- 1Department of Biochemistry and Biotechnology, Sardar Bhagwan Singh University, Dehradun, India
- 2Department of Civil Engineering, Yeungnam University, North Gyeongsang, Republic of Korea
- 3Agency for Consultation and Research in Oceanography, La Roche Canillac, France
Introduction
Most regions of the World are experiencing progressive degradation of their water and land resources, and the growing amount of organic waste produced globally has become a concern for public health. Furthermore, improper handling of these organic wastes can cause them to auto-degrade, which can result in the unintentional release of hazardous by-products, including greenhouse gases (GHG), carbon dioxide, and methane, into the atmosphere (Cayetano et al., 2022). Over the last 60 years, as a result of these anthropogenic emissions, the ocean has undergone major changes (Rhein et al., 2013; Gattuso et al., 2015; Venegas et al., 2023)The most noticeable changes are the global dispersion of man-made pollutants, the warming of the oceans, and rising sea levels (Venegas et al., 2023). To avoid ecological and socio-economic catastrophes, it is necessary to consider technological approaches to resolve this issue.
Microbial communities, which feed on autochthonous organic matter (algal detritus and exudates) and allochthonous organic matter (dissolved and particulate plant and animal components) recycle carbon in rivers (Engel et al., 2017). Therefore, dissolved organic waste quality and quantity determine microbial community composition and functionality and the types of biofilms they produce.
Biofilm formation partly prevents microbial wash-out by enabling solid-liquid separability. Because both natural and human-induced biofilms are generally more productive than any comparable planktonic community (Philipp et al., 2023), they are highly significant in any habitat they occupy. Therefore, they can extend the solids retention period, making high-rate treatment possible in systems with a short retention time.
A study by Wurl et al. (2016) described ocean slicks as wave-damped patches at the sea surface resulting from surface-active organic material accumulation. They discovered that in slicks as opposed to the underlying bulk water at several sites in the North Pacific, South China Sea, and Baltic Sea, there were up to 40 times more transparent exopolymer particles (TEP), the building blocks of all biofilm. They concluded that slicks with an excessive build-up of particles and bacteria may have characteristics similar to those of biofilms. Additionally, they calculated that slicks can lower CO2 fluxes by up to 15%, meaning they regulate air-sea interactions on a local and regional scale.
A related study conducted by Mustaffa et al. (2020) quantifies the impact of slicks and natural surfactants on the In situ gas transfer velocity (k) of CO2. A floating chamber-equipped drifting buoy was used to assess the In situ concentration of CO2, while the catamaran Sea Surface Scanner (S3) was used to sample the SML and associated underlying water. They discovered a noteworthy 23% decrease in k over surfactant concentrations of 200 μg Teq l−1, which were typical for the SML except for the Western Pacific. In addition, they noted a further 62% decrease in natural slicks, which, when taken into account with the known frequency of slick coverage, reduced global CO2 fluxes by 19%.
Also, the sea surface microlayer (SML), the uppermost portion of the ocean surface in direct contact with the atmosphere, may have a substantial impact on a variety of biogeochemical processes, such as the air-sea exchange of energy and materials, including greenhouse gases, and thus of their planet-wide geochemical cycles, as represented in Figure 1.
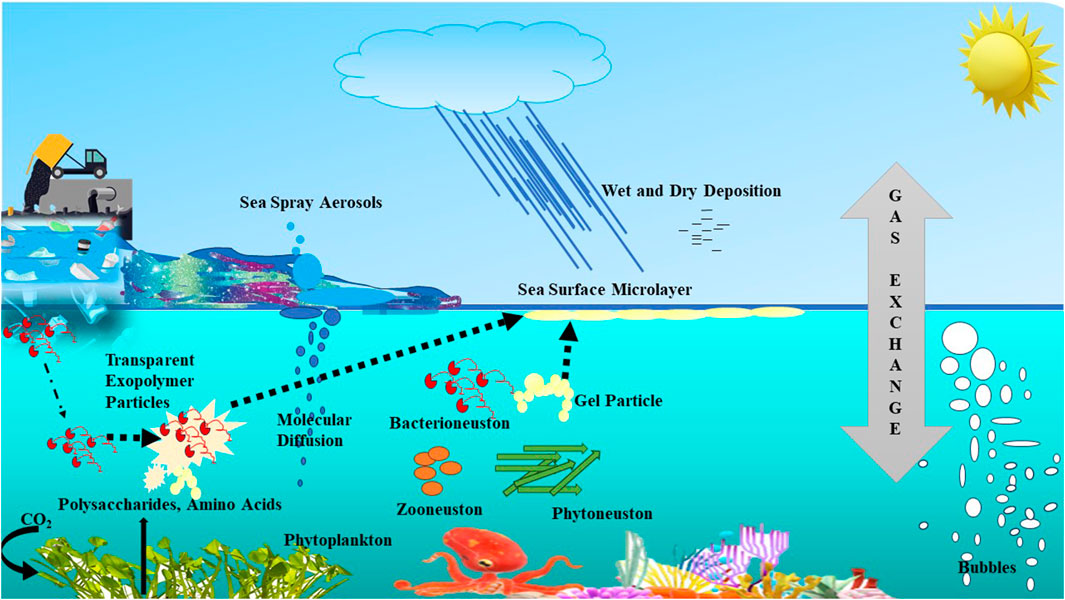
Figure 1. A schematic representation of the mechanisms governing movement across the Sea Surface Microlayer (SML).
The SML generally has higher levels of hydrocarbons, polysaccharides, and amino acids (Engel et al., 2004; Astrahan, 2018). Cunliffe et al. (2011) showed that these high SML concentrations of organic matter may combine to form a gel-like layer of sticky Transparent Exopolymer Particles (TEP). According to Passow and Alldredge (1994), these organic aggregates contain bacteria. Thus, a deeper comprehension of the SML is essential for an enhanced understanding of its structure and function.
Furthermore, the surface-active components of the SML influence air-sea gas exchange in two main ways: Firstly, by decreasing sea surface hydrodynamic motions and so altering turbulent energy transfer, and secondly by acting as a physicochemical barrier to molecular diffusion (McKenna and McGillis, 2004).
Surfactants building up at the air-sea interface are an additional poorly defined descriptor of air-sea gas exchange. According to recent research conducted in the Atlantic Ocean, surfactant enrichment of the SML may be a common occurrence in the open ocean up to wind speeds of around 13 m s−1 or even higher (Sabbaghzadeh et al., 2017). This is significant because, by forming a physico-chemical barrier or by changing features of sea surface hydrodynamics like turbulent energy transfer, micro-scale wave breaking and surface renewal, and by damping small capillary waves, surfactants can change the hydrodynamic properties of the interface and suppress gas exchange (McKenna and McGillis, 2004; Garbe et al., 2014; Pereira et al., 2016).
The SML communities often referred to as “Neuston”, are largely made up of several taxa of autotrophic phytoneuston and heterotrophic bacterioneuston, which flourish in this comparatively enriched organic matrix (Liss and Duce, 1997; Cunliffe et al., 2011). Experimental studies In situ have used free-floating gas exchange boxes (Conrad and Seiler, 1988; Frost, 1999) and a laboratory gas exchange tank (Upstill-Goddard et al., 2003) to investigate the direct role of the bacterioneuston in air-sea gas exchange.
Furthermore, research indicates that wind speed promotes the growth of bacterioneuston communities, indicating that wind-induced wave dynamics affect sea surface microlayer as represented in Figure 2.
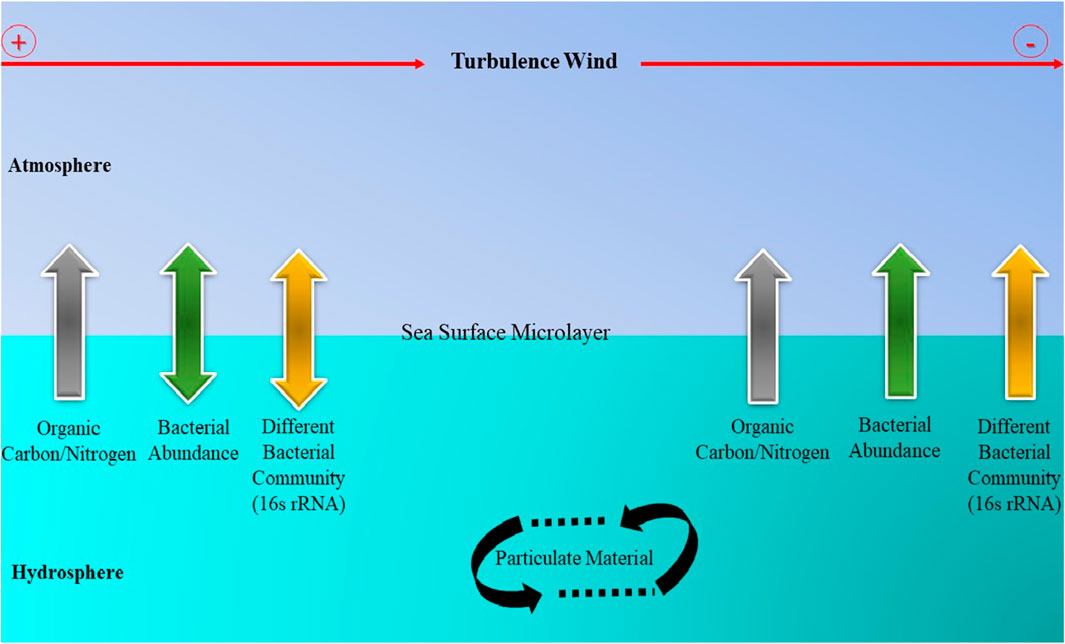
Figure 2. A representation of how wind speed affects the bacterioneuston parameters and organic matter enrichment in the Sea-Surface Microlayer.
Rahlff et al., 2017(a) study investigated the Bacterioneuston community during controlled wind speed induction in an annular wind-wave tunnel (WWT). They discovered that high pCO2 and low wind speed together induced bacterial cell enrichment in the SML over, with high enrichment of bacterial cells detected at an U10 of ≤4.1 m s−1 in the field and ≤5.6 m s−1 in the WWT. Similar to this, Rahlff et al., 2017(b), explored the Surface In Situ Incubator (SISI), a novel and reasonably priced research instrument that oceanographers can use to conduct regular incubation studies in the SML and near-surface layer. This enables the SML and water samples from 1 m to 5 m to be incubated simultaneously at their respective depths of origin. Their result suggests that this SISI is a useful instrument for routinely monitoring the physiological function of plankton-oxygen turnover in biogeochemical cycling and gas exchange processes at and near the sea surface because it provides the opportunity to analyze this process within the SML under the natural effect of abiotic factors.
Studies by Conrad and Seiler (1988), Frost (1999), Upstill-Goddard et al. (2003), Nakajima et al. (2013), and others have demonstrated that the bacterioneuston directly affects air-sea gas exchange by consuming and creating trace gases (e.g., CO, H2, CH4, N2O). Methanotrophs, or bacteria that oxidize methane, were added to laboratory experiments (Upstill-Goddard, 2006) to confirm the possibility of actively controlling the air-sea methane exchange by bacterioneuston; their result suggests it led to enhancement of the apparent transfer velocity by 12%–10%. Their results indicated that bacterioneuston is closely involved in the cycling of some gas traces, such as CH4 and CO2, and that, depending on the prevailing microbial and biogeochemical conditions, it may operate as a minor gas sink or source.
Subtle chemical and biological complexity inherent in the SML is another significant obstacle. A significant portion of the organic material in the SML and the resulting aerosol particles is yet unknown at the molecular level. However, individual compound analysis can only account for a small portion of the total organic matter pool (van Pinxteren et al., 2012; van Pinxteren and Herrmann, 2013). Examples of these compounds include organic amino compounds and carbonyl compounds, which are found in marine aerosol particles and the SML demonstrates the link between the ocean and the atmosphere.
Therefore, a better understanding of the molecular complexity of the marine organic matter and how physical processes, chemical reactions, and organisms affect the composition and size distribution of organic matter may be essential for both improving computations of the sources and sinks of organic aerosols in ocean-atmosphere models and better-describing processes in the SML.
One other component is sea spray aerosol (SSA), which is a suspension of particles in the air, that are created right at the sea surface by bubbles that burst from breaking waves or rain. Owing to the hygroscopic and size-spectrum characteristics of its constituent particles, SSA serves as a medium for the uptake and reactivity of trace gases and it is a primary source of cloud condensation nuclei (CCN) (Engel et al., 2017). In addition to the complex dynamics of its impact on the radiative and microphysical properties of sea clouds, SSA is a significant source of uncertainty when assessing the important but indirect climatic forcing caused by particulate matter in the atmosphere.
In a lab-based investigation, Cochran et al. (2016) showed how surface-active organic molecules might theoretically predict the relative coverages of the air-water interface and the relative contributions of these molecules to artificially manufactured sea spray aerosol. It is still unknown how much of the primary organic aerosol emissions come from organic matter that has been adsorbed on rising bubbles or is already enriched within the SML. According to Bakker et al. (2014), Liss et al. (2014), and Carpenter and Nightingale (2015), the SML produces or consumes a range of climate-relevant gases, including CO, CO2, NO, and halocarbons. Nonetheless, new methods are needed to determine gas fluxes In situ.
Research implies that the ocean may serve as a potential source of perfluoroalkyl acids (PFAAs) for the atmosphere (Johansson et al., 2019). The estimated fluxes of perfluorooctanoic acid (PFOA) and perfluorooctanesulfonic acid (PFOS) from SSA to the atmosphere were comparable with the other two sources of atmospheric PFAAs (i.e., direct emission from manufacturing sources and degradation from volatile precursors), based on laboratory-derived enrichment factors (EFs) and reported median concentrations in seawater (Reth et al., 2011; Johansson et al., 2019; Sha et al., 2020).
Therefore, for 2 years, Sha et al., 2021 undertook a study involving routine air monitoring at two Norwegian coastal sites (Andøya and Birkenes) to gather aerosol samples that included a wide range of SSA, PFAAs, and tracer ions. They noticed In the samples from both locations, there were significant correlations (p < 0.05) between the amounts of PFAA, Na+, and the SSA tracer ion, with Pearson’s correlation coefficients (r) ranging from 0.4 to 0.8. Compared to samples from Birkenes, which is situated closer to urban areas and farther from the coast than Andøya, correlations were found for a greater number of PFAA species in the Andøya samples. According to these strong relationships, SSA is a major source of atmospheric PFAAs that are released into coastal areas.
There is still much to learn about the exact and varied methods by which organic components move from the ocean to the atmosphere through the SML. The SML and bubble film surfaces promote substantial partitioning of surface-active molecule components between the sea and the air. The roles played by the surface activity of the different organic components in transfer processes are undoubtedly major, but their details remain poorly understood. On the other hand, validating models of how future changes in global temperature, acidity, eutrophication, wind speeds, precipitation, and taxon composition will affect organic matter-mediated air-sea fluxes, particularly the lowering of CO2 gas exchange, will be an intriguing challenge.
Therefore, creating a standard (reference) model of the SML that incorporates all pertinent physical, chemical, and biological processes is required to comprehend the roles of the SML in the Earth system and ought to be the main objective of future SML research. Whether microbial community exploits these habitats as nutrient-rich resources before being moved to sea-spray aerosols, transferred to beaches, or returned to bulk water still needs to be elucidated with the help of novel methods. These methods must incorporate the microbial population living on the sea surface. Moreover, it will take coordinated research by interdisciplinary teams with experience in physical oceanography, meteorology, bulk-phase, and surface rheology, phytoplankton physiological ecology, and genomics to resolve the role of biological components in phenomenological models of air-sea exchange of CO2 and other materials and properties.
Author contributions
MK: Writing–original draft, Writing–review and editing. SK: Writing–original draft, Writing–review and editing, Conceptualization, Formal Analysis, Investigation, Supervision, Visualization. AB: Writing–review and editing, Investigation, Supervision, Visualization. IJ: Writing–original draft, Writing–review and editing.
Funding
The author(s) declare that no financial support was received for the research, authorship, and/or publication of this article.
Acknowledgments
Authors are thankfull to SBS University for providing space and facility to carry out this work.
Conflict of interest
The authors declare that the research was conducted in the absence of any commercial or financial relationships that could be construed as a potential conflict of interest.
Publisher’s note
All claims expressed in this article are solely those of the authors and do not necessarily represent those of their affiliated organizations, or those of the publisher, the editors and the reviewers. Any product that may be evaluated in this article, or claim that may be made by its manufacturer, is not guaranteed or endorsed by the publisher.
References
Astrahan, P. (2018). Monocyclic aromatic hydrocarbons (phthalates and BTEX) and aliphatic components in the SE Mediterranean costal Sea-surface microlayer (SML): origins and phase distribution analysis. Mar. Chem. 205, 56–69. doi:10.1016/j.marchem.2018.07.009
Bakker, D. C., Bange, H. W., Gruber, N., Johannessen, T., Upstill-Goddard, R. C., Borges, A. V., et al. (2014). “Air-sea interactions of natural long-lived greenhouse gases (CO2, N2O, CH4) in a changing climate,” in Ocean-atmosphere interactions of gases and particles.
Carpenter, L. J., and Nightingale, P. D. (2015). Chemistry and release of gases from the surface ocean. Chem. Rev. 115 (10), 4015–4034. doi:10.1021/cr5007123
Cayetano, R. D. A., Kim, G. B., Park, J., Yang, Y. H., Jeon, B. H., Jang, M., et al. (2022). Biofilm formation as a method of improved treatment during anaerobic digestion of organic matter for biogas recovery. Bioresour. Technol. 344, 126309. doi:10.1016/j.biortech.2021.126309
Cochran, R. E., Laskina, O., Jayarathne, T., Laskin, A., Laskin, J., Lin, P., et al. (2016). Analysis of organic anionic surfactants in fine and coarse fractions of freshly emitted sea spray aerosol. Environ. Sci. Technol. 50 (5), 2477–2486. doi:10.1021/acs.est.5b04053
Conrad, R., and Seiler, W. (1988). Influence of the surface microlayer on the flux of nonconservative trace gases (CO, H 2, CH 4, N 2 O) across the ocean-atmosphere interface. J. Atmos. Chem. 6, 83–94. doi:10.1007/bf00048333
Cunliffe, M., Upstill-Goddard, R. C., and Murrell, J. C. (2011). Microbiology of aquatic surface microlayers. FEMS Microbiol. Rev. 35 (2), 233–246. doi:10.1111/j.1574-6976.2010.00246.x
Engel, A., Bange, H. W., Cunliffe, M., Burrows, S. M., Friedrichs, G., Galgani, L., et al. (2017). The ocean's vital skin: toward an integrated understanding of the sea surface microlayer. Front. Mar. Sci. 4, 165. doi:10.3389/fmars.2017.00165
Engel, A., Thoms, S., Riebesell, U., Rochelle-Newall, E., and Zondervan, I. (2004). Polysaccharide aggregation as a potential sink of marine dissolved organic carbon. Nature 428 (6986), 929–932. doi:10.1038/nature02453
Frost, T. (1999). “Environmental controls of air-water gas exchange,”. England: University of Newcastle upon Tyne. Doctoral dissertation.
Garbe, C. S., Rutgersson, A., Boutin, J., de Leeuw, G., Delille, B., Fairall, C. W., et al. (2014). “Transfer across the air-sea interface,” in Ocean-atmosphere interactions of gases and particles, 55–112.
Gattuso, J. P., Magnan, A., Billé, R., Cheung, W. W., Howes, E. L., Joos, F., et al. (2015). OCEANOGRAPHY. Contrasting futures for ocean and society from different anthropogenic CO₂ emissions scenarios. Science 349 (6243), aac4722. doi:10.1126/science.aac4722
Johansson, J. H., Salter, M. E., Navarro, J. A., Leck, C., Nilsson, E. D., and Cousins, I. T. (2019). Global transport of perfluoroalkyl acids via sea spray aerosol. Environ. Sci. Process. Impacts 21 (4), 635–649. doi:10.1039/c8em00525g
Liss, P. S., Marandino, C. A., Dahl, E. E., Helmig, D., Hintsa, E. J., Hughes, C., et al. (2014). “Short-lived trace gases in the surface ocean and the atmosphere,” in Ocean-atmosphere interactions of gases and particles, 1–54.
McKenna, S. P., and McGillis, W. R. (2004). The role of free-surface turbulence and surfactants in air–water gas transfer. Int. J. Heat Mass Transf. 47 (3), 539–553. doi:10.1016/j.ijheatmasstransfer.2003.06.001
Mustaffa, N. I. H., Ribas-Ribas, M., Banko-Kubis, H. M., and Wurl, O. (2020). Global reduction of in situ CO2 transfer velocity by natural surfactants in the sea-surface microlayer. Proc. R. Soc. A 476 (2234), 20190763. doi:10.1098/rspa.2019.0763
Nakajima, R., Tsuchiya, K., Nakatomi, N., Yoshida, T., Tada, Y., Konno, F., et al. (2013). Enrichment of microbial abundance in the sea-surface microlayer over a coral reef: implications for biogeochemical cycles in reef ecosystems. Mar. Ecol. Prog. Ser. 490, 11–22.
Passow, U., and Alldredge, A. L. (1994). Distribution, size and bacterial colonization of transparent exopolymer particles (TEP) in the ocean. Mar. Ecol. Prog. Ser. 113, 185–198. doi:10.3354/meps113185
Pereira, R., Schneider-Zapp, K., and Upstill-Goddard, R. C. (2016). Surfactant control of gas transfer velocity along an offshore coastal transect: results from a laboratory gas exchange tank. Biogeosciences 13 (13), 3981–3989. doi:10.5194/bg-13-3981-2016
Philipp, L. A., Bühler, K., Ulber, R., and Gescher, J. (2023). Beneficial applications of biofilms. Nat. Rev. Microbiol. 22, 276–290. doi:10.1038/s41579-023-00985-0
Rahlff, J., Stolle, C., Giebel, H. A., Brinkhoff, T., Ribas-Ribas, M., Hodapp, D., et al. (2017a). High wind speeds prevent formation of a distinct bacterioneuston community in the sea-surface microlayer. FEMS Microbiol. Ecol. 93 (5), fix041. doi:10.1093/femsec/fix041
Rahlff, J., Stolle, C., and Wurl, O. (2017b). SISI: a new device for in situ incubations at the ocean surface. J. Mar. Sci. Eng. 5 (4), 46. doi:10.3390/jmse5040046
Reth, M., Berger, U., Broman, D., Cousins, I. T., Nilsson, E. D., and McLachlan, M. S. (2011). Water-to-air transfer of perfluorinated carboxylates and sulfonates in a sea spray simulator. Environ. Chem. 8 (4), 381–388. doi:10.1071/en11007
Rhein, M., Rintoul, S. R., Aoki, S., Campos, E., Chambers, D., Feely, R. A., et al. (2013) “Observations: ocean,” in Climate change 2013: the physical science basis. Contribution of working group I to the fifth assessment report of the intergovernmental panel on climate change. Editors T. F. Stocker, D. Qin, G.-K. Plattner, M. Tignor, S. K. Allen, J. Boschunget al. (Cambridge, United Kingdom and New York, NY: Cambridge University Press), 255–316.
Sabbaghzadeh, B., Upstill-Goddard, R. C., Beale, R., Pereira, R., and Nightingale, P. D. (2017). The Atlantic Ocean surface microlayer from 50°N to 50°S is ubiquitously enriched in surfactants at wind speeds up to 13 m s−1. Geophys. Res. Lett. 44 (6), 2852–2858. doi:10.1002/2017GL072988
Sha, B., Johansson, J. H., Benskin, J. P., Cousins, I. T., and Salter, M. E. (2020). Influence of water concentrations of perfluoroalkyl acids (PFAAs) on their size-resolved enrichment in nascent sea spray aerosols. Environ. Sci. Technol. 55 (14), 9489–9497. doi:10.1021/acs.est.0c03804
Sha, B., Johansson, J. H., Tunved, P., Bohlin-Nizzetto, P., Cousins, I. T., and Salter, M. E. (2021). Sea spray aerosol (SSA) as a source of perfluoroalkyl acids (PFAAs) to the atmosphere: field evidence from long-term air monitoring. Environ. Sci. Technol. 56 (1), 228–238. doi:10.1021/acs.est.1c04277
Upstill-Goddard, R. C. (2006). Air–sea gas exchange in the coastal zone. Estuar. Coast. Shelf Sci. 70 (3), 388–404. doi:10.1016/j.ecss.2006.05.043
Upstill-Goddard, R. C., Frost, T., Henry, G. R., Franklin, M., Murrell, J. C., and Owens, N. J. (2003). Bacterioneuston control of air-water methane exchange determined with a laboratory gas exchange tank. Glob. Biogeochem. cycles 17 (4). doi:10.1029/2003gb002043
van Pinxteren, M., and Herrmann, H. (2013). Glyoxal and methylglyoxal in Atlantic seawater and marine aerosol particles: method development and first application during the Polarstern cruise ANT XXVII/4. Atmos. Chem. Phys. 13 (23), 11791–11802. doi:10.5194/acp-13-11791-2013
van Pinxteren, M., Muller, C., Iinuma, Y., Stolle, C., and Herrmann, H. (2012). Chemical characterization of dissolved organic compounds from coastal sea surface microlayers (Baltic Sea, Germany). Environ. Sci. Technol. 46 (19), 10455–10462. doi:10.1021/es204492b
Venegas, R. M., Acevedo, J., and Treml, E. A. (2023). Three decades of ocean warming impacts on marine ecosystems: a review and perspective. Deep Sea Res. Part II Top. Stud. Oceanogr. 212, 105318. doi:10.1016/j.dsr2.2023.105318
Keywords: sea surface microlayer (SML), biofilms, sea spray aerosol (SSA), greenhouse gases, slicks
Citation: Kumari M, Karn SK, Babu AG and Jenkinson IR (2024) Role of the sea surface biofilm in regulating the Earth’s climate. Front. Environ. Sci. 12:1390660. doi: 10.3389/fenvs.2024.1390660
Received: 26 February 2024; Accepted: 19 April 2024;
Published: 20 May 2024.
Edited by:
Wei He, China University of Geosciences, ChinaReviewed by:
Shrameeta Shinde, Miami University, United StatesNur Ili Hamizah Mustaffa, Universiti Putra Malaysia, Malaysia
Copyright © 2024 Kumari, Karn, Babu and Jenkinson. This is an open-access article distributed under the terms of the Creative Commons Attribution License (CC BY). The use, distribution or reproduction in other forums is permitted, provided the original author(s) and the copyright owner(s) are credited and that the original publication in this journal is cited, in accordance with accepted academic practice. No use, distribution or reproduction is permitted which does not comply with these terms.
*Correspondence: Santosh Kumar Karn, c2FudG9zaGthcm5AZ21haWwuY29t, c2FudG9zaGthcm4uc2JzdUBnbWFpbC5jb20=