LSP had the highest (50 eggs/L). Violin plots (Figure 2) showed inlet helminth egg concentrations were concentrated around 8,103 eggs/L, while outlet concentrations were below 20 eggs/L for ABR, DEWATS, and WSP, and around 55 eggs/L for LSP and UPF.
The highest median inlet COD concentration was found in UPF (4,910 mg/L) and the lowest in WSP (3,603 mg/L). The IQR was greatest in DEWATS (22,834 mg/L) and least in ABR (8,531 mg/L). In outlet samples, LSP had the highest median COD concentration (1,320 mg/L) and WSP the lowest (115 mg/L). Violin plots (Figure 2) showed inlet COD concentrations were concentrated around 2,981 mg/L, while outlet concentrations were below 1,097 mg/L for ABR, DEWATS, LSP, and UPF, and 148 mg/L for WSP.
The minimum and maximum median inlet BOD concentrations were observed in WSP (1,178 mg/L) and UPF (1745 mg/L), respectively. For outlet samples, WSP had the lowest median BOD concentration (36.5 mg/L), nearly within the Bangladesh standard, while LSP had the highest (366 mg/L). The IQR was highest in UPF and lowest in WSP. Violin plots (Figure 2) indicated inlet BOD concentrations were concentrated around 1808 mg/L, while outlet concentrations were above 244.7 mg/L for ABR, DEWATS, LSP, and UPF, and 33.1 mg/L for WSP.
For inlet TS concentrations, LSP exhibited the highest median (8,046.6 mg/L) and WSP the lowest (5,591.6 mg/L). The IQR was highest in DEWATS (20,949.7 mg/L) and lowest in UPF (7,363.6 mg/L). In outlet samples, DEWATS had the highest median TS concentration (4,087.4 mg/L) and WSP the lowest (870.9 mg/L). Violin plots (Figure 2) showed inlet TS concentrations were concentrated around 4,914.7 mg/L, while outlet concentrations were around 2,981 mg/L for ABR, DEWATS, LSP, and UPF, and 1,097 mg/L for WSP.
For inlet TSS samples, UPF had the highest median value (2,850 mg/L), and ABR the lowest (2,360 mg/L). The IQR was highest in DEWATS (19,975 mg/L) and lowest in ABR (6,793 mg/L). In outlet samples, LSP had the highest median value (415 mg/L) and WSP the lowest (43.5 mg/L). The IQR was highest in UPF (571 mg/L) and lowest in WSP (137 mg/L). Violin plots (Figure 2) indicated inlet TSS concentrations were concentrated around 2,981 mg/L, while outlet concentrations were around 148 mg/L for ABR, DEWATS, and UPF, and 1,097 mg/L for LSP, with less than 54.6 mg/L for WSP.
The highest median inlet TN concentration was observed in DEWATS (2,415.6 mg/L) and the lowest in WSP (1862.8 mg/L). The IQR was highest in DEWATS (1875 mg/L) and lowest in ABR (1,343 mg/L). For outlet samples, WSP had the lowest median TN concentration (115 mg/L) and LSP the highest (1,225 mg/L). The IQR was lowest in DEWATS (112.49 mg/L) and highest in UPF (201.79 mg/L). Violin plots (Figure 2) showed inlet TN concentrations were concentrated around 2,981 mg/L, while outlet concentrations were around 1808 mg/L for ABR, DEWATS, LSP, and UPF, and 121.5 mg/L for WSP.
For inlet PO4 concentrations, DEWATS had the lowest median value (44.4 mg/L) and spread (63.4 mg/L), whereas UPF had the highest median (108 mg/L) and spread (91.9 mg/L). For outlet samples, WSP had the lowest median concentration (12.8 mg/L) and spread (16.7 mg/L), while LSP had the highest median (42.2 mg/L) and UPF had the highest spread (68.5 mg/L). Violin plots (Figure 2) showed inlet PO4 concentrations were concentrated around 99.48 mg/L, while outlet concentrations were around 33.11 mg/L for ABR, DEWATS, LSP, and UPF, and 13.46 mg/L for WSP.
Inlet pH values ranged from 7.4 to 7.7 with minimal variation (IQR = 0.3–0.5). Outlet pH values were generally higher than inlet values for all technologies except DEWATS. LSP exhibited the widest pH range (7–12). Violin plots (Figure 2) indicated inlet pH concentrations around 7.7, while outlet pH concentrations were above 8 for all technologies except DEWATS.
3.3 Removal efficiency of the technologies for different parameters
COD removal efficiencies in various fecal sludge treatment technologies at Rohingya camps revealed that WSP were the most effective, achieving a 98.3% removal rate. DEWATS followed with a 94.4% removal rate, while UPF and ABR had similar efficiencies of 91.7% and 90.6%, respectively. LSP had the lowest efficiency at 81.6% (Figure 3). Seasonally, all technologies performed best during the monsoon, with WSP consistently showing the highest efficiency across all seasons and LSP the lowest, particularly in winter (Table 5).
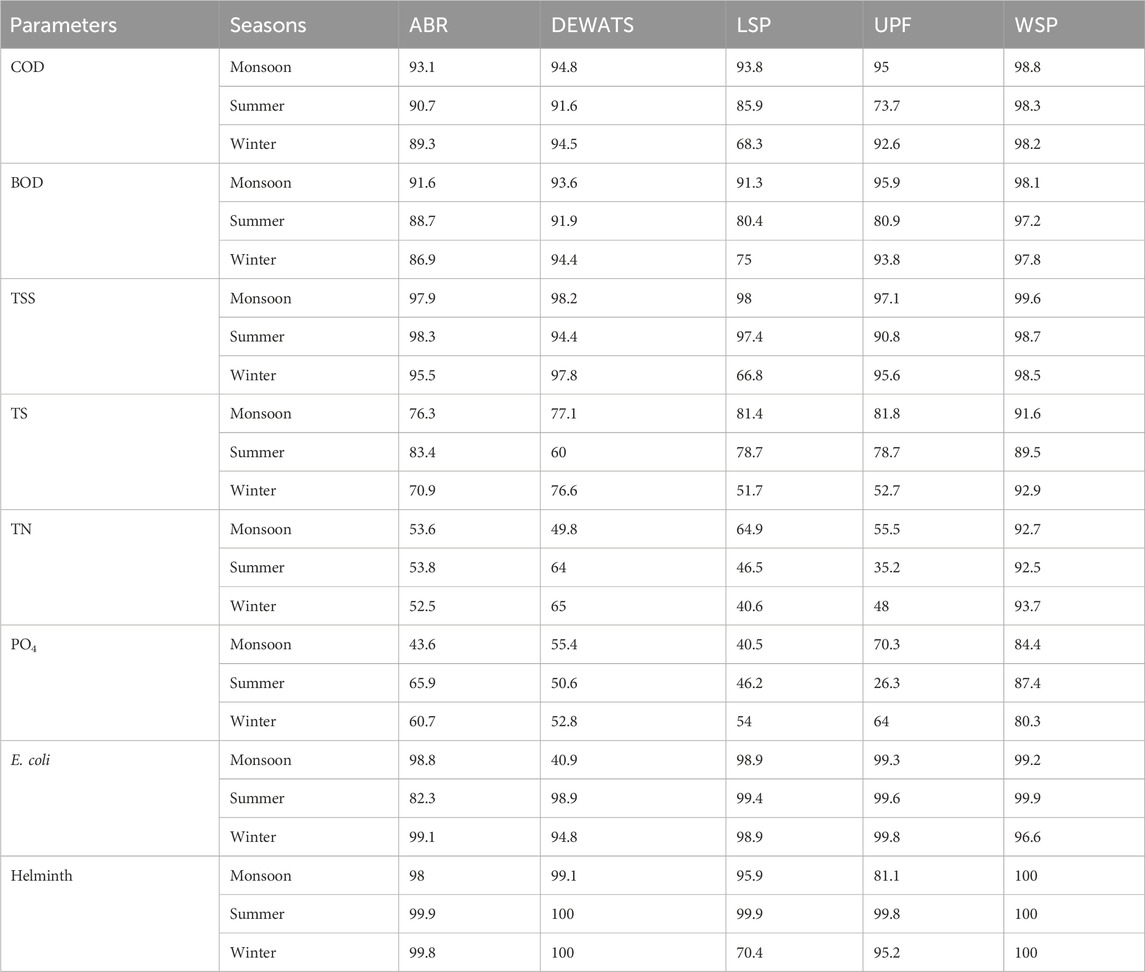
Table 5. Seasonal variations of removal efficiencies (%) among different parameters in the selected technologies.
For BOD removal, WSP had the highest efficiency at 97.8%, while LSP had the lowest at 80.9%. The removal efficiencies of DEWATS and UPF were similar, at 93.9% and 93.5%, respectively, with ABR achieving 88.5%. The mean BOD removal efficiency ranking was WSP > DEWATS > UPF > ABR > LSP (Figure 3). Seasonally, WSP maintained the highest BOD removal efficiency across all three seasons, with minimal variation. LSP consistently showed the lowest efficiency, particularly in winter (75%) compared to monsoon (91.3%) and summer (80.4%). There were no significant seasonal differences in the BOD removal efficiencies of DEWATS and ABR (Table 5).
All tested technologies effectively reduced TSS after the final treatment. WSP had the highest removal efficiency at 98.7%, while LSP was the least efficient at 86.3%. WSP, DEWATS, and ABR showed similar TSS removal efficiencies (Figure 3). Seasonally, WSP remained the most efficient across all seasons, with no significant variations. The other technologies performed best during the monsoon compared to winter and summer. LSP had a higher removal efficiency in monsoon (98%) and summer (97.4%), but significantly lower in winter (66.8%) (Table 5).
In terms of removal efficiency, WSP achieved the highest TS removal at 92.1%, while LSP had the lowest at 68.6% (Figure 3). The ranking of technologies based on TS removal efficiency is WSP > ABR > DEWATS > UPF > LSP (Figure 3). WSP consistently performed best across all seasons. Most technologies showed improved performance during the monsoon compared to winter and summer. UPF had the lowest removal efficiency in summer at 48.6%. LSP showed a significant drop in efficiency during winter at 51.7%. DEWATS and UPF performed better in the monsoon, while ABR had higher efficiency in summer than in the monsoon and winter (Table 5).
The WSP demonstrated the highest TN removal efficiency at 93.3%, outperforming other treatment technologies. Conversely, the UPF exhibited the lowest efficiency at 48.8%. Among the remaining technologies, no significant differences in removal efficiency were observed (Figure 3). Seasonally, WSP performed best during the monsoon, while DEWATS and LSP showed lower efficiency in the monsoon, summer, and winter, respectively. Notably, ABR maintained consistent TN removal efficiency across seasons, while DEWATS exhibited lower efficiency during the monsoon compared to summer and winter (Table 5).
Evaluating phosphate removal, WSP demonstrated the strongest capacity (82.8%), followed by UPF (63.7%), ABR (55.3%), DEWATS (53.7%), and lastly LSP at 49.2%. Notably, WSP and DEWATS maintained consistent performance across seasons, while UPF, ABR, and LSP exhibited variations, with UPF performing worst in summer and LSP in monsoon.
For the inlet samples, pH did not vary a lot. The median inlet pH value ranged from 7.4 to 7.7 with the least spread (IQR = 0.3–0.5) than other parameters. For the outlet samples, pH showed a comparatively higher value than their respective inlets for all the technology except DEWATS. On the other hand, LSP had the widest range which was from 7 to 12.
An evaluation of E. coli removal efficiency revealed UPF achieved the highest overall reduction (99.7%), followed by LSP (98.9%), ABR (98.6%), and WSP (97.3%). Notably, DEWATS displayed the lowest efficiency (75.6%). Seasonal variations impacted DEWATS and ABR significantly, with DEWATS dropping to 40.9% removal in monsoon compared to summer and winter. ABR also showed a substantial decrease in summer (82.3%) compared to other seasons. Conversely, WSP, UPF, and LSP maintained consistent performance throughout the year, with WSP achieving the best overall removal across all seasons.
WSP achieved the highest helminth removal efficiency at 100%, followed by DEWATS at 99.8% and ABR at 99.7%. UPF and LSP had lower efficiencies, at 93.7% and 82.3%, respectively (Figure 3). WSP consistently performed best across all seasons with 100% removal. DEWATS also maintained high efficiency (100% in summer and winter, 99.1% in monsoon), as did ABR (99.9% in summer, 99.8% in winter, 98% in monsoon). UPF was most effective in summer (99.8%), while LSP’s efficiency dropped significantly in winter (70.4%) compared to monsoon (95.9%) and summer (99.9%) (Table 5).
3.4 Removal efficiency of different technologies based on the regulatory guidelines
The outlet characteristics were compared with the Bangladesh Department of Environment (DoE) standards for sewage discharge to the receiving water bodies and land (The Environment Conservation Rules, 2023, 2023). For some parameters, Bangladesh does not have standard guidelines, in that case, outlets were compared with US, EU and WHO outlet/wastewater discharge guidelines (World Health Organization, 2006; Lu et al., 2016). Available guidelines are particularly not for the fecal sludge treatment plant outlets, but for a mixture of different wastewater including sewage discharge. Although it is evident that different technologies reduced the concentrations of most of the parameters from inlet to outlet, the concentrations recorded for BOD, COD, TN, TP, TSS, phosphate, E. coli and helminth eggs of the outlet exceeded the DoE discharge standards. However, some of the measured parameters during the study period were found to be within the guideline value (Figure 4).
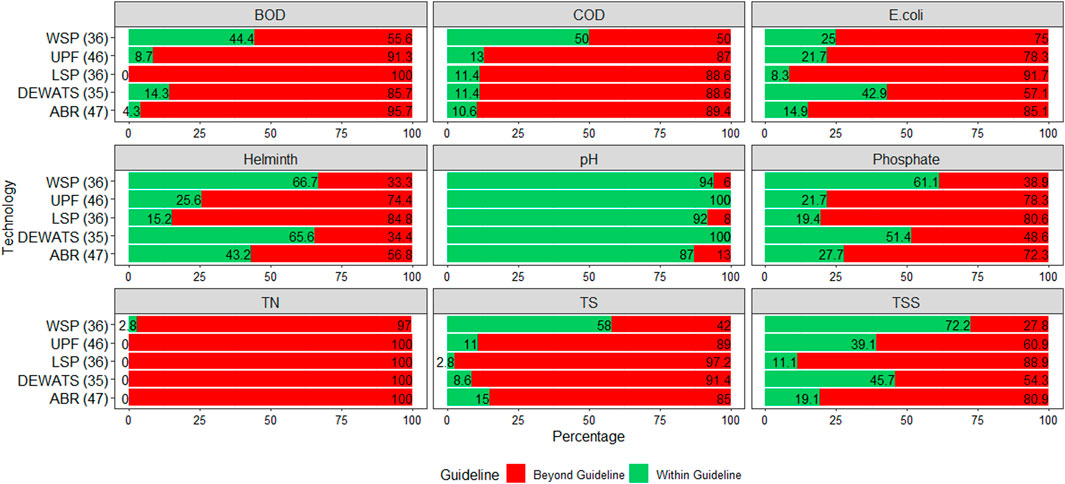
Figure 4. Regulatory guideline wise comparison (within or beyond) of different parameters among different treatment technologies.
In the case of COD and BOD, around 44.4% of the WSP outlet observations were within the guideline. However, none of the observations in LSP followed the standard guideline for BOD. In the case of pH, 100% of the observations of UPF and DEWATS were within the set guideline. In addition, pH of almost 95% of the observations in WSP and LSP followed the guideline value. In the case of phosphates, the highest percentages (61.1%) of the observations of WSP were within the standard guidelines followed by DEWATS (51.4%) and other technologies. In the case of TS, TSS and helminth, WSP showed better performances compared to other technologies based on standard guidelines (Figure 4). Almost 43% of outlet observations in DEWATS followed the discharge standard for E. coli. But for rest of the technologies it was less than 26%. For TN, none of the observations of four out of five technologies followed the standard discharge guideline and only 2.8% of the WSP followed the standard limit of outlet. Based on discharge guidelines, it can be said that WSP performed better than other FS treatment technologies in the camps. Technology-wise comparison of the percentages of outlet observations for a particular parameter within the standard guideline is depicted in Figure 4.
3.5 Correlation among different physicochemical and microbial parameters of inlets and outlets
Correlation matrices for different FSM technologies present significant relationships among different FS quality parameters. Blank spaces denote non-significant correlations, blue-colored numbers indicate a significant positive monotonic correlation and orange-colored numbers indicate a significant negative monotonic correlation. The deeper the indicating color the stronger the correlation. The Correlation matrices are depicted in Figures 5A–E.
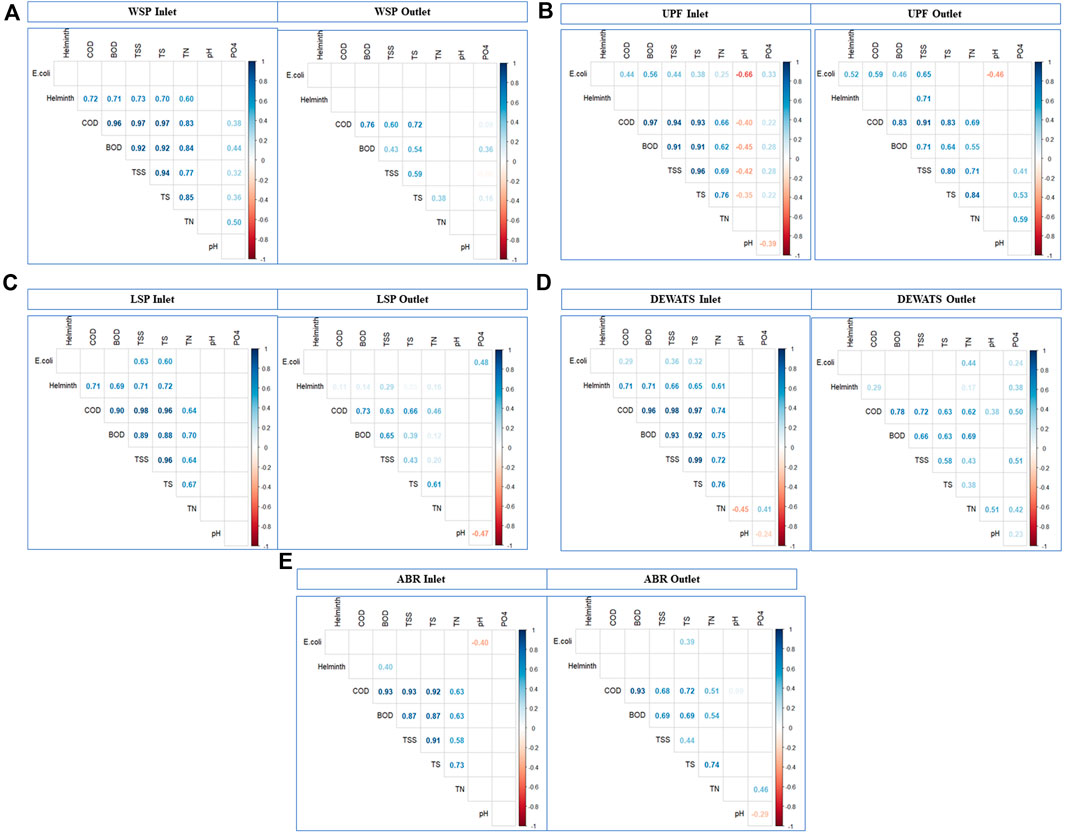
Figure 5. (A) Correlation matrix among FS quality parameters in inlet and outlet samples of ABR technology. (B) Correlation matrix among FS quality parameters in inlet and outlet samples of DEWATS technology. (C) Correlation matrix among FS quality parameters in inlet and outlet samples of LSP technology. (D) Correlation matrix among FS quality parameters in inlet and outlet samples of UPF technology. (E) Correlation matrix among FS quality parameters in inlet and outlet samples of WSP technology.
In ABR, E. coli was observed to have a moderate negative monotonic correlation with pH of the inlet whereas no correlation was found in the outlet. In the case of COD, the inlet had a very strong positive monotonic correlation with BOD, TSS, TS but in the outlet, the level of relationship demoted to a strong positive for both TSS and TS. Inlet BOD showed a very strong positive correlation with TSS and TS which decreased to a strong positive in the outlet (Figure 5A).
In DEWATS, the helminth of the inlet showed a strong positive correlation with COD, BOD, TSS, TS and TN whereas a weak positive correlation was observed with COD and PO4 in the outlet. Though COD showed a very strong positive correlation with BOD, TSS, TS in the inlet that was reduced to a strong positive correlation in the outlet. The strength of the positive correlation of BOD with TSS and TS decreased from very strong to strong from inlet to outlet. TSS and TS also showed a similar trend in the inlet and outlet. TN showed a moderate negative correlation with pH and pH showed a weak negative correlation with PO4 in inlet whereas in outlet both correlations were positive (Figure 5B).
In the case of LSP, a strong positive correlation of helminth was found with BOD, COD, TSS, and TS in the inlet whereas in the outlet no such correlation was observed. Though inlet E. coli had a strong positive correlation with TSS and TS, in the outlet only PO4 showed a moderate positive correlation with E. coli. Inlet COD and BOD had a very strong positive correlation with TSS and TS, whereas the level of correlation decreased in the outlet for each case (Figure 5C).
In UPF, E. coli showed a strong negative correlation with pH in the inlet whereas, the level of correlation decreased to moderate negative in the outlet. The level of correlation among COD, BOD and TSS decreases from inlet to outlet. Helminth showed a strong positive correlation with TSS in the outlet whereas no such correlation was observed in the inlet. Inlet pH showed a moderate negative correlation for COD, BOD, TSS and weak negative with TS whereas in outlet no such relation was observed (Figure 5D).
From WSP, the overall strength of correlation among different parameters decreases from inlet to outlet significantly. Inlet helminth showed a strong positive correlation with COD, BOD, TSS, TS and TN whereas no such correlation was observed in the outlet. Inlet COD, BOD, TSS and TS have a very strong to strong positive correlation among themselves whereas the level of correlation decreases in the outlet (Figure 5E).
4 Discussion
The performance of different FS treatment technologies in the Rohingya camps at Cox’s Bazar, Bangladesh, was assessed through various physicochemical and microbiological parameters. Significant variability was observed in the performance of these systems, with many plants failing to consistently meet discharge guidelines for key parameters such as E. coli, helminth egg count, COD, BOD, total nitrogen, TSS, and phosphate.
High median inlet E. coli counts across all technologies highlight the significant microbial contamination in the untreated sludge. The highest median inlet count was observed in WSP (4.15 × 10^6 cfu/100 mL), and the lowest in ABR systems (9 × 10^5 cfu/100 mL). The variability in E. coli removal efficiency across different plants and rounds reflects operational and maintenance inconsistencies (Tilley et al., 2014). This typical high bacterial load in fecal sludge from densely populated settings like the Rohingya camp was consistent with previous findings in similar humanitarian settings (Strande and Brdjanovic, 2014).
High removal efficiency in removing bacterial load with consistent operational conditions is considered an effective technology for fecal sludge treatment (Aghalari et al., 2020; Alam et al., 2021). UPF achieved the highest E. coli removal efficiency, indicating effective filtration and possibly disinfection processes. This high efficiency is crucial in preventing pathogen transmission in humanitarian settings (Aghalari et al., 2020). LSP and ABR also showed high efficiencies, benefiting from chemical stabilization and anaerobic digestion, respectively. These processes are known for their pathogen-reduction capabilities (Aghalari et al., 2020; UNHCR, 2024). The lower efficiency of DEWATS in E. coli removal, particularly during the monsoon, suggests challenges in maintaining effective microbial control under high loading and variable conditions. Seasonal variations highlighted the need for robust operational controls to ensure consistent pathogen removal (Strande and Brdjanovic, 2014). The outlet discharge guidelines were not consistently met by the treatment technologies used in this study. Outlet E. coli counts met the guidelines more consistently in DEWATS, with notable performance in Plant 11 across multiple rounds. This suggests better pathogen reduction potential in DEWATS systems, possibly due to their extended hydraulic retention times and multiple treatment stages (Lanoix, 1958). In contrast, ABR systems showed fewer instances of guideline compliance, indicating the need for improved operational management or supplementary disinfection processes. Failure to meet regulatory standards for E. coli levels in effluents can disrupt local ecosystems, leading to imbalances in natural microbial communities and the spread of antimicrobial resistance, as these bacteria can harbor and transfer resistance genes to other pathogens, affecting the overall management and sustainability of water resources.
Helminth eggs are particularly challenging to remove due to their resilience and size. Helminth egg counts in the inlet were highest in DEWATS (19,326 eggs/L) and lowest in ABR (6,250 eggs/L), with DEWATS also exhibiting the highest IQR. The outlet helminth counts were often within acceptable limits for DEWATS and WSP, indicating effective reduction. WSPs achieved complete helminth removal, benefiting from prolonged retention times and sedimentation processes that effectively capture and remove helminth eggs. This is consistent with findings by Jimenez, (2007), who highlighted the effectiveness of extended retention times in pathogen removal (Jimenez, 2007). DEWATS and ABR also showed high helminth removal efficiencies, reflecting effective sedimentation and biological processes that capture and degrade helminth eggs. The consistent performance across seasons further supports the reliability of these systems in helminth removal (Amoah et al., 2018; Aghalari et al., 2020). The lower efficiency of LSP in helminth removal suggests limitations in the chemical stabilization process, which may not be as effective as physical and biological processes in capturing and removing helminth eggs. The significant drop in efficiency during winter indicates challenges with chemical reactions and microbial activity at lower temperatures (Morgan-Sagastume and Allen, 2003; Leitão et al., 2006; Amoah et al., 2018). The study found sporadic compliance with helminth guidelines, with notable successes in DEWATS (e.g., Plant 10 in rounds 2, 8–12) and WSP systems (e.g., Plant 9 in rounds 2 and 8–12). Jimenez, (2007) noted that helminth removal often requires additional treatment steps such as prolonged retention times or specific filtration methods, which may not always be consistently applied in humanitarian settings (Jimenez, 2007).
High inlet concentrations of COD and BOD are typical in fecal sludge, indicative of substantial organic pollution. The inlet concentrations of COD and BOD exceeded the thresholds of 400 mg/L and 145 mg/L respectively, across all plants, emphasizing typical high organic load in untreated FS (Diener et al., 2014). UPF had the highest median inlet COD (4,910 mg/L) and BOD (1745 mg/L), indicating substantial organic matter content. The WSP demonstrated the highest BOD and COD removal efficiency, which is consistent with their extended retention times and natural treatment processes that enhance the biodegradation of organic matter. WSPs are particularly effective in organic matter removal due to sunlight penetration, algal activity, and sedimentation processes (Mara, 2013). The high efficiency across seasons indicates the robustness of WSPs in maintaining stable treatment performance. The DEWATS and UPF systems also showed high BOD and COD removal efficiencies, reflecting their designed robustness in handling high organic loads through a combination of anaerobic and aerobic processes. LSP had the lowest COD removal efficiency, likely due to the chemical nature of the treatment, which may not fully address biodegradable organic matter. Lime stabilization is effective for pathogen reduction, but it may be less effective for organic matter removal compared to biological processes (UNHCR, 2024). The significant seasonal variation, particularly lower efficiency in winter, highlights the temperature dependence of biological activity (Morgan-Sagastume and Allen, 2003; Leitão et al., 2006; UNHCR, 2024). ABR systems showed slightly lower efficiencies, potentially due to limited retention times and less effective post-treatment stages. ABRs primarily rely on anaerobic digestion, which can be less efficient in colder temperatures (Aghalari et al., 2020). There was inconsistent wastewater discharge compliance; however, certain rounds (such as ABR Plant 7 in round 5 for both COD and BOD) met the guidelines. Strande and Brdjanovic, 2014 discussed that achieving consistent reductions in organic load requires optimized operational conditions and possibly advanced treatment stages which might not be feasible in all decentralized systems (Strande and Brdjanovic, 2014).
Persistently high total nitrogen levels in the outlet indicate a significant challenge for all technologies evaluated. High inlet TN levels, with the highest median in DEWATS (2,415.6 mg/L), and persistent guideline exceedance in outlets, suggest challenges in nitrogen removal across all technologies. Nitrogen removal involves complex processes like nitrification and denitrification, which require specific conditions and operational controls, that may not be feasible in low-resource settings (Tilley et al., 2014). Effective nitrogen removal is particularly challenging in decentralized systems without tailored biological processes (Eddy et al., 2014). WSPs achieved the highest TN removal efficiency, benefiting from natural nitrification-denitrification processes facilitated by aerobic and anaerobic zones. This efficiency is particularly notable during the monsoon, likely due to increased microbial activity and dilution effects (Mara, 2013). UPF’s lower efficiency indicates potential limitations in achieving effective nitrogen removal, possibly due to insufficient retention time or incomplete nitrification-denitrification cycles. The variability in performance across seasons suggests operational challenges (CGIAR, n.d.; Al-Muyeed, 2017; Obeidat et al., 2024). The consistent performance of ABR in TN removal across seasons indicates stable anaerobic digestion processes, which are less temperature-dependent compared to aerobic processes (Aqanaghad et al., 2018).
TS and TSS removal showed inconsistent results in almost all the treatment technologies. The better performance of WSP in terms of lower outlet TSS highlights the efficacy of extended settling periods and possible natural pathogen die-off mechanisms (Rose et al., 2015). Outlet TSS levels showed better compliance compared to other parameters, particularly in DEWATS and WSP systems (e.g., DEWATS in Plant 11, rounds 2–12). Effective sedimentation and filtration mechanisms in these systems likely contribute to better performance. Proper design and maintenance of settling and filtration units are important in managing TSS (Tilley et al., 2014). WSPs achieved the highest TSS removal efficiency, likely due to effective sedimentation and natural filtration processes in the ponds (Mara, 2013). The stability of TSS removal across seasons reflects the consistent physical and biological processes at play. DEWATS and ABR systems also showed high TSS removal efficiencies, benefiting from multiple treatment stages that enhance sedimentation and filtration. On the other hand, LSP’s lower efficiency in TSS removal may be due to its reliance on chemical stabilization, which might not fully capture suspended solids compared to physical and biological processes. The sharp decline in efficiency during winter indicates potential issues with chemical precipitation at lower temperatures (Morgan-Sagastume and Allen, 2003; Leitão et al., 2006; UNHCR, 2024). DEWATS had the highest median inlet TS (8,046.6 mg/L) and outlet TS (4,087.4 mg/L), pointing to substantial solids retention but also potential re-suspension or insufficient settling. WSPs were the most effective in TS removal, benefiting from prolonged retention times and natural sedimentation. This high efficiency is consistent across seasons, indicating a stable performance of the ponds (Mara, 2013). ABR systems also showed high TS removal efficiencies, reflecting their design to handle high solid loads through sedimentation and biological processes (Strande and Brdjanovic, 2014). UPF’s lower efficiency in summer suggests issues with solid retention under high-loading conditions, possibly due to clogging or reduced filtration efficiency. LSP’s significantly lower efficiency, particularly in winter, suggests limitations in chemical treatment processes under colder conditions. The reduced microbial and chemical activity could lead to less effective solid removal (Morgan-Sagastume and Allen, 2003; Leitão et al., 2006; UNHCR, 2024).
Phosphate levels were generally within regulatory limits in several treatment rounds, indicating effective removal processes in place (e.g., ABR Plant 1 in rounds 2-4, DEWATS Plant 11 in rounds 3–12). Chemical precipitation and biological uptake are common mechanisms for phosphate removal (Crittenden et al., 2012). The better phosphate removal observed in certain rounds in UPF and WSP indicates potential variability in phosphate precipitation and microbial uptake processes. WSPs showed the highest phosphate removal efficiency and this efficiency remained consistent across seasons, highlighting the robustness of the natural treatment processes (Mara, 2013). The performance of UPF, ABR, and DEWATS systems indicates some capacity for phosphate removal, but with significant variations across seasons. This variability suggests that while biological processes contribute to phosphate removal, operational conditions such as retention time and microbial activity play crucial roles (Strande and Brdjanovic, 2014). LSP’s lower efficiency highlights the limitations of chemical precipitation processes, which may not be as effective as biological uptake in removing phosphates. Seasonal variations further suggest that colder temperatures hinder chemical reactions and microbial activity (Morgan-Sagastume and Allen, 2003; Leitão et al., 2006; UNHCR, 2024).
Maintaining pH within the optimal range is crucial for biological treatment processes. The study found that pH levels were generally compliant, with few exceptions (e.g., ABR Plant 1 round 2). pH stability is essential for microbial activity and overall treatment efficiency (Mara, 2013). Inlet pH values were relatively stable across technologies, ranging from 7.4 to 7.7, but outlet pH values were generally greater than 8 except for DEWATS (7.6). The increase in pH could be attributed to the transformation of ammonium into ammonia during the biological treatment process, enhancing the alkalinity of the treated sludge (Moestedt et al., 2016). This indicates a lower efficiency in the anaerobic process. The conversion of ammonium into ammonia decreases the useable form of nitrogen in the biological treatment process, which can hinder microbial growth and be toxic to aquatic life (Tilley et al., 2014). The wider pH range observed in LSP, extending up to 12, might be due to lime addition or other chemical treatments used to stabilize the sludge (Jimenez, 2007).
Though the FSTPs evaluated in this study achieved some level of pollutant removal, their effluent often exceeded discharge guidelines. This necessitates the implementation of post-treatment options like constructed wetlands (reed beds) to achieve acceptable discharge quality. These low-maintenance, nature-based systems can further reduce pathogen levels, organic matter, and nutrients, making the treated effluent safer for environmental release.
The present study has some limitations. The performance evaluation was based on some selected chemical and microbiological parameters only, the contribution of other factors was not taken into consideration. Fecal sludge parameter studies are limited by the number of variables that can be investigated as well as by the lack of standardization making it difficult to compare results among different studies. Therefore, a standardized approach may lead to an effective comparison among multiple studies. So far to our knowledge, there is a lack of studies on multiple treatment technologies of FSTPs. However, this study extensively investigated five FSTPs based on a set of microbiological and physicochemical parameters in a humanitarian setting which makes a foundation and scope of future studies.
5 Conclusion
The comprehensive assessment of five different fecal sludge treatment technologies in the Rohingya camp at Cox’s Bazar, Bangladesh, provides valuable insights into their removal efficiencies and treatment performance for various parameters. This study also highlights the complexity and challenges of fecal sludge management in humanitarian settings. Although some of the tested technologies showed better performance with higher removal efficiencies than others with respect to different physicochemical and microbial parameters, none of the technologies fully followed the national and international discharge standards in terms of the parameters monitored. The beyond-guideline concentrations of different physicochemical and microbiological parameters in treated fecal sludge of different treatment technologies at the camps might be due to the high initial volume overloading of FS with elevated organic and inorganic pollutants. Seasonal variations significantly affected treatment efficiencies. Most technologies performed better during the monsoon, likely due to increased microbial activity and dilution effects from rainfall. While all technologies demonstrated some capacity for contaminant removal, their performance varied across parameters and seasons, highlighting the importance of considering operational factors, treatment design and the integration of supplementary treatment processes in achieving consistent treatment efficiency. However, challenges remain in achieving consistent compliance with outlet guidelines. Therefore, extensive monitoring of the inlet and outlet with prompt outcomes is essential for achieving treatment goals, process control, and compliance with discharge standards. This will help to reduce the environmental health impacts caused by inadequate fecal sludge management.
Data availability statement
The original contributions presented in the study are included in the article/Supplementary Material, further inquiries can be directed to the corresponding author.
Author contributions
MRI: Conceptualization, Investigation, Supervision, Writing–review and editing, Data curation, Formal Analysis, Methodology, Visualization, Writing–original draft. MA: Data curation, Formal Analysis, Methodology, Visualization, Writing–original draft, Writing–review and editing. MM: Data curation, Formal Analysis, Methodology, Visualization, Writing–original draft, Writing–review and editing, Conceptualization. FG: Formal Analysis, Writing–original draft, Software, Validation. MSH: Formal Analysis, Writing–original draft, Data curation, Methodology, Writing–review and editing. MTH: Writing–review and editing, Writing–original draft. PP: Writing–review and editing, Data curation, Project administration. MTI: Writing–review and editing, Formal Analysis, Software. SU: Writing–review and editing, Methodology. MSI: Funding acquisition, Investigation, Project administration, Supervision, Validation, Writing–review and editing. MFH: Data curation, Resources, Writing–review and editing. MR: Resources, Supervision, Writing–review and editing. AC: Investigation, Writing–review and editing. TA: Funding acquisition, Project administration, Resources, Writing–review and editing. MR: Funding acquisition, Project administration, Resources, Writing–review and editing. MW: Funding acquisition, Project administration, Resources, Writing–review and editing. ZM: Conceptualization, Funding acquisition, Investigation, Supervision, Validation, Writing–review and editing.
Funding
The author(s) declare that financial support was received for the research, authorship, and/or publication of this article. This study was funded by unicef, contract number 43282825.
Acknowledgments
icddr,b acknowledges with gratitude the commitment of unicef to its research efforts. icddr,b is also grateful to the Governments of Bangladesh and Canada for providing unrestricted support.
Conflict of interest
The authors declare that the research was conducted in the absence of any commercial or financial relationships that could be construed as a potential conflict of interest.
Publisher’s note
All claims expressed in this article are solely those of the authors and do not necessarily represent those of their affiliated organizations, or those of the publisher, the editors and the reviewers. Any product that may be evaluated in this article, or claim that may be made by its manufacturer, is not guaranteed or endorsed by the publisher.
Supplementary material
The Supplementary Material for this article can be found online at: https://www.frontiersin.org/articles/10.3389/fenvs.2024.1397389/full#supplementary-material
References
Aghalari, Z., Dahms, H.-U., Sillanpää, M., Sosa-Hernandez, J. E., and Parra-Saldívar, R. (2020). Effectiveness of wastewater treatment systems in removing microbial agents: a systematic review. Glob. Health 16, 13–11. doi:10.1186/s12992-020-0546-y
Alam, M.-U., Ferdous, S., Ercumen, A., Lin, A., Kamal, A., Luies, S. K., et al. (2021). Effective treatment strategies for the removal of antibiotic-resistant bacteria, antibiotic-resistance genes, and antibiotic residues in the effluent from wastewater treatment plants receiving municipal, hospital, and domestic wastewater: protocol for a systematic review. JMIR Res. Protoc. 10, e33365. doi:10.2196/33365
Al-Muyeed, A. (2017) Technical guidelines for designing a decentralised waste water treatment system, 288701, 27.
Altare, C., Kahi, V., Ngwa, M., Goldsmith, A., Hering, H., Burton, A., et al. (2019). Infectious disease epidemics in refugee camps: a retrospective analysis of UNHCR data (2009-2017). J. Glob. Heal. Rep. 3, e2019064. doi:10.29392/joghr.3.e2019064
Amoah, I. D., Reddy, P., Seidu, R., and Stenström, T. A. (2018). Removal of helminth eggs by centralized and decentralized wastewater treatment plants in South Africa and Lesotho: health implications for direct and indirect exposure to the effluents. Environ. Sci. Pollut. Res. 25, 12883–12895. doi:10.1007/s11356-018-1503-7
Aqanaghad, M., Mousavi, G., and Ghanbari, R. (2018). Anaerobic baffled reactor and hybrid anaerobic baffled reactor performances evaluation in municipal wastewater treatment. Iran. J. Heal. Saf. Environ. 5, 1027–1034.
Ayres, R. M., and Mara, D. D.World Health Organization (1996). Analysis of wastewater for use in agriculture: a laboratory manual of parasitological and bacteriological techniques. Geneva: World Health Organization.
CGIAR (n.d.). Design and development of bio-treatment technique for Decentralized Wastewater Treatment system.
Crittenden, J. C., Trussell, R. R., Hand, D. W., Howe, K. J., and Tchobanoglous, G. (2012). MWH’s water treatment: principles and design. John Wiley and Sons.
Diener, S., Semiyaga, S., Niwagaba, C. B., Muspratt, A. M., Gning, J. B., Mbéguéré, M., et al. (2014). A value proposition: resource recovery from faecal sludge—can it be the driver for improved sanitation? Resour. Conserv. Recycl. 88, 32–38. doi:10.1016/j.resconrec.2014.04.005
Eddy, M., Abu-Orf, M., Bowden, G., Burton, F. L., Pfrang, W., Stensel, H. D., et al. (2014). Wastewater engineering: treatment and resource recovery. New York: McGraw Hill Education.
Evans, J. D. (1996). Straightforward statistics for the behavioral sciences. Pacific Grove, CA: Thomson Brooks/Cole Publishing Co.
Hossain, M. S., Ali, S., Hossain, M., Uddin, S. Z., Moniruzzaman, M., Islam, M. R., et al. (2021). ESBL producing Escherichia coli in faecal sludge treatment plants: an invisible threat to public health in Rohingya camps, Cox’s bazar, Bangladesh. Front. Public Heal. 9, 783019. doi:10.3389/fpubh.2021.783019
Inter Sector Coordination GroupCluster (2018). Bangladesh: fecal sludge management summary map. Available at: https://reliefweb.int/map/bangladesh/bangladesh-fecal-sludge-management-summary-map-december-24-2018 (Accessed January 21, 2019).
Inter Sector Coordination Group (2018). WASH sector strategy for rohingyas influx March to december 2018. Available at: https://reliefweb.int/report/bangladesh/wash-sector-strategy-rohingyas-influx-march-december-2018.
Inter Sector Coordination Group (2022). Bangladesh Cox’s bazar: WASH infrastructure (fecal sludge treatment plants - FSTPs) location map. Available at: https://reliefweb.int/map/bangladesh/bangladesh-coxs-bazar-wash-infrastructure-fecal-sludge-treatment-plants-fstps-location-map-updated-may-09-2022 (Accessed September 20, 2022).
Islam, M. M., Jarna, R. N., Khan, D. H., Nayeem, M., Rushmi, Z. T., Rahman, M. M., et al. (2019). Prevalence of diseases among Rohingya refugees in Bangladesh: a comprehensive study. IOSR J. Nurs. Heal. Sci. 8, 14–21. doi:10.9790/1959-0802091421
Jimenez, B. (2007). Helminth ova removal from wastewater for agriculture and aquaculture reuse. Water Sci. Technol. 55, 485–493. doi:10.2166/wst.2007.046
Klingel, F., Montangero, A., Koné, D., and Strauss, M. (2002). Fecal sludge management in developing countries. A Plan. Man. EAWAG Swiss Fed. Inst. Environ. Sci. Technol. SANDEC Dep. Water Sanit. Dev. Ctries.
Leitão, R. C., Van Haandel, A. C., Zeeman, G., and Lettinga, G. (2006). The effects of operational and environmental variations on anaerobic wastewater treatment systems: a review. Bioresour. Technol. 97, 1105–1118. doi:10.1016/j.biortech.2004.12.007
Lu, X., Zhou, B., Vogt, R. D., Seip, H. M., Xin, Z., and Ekengren, Ö. (2016). Rethinking China’s water policy: the worst water quality despite the most stringent standards. Water Int. 41, 1044–1048. doi:10.1080/02508060.2016.1219188
Mahmood, S. S., Wroe, E., Fuller, A., and Leaning, J. (2017). The Rohingya people of Myanmar: health, human rights, and identity. Lancet 389, 1841–1850. doi:10.1016/s0140-6736(16)00646-2
Microsoft Corporation (2019). Microsoft Excel. Available at: https://office.microsoft.com/excel
Moe, C. L., and Rheingans, R. D. (2006). Global challenges in water, sanitation and health. J. Water Health 4, 41–57. doi:10.2166/wh.2006.0043
Moestedt, J., Müller, B., Westerholm, M., and Schnürer, A. (2016). Ammonia threshold for inhibition of anaerobic digestion of thin stillage and the importance of organic loading rate. Microb. Biotechnol. 9, 180–194. doi:10.1111/1751-7915.12330
Morgan-Sagastume, F., and Allen, D. G. (2003). Effects of temperature transient conditions on aerobic biological treatment of wastewater. Water Res. 37, 3590–3601. doi:10.1016/s0043-1354(03)00270-7
Ngakane, L. (2021). Health concerns related to housing, sanitation, water access and waste disposal in a poor mixed urban community. Mbekweni Paarl.
Nicholson, F. A., Smith, S. R., Alloway, B. J., Carlton-Smith, C., and Chambers, B. J. (2003). An inventory of heavy metals inputs to agricultural soils in England and Wales. Sci. Total Environ. 311, 205–219. doi:10.1016/s0048-9697(03)00139-6
Obeidat, N., Shatanawi, K., Kassab, G., and Halalsheh, M. (2024). Performance of decentralized wastewater treatment system employing Upflow anaerobic sludge blanket and Vertical Flow Constructed Wetland. Case Stud. Chem. Environ. Eng. 9, 100695. doi:10.1016/j.cscee.2024.100695
Otaka, G., Okullo, A., Niwagaba, C. B., Kulabako, R. N., and Katukiza, A. Y. (2019). Evaluation of the efficiency and benefits of a pilot scaled decentralized faecal sludge treatment system in kampala.
Pfaff, J. D. (1993). Method 300.0 Determination of inorganic anions by ion chromatography. U. S. Environ. Prot. agency, Off. Res. Dev. Environ. Monit. Syst. Lab. 28.
R Core Team (2021). R: a language and environment for statistical computing. Available at: https://www.r-project.org/.
Rice, E. W., Baird, R. B., and Eaton, A. D. (2017). Standard methods for the examination of water and wastewater. 23rd ed.
Richardson, S. D., and Ternes, T. A. (2014). Water analysis: emerging contaminants and current issues. Anal. Chem. 86, 2813–2848. doi:10.1021/ac500508t
Rose, C., Parker, A., Jefferson, B., and Cartmell, E. (2015). The characterization of feces and urine: a review of the literature to inform advanced treatment technology. Crit. Rev. Environ. Sci. Technol. 45, 1827–1879. doi:10.1080/10643389.2014.1000761
Schmid, H., and Huber, A. (2014). Measuring a small number of samples, and the 3v fallacy: shedding light on confidence and error intervals. IEEE Solid-State Circuits Mag. 6, 52–58. doi:10.1109/mssc.2014.2313714
Smith, V. H., Tilman, G. D., and Nekola, J. C. (1999). Eutrophication: impacts of excess nutrient inputs on freshwater, marine, and terrestrial ecosystems. Environ. Pollut. 100, 179–196. doi:10.1016/s0269-7491(99)00091-3
Strande, L., and Brdjanovic, D. (2014). Faecal sludge management: systems approach for implementation and operation. London: IWA publishing.
Strande, L., Schoebitz, L., Bischoff, F., Ddiba, D., Okello, F., Englund, M., et al. (2018). Methods to reliably estimate faecal sludge quantities and qualities for the design of treatment technologies and management solutions. J. Environ. Manage. 223, 898–907. doi:10.1016/j.jenvman.2018.06.100
Tilley, E., Ulrich, L., Lüthi, C., Reymond, P., and Zurbrügg, C. (2014). Compendium of sanitation systems and technologies.
UNICEF (2020). Variation of microbiological and physicochemical properties of untreated (inlet) and treated (outlet) faecal sludge samples of different treatment plants in the existing FSM technologies from the 5th round of sampling in the Rohingya Camps, Cox’s Bazar.
Wijnroks, M., Bloem, M. W., Islam, N., Rahman, H., Das, S. K., Hye, A., et al. (1993). Surveillance of the health and nutritional status of Rohingya refugees in Bangladesh. Disasters 17, 348–356. doi:10.1111/j.1467-7717.1993.tb00507.x
World Health Organization (2006). WHO guidelines for the safe use of wasterwater excreta and greywater. Geneva: World Health Organization.
Keywords: E. coli, physicochemical parameters, removal efficiency, fecal sludge management, humanitarian settings, Rohingya camps, wastewater treatment, Bangladesh
Citation: Islam MR, Alam MAU, Moniruzzaman M, Galib FC, Hossain MS, Hussain MT, Paul P, Islam MT, Uddin SZ, Islam MS, Hossain MF, Rahman MM, Chowdhury AIA, Ananya TH, Rahman MA, Worth M and Mahmud ZH (2024) Exploring fecal sludge treatment technologies in humanitarian settings at Cox’s Bazar, Bangladesh: a comprehensive assessment of treatment efficiency through characterization of fecal sludge. Front. Environ. Sci. 12:1397389. doi: 10.3389/fenvs.2024.1397389
Received: 07 March 2024; Accepted: 05 August 2024;
Published: 20 August 2024.
Edited by:
Ahmed El Nemr, National Institute of Oceanography and Fisheries (NIOF), EgyptReviewed by:
Ciara Keating, Durham University, United KingdomHeinz-Peter Mang, University of Science and Technology Beijing, China
Copyright © 2024 Islam, Alam, Moniruzzaman, Galib, Hossain, Hussain, Paul, Islam, Uddin, Islam, Hossain, Rahman, Chowdhury, Ananya, Rahman, Worth and Mahmud. This is an open-access article distributed under the terms of the Creative Commons Attribution License (CC BY). The use, distribution or reproduction in other forums is permitted, provided the original author(s) and the copyright owner(s) are credited and that the original publication in this journal is cited, in accordance with accepted academic practice. No use, distribution or reproduction is permitted which does not comply with these terms.
*Correspondence: Zahid Hayat Mahmud, emhtYWhtdWRAaWNkZHJiLm9yZw==