- 1MIT Center for Sustainability Science and Strategy, Massachusetts Institute of Technology, Cambridge, MA, United States
- 2Shell Scenarios Team, Shell International, London, United Kingdom
- 3Shell International Exploration and Production B. V., The Hague, Netherlands
- 4Shell Global Solutions International B.V., Amsterdam, Netherlands
Achieving a low-carbon future requires a comprehensive approach that combines emission mitigation options from economic activities with the sustainable use of land for numerous needs: food production, energy production, carbon sequestration, nature preservation and broad ecosystem services. Using the MIT Integrated Global System (IGSM) framework we analyze land-use competition in a 1.5°C climate stabilization scenario, in which demand for bioenergy and natural sinks increase along with the need for sustainable farming and food production. We find that to address the numerous trade-offs, effective approaches to nature-based solutions (NBS) and agriculture practices are essential. With proper regulatory policies and radical changes in current practices, global land is sufficient to provide increased consumption of food per capita (without large diet changes) over the century while also utilizing 2.5–3.5 billion hectares (Gha) of land for NBS practices that provide a carbon sink of 3–6 gigatonnes (Gt) of CO2 per year as well as 0.4–0.6 Gha of land for energy production—0.2–0.3 Gha for 50–65 exajoules (EJ) per year of bioenergy and 0.2–0.35 Gha for 300–600 EJ/year of wind and solar power generation. We list the competing uses of land to reflect the trade-offs involved in land use decisions, and note that while there is sufficient land in our scenario, attaining this outcome, capable of delivering a 1.5°C future, requires effective policies and measures at national and global levels that promote efficient land use for food, energy and nature (including carbon sequestration) and ensure long-term commitments by decision makers from governments and industry in order to realize the benefits of climate change mitigation.
1 Introduction
A transformation of both the energy system and land use are necessary in order to limit global warming to 1.5°C while also meeting needs for food and environmental sustainability (IPCC, 2023). This has led to widespread concern that there may not be enough land to meet all the needs for food and nature whilst providing land for urban environments, energy production, and nature-based carbon sequestration (e.g., Searchinger and Heimlich, 2015; Rulli et al., 2016; Fehrenbach et al., 2023). Following the goals of the Paris Agreement (UN, 2015), numerous governments, international organizations and companies advocate for and explore sustainable pathways to net-zero emissions. Achieving the net-zero targets requires not only reducing anthropogenic greenhouse gas (GHG) emissions, but also increasing removals of GHG from the atmosphere (IPCC, 2023; Morris et al., 2023).
Recent studies have shown that the land sector plays an especially important role in such low-emissions pathways, particularly through land-based carbon dioxide removal (e.g., bioenergy with carbon capture and storage (BECCS), afforestation/reforestation and other nature-based solutions (NBS)), energy crops and changing agricultural practices (Roe et al., 2019; Hasegawa et al., 2021; Morris et al., 2024). Land for the expansion of wind and solar generation, and potentially direct air capture facilities, adds further pressure for land (e.g., MIT Joint Program, 2023; van de Ven et al., 2021). Given the broad range of climate change mitigation options that rely on land, careful consideration of trade-offs between those options is needed. For example, those options vary in terms of the amount of land required, their mitigation/sequestration potential, their energy production, their implications for food security, and their impact on wider ecosystem services such as the ability to improve biodiversity or combat desertification and land degradation.
Particular attention has been given to trade-offs between using land for BECCS and/or NBS vs. for food production and/or nature preservation (e.g., Boysen et al., 2017; Busch et al., 2019; IPCC, 2020; Donnison et al., 2020; Seddon et al., 2020; Seddon et al., 2021; Vera et al., 2022). While globally, the sheer amount of useable land (see Section 2) does not appear to be a limiting factor for multiple uses of land, it is not clear how realistic it is to pursue all potential options at once. Lastly, increasing land competition may impact the resilience of natural land carbon sinks that play a crucial role in partially mitigating anthropogenic climate change (Ruehr et al., 2023).
Decisions will need to be made about how to best use land in any given location for a sustainable low-carbon future. Toward that end, there are many estimates of the amount of land that could be demanded for different purposes. The Food and Agriculture Organization (FAO) of the United Nations estimated that the global arable land area must increase by 165 million hectares (Mha)1 to guarantee food and material supplies by 2050, which corresponds to an expansion of arable land by 11% (FAO, 2018). FAO stated that the additional land requirement may increase to 325 Mha (or 21% expansion) under less favorable prospects for population growth, dietary changes, climate impacts on yields, rate of technological progress and institutional aspects (FAO, 2018). For the high warming scenarios, several studies project substantial crop yield losses of 10% or more (Hsiang et al., 2017; Robertson et al., 2018; Sue Wing et al., 2021; Hasegawa et al., 2022; IPCC, 2023; Rezaei et al., 2023). Climate change mitigation helps to alleviate these damages. For 1.5°C stabilization, crop yield impacts are estimated to be close to zero (Rezaei et al., 2023; Hasegawa et al., 2022), which provides another incentive for rapid global emission reduction actions.
Emission mitigation scenarios often envision substantial increases in bioenergy production. Fajardy et al. (2021) evaluated that land needs for bioenergy by 2100 under 1.5°C or 2°C stabilization may require between 80 Mha (to produce only 22 EJ/yr of primary energy) and more than 500 Mha (to achieve 300–400 EJ/yr), depending on BECCS availability, biomass yields and climate stabilization targets. Falling within that range, Smith et al. (2016) estimated 380 Mha of land required for 170 EJ/yr of bioenergy in 2100. To provide context, the current global total energy consumption is about 630 EJ/year, of which about 10% is from bioenergy and traditional use of biomass (IEA, 2023).
The use of NBS, sometimes also referred as Natural Climate Solutions (NCS; Environmental Defense Fund, 2024), has been estimated to require active management of more than 4 billion hectares (Gha) to achieve their maximum potential contribution of ∼30 GtCO2e/yr in mitigation (Roe et al., 2021). However, that is a technical maximum combining all NBS activities at once, and a more feasible estimate is in the range of 2 Gha to achieve a cost-effective potential contribution to mitigation. For context, the global land area dedicated to agriculture (crops and livestock) exceeds 4 Gha and estimates of additional land area available for reforestation are 678–900 Mha (Griscom et al., 2017; Bastin et al., 2019). At the same time, estimates of global area of abandoned agriculture are quite sizeable, between 390 Mha and 475 Mha (Campbell et al., 2008).
With many competing demands for land, the question arises whether there is enough land to meet climate goals and agricultural/food needs while preserving and restoring ecosystem functions, including biodiversity. Is it possible to allocate land for food, carbon sequestration, energy and nature globally and regionally to address both human and environmental needs? What are the required actions and changes in land use to achieve these needs?
The goal of this paper is to assess the land competition that arises while achieving a 1.5°C temperature stabilization target. In particular, this paper seeks to determine whether global land can meet competing needs for food, carbon sequestration, energy, and nature preservation. To quantify the trajectories of land use and its implications, we use a tool that provides an interconnected representation of the physical and socio-economic systems, the MIT Integrated Global System Modeling (IGSM) framework (Sokolov et al., 2018). We employ MIT IGSM and its socio-economic component, the MIT Economic Projection and Policy Analysis (EPPA) model, to assess the land implications of the Sky 2050 scenario (Shell, 2023), where the world is developing in increasingly sustainable directions across both land and energy systems. Sky 2050 is a 1.5°C scenario with an overshoot (category C2 by the definition of the IPCC (IPCC, 2023)) which was designed to achieve a global net-zero target for total anthropogenic CO2 emissions (i.e., energy + industry + land use) in the year 2050. When including non-CO2 GHGs, net emissions in this scenario decline to zero in 2062 and stay below zero until 2100. The profiles for GHG emissions and the temperature implications of this scenario are described in Sokolov et al. (2023). While for illustrative purposes we focus on Sky 2050 (namely because it involves a detailed consideration of NBS and provides public information for many relevant energy and land characteristics), similar land competition and trade-offs will be required in other scenarios that target 1.5°C stabilization, such as those summarized by IPCC (2023) or those developed by other entities (e.g., IEA, 2023; MIT Joint Program, 2023).
In the following sections, this paper: i) summarizes current global land use, ii) describes the methods we use for assessing future trajectories for land use, iii) discusses global and regional land-use implications, iv) evaluates the feasibility of achieving the land-use optimization needed by such a 1.5°C scenario, and v) discusses the needs for fast and radical changes to achieve climate stabilization goals.
2 Current global land use and land-use emissions
Land covers almost 15 Gha of our planet’s surface, distributed among habitable land, glaciers (ice covered areas), and barren areas (FAO, 2019). As we discuss later, different sources (e.g., FAO, IPCC) use different land use/land cover categorization, and, as a result, the total 15 Gha is sometimes divided in a different fashion between several land use classes. In Figure 1, we use the data from FAO (2019) to illustrate how the current use of the 10.4 Gha of habitable land is split between five major categories: two categories are related to agriculture (crops and livestock) and three categories are related to other uses and covers (forests, shrubland, and urban areas).
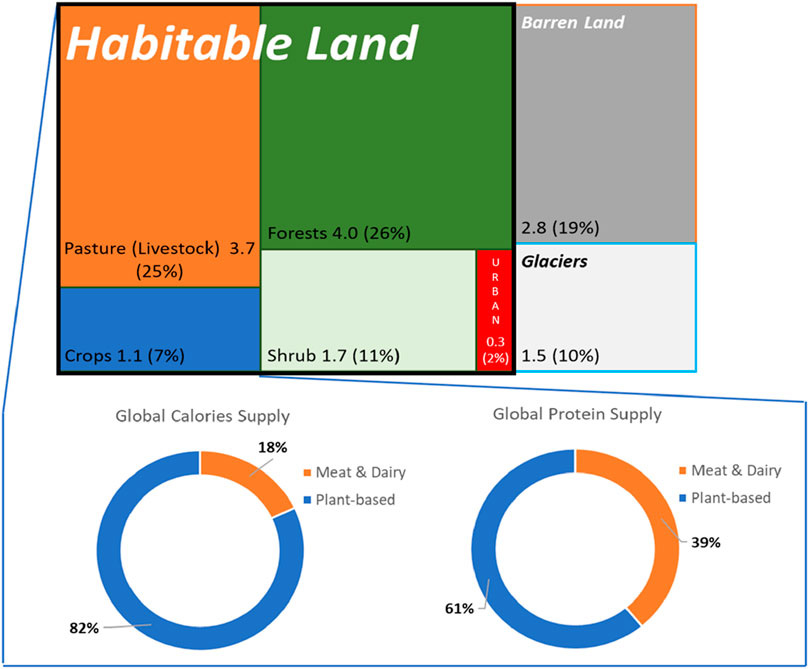
Figure 1. Current global land use (in gigahectares, Gha, and percentage of total) by land category and shares of animal and plant-based sources in global supply of calories and protein. Data source: Ritchie and Roser (2013), FAO (2019).
As shown, the global area dedicated to livestock production occupies 3.7 Gha and global cropland uses another 1.1 Gha. These categories of agricultural land provide different contributions for the global supply of calories and protein. Although the global area dedicated to crops is only one third of the area for livestock and dairy, crops provide 82% of the total calories and 61% of the global protein supply (FAO, 2023). As for other land uses, forests and shrublands account for 4.0 Gha and 1.7 Gha, respectively. Urban areas and freshwater areas occupy additional 0.3 Gha.
Land used for energy production is not typically a land use/land cover category estimated in most global data sources, and therefore needs to be estimated. To evaluate the land areas used for energy production, we apply the estimates from Shell (2023) for wind and solar and the EPPA model estimates for bioenergy based on the approach from Winchester and Reilly (2015). The 2020 land requirements for wind and solar are consistent with the estimates from the U. S. National Renewable Laboratory (NREL, 2019), where 1 MW of solar generating capacity requires about 1 ha of land, and 1 MW of wind capacity requires 15–20 ha of land. For wind, only 1%–2% of that area is used directly by turbines and other supporting infrastructure, and the remaining area might be used for other purposes (e.g., farming). However, in this study we are interested in the upper bound for a potential land use. Hence, in our estimates we assign the full area to wind generation. We also separate onshore and offshore wind based on the BNEF 2022 Energy Outlook (BloombergNEF, 2022), and our wind-related reporting reflects only the areas for onshore generation. Over time, the amount of wind and solar energy produced on a particular land area is prescribed to increase due to improved packing density and conversion efficiency. We follow Shell (2023) and use the following trajectories for energy generated per year per ha: 3.4 TJ/ha for solar and 0.57 TJ/ha for wind in 2020, 7.4 TJ/ha for solar and 0.58 TJ/ha for wind in 2050, and 9.5 TJ/ha for solar and 0.58 TJ/ha for wind in 2100.
Table 1 shows the resulting estimates of global and regional land use for energy. In the regions with substantial bioenergy production (Brazil, Europe, United States), the share of land used for energy is between 1.7% and 2.6%. However, the global share of land dedicated to energy is less than 1%. Note that we do not include the land areas for fossil fuel production and mining of critical materials.
Changes in the uses of land induce changes in related emissions. According to IPCC (2023), the corresponding net anthropogenic GHG emissions from land use, land-use change and forestry (LULUCF) are subject to large uncertainties and high annual variability. Global LULUCF CO2 emissions (annual average for 2010–2019) are estimated to be in the range of 5.9 ± 4.1 GtCO2e/yr (IPCC, 2023). The Global Carbon Project in its 2023 edition estimates the 2022 global net anthropogenic land-use change emissions to be 4.2 GtCO2e/yr (Friedlingstein et al., 2023). While different editions of the Global Carbon Project provide different estimates for historic land-use change emissions, the recent estimates seem to settle at a number around 4 GtCO2e/yr.
It is worth to note that alternative methodological approaches for estimating emissions from Agriculture, Forestry and Other Land Uses (AFOLU) lead to different values. National GHG inventory reporting separates the net flux from LULUCF and the net flux from Agriculture (IPCC, 2023). They include direct human-induced effects and, in most cases, indirect effects due to anthropogenic environmental change. These include changes in biomass carbon stock in forest land that may include unmanaged natural forest. Food and Agriculture Organization of the United Nations (FAO) follows the similar approach in reporting emissions from agri-food systems that include land use and land use change emissions. However, according to IPCC (2023), for calculating anthropogenic land CO2 in integrated assessment models and book-keeping models, such as Global Carbon Project, only the impacts of direct effects and only for those areas that are subject to intense and direct management are considered. These models also estimate non-anthropogenic land CO2 flux, and separations between anthropogenic and non-anthropogenic land CO2 fluxes are not always consistent (IPCC, 2023). Typically, national GHG inventories report lower net land-use emissions with a global total between 1 and 1.5 GtCO2e/yr in 2016–2019 (IPCC, 2023). For the Sky 2050 scenario, we have used the data consistent with the Global Carbon Budget estimates with historic land-use change CO2 emissions around 4 GtCO2e/yr (Sokolov et al., 2023).
How LULUCF emissions will change in the future depends on future decisions about how to use land, given competition for multiple uses. The IPCC (2023) points to the potential for anthropogenic land-use to transition from being a net-source of GHG emissions today to a large net-sink (8–14 GtCO2e/yr) by mid-century, if land-use carbon mitigation is supported at the level of 100 USD/tCO2e. In addition, IPCC’s illustrative mitigation pathways include net CO2 removal on managed land with a range of 0.23–6.38 GtCO2/yr in 2050. On such timescales, the natural land carbon sink also acts to partially buffer CO2 exchange between the atmosphere and biosphere through CO2 fertilization of photosynthesis (see Ruehr et al. (2023) for a comprehensive review).
3 Methods
To explore potential future land competition, we combine detailed bottom-up modeling/estimates (i.e., an approach that considers specific activities, pathways, and challenges at a detailed level) of land uses from the Sky 2050 scenario with the top-down modeling (i.e., an aggregate-level representation of the main driving forces and their impacts) of land use change using the MIT Economic Projection and Policy Analysis (EPPA) model, each of which is described below.
3.1 Bottom-up modeling of the potential for nature-based solutions (NBS)
The Sky 2050 scenario is designed to meet the goal of net-zero (anthropogenic) CO2 by 2050; it is a “high overshoot” 1.5°C pathway (i.e., C2 category), based on IPCC’s definition, sitting in the middle of the IPCC scenarios’ C2 range (Sokolov et al., 2023). The scenario is unique among 1.5°C scenarios due to its detailed consideration of NBS, using country level modeling for both energy and the development of NBS, in an integrated narrative. NBS achieve atmospheric CO2 reduction through the conservation, restoration, or altered management of natural ecosystems (Waring et al., 2023). The Sky 2050 scenario seeks to recognize that implementing change, scaling up technologies or rolling out changes in land-use takes time to build when they are in their infancy today. The land-use analysis in the scenario draws heavily on the academic literature reviewed by the latest assessment of scientific findings (Sixth Assessment Report) by the Intergovernmental Panel on Climate Change (IPCC, 2023) for the long-term and cumulative potentials for NBS.
In particular, data developed by Cook-Patton et al. (2020) and the underlying references of Roe et al. (2021) are used for the modeling of the scale-up of NBS and the total ultimate potential land areas and cumulative CO2 impact for protection, improved management or restoration. While the Sky 2050 scenario is highly ambitious, it does apply cost constraints for all NBS activities that require a change in land use or management (e.g., reforestation, regenerative agriculture, peatland/forest management). In related literature this is commonly referred to as the “cost-effective” available area and implies that implementing the NBS activity would require no more than 100 USD/tCO2 (Roe et al., 2021; IPCC, 2023). The analysis is built up from a combination of changing land areas under management together with profiles of CO2 stored and taken up through time in different ecosystems. Temporal profiles are based on an analysis that uses country-, NBS pathway-, and scenario-specific profiles of how hectares under management (i.e., “enrolled”) scale up.
The approach assesses how five categories of barriers (economic, political, technological, socio-cultural and environmental) will affect the deployment rate, separately across 19 different NBS pathways for 200+ countries, for each scenario. For simplicity, all pathways start this enrollment in 2023, but many do not reach material scale for several years or even decades, dependent on the pathway and country. The greater the number of barriers to overcome, the slower the scale-up to the peak rate (ha/yr) of enrollment. The calibration of peak rates is based on a combination of expert elicitation, comparison with historic enrollment rates of ambitious NBS projects (e.g., US national forest expansion in the 1930s, large-scale reforestation campaigns in China in recent years) and validation against current ambitions (e.g., United Kingdom net-zero reforestation ambitions – Committee of Climate Change, 2020). The resulting anthropogenic land-use emissions fall from a net source of 4 GtCO2/yr today to a net sink of −6.1 GtCO2/yr in 2050 (Sokolov et al., 2023). The cumulative anthropogenic land-use emissions in 2023–2100 in the Sky 2050 scenario are −312 GtCO2.
The second part of the calculation uses existing literature (Roe et al., 2019) to distribute rates of CO2 removal and avoidance on a unit area and time basis (i.e., tCO2/ha/yr). This uses country-specific rates for each different NBS type and applies a temporal distribution that describes that activity’s temporal impact on mitigation (e.g., 1 hectare of avoided deforestation generates all emission reductions at the time the area is protected, whereas emission reductions generated by reforestation follow a lognormal distribution starting from the year trees are planted). For more detail on this calculation, see Barros et al. (2023). Many of these temporal profiles are generalized across several countries or climatic zones, but do represent the fact that reforestation in tropical zones may reach peak CO2 sequestration rates within 6 years whereas reforestation in mid or high latitudes may take 10+ years after saplings have been planted.
Additionally, forests in different regions may reach equilibrium and limit additional sequestration over different time horizons (Cook-Patton et al., 2020). Avoidance pathways use the most recent assessment of current carbon stock levels. For some, such as avoided deforestation, if a hectare is protected then the emission reductions are accounted for in the year in which the hectares are enrolled, but the land area is considered enrolled and managed indefinitely, despite no longer generating any emission reductions. For other NBS activities, such as fire management in savannahs, avoided emissions can be ongoing. An important result from the two steps of the calculation is that both the scaling up of capacity to enroll hectares in land management programs, and factoring in the time from enrollment to CO2 uptake, act as lags in removing CO2 from the atmosphere. This bottom-up approach produces projections of land use that account for realistic constraints to the deployment of NBS.
3.2 Top-down modeling: the economic projection and policy analysis (EPPA) model
To assess land-use competition between different categories of land, we use the MIT Economic Projection and Policy Analysis (EPPA) model. EPPA is a dynamic multi-sector, multi-region computable general equilibrium (CGE) model of the world economy (Paltsev et al., 2005; Chen et al., 2022). It is designed to develop projections of economic growth, energy transitions and anthropogenic emissions of greenhouse gas and air pollutants. Land use changes in EPPA are explicitly modeled by land use transitions among five major land categories (cropland, pasture, managed forest, natural grassland and natural forest) taking into account costs of transitions and associated emissions and maintaining consistent supplemental physical accounts of land (Gurgel et al., 2016; Gurgel et al., 2021).
Conversion of natural areas to agriculture follows a land supply response based on land conversions observed over the past few decades. Land can move to a less intensely managed use (e.g., from cropland to pasture or forest) or be abandoned completely and return to “natural” grass or forest land if investment in managed land is not maintained. Direct and indirect emissions associated with land use changes are represented in the model. Agricultural goods (e.g., crops, livestock, forestry) are produced by combining land, capital, labor, energy and intermediate inputs under multi-nested Constant Elasticity of Substitution (CES) functions. Alternative bioenergy technologies (e.g., liquid biofuels, bioelectricity, BECCS) are considered in EPPA, and require cropland areas to be deployed.
Future projections in the EPPA model are driven by economic decisions related to savings and investments, productivity improvements in labor, capital, land and energy, changing demand for goods and services due to income growth and international trade, and depletion of natural resources. These economic drivers, combined with imposed policies such as GHG emissions constraints, determine the economic trajectories over time and across scenarios and, consequently, land use changes. In terms of policies, EPPA uses a 1.5°C increase by the end-of-century with a 50% likelihood given an assessment of uncertainty in climate response to greenhouse gas forcing in the MIT Earth System Model (MESM). Global GHG emissions are driven by regional emissions caps based on the existing NDC commitments by 2030 and proportional historical contributions to GHG concentrations beyond that, assuming differentiated responsibilities across regions. In order to capture the potential contribution and land use competition from large adoption of NBS, in EPPA emissions from land use changes are included under the cap and afforestation/reforestation activities can generate carbon credits toward the climate stabilization goal.
A strength of the EPPA model is its economy-wide representation, which captures economic dynamics across multiple sectors of the economy, driving market-based demand for land. However, as a top-down model, EPPA is limited to aggregated representations of land types and land management/mitigation options. It lacks the level of detail considered in the Sky 2050 scenario, particularly details related to NBS. Therefore, to assess potential future land competition among food production, energy production, and carbon sequestration via NBS, we combine the detailed bottom-up estimates of land uses from the Sky 2050 scenario with the top-down modeling of land use change using the EPPA model.
We do this by overlaying the demanded areas for alternative land uses from Sky 2050 in EPPA and evaluating the consistency between them. More specifically, assuming the same climate stabilization target, we combine the NBS adoption rates and projections of land used for renewable energy sources from Sky 2050 with land use projections from EPPA which account for changes in income, food and energy consumption and prices due to the climate stabilization target and future development of societies. We then verify if the detailed bottom-up Sky 2050 land use requirements are compatible with the top-down EPPA market mediated projections on land demand to achieve the 1.5°C target while also satisfying future demand for food, energy and other land-intensive materials.
4 Results
4.1 Global land use: 1700–2100
Global land use was quite stable among broad land use categories before the Industrial Revolution (Figure 2). In the middle of the nineteenth century, changes in land use from natural vegetation to pasture and cropland accelerated—1.08 Gha of natural forests and natural grassland that existed in 1800 became agricultural areas by 1900, and had risen to 3.41 Gha converted by 2000. The most recent 50 years have experienced declining rates of land use conversion worldwide. As shown in Figure 2, strong decarbonization efforts in the 21st century require a halt of historical deforestation trends and a movement toward forest regrowth and more efficient management of existing pasture and grassland areas. It also requires increasing amounts of land used for renewables (bioenergy, wind and solar). At the same time, even with productivity increases, the total amount of cropland must grow somewhat for food production to keep up with population and economic growth, while the significant potential for pasture productivity increases allows land for pasture to slightly decline while still meeting growing demand for products from livestock. Our results are consistent with the IPCC (2023) summary of 1.5°C scenarios that require substantial action on both land and energy.
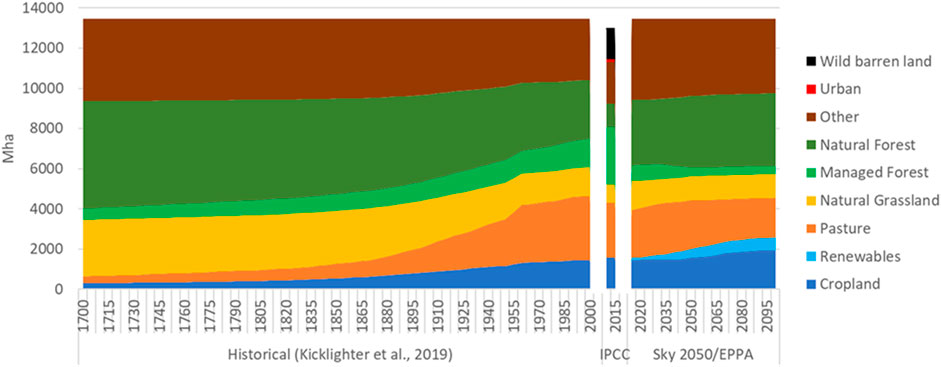
Figure 2. Historical global land use and future projections by broad categories (in Mha). Data source: Kicklighter et al. (2019) for 1700–2000, IPCC (2020) for 2015, EPPA model projections for 2020–2100.
A relevant aspect of the land use change assessment is the fact that different databases use alternative classifications of land use categories, which poses challenges in comparing specific land use types, as can be noticed by the three alternative datasets displayed in Figure 2. Major differences among the datasets are due to land use/land cover categorization and the number of land use types. Kicklighter et al. (2019) land use is based on land use transitions from Hurtt et al. (2011) from the year 1500 to the year 2100. IPCC land use areas rely on the data and approaches described in Lambin and Meyfroidt (2011), Luyssaert et al. (2014), and Erb et al. (2016).
While a consistent alignment between different land datasets remains challenging, in our modeling we combine the data from FAO (2019) and Kicklighter et al. (2019) to disaggregate total forest into natural and managed forest and to split grasslands into pastures and natural grasslands. We map land use categories from alternative databases to the categories used in the EPPA model (see Supplementary Table SA1 in the Supplemental Material) to allow a proper comparison. The total land area in Kicklighter et al. (2019) and EPPA is consistent and equals to 13.46 Gha, while IPCC reports the global ice-free area as 13 Gha. These numbers are close to the global area reported in Figure 1 (13.5 Gha), when glaciers are removed from the overall number. Overall, the general historical trends are similar in direction and relative size across most databases and in our representation.
4.2 Nature-based solutions
Under the decarbonization ambitions of a 1.5°C scenario, there is significant potential for NBS to be deployed at large scale. Figure 3 presents the required annual adoption of NBS projections in the Sky 2050 scenario. The two largest adopted options are related to agricultural areas, such as NBS in cropland (including both biochar and a broad suite of regenerative agricultural practices to reduce emissions and increase soil carbon sequestration) and optimal grazing in pasture areas, which grow fast after 2023, achieve adoption peak rates above 50 Mha per year by 2040, and saturate at the beginning of the 2070s. Natural forest protection also increases, experiencing annual maximum deployment rates of 21 Mha by 2040s and declining thereafter, ceasing completely by 2080. Fire management deployment for grasslands and savannahs expands to 10 Mha by 2040 and keeps this rate of adoption until the end of the century. While this pathway is expressed as 10 Mha/yr, it does not necessitate the enrollment of new area each year; emission reductions can be derived from reducing the intensity/severity of fires in the same area each year. Other relevant NBS practices do not achieve such large rates of adoption, but may accumulate to sizeable amounts by the end of the century, which is the case of reforestation of natural forest areas, achieving 202 Mha, and grassland protection, which covers 246 Mha.
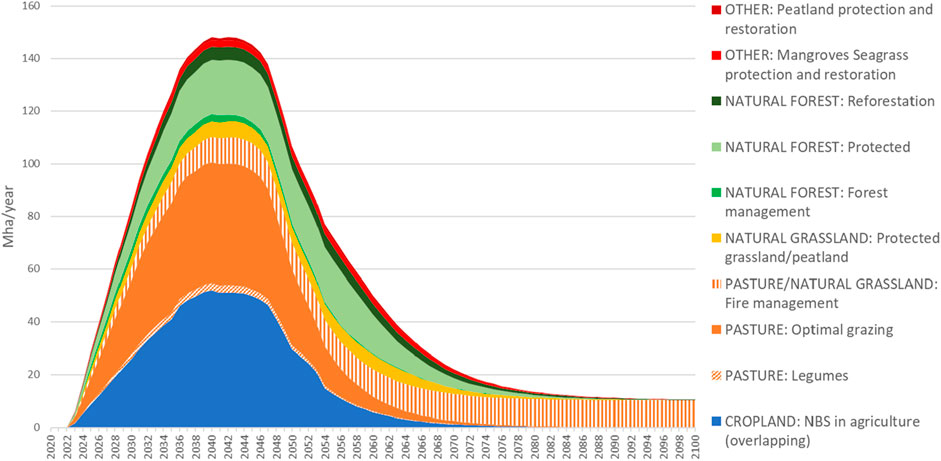
Figure 3. Annual increments in land areas for different categories of nature-based solutions. Source: authors calculations.
As NBS areas increase through the end of the century, they will compete with other land uses, such as food production, bioenergy and other renewable sources. Figure 4 shows how these competing uses can fit in EPPA’s projections of land requirements under 1.5°C stabilization. Adoption of NBS practices in crop production accelerates after 2030 and covers more than 60% of total cropland area by the end of the century. Bioenergy production requires 286 Mha by the end of the century, while land dedicated to solar and onshore wind generation achieves 347 Mha. Natural forest areas grow by 355 Mha from 2015 to 2100, which is larger than the 202 Mha projected reforestation areas in Sky 2050, since in the EPPA model we also represent market incentives, such as carbon offsets, in reforestation projects that leads to a larger increase in natural forest areas. Almost half of the natural forest area is explicitly convening some NBS by 2100, including forest protection and improved management, and only a very small amount is attributed to reforestation. Despite the expansion of NBS, forest protection and restoration, between 2020 and 2100 forestry products per capita are increased by 213%.
Cropland and forest expansion are possible due to a sharp contraction of 13% (−420 Mha) in pasture areas from 2015 to 2100, as well as some loss of natural grassland areas (−282 Mha). Such strong conversion of pasture areas is driven by increasing GHG prices on land use changes and methane-intensive livestock activities, but also due to current low productivity in grazing activities in several developing countries around the world, which makes the intensification of livestock practices relatively cheap when GHG emissions are constrained. The remaining pasture area by 2100 is still large enough to accommodate the NBS projections from Sky 2050, which covers 42% of the total pasture area. Sky 2050’s projection of NBS related to cropland covers 61% of total cropland by the end of the century, and improved grazing practices are adopted by 42% of total grasslands. We allocate several NBS opportunities not directly related to major agricultural and natural land use types represented in EPPA, such as protection and restoration of peatland, mangrove, seagrass and salt marshes, on EPPA’s “other” land use category, since these are quite small areas compared to other NBS options.
Focusing specifically on agriculture, NBS is projected to grow significantly. The 4.7 Gha of land dedicated to agriculture and bioenergy in 2015 is projected to reach 5.0 Gha by 2030, and then stabilize throughout the period to 2100 (Figure 5), according to EPPA projections under a 1.5°C stabilization scenario. The allocation of agricultural land among different uses will be very different by 2100 from today, and will include large areas of NBS. NBS in pasture areas will grow from 127 Mha in 2030 to 1.0 Gha by 2050 and 1.2 Gha by 2100, while NBS in cropland will evolve from 111 to 990 Mha by mid-century and 1.2 Gha by 2100.
Total land managed for NBS by the end of the century is projected to be about 3.5 Gha, of which 0.77 Gha is related to forest, 1.17 Gha to cropland, 1.17 Gha to pasture, 0.26 Gha to grassland and 0.15 Gha to other land types. Globally, there is enough land in each category to accommodate the NBS projections from Sky 2050, while also ensuring growing demand for food and other land-based products is met. In particular, our results show that despite economic and population growth (MIT Joint Program, 2023) and increasing demand for food, NBS does not interfere with the provision of food, and in fact, between 2020 and 2100 the consumption of total nutrients per capita (based on the overall changes in agriculture and food production) are increased by 161%. Still, it is important to note again that this scenario represents an ambitious expansion of NBS, which can present challenges at the regional scale (see Section 4.5). An increase in total nutrients means that supply of some specific nutrients (such as high-calories energy-yielding nutrients) will grow slower than other nutrients (such as vitamins, minerals, dietary fibers and proteins), but that the overall per capita needs are improving over time if a proper food distribution for healthier diets is in place, especially in developing regions of the world.
4.3 Land for energy
Table 2 shows the projected land areas used for bioenergy, onshore wind and solar power generation in 2050 for the selected regions in the Sky 2050 scenario. Table 3 provides the corresponding information for 2100. Global land area dedicated to bioenergy is projected to more than double by mid-century. It grows from about 100 Mha in 2020 (see Table 1) to 242 Mha in 2050 and 286 Mha by 2100. Dedicated biomass growing areas enable growing bioenergy consumption. The total commercial bioenergy use (i.e., including first-generation biofuels, second-generation biofuels and commercial solid biomass, but excluding traditional bioenergy used for heating and cooking) grows from about 20 EJ in 2020 to about 50 EJ in 2050, and about 70 EJ in 2100. We consider this as a conservative projection for bioenergy demand growth, because some alternative scenarios project a larger role for bioenergy with carbon capture (IPCC, 2018; Fajardy et al., 2021). In the Sky 2050 scenario, we take into account sustainability criteria preventing unintended consequences on global food security and environmental quality.
Land used for wind and solar generation grows much faster because of accelerated deployment of these sources of energy in a decarbonized world. In 2020, the global areas for wind and solar generation were 8 Mha and 0.6 Mha, respectively. By 2050, they are projected to grow to 180 Mha and 21 Mha. By the end of the century, the areas grow further to about 300 Mha for wind power and to 45 Mha for solar power. As shown in Tables 2, 3, total energy-dedicated land areas are reaching 7%–9% of the total land areas in the United States, China and India. Globally, land for energy is increasing from less than 1% of total land area in 2020, to about 3% in 2050, and to about 5% in 2100 (about 0.6 Gha). To achieve this scale of transition would require regulatory incentives from policy makers that not only bring the substantial investments needed to scale up low-carbon energy sources, but also address the social and environmental justice issues related to the associated land implications.
The amount of renewable energy in the Sky 2050 scenario is consistent with similar scenarios in other energy outlooks. For example, the International Energy Agency in its net-zero scenario (IEA, 2023) projects that in 2050 the share of wind and solar in global primary energy will be 41% and the corresponding share for bioenergy is 18%. The Sky 2050 scenario is slightly more optimistic about wind and solar generation with their 2050 combined share of 43%, and slightly less optimistic about bioenergy with its share at about 10% of total primary energy. The differences in bioenergy are mostly related to different assumptions about traditional biomass and solid commercial biomass.
4.4 CO2 sequestration
Figure 6 presents the CO2 sequestration contributions from NBS deployed in major land use types in the Sky 2050 scenario. The correspondence between land use types and NBS categories for CO2 sequestration is provided in Supplementary Table SA2 of the Supplemental Material. Forest NBS opportunities provide the largest contribution and can reach more than 3.7 GtCO2/year by mid-century, but decline thereafter due to several reforested areas approaching or reaching a maturation age at which point the net sequestration of carbon on living biomass is small. NBS in agricultural activities is the second largest CO2 sink and may sequester around 2 GtCO2/year for several decades. NBS in Grassland contributes to sequestering at most 1 GtCO2/year by mid-century.
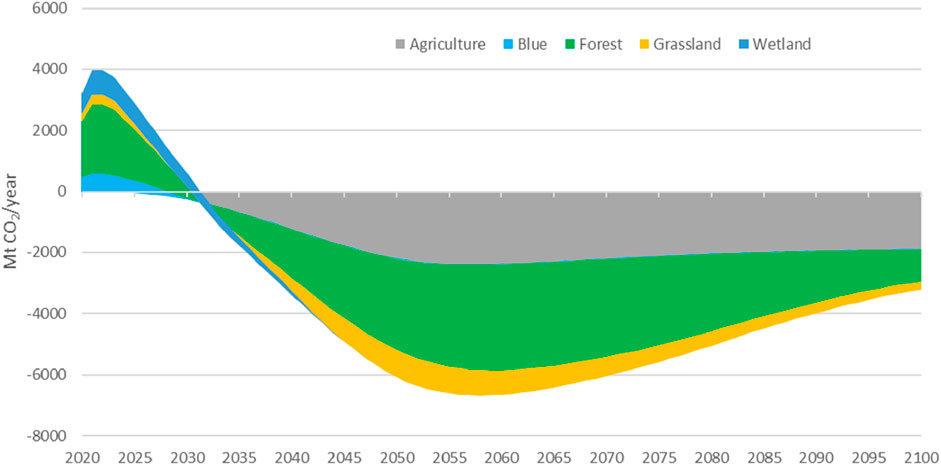
Figure 6. Annual carbon emissions and sequestration from different NBS types (agriculture, blue, wetland, grassland, forest). Source: authors calculations.
Among the NBS options in agriculture areas (Figure 7), biochar provides the largest sequestration from a single activity and is the only one where emission reductions are continuing to increase at the end of the century. Other options, such as optimal grazing practices, legumes in pastures, regenerative arable agriculture, and agroforestry may contribute with substantial CO2 removals at mid-century, but as carbon in soils reach a saturation level and adopted areas stabilize around equilibrium carbon stocks approaching the end of the century, their contribution to sequester additional CO2 reduces.
For biochar, there is uncertainty in the literature about application rates, the effects of repeated application and long terms stability (e.g., Jeffery et al., 2017; Tisserant and Cherubini, 2019; Lehmann et al., 2021; Woolf et al., 2021). In our study, we assume that biochar is applied to soils or sediments and no additional impact of the biochar on plant growth or “additional” soil carbon sequestration is factored in. The biochar is applied to land at appropriate rates and in appropriate contexts to ensure negligible effects on existing carbon cycling in that site/ecosystem. It should be noted that we aim to be conservative for the overall NBS estimates and we only include pathways that have more robust scientific evidence. Some researchers are working on other pathways (such as improved aquaculture, enhanced rock weathering, permafrost protection, advanced rice management, advanced manure management and change in cattle diets, etc.), and some of these may well come to fruition. This would potentially increase our overall NBS estimates.
4.5 Regional land-use changes
Land use at the global level can accommodate the multiple demands expected under a 1.5°C climate stabilization scenario. At the regional level, however, challenges to integrate all land uses may arise. We explore such potential challenges by considering land use allocation in a set of selected major countries and regions in the world (Figure 8).
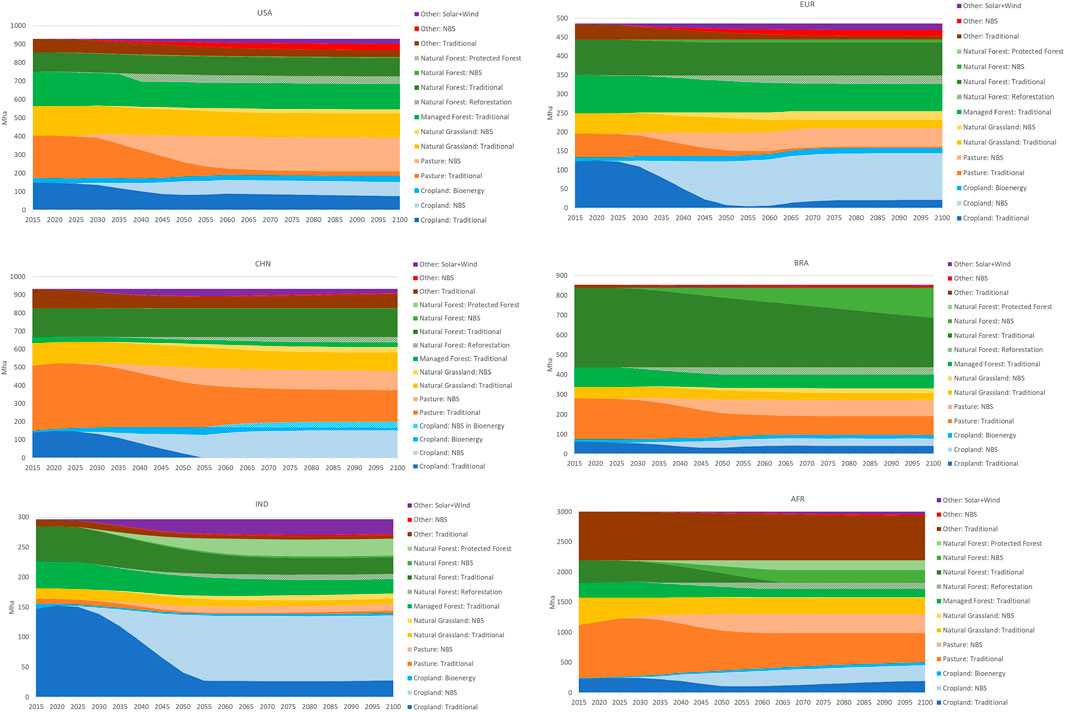
Figure 8. Land use evolution (2015–2100) in selected regions (United States, Europe, China, India, Brazil, Africa). Source: authors calculations.
In the United States, NBS related to livestock activities occupies 88% of total available pasture land by 2100. Almost half of the other land use category is needed to accommodate other types of NBS and solar energy, while we assume onshore-wind is placed on cropland areas. In the case of Europe, the placement of wind, solar and some NBS on Other land is even more challenging, requiring 74% of it, which means a possible competition with urban and infrastructure areas. NBS in cropland and pastures also may raise concerns, since only 16% and 12% of these land categories, respectively, are not adopting some kind of NBS by 2100.
In emerging economies, we observe mixed outcomes. In China, the amount of required NBS in crops to achieve 1.5°C needs to cover the total cropland available plus most of the area dedicated to bioenergy crops. India faces several land use competition issues: wind, solar and some NBS will require 80% of the Other land category by 2100, around 70% of the already limited pasture areas need to adopt some NBS, and 73% of the 150 Mha of cropland will be required to produce crops using NBS approaches.
Among the major food and bioenergy producers in the world, only Brazil seems to face moderate pressures on land use constraints due to NBS adoption in agriculture and major natural land use categories. However, the country has a very small area of the Other land use category, and the deployment of solar, wind and other NBS types will require the equivalent of 65% of this area, which poses questions about feasibility and competition with urban and infrastructure uses, which may be alleviated if wind, solar and other NBS are placed elsewhere.
Figure 9 provides a snapshot for the shares of land in a particular use in 2050 for the selected major regions and the globe. The figure shows the shares for traditional practices, land for energy, NBS, and proactive land management, such as restoration and protection. Our projections for 2050 illustrate a substantial deployment of advanced land practices, especially for NBS in cropland in Europe and India, NBS in pasture in United States, Europe, China, Brazil, and Africa, and NBS in natural forests in Brazil. At the same time, some regions still rely heavily on traditional land uses, such as pasture in China and Africa, and natural grassland and natural forests in most of the regions.
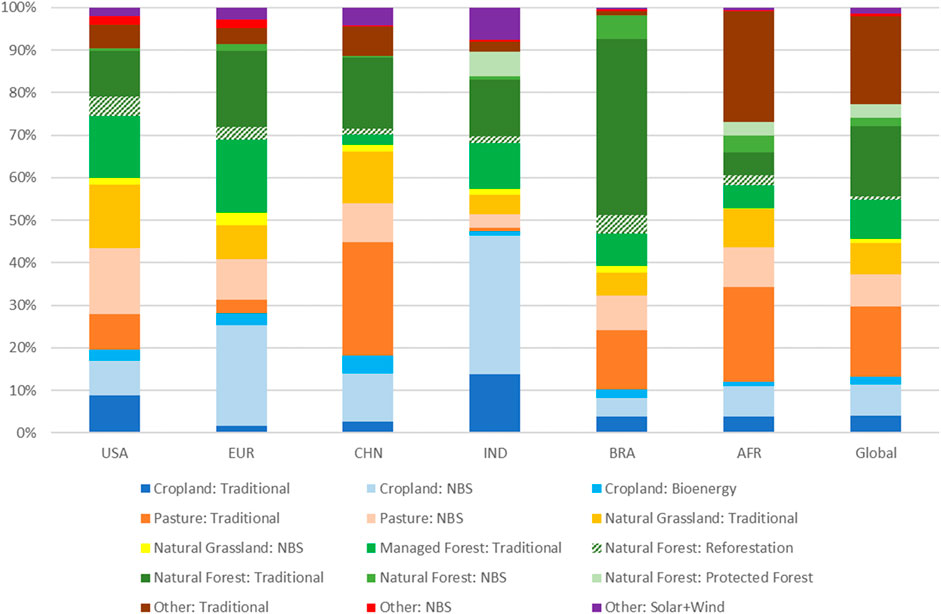
Figure 9. Comparison of 2050 land use shares (%) in selected regions (United States, Europe, China, India, Brazil, Africa, and The World). Source: authors calculations.
5 Discussion
Achieving the climate stabilization goal of 1.5°C by the end of the century will require transformative societal changes in policy, consumption patterns, and land management practices, including a reversal of current trends on land use changes and a reconfiguration of land cover distribution and agricultural activities. There are many opportunities to mitigate emissions and sequester carbon in soils through nature-based solutions (such as agroforestry or soil carbon sequestration), but also, many challenges to accommodate such practices in synergistic ways with food provision and renewable energy requirements. We assessed the potential competition and complementarity of land use demands among NBS practices, agricultural production and renewable energy by combining a detailed bottom-up scenario of future deployment of NBS and renewables to achieve the 1.5°C stabilization goal with a global integrated assessment model projecting the economics of land use changes and overall GHG mitigation to achieve the same climate goal.
We show that the global land system can accommodate the 3.5 Gha of NBS projected, which helps to remove about 6 GtCO2/year. However, we identify several perceived trade-offs related to land use competition. First, there are concerns that NBS activities may affect agricultural output and food production. Our modelling shows that ambitious NBS deployment does not need to threaten the provision of food, with results showing a 161% increase in nutrients per capita between 2020 and 2100 despite the deployment of NBS. This is in line with the consensus that effective adoption of regenerative agricultural practices does not negatively impact yields, and typically increases nutrient cycling efficiencies (Van Balen et al., 2023; Tonitto et al., 2006; Allam et al., 2023).
The second potential concern is related to land required for energy production (primarily for wind, solar, and biomass) and its competition with the land for food and preserving nature. The Sky 2050 scenario was deliberately designed to limit bioenergy demand to levels well below published potential resource base estimates. We show that land for bioenergy and renewable energy takes only about 3%–5% of total land even under aggressive assumptions about solar and wind energy deployment. Another concern is related to forest products, in particular to the trade-offs between timber production (that can lead to such outcomes as destruction of forest cover, loss of biodiversity, soil erosion, ecological imbalance and other negative impacts) and forest protection and reforestation. Our results show that between 2020 and 2100 forestry products per capita are increased by 213%, while we also represent NBS, forest protection and restoration.
A trade-off with biodiversity has been gaining substantial attention recently (Environmental Defense Fund, 2024), as well as impacts on water availability (Schlosser et al., 2014; MIT Joint Program, 2023). While in our current study we do not capture the impacts on biodiversity and water, we fully support the need for further examination of the issue. A principle of the Sky 2050 scenario is that the NBS is not just optimizing for CO2, but taking a more holistic view of appropriate ecosystem restoration, of which just one of the benefits is increasing the land stock of CO2. However, putting the regulations in place to deliver this is far from straightforward. In particular, there is a need for systematic biodiversity indicators (Rouge and Schlosser, 2023) that would help to understand and predict the fate of global biodiversity amidst an increasingly complex and changing world. Among the objectives is the ability to construct a comprehensive metric that not only quantifies the current state of biodiversity, but also captures future trends that are driven by a variety of stressors across environmental, social, and economic systems.
In this study we focus on nature-based solutions rather than on engineering carbon capture solutions, such as bioenergy with carbon capture and storage (BECCS) or direct air CO2 capture and storage (DACCS). An additional trade-off with engineered carbon dioxide removal (CDR) options would put additional pressure on land, however, these options should be deployed as an addition to the efforts for emission mitigation. While the cost of these engineered CDR options appears to be higher than most NBS, they could compete with NBS for land in the future, depending on how their costs evolve, the rate of carbon sequestration per ha they can achieve, demand for negative emissions and policies around using offsets, particularly international offsets.
While we share concerns (e.g., Searchinger and Heimlich, 2015; Rulli et al., 2016; Fehrenbach et al., 2023) about the urgent need for advancing sustainable approaches to land management for food and nature, our results suggest that, at global level, in the second half of the 21st century land is available to provide 3-6 GtCO2/year sink through 2.5–3.5 Gha of NBS practices of protecting, managing, and restoring land, and, at same time, providing 325–650 EJ/year of renewable energy, using 0.4–0.6 Gha of land, including 0.2–0.3 Gha for bioenergy and 0.2–0.35 Gha for wind and solar power generation. Our regional exploration of land competition between different uses suggests that it is possible to fit the land for major human needs, while protecting and restoring land. These ranges of NBS deployment over time would not disturb food and primary goods production from agriculture more than the multiple economic pressures which are associated with a 1.5°C stabilization target, such as changes in energy and good prices and impacts on income and overall demand.
However, to achieve such an outcome, NBS practices in agriculture need to be massively adopted by farmers, mostly by mid-century, and in such a way that will maintain observed trends in crop yield improvements. In the case of the livestock sector, pasture yields and productivity gains must accelerate, at the same time as NBS is adopted, in order to free up land for other uses, such as the regrowth of forest areas. Such deployment of NBS requires engagement of local communities, especially in places where the majority of land is owned by small landholders. Special attention needs to be paid to protection of indigenous communities, whose rights to the land they occupy are often not formally recognized (Waring et al., 2023). In addition to engagement and communication, adoption of NBS will likely also require incentives, which can have different implications for equity depending on how those incentives are designed.
In the face of competition for relatively limited “cheap and easy” land for various options, it will be crucial to have the right policies and incentives in place to ensure that the land is used in ways that is best for both human and environmental goals. It will be crucial to ensure that policies that encourage certain land uses (such as for carbon sequestration or energy) do not negatively impact land uses needed to meet other societal goals, such as food security to biodiversity. It is also important to understand that future competition between the different land uses critically depends on a number of factors, including economic and population growth, land productivity, crop value, and how it may be impacted by changing climate, land and technology costs, and policy design and stringency.
For NBS to contribute to achieving climate targets, policies are needed to appropriately price land use emissions/sequestration and/or require certain practices. This can be achieved by covering the land sector under an emissions policy, by creating separate offset markets for sequestered carbon, or other regulatory policies such as NBS requirements or targets for carbon removal. Regardless of the policy approach for NBS, careful monitoring, accounting and crediting of emissions is needed, which requires agreement on important questions related to permanence, additionality and leakage. We can learn from existing voluntary offset markets as well as policies being implemented in various countries.
While our analysis is intended to provide the “big picture” regarding land competition in a world that is developing in increasingly sustainable directions across both land and energy systems and as such many aspects of energy and agricultural operations are beyond the scope of our modeling (and therefore the exact numerical values should be treated with a great degree of caution), several recommendations can be offered based on our assessment. The novelty of our study is in providing a clear message that it is possible to fit the land for major human needs, while protecting and restoring land. Our study shows the feasibility of achieving the land-use optimization needed for a climate stabilization scenario. With all inherent uncertainty about the potential cost reductions for existing technologies and deployment of new regulatory and technological options, one message is clear: there is an urgent need for advancing sustainable land management for food and nature.
We also stress the crucial importance of detailed regional and local evaluations of the pathways for achieving sustainability. Government authorities and industry participants should encourage these studies and involve local and international experts. Such studies will provide a valuable framework for understanding the challenges and opportunities ahead, guiding the world toward a sustainable future.
Data availability statement
The original contributions presented in the study are included in the article/Supplementary Material, further inquiries can be directed to the corresponding author.
Author contributions
AG: Conceptualization, Formal Analysis, Investigation, Methodology, Software, Writing–original draft, Writing–review and editing. JM: Data curation, Investigation, Validation, Writing–original draft, Writing–review and editing. MH: Conceptualization, Data curation, Formal Analysis, Methodology, Validation, Writing–original draft, Writing–review and editing. AR: Data curation, Formal Analysis, Investigation, Methodology, Writing–original draft, Writing–review and editing. RP: Data curation, Investigation, Writing–original draft, Writing–review and editing. SP: Conceptualization, Data curation, Formal Analysis, Investigation, Methodology, Supervision, Writing–original draft, Writing–review and editing.
Funding
The author(s) declare that no financial support was received for the research, authorship, and/or publication of this article.
Acknowledgments
The authors gratefully acknowledge David Hone and Solene Chiquier for their valuable inputs to the analysis. Development of the EPPA-IGSM framework used in the analysis is supported by an international consortium of government, industry and foundation sponsors of the MIT Center for Sustainability Science and Strategy. For a complete list, see: https://cs3.mit.edu/sponsors/current. Whilst Shell has provided no direct financial support to this research, Shell does support the MIT Center for Sustainability Science and Strategy (formerly the MIT Joint Program on the Science and Policy of Global Change) with an annual sponsorship and occasional supplementary donations. The last supplementary donation was in 2021 to defray costs exploring issues around scenarios and land-use implications. Shell participated actively in this study, supplying the background data behind their scenarios. MIT remains responsible for all analysis and conclusions.
Conflict of interest
Author MH was employed by Shell International. Author AR was employed by Shell International Exploration and Production B. V. Author RP was employed by Shell Global Solutions International B.V.
The remaining authors declare that the research was conducted in the absence of any commercial or financial relationships that could be construed as a potential conflict of interest.
Publisher’s note
All claims expressed in this article are solely those of the authors and do not necessarily represent those of their affiliated organizations, or those of the publisher, the editors and the reviewers. Any product that may be evaluated in this article, or claim that may be made by its manufacturer, is not guaranteed or endorsed by the publisher.
Supplementary material
The Supplementary Material for this article can be found online at: https://www.frontiersin.org/articles/10.3389/fenvs.2024.1393327/full#supplementary-material
Footnotes
11 square kilometer (sq km) = 100 hectares (ha).
References
Allam, M., Radicetti, E., Ben Hassine, M., Jamal, A., Abideen, Z., and Mancinelli, R. (2023). A meta-analysis approach to estimate the effect of cover crops on the grain yield of succeeding cereal crops within European cropping systems. Agriculture 13 (9), 1714. doi:10.3390/agriculture13091714
Barros, F., Lewis, K., Robertson, A., Pennington, R. T., Hill, T., Matthews, C., et al. (2023). Cost-effective restoration for carbon sequestration across Brazil’s biomes. Sci. Total Environ. 876, 162600. doi:10.1016/j.scitotenv.2023.162600
Bastin, J., Finegold, Y., Garcia, C., Mollicone, D., Rezende, M., Routh, D., et al. (2019). The global tree restoration potential. Science 365 (6448), 76–79. doi:10.1126/science.aax0848
BloombergNEF (2022). New energy Outlook. Available at: https://about.bnef.com/new-energy-outlook/.
Boysen, L., Lucht, W., and Gerten, D. (2017). Trade-offs for food production, nature conservation and climate limit the terrestrial carbon dioxide removal potential. Glob. Change Biol. 23 (10), 4303–4317. doi:10.1111/gcb.13745
Busch, J., Engelmann, J., Cook-Patton, S., Griscom, B., Kroeger, T., Possingham, H., et al. (2019). Potential for low-cost carbon dioxide removal through tropical reforestation. Nat. Clim. Change 9, 463–466. doi:10.1038/s41558-019-0485-x
Campbell, J., Lobell, D., Genova, R., and Field, C. (2008). The global potential of bioenergy on abandoned agriculture lands. Environ. Sci. Technol. 42, 5791–5794. doi:10.1021/es800052w
Chen, Y., Paltsev, S., Gurgel, A., Reilly, J., and Morris, J. (2022). A multisectoral dynamic model for energy, economic, and climate scenario analysis. Low Carbon Economy 13, 70–111. doi:10.4236/lce.2022.132005
Committee on Climate Change (2020). Land use: policies for a net zero UK. Available at: https://www.theccc.org.uk/publication/land-use-policies-for-a-net-zero-uk/.
Cook-Patton, S., Leavitt, S., Gibbs, D., Harris, N. L., Lister, K., Anderson-Teixeira, K. J., et al. (2020). Mapping carbon accumulation potential from global natural forest regrowth. Nature 585, 545–550. doi:10.1038/s41586-020-2686-x
Donnison, C., Holland, R. A., Hastings, A., Armstrong, L. M., Eigenbrod, F., and Taylor, G. (2020). Bioenergy with Carbon Capture and Storage (BECCS): finding the win–wins for energy, negative emissions and ecosystem services—size matters. GCB Bioenergy 12 (8), 586–604. doi:10.1111/gcbb.12695
Environmental Defense Fund (2024). Natural climate solutions crediting handbook. Available at: https://www.edf.org/natural-climate-solutions/handbook.
Erb, K.-H., Lauk, C., Kastner, T., Mayer, A., Theurl, M., and Haberl, H. (2016). Exploring the biophysical option space for feeding the world without deforestation. Nat. Commun. 7, 11382. doi:10.1038/ncomms11382
Fajardy, M., Morris, J., Gurgel, A., Herzog, H., Mac Dowell, N., and Paltsev, S. (2021). The economics of bioenergy with carbon capture and storage (BECCS) deployment in a 1.5°C or 2°C world. Glob. Environ. Change 68, 102262. doi:10.1016/j.gloenvcha.2021.102262
FAO (2018). The future of food and agriculture – alternative pathways to 2050. Rome, Italy: Food and Agricultural Organization of the United Nations. Available at: https://www.fao.org/global-perspectives-studies/resources/detail/en/c/1157074/.
FAO (2019). Land use. Available at: https://www.fao.org/faostat/en/#data/RL.
FAO (2023). Food balances. Available at: https://www.fao.org/faostat/en/#data/FBS.
Fehrenbach, H., Burck, S., and Wehrle, A. (2023). The carbon and food opportunity costs of biofuels in the EU27 plus UK. Institute for Energy – Heidelberg. Available at: https://www.transportenvironment.org/wp-content/uploads/2023/03/ifeu-study-COC-biofuels-EU_for-TE-2023-03-02_clean.pdf.
Griscom, B., Adams, J., Ellis, P., Houghton, R., Lomax, G., Miteva, D. A., et al. (2017). Natural climate solutions. Proc. Natl. Acad. Sci. U. S. A. 114 (44), 11645–11650. doi:10.1073/pnas.1710465114
Gurgel, A., Chen, Y.-H. H., Paltsev, S., and Reilly, J. (2016). “CGE models: linking natural resources to the CGE framework,” in The WSPC reference on natural resources and environmental policy in the era of global change: v. 3 – computable general equilibrium models of society. Editors A. Dinar,, and T. Briant (New Jersey: World Scientific Publishing), 57–98.
Gurgel, A., Reilly, J., and Blanc, E. (2021). Challenges in simulating economic effects of climate change on global agricultural markets. Clim. Change 166, 29. doi:10.1007/s10584-021-03119-8
Hasegawa, T., Fujimori, S., Frank, S., Humpenöder, F., Bertram, C., Després, J., et al. (2021). Land-based implications of early climate actions without global net-negative emissions. Nat. Sustain. 4 (12), 1052–1059. doi:10.1038/s41893-021-00772-w
Hasegawa, T., Wakatsuki, H., Ju, H., Vyas, S., Nelson, G. C., Farrell, A., et al. (2022). A global dataset for the projected impacts of climate change on four major crops. Sci. Data 9 (1), 58. doi:10.1038/s41597-022-01150-7
Hsiang, S., Kopp, R., Jina, A., Rising, J., Delgado, M., Mohan, S., et al. (2017). Estimating economic damage from climate change in the United States. Science 356 (6345), 1362–1369. doi:10.1126/science.aal4369
Hurtt, G., Chini, L., Frolking, S., Betts, R., Feddema, J., Fischer, G., et al. (2011). Harmonization of land-use scenarios for the period 1500–2100: 600 years of global gridded annual land-use transitions, wood harvest, and resulting secondary lands. Climatic Change 109, 117. doi:10.1007/s10584-011-0153-2
IEA (International Energy Agency) (2023). World energy Outlook. Available at: https://www.iea.org/reports/world-energy-outlook-2023.
IPCC (Intergovernmental Panel on Climate Change) (2018). Special report. Glob. Warming. Available at: https://www.ipcc.ch/sr15/.
IPCC (Intergovernmental Panel on Climate Change) (2020). Special report. Clim. Change Land. Available at: https://www.ipcc.ch/srccl/.
IPCC (Intergovernmental Panel on Climate Change) (2023). AR6 synthesis report: climate change 2023. Available at: https://www.ipcc.ch/report/ar6/syr/.
Jeffery, S., Abalos, D., Prodana, M., Bastos, A., Groenigen, J., Hungate, B., et al. (2017). Biochar boosts tropical but not temperate crop yields. Environ. Res. Lett. 12, 053001. doi:10.1088/1748-9326/aa67bd
Kicklighter, D. W., Melillo, J. M., Monier, E., Sokolov, A. P., and Zhuang, Q. (2019). Future nitrogen availability and its effect on carbon sequestration in Northern Eurasia. Nat. Commun. 10 (1), 1–19. doi:10.1038/s41467-019-10944-0
Lambin, E., and Meyfroidt, P. (2011). Global land use change, economic globalization, and the looming land scarcity. PNAS 108 (9), 3465–3472. doi:10.1073/pnas.1100480108
Lehmann, J., Cowie, A., Masiello, C., Kammann, C., Woolf, D., Amonette, J., et al. (2021). Biochar in climate change mitigation. Nat. Geosci. 14, 883–892. doi:10.1038/s41561-021-00852-8
Luyssaert, S., Jammet, M., Stoy, P., Estel, S., Pongratz, J., Ceschia, E., et al. (2014). Land management and land-cover change have impacts of similar magnitude on surface temperature. Nat. Clim. Change 4 (5), 389–393. doi:10.1038/nclimate2196
MIT Joint Program (2023). Glob. Change Outlook. Available at: https://globalchange.mit.edu/news-media/jp-news-outreach/accelerated-climate-action-needed-sharply-reduce-current-risks-life-and.
Morris, J., Chen, H., Gurgel, A., Reilly, J., and Sokolov, A. (2023). Net zero emissions of greenhouse gases by 2050: achievable and at what cost?. Clim. Change Econ. 14 (4), 2340002. doi:10.1142/s201000782340002x
Morris, J., Gurgel, A., Mignone, B., Kheshgi, H., and Paltsev, S. (2024). Mutual reinforcement of land-based carbon dioxide removal and international emissions trading in deep decarbonization scenarios. Nat. Commun. 15, 7160. doi:10.1038/s41467-024-49502-8
NREL (2019). Golden, CO: National Renewable Energy Laboratory. Available at: https://www.energy.gov/sites/default/files/2019/05/f63/gagne-rule-thumb-ppt.pdf.
Paltsev, S., Reilly, J., Jacoby, H., Eckaus, R., McFarland, J., Sarofim, M., et al. (2005). The MIT emissions prediction and policy analysis (EPPA) model: version 4. MIT Joint program report 125. Available at: https://globalchange.mit.edu/publication/14578.
Rezaei, E. E., Webber, H., Asseng, S., Boote, K., Durand, J. L., Ewert, F., et al. (2023). Climate change impacts on crop yields. Nat. Rev. Earth & Environ. 4 (12), 831–846. doi:10.1038/s43017-023-00491-0
Ritchie, H., and Roser, M. (2013). Land use. Available at: https://ourworldindata.org/land-use.
Robertson, A., Zhang, Y., Sherrod, L., Rosenzweig, S., Ma, L., Ahuja, L., et al. (2018). Climate change impacts on yields and soil carbon in row crop dryland agriculture. J. Environ. Qual. 47, 684–694. doi:10.2134/jeq2017.08.0309
Roe, S., Streck, C., Beach, R., Busch, J., Chapman, M., Daioglou, V., et al. (2021). Land-based measures to mitigate climate change: potential and feasibility by country. Glob. Change Biol. 27 (23), 6025–6058. doi:10.1111/gcb.15873
Roe, S., Streck, C., Obersteiner, M., Frank, S., Griscom, B., Drouet, L., et al. (2019). Contribution of the land sector to a 1.5 °C world. Nat. Clim. Change 9 (11), 817–828. doi:10.1038/s41558-019-0591-9
Rouge, K., and Schlosser, A. (2023). Building a composite indicator for biodiversity through supervised learning and linked indicator sets. Cambridge, MA: MIT Joint Program on the Science and Policy of Global Change. Available at: http://globalchange.mit.edu/publication/17984.
Ruehr, S., Keenan, T. F., Williams, C., Zhou, Y., Lu, X., Bastos, A., et al. (2023). Evidence and attribution of the enhanced land carbon sink. Nat. Rev. Earth & Environ. 4 (8), 518–534. doi:10.1038/s43017-023-00456-3
Rulli, M., Bellomi, D., Cazzoli, A., De Carolis, G., and D’Odorico, P. (2016). The water-land-food nexus of first-generation biofuels. Sci. Rep. 6, 22521. doi:10.1038/srep22521
Schlosser, A., Strzepek, K., Gao, X., Fant, C., Blanc, E., Paltsev, S., et al. (2014). The future of global water stress: an integrated assessment. Earth's Future 2 (8), 341–361. doi:10.1002/2014EF000238
Searchinger, T., and Heimlich, R. (2015). Avoiding bioenergy competition for food crops and land. Washington, DC: World Resources Institute. Available at: https://www.wri.org/research/avoiding-bioenergy-competition-food-crops-and-land.
Seddon, N., Chausson, A., Berry, P., Girardin, C. A., Smith, A., and Turner, B. (2020). Understanding the value and limits of nature-based solutions to climate change and other global challenges. Philosophical Trans. R. Soc. B 375 (1794), 20190120. doi:10.1098/rstb.2019.0120
Seddon, N., Smith, A., Smith, P., Key, I., Chausson, A., Girardin, C., et al. (2021). Getting the message right on nature-based solutions to climate change. Glob. Change Biol. 27 (8), 1518–1546. doi:10.1111/gcb.15513
Shell (2023). The energy security scenarios. Available at: https://www.shell.com/energy-and-innovation/the-energy-future/scenarios/the-energy-security-scenarios.html.
Smith, P., Davis, S. J., Creutzig, F., Fuss, S., Minx, J., Gabrielle, B., et al. (2016). Biophysical and economic limits to negative CO2 emissions. Nat. Clim. Change 6 (1), 42–50. doi:10.1038/nclimate2870
Sokolov, A., Kicklighter, D., Schlosser, A., Wang, C., Monier, E., Brown-Steiner, B., et al. (2018). Description and evaluation of the MIT Earth system model (MESM). AGU J. Adv. Model. Earth Syst. 10 (8), 1759–1789. doi:10.1029/2018ms001277
Sokolov, A., Paltsev, S., Gurgel, A., Haigh, M., Hone, D., and Morris, J. (2023) Temperature implications of the 2023 Shell energy security scenarios: Sky 2050 and archipelagos. Cambridge, MA: MIT Joint Program Report. Available at: http://globalchange.mit.edu/publication/17983.
Sue Wing, I., De Cian, E., and Mistry, M. N. (2021). Global vulnerability of crop yields to climate change. J. Environ. Econ. Manag., 109, 102462. doi:10.1016/j.jeem.2021.102462
Tisserant, A., and Cherubini, F. (2019). Potentials, limitations, co-benefits, and trade-offs of biochar applications to soils for climate change mitigation. Land, 8 (12), 179. doi:10.3390/land8120179
Tonitto, C., David, M., and Drinkwater, L. (2006). Replacing bare fallows with cover crops in fertilizer-intensive cropping systems: a meta-analysis of crop yield and N dynamics. Agric. Ecosyst. Environ. 112 (1), 58–72. doi:10.1016/j.agee.2005.07.003
UN (United Nations) (2015). The Paris Agreement. Available at: https://unfccc.int/process-and-meetings/the-paris-agreement.
Van Balen, D., Cuperus, F., Haagsma, W., De Haan, J., Van Den Berg, W., and Sukkel, W. (2023). Crop yield response to long-term reduced tillage in a conventional and organic farming system on a sandy loam soil. Soil Tillage Res. 225, 105553. doi:10.1016/j.still.2022.105553
Van de Ven, D., Capellan-Peréz, I., Arto, I., Cazcarro, I., de Castro, C., Patel, P., et al. (2021). The potential land requirements and related land use change emissions of solar energy. Sci. Rep. 11 (1), 2907. doi:10.1038/s41598-021-82042-5
Vera, I., Wicke, B., Lamers, P., Cowie, A., Repo, A., Heukels, B., et al. (2022). Land use for bioenergy: synergies and trade-offs between sustainable development goals. Renew. Sustain. Energy Rev. 161, 112409. doi:10.1016/j.rser.2022.112409
Waring, B., Gurgel, A., Koberle, A., Paltsev, S., and Rogelj, J. (2023). Natural Climate Solutions must embrace multiple perspectives to ensure synergy with sustainable development. Front. Clim. 5, 1216175. doi:10.3389/fclim.2023.1216175
Winchester, N., and Reilly, J. (2015). The feasibility, costs, and environmental implications of large-scale biomass energy. Energy Econ. 51, 188–203. doi:10.1016/j.eneco.2015.06.016
Keywords: land use, decarbonization, sustainability, climate, greenhouse gas, nature-based solutions, carbon removals
Citation: Gurgel A, Morris J, Haigh M, Robertson AD, van der Ploeg R and Paltsev S (2024) Land-use competition in 1.5°C climate stabilization: is there enough land for all potential needs?. Front. Environ. Sci. 12:1393327. doi: 10.3389/fenvs.2024.1393327
Received: 28 February 2024; Accepted: 28 October 2024;
Published: 12 November 2024.
Edited by:
Chenxi Li, Xi’an University of Architecture and Technology, ChinaReviewed by:
Maurizio Tiepolo, Polytechnic University of Turin, ItalyEve Bohnett, University of Florida, United States
Copyright © 2024 Gurgel, Morris, Haigh, Robertson, van der Ploeg and Paltsev. This is an open-access article distributed under the terms of the Creative Commons Attribution License (CC BY). The use, distribution or reproduction in other forums is permitted, provided the original author(s) and the copyright owner(s) are credited and that the original publication in this journal is cited, in accordance with accepted academic practice. No use, distribution or reproduction is permitted which does not comply with these terms.
*Correspondence: Sergey Paltsev, cGFsdHNldkBtaXQuZWR1