- Department of Environmental and Biological Chemistry, Chungbuk National University, Cheongju, Republic of Korea
Cationic metals such as lead (Pb) and metalloids such as arsenic (As) in contaminated soil can be simultaneously immobilized by iron phosphate because As(V) is stabilized by binding to iron (hydr)oxides and metals precipitate with phosphate. However, phosphate competes with As for sorption sites, which may affect the simultaneous stabilization of Pb and As. Therefore, the purpose of this study was to evaluate the simultaneous stabilization of As and Pb using iron phosphate both in single- and multi-metal solutions and soil. In both single- and multiple-element solutions, Pb was completely removed by iron phosphate. Arsenic immobilization was explained by the Freundlich isotherm. Arsenate [As(V)] removal by iron phosphate decreased with increasing pH, while arsenite [As(III)] removal increased with increasing pH. The extraction of bioavailable As from contaminated soil increased after incubation with iron phosphate, whereas the concentration of bioavailable Pb decreased. The increase in bioavailable As can be attributed to As substitution by phosphate, which was not immobilized by iron. Although both As and Pb can be removed by iron phosphate in aqueous solutions, an iron phosphate mineral with relatively low Ksp should be used to simultaneously immobilize As and Pb in soil.
1 Introduction
Place’s Agricultural land surrounding abandoned mines is often contaminated with metal(loid)s. Arsenic (As) is one of the elements found in higher concentrations in mine sites, often associated with metals such as cadmium (Cd), copper (Cu), lead (Pb), and zinc (Zn) (Bolan et al., 2014). These pollutants adversely affect plants growing on croplands and migrate into the surrounding waterways and air, thereby increasing environmental pollution problems (Esshaimi et al., 2012). Arsenic is a highly toxic carcinogen, and the consumption of As-contaminated rice may cause liver, kidney, lung, and skin cancer (Yu et al., 2003; Chikkanna et al., 2019).
Metals such as Cd, Cu, Pb, and Zn are some of the elements usually found near mine sites in concentrations that might be toxic to biological systems (Han et al., 2002; Sayadi, 2014). Metals can be absorbed and bioaccumulated in crops and living organisms to high concentrations. This poses significant threats to the overall health of ecosystems and may ultimately lead to the death of organisms (Nagajvoti et al., 2010). Soil pollutants such as metals and metalloids often occur with more than one element in increased concentration, and it is necessary to control them simultaneously (Martin, 2012).
However, the physicochemical properties of metals such as Pb and metalloids such as As are very different, so it is difficult to control them simultaneously. Arsenic exists as a neutral form, such as organic water-soluble H3AsO3 and AsO(OH)3, or as an oxyanion in the natural environment, so it has different chemical properties from metallic contaminants that normally exist in cationic forms (Stollenwerk, 2003; Cortes-Arriagada and Ortega, 2020). Toxic metal(loid)s such as As, Cd, Cu, Pb, and Zn can be stabilized by changing the pH and redox potential (Sharma et al., 2015). For example, as the pH increases, arsenate adsorption decreases because the iron (Fe) mineral surface has a net negative charge, weakening its binding to minerals and increasing its mobility and toxicity (Raven et al., 1998). In contrast, Pb precipitates as lead hydroxide at a pH higher than 6, reducing mobility and toxicity (Payne and Abdel-Fattah, 2004). Furthermore, lower Eh conditions contribute to the reduction of As(V) to As(III), increasing the toxicity and release of metals bound to Fe/Mn oxides because of the decomposition of Fe/Mn oxides under reducing conditions (Yang et al., 2022). The mobility of metals in soil generally decreases with increasing pH; however, As(V) can be desorbed or mobilized because its mobility increases with increasing pH, causing it to diffuse into the environment (Stollenwerk, 2003; Kim et al., 2011).
Most of As exists as arsenite [As(III)] and arsenate [As(V)] in soil and water environments, and As(III) is more mobile and toxic than As(V) (Moon et al., 2004). Both As(III) and As(V) adsorb to iron (hydr)oxides such as goethite and ferrihydrite, which effectively remove As and lower its toxicity (Smedley and Kinniburgh, 2002; Sundar and Chakravarty, 2010; Park et al., 2016; Zhang et al., 2019). Specifically, the ferric-arsenate [Fe(III)–As(V)] precipitate and its natural mineral form (scorodite, FeAsO4·2H2O) occur in the As-contaminated soil (Zhao et al., 2021). Once As in the soil is co-precipitated with iron (hydr)oxides as ferric-arsenate, the toxicity and mobility of As will be reduced (Aredes et al., 2013).
The stabilization mechanism of metals such as Cd and Pb includes precipitation with phosphates by substituting cations in phosphate compounds (Wang et al., 2001; Bolan et al., 2014). In addition, because phosphate is a nutrient essential for plant growth, treatment with phosphates tends to promote growth in metal-contaminated soils (Cao et al., 2003; Soares and Siqueira, 2008). However, phosphate treatment in contaminated soil adversely affects the stability of As because its chemical properties are similar to those of phosphates, which induces competition for adsorption sites on iron oxides (Hughes, 2002; Theodoratos et al., 2002; Kaur et al., 2011). Therefore, it is difficult to simultaneously control As and metals by phosphate treatment in soil.
Cui et al. (2010) suggested that As and Pb could be immobilized by Fe and phosphate, respectively, and they amended iron as ferrous sulfate and phosphate as calcium magnesium phosphate, phosphate rock, and single super-phosphate. However, because oxyanions such as As and antimony (Sb) in the soil environment are stabilized by iron oxides and metals with divalent cations are stabilized by phosphate (Liang et al., 2014; Sazakli et al., 2015), compounds containing both Fe and phosphate may simultaneously control multiple metals and metalloids. Therefore, the objective of this study was to evaluate the possibility of simultaneous stabilization of As and Pb using iron phosphate. The concomitant removal of As and Pb by iron phosphate was evaluated in solutions with different pH values. In addition, the application of iron phosphate for the remediation of soil contaminated with multiple metals and metalloids was tested.
2 Materials and methods
2.1 Iron phosphate synthesis
Iron phosphate (FePO4) was prepared by mixing 25 mL of 0.83 M Iron(III) chloride hexahydrate and 25 mL of 0.83 M dibasic anhydrous sodium phosphate in a 50-mL conical tube. After mixing, the solution was left to react for 30 min, and the white precipitate was recovered by centrifugation at 4,000 rpm for 10 min. The precipitate was washed several times with deionized water to remove the remaining ions and then dried in an oven at 60°C.
The mineralogy was confirmed using X-ray diffraction (Rigaku, JP/SmartLab, 9 kW). The surface structure and composition were analyzed using scanning electron microscopy combined with an energy-dispersive X-ray spectroscopy (EDS) (Zeiss Ultra Plus). Specific surface areas were measured using the Brunauer–Emmett–Teller (BET) protocol (Micromeritics, ASAP 2020).
2.2 Metal removal using iron phosphate
Metal removal experiments were conducted in single and mixed solutions of As and Pb. The As(V) stock solution was prepared from sodium arsenate heptahydrate (Na2HAsO4·7H2O). The As(III) stock solution was prepared from sodium arsenite (NaAsO2). The Pb stock solution was prepared from lead nitrate [Pb(NO3)2]. The As concentration in the solutions ranged from 0.5 to 10 mg/L, and the concentration for the Pb solutions was 5–100 mg/L. Solutions containing both As and Pb were prepared by mixing stock solutions to adjust the final concentration to be the same as for a single-element solution. To evaluate the pH effect on metal or As immobilization, the solution pH was adjusted using acetate buffer, Tris-HCl buffer, and glycine buffer to achieve pH 5, 7, and 10, respectively.
The experiment was conducted by mixing 0.05 g of iron phosphate and 20 mL of elemental solution in a 50-mL conical tube and shaking it for 24 h at 180 rpm (Kim and Park, 2023). The suspension was filtered using a 0.45 μm syringe filter, and the concentrations of As, Pb, Fe, and P were measured using inductively coupled plasma optical emission spectroscopy (ICP-OES, PerkinElmer, Avio 500).
Through the calculation of the adsorption isotherm, the chemical reactions and properties of the minerals and solutions can be determined (Olsen and Watanabe, 1957). The data on the immobilization of As by iron phosphate were analyzed using the Langmuir and Freundlich isotherm models. The Langmuir (Eq. 1) and Freundlich (Eq. 2) isotherms can be written as follows:
where,
2.3 Incubation of metal- and arsenic-contaminated soils with iron phosphate
Loamy sand soil contaminated with metal(loid)s such as As, Cd, Cu, Pb, and Zn was collected around an abandoned metal mine, dried at room temperature, and sieved to collect particles <2 mm in size. The pH and EC of the soil were measured after shaking 5 g of soil in 25 mL of deionized water for 30 min. The organic matter in the soil was analyzed using the Walkley–Black method (Walkley and Black, 1934). The total element concentrations were determined by digesting the soil with aqua regia. The chemical properties of the soil are presented in Supplementary Table S1. To immobilize the As, Cd, Cu, Pb, and Zn with iron phosphate, 10 g of mine soil was mixed with different amounts of iron phosphates (5, 10, and 20 g/kg). The water content of the soil was kept at 15% by adding 2 mL of deionized water and then incubating it in an incubator at 25°C for 7 days. To evaluate the immobilization of the target elements in the soil, they were extracted from soil samples in 25 mL of deionized water and 50 mL of 0.05 M (NH4)2SO4 for 2 h at 180 rpm in a shaker (Taghizadeh-Toosi et al., 2012). The supernatant was separated by centrifugation at 4,000 rpm for 10 min and then filtered through a 0.45-μm syringe filter. Then, the bioavailable metal and As concentrations were analyzed using ICP-OES.
3 Results and discussion
3.1 Adsorption isotherm
XRD and SEM-EDX analysis showed amorphous iron phosphate with fine particles (Supplementary Figures S1, S2). The BET specific surface area of the iron phosphate was measured and found to be 72.2 ± 0.2 m2/g due to the small size of the nanoparticles.
Arsenate was immobilized by iron phosphate, and the immobilization rate ranged from 50.7% to 63.7%, depending on the initial As(V) concentration. As the concentration of As in the initial solution increased, the phosphorus (P) concentration increased in the final solution (Table 2). This suggests that As(V) substitutes for phosphate in iron phosphate (Tawfik and Viola, 2011). Arsenate, as the phosphate analog, induced the release of phosphate because of competition for sorption sites (Lambkin and Alloway, 2003). However, Fe was not released during As(V) immobilization, indicating that As(V) reacted with Fe and was removed from the solution.
The immobilization data were well-fitted to both the Langmuir and Freundlich isotherms. The Freundlich adsorption isotherm better explained As(V) adsorption (R2 = 0.99) than the Langmuir adsorption isotherm did (R2 = 0.96) (Figure 1; Table 1). The absence of a plateau in Figure 1 further supports the conclusion that the Freundlich adsorption isotherm is a more suitable model for explaining the adsorption behavior observed in the study. The maximum adsorption amount (Qm) calculated based on the Langmuir isotherm model was 5.12 mg/g (Table 1). The Langmuir isotherm implies monolayer adsorption, whereas the Freundlich adsorption isotherm shows multilayer adsorption (Priya et al., 2022). A better fit to the Freundlich adsorption isotherm suggests that the adsorbent has a higher adsorption capacity (Zhou et al., 2017). Although the immobilization of As(V) and As(III) by iron phosphate was explained by the Freundlich adsorption isotherm, the surface precipitation of As by iron phosphate cannot be excluded. Ardes et al. (2013) showed that surface precipitation might result from the kinetics of adsorption/desorption, which can be recognized as ternary adsorption. Tiwari and Pandey (2013) also explained that Fe(III) and As(V) were precipitated, followed by the complexation of As with ferrihydrite.
The precipitation reaction requires partial dissolution of Fe from minerals to induce surface precipitate. When iron phosphate reacted with As, dissolved Fe resulted in the formation of various precipitates associated with As(V) (Lenoble et al., 2005). Iron was released in the solution at a pH higher than 7, which indicates the possibility of surface precipitation in alkaline conditions (Table 3). Under the experimental condition (pH 3), As exists as H3AsO3 and Fe is in the form of Fe2+ according to the Eh–pH diagram of As and Fe, which might contribute to both adsorption and surface precipitation (Figure 2A). However, Fe2+ was not detected in the experimental solution, suggesting that As sorption was mainly attributed by the adsorption process (Aredes et al., 2013). Gallegos-Garcia et al. (2012) also reported that As showed a high affinity for iron (hydr)oxide minerals, and ion exchange adsorption of As on the mineral surface was the As removal mechanism when Fe was not eluted.
To evaluate the possibility of As precipitation with iron phosphate, geochemical modeling using PHREEQC was implemented with the following solution properties: temperature, 25°C; pH, 3, 5, 7, and 10; pe, 4; solution density, 1; As(V), 10 mg/L; sodium (Na), 9.7 mg/L; Fe, 0, 0, 29.3, and 14.6 mg/L; P, 10.3, 9.1, 42.1, and 96.6 mg/L; and strengite (FePO4·2H2O), 2.5 g/L, respectively. The results showed that the saturation index of FeAsO4·2H2O ranged from −5 to −7 according to pH, indicating that there is a possibility of As precipitation with Fe if the condition changes (Figure 3). At pH 7, the saturation index of As2O3 and native As increased, which is related to the release of Fe from iron phosphate, resulting in the possibility of As mineral precipitation (Figure 3).
Arsenite was not immobilized by iron phosphate at the initial low As(III) concentration and was only slightly removed at a higher As(III) concentration (Figure 4). Because As(III) has higher mobility than As(V), it is estimated that the immobilization rate was lower (Oremland and Stolz, 2003). Therefore, it is necessary to oxidize As(III) and adsorb it as As(V) in the real environment.
Lead was not detected in the solution, and the concentration of released P decreased as the Pb concentration increased because of the precipitation of P with Pb (Table 2). Because almost 100% of Pb was removed, an adsorption isotherm model was not applied for Pb removal. According to the Eh–pH diagram of Pb and phosphate, Pb and P exist as Pb2+ and H2PO4−, respectively, in the experimental condition, precipitation might be the main mechanism of Pb removal in the solution (Figure 2B). The phosphate concentration increased with increasing pH in the solution, showing that there is a possibility of Pb precipitation with phosphate (Table 3). Cao et al. (2003) also reported that Pb removal from the solution by phosphate primarily involves the precipitation of metal phosphate along with some ion exchange processes and surface complexation reactions on the phosphate rock.
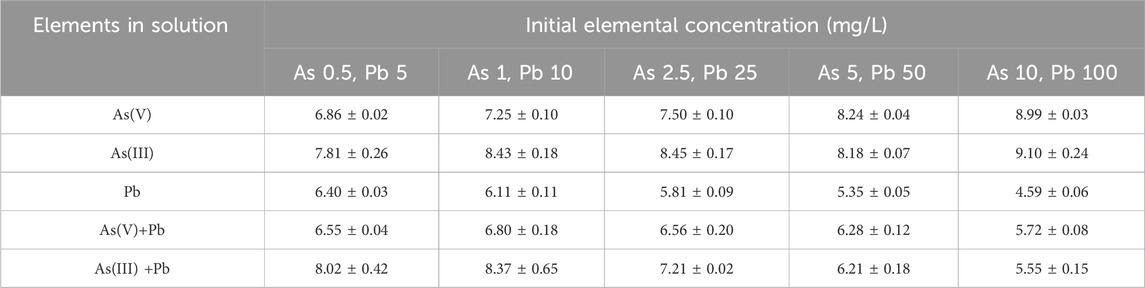
Table 2. Phosphorus concentration (mg/L) in solution after the removal of As(V), As(III), and Pb by iron phosphate.
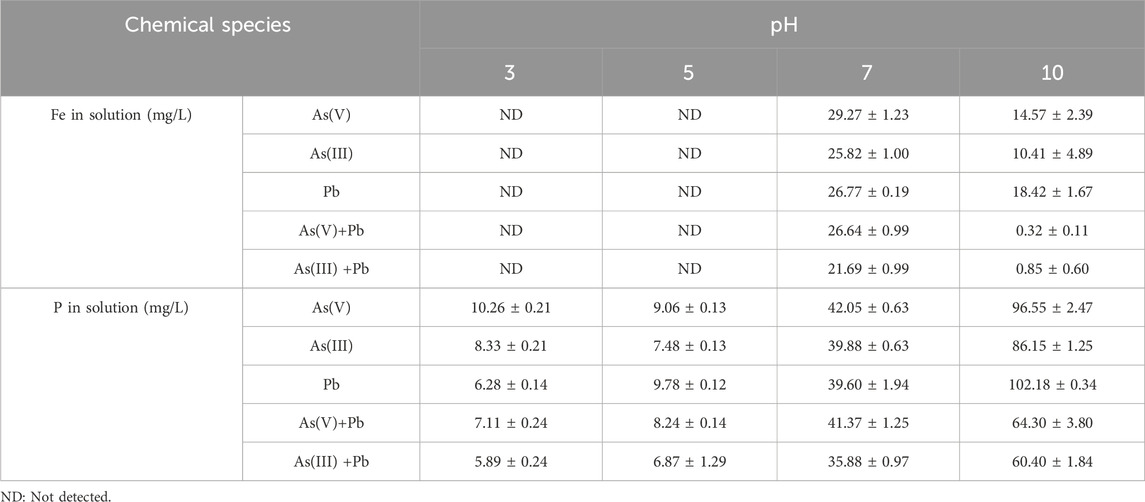
Table 3. Iron and P concentrations after the removal of As(V), As(III), and Pb at different pH values.
In the mixed solution of As(V) and Pb, the initial As(V) and Pb concentrations decreased, indicating that As(V) reacted with Pb and precipitated. When soil is contaminated with both As and Pb and lead arsenate is formed, their bioavailability is reduced compared to when As and Pb are present separately in the soil. Thus, in this case, having both reduces their environmental impact (Gamble et al., 2018; Li et al., 2019).
In the simultaneous removal of As(III) and Pb by iron phosphate, As(III) did not react with Pb. Arsenite removal was higher in the mixed solution than in the single-element solution, and 100% of Pb was removed by iron phosphate (Figure 4). The reason for the higher removal of both As(III) and Pb in the mixed solution can be attributed to the oxidation of As(III) by Fe(III), followed by phosphate substitution and the reaction of Pb with the released phosphate (Lenoble et al., 2005). The Fe released from the reaction of Pb and phosphate might participate in the reaction of Fe with As(III), leading to a higher As(III) removal rate in mixed solution than in single element solution. Arsenite adsorption in the mixed solution was better explained by the Freundlich isotherm than by the Langmuir isotherm (Figure 1).
3.2 Effect of pH on metal and arsenic removal
As the pH increased, As(V) immobilization decreased, while As(III) immobilization showed the opposite tendency (Figure 5). Decreased As(V) immobilization can be explained by negatively charged As species predominant in the pH range of 2–12 and an increase in negative mineral surface at a higher pH than pHZPC (Mamindy-Pajany et al., 2011). Arsenic is oxidized at low pH and mainly exists in the form of As(V) in the natural environment (Bissen and Frimmel, 2003; Tabelin et al., 2020). The removal rates of As(V) and As(III) are contradictory because As(V) competes with hydroxide ions, which promotes desorption when the pH increases, thereby increasing mobility (Kim et al., 2019).
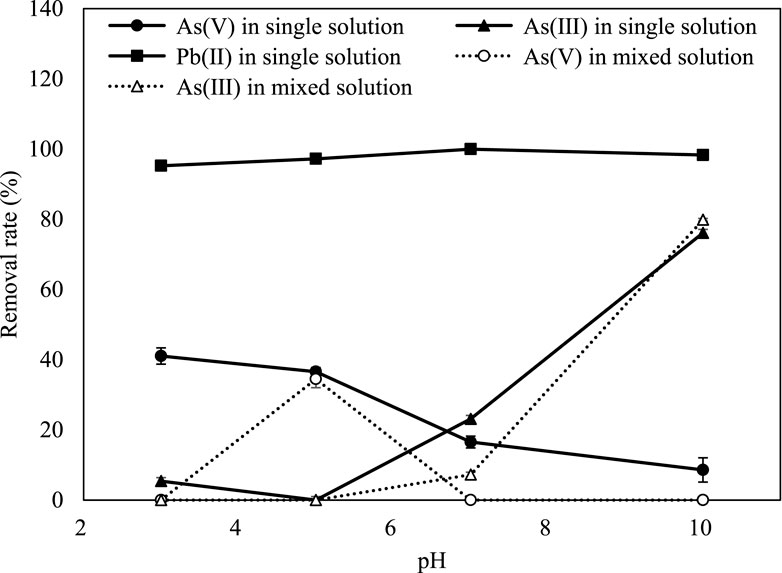
Figure 5. Removal rate of As(V), As(III), and Pb in a single solution and As(V) and As(III) when mixed with Pb according to different solution pH values.
The iron concentration was analyzed and found to be the highest at pH 7.8 in the adsorption experiment. This might be because iron is oxidized and precipitated as a hydroxide as the pH increases (Table 3) (Liu et al., 2005). The phosphorus concentration also increased as the pH rose due to an increase in the substitution of As(V) and OH− for phosphate (Table 3) (Borgnino et al., 2006; Barrow, 2017). The phosphorus concentration in the As(III)-dissolved solution also increased with increasing pH because of the substitution of phosphate by OH− (Deng et al., 2018). As the pH increased, more phosphate dissolved in the solution, which enhanced the rate of Pb removal (Table 3; Figure 5). However, Pb has a high removal rate (>90%) at all pH levels, so a slight effect of pH on Pb removal was observed (Figure 5). This is mainly because Pb has a high removal rate compared to other elements and less adsorption competition with hydrogen ions (Weng et al., 2011; Kocabaş-Ataklı and Yürüm, 2013).
The pH effect on the simultaneous removal of As and Pb by iron phosphate in a mixed solution was evaluated. However, the initially measured As and Pb concentrations were low in various pH ranges because lead arsenate was formed (Figure 5). The co-precipitation of As(III) and Pb increased with increasing pH, and As(III) further increased in the mixed solution compared to the single As(III) solution (Figure 5). The iron concentration increased to pH 7 and decreased after that, which might react with As(III) (Table 3).
3.3 Contaminated soil incubation experiments
As the amount of added iron phosphate increased, the concentration of extracted As increased (Figure 6A). Free phosphate ions existed in the iron phosphate substituted for As in the soil and thus increased As mobility. Although bioavailable As increased with increasing iron phosphate, the Pb concentration in the extraction decreased with increasing application of iron phosphate (Figure 6B). Because the reactivity of phosphate for immobilization with Pb (and for As substitution) is higher than that of iron for immobilization with As, the extracted As was not immobilized by the released Fe (Figure 4). In addition, the relatively high solubility product of iron phosphate might cause the substitution of arsenate by phosphate because phosphate is a chemical analog of As(V) (Strawn, 2018).
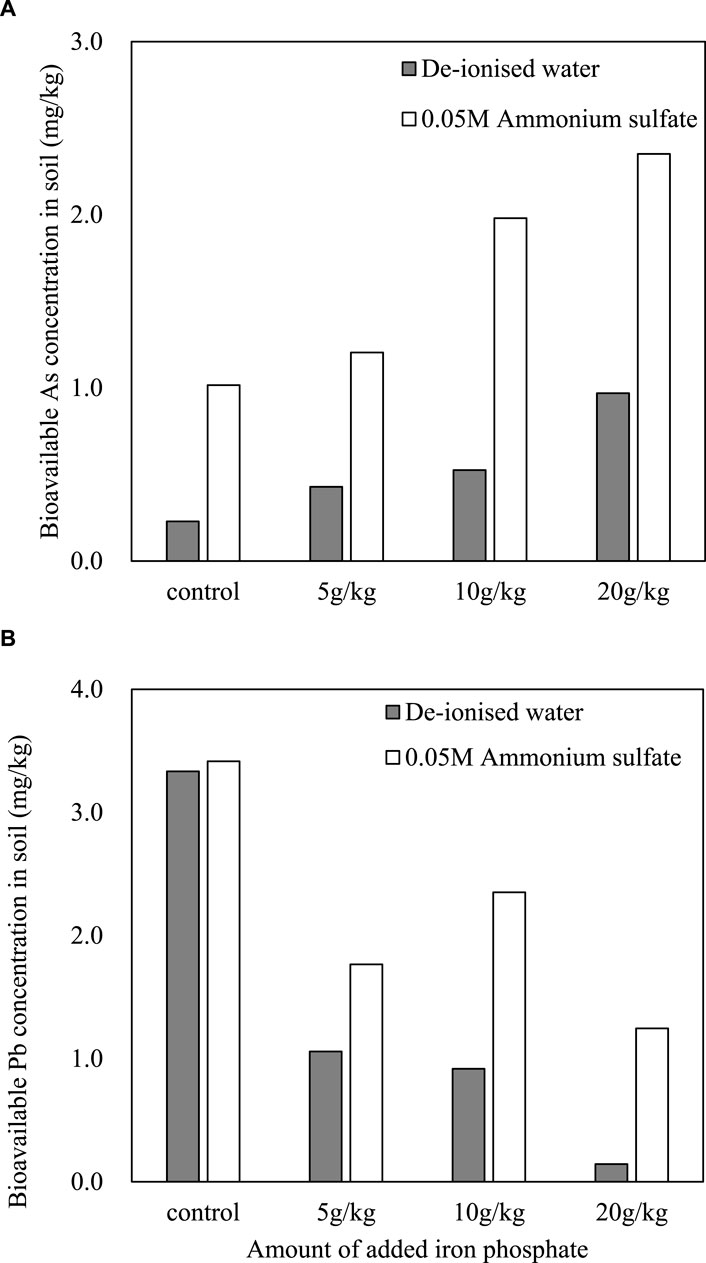
Figure 6. Arsenic (A) and Pb (B) concentrations extracted with de-ionized water and 0.05 M ammonium sulfate from mine soil after incubation with iron phosphate.
Lenoble et al. (2005) showed that As was removed by iron phosphate in solution by solid dissolution and phosphate/arsenate exchange. However, they did not test As immobilization in soil. In our study, As mobility increased with iron phosphate, even though As was removed by iron phosphate in solution. In addition, a variety of minerals and competing ions in soil might result in different stabilizations of As in the solution and soil. Therefore, the reactivity of phosphate should be considered for the simultaneous immobilization of As and Pb using iron phosphate compounds in soil. With the reduced reactivity of phosphate, the substitution of As by phosphate will be less, and the simultaneous immobilization of both As and metals can be achieved. For example, oxidizing a part of pyrite (FeS2) and coating the surface of pyrite with phosphoric acid to produce iron phosphate (FePO4) decreased As and some of the metals in soil (Fytas and Evaneglou, 1998). Although the immobilization rate of iron phosphate varies from metal to metal, it is possible to simultaneously adsorb As, Cd, and Pb (Yuan et al., 2017).
4 Conclusion
Both As and Pb showed higher removal in a mixed-element solution than in single-element solutions. In the case of As(III), a small amount was adsorbed with iron phosphate in the single-element solution, but in the mixed solution with Pb, As(III) removal increased. Therefore, a synergistic effect was found when iron phosphate was applied to multiple-element-contaminated water. The removal of As was affected by pH, and the pH effect on the removal revealed a contrast between As(III) and As(V). The treatment of metal(loid)-contaminated soil with iron phosphate decreased the bioavailable Pb concentration but increased the As concentration. This suggests that the competition between As and phosphate interfered with As immobilization in the soil. Therefore, iron phosphate is a potential candidate for the remediation of water contaminated with a mixture of As and metals. However, when treating contaminated soil, it is necessary to control the pH and consider the reactivity of iron phosphate.
Data availability statement
The original contributions presented in the study are included in the article/Supplementary Material; further inquiries can be directed to the corresponding author.
Author contributions
HK: writing–original draft. JP: writing–original draft and writing–review and editing.
Funding
The author(s) declare that financial support was received for the research, authorship, and/or publication of this article. This research was supported by the “Regional Innovation Strategy (RIS)” through the National Research Foundation of Korea (NRF) funded by the Ministry of Education (MOE) (2021RIS-001).
Conflict of interest
The authors declare that the research was conducted in the absence of any commercial or financial relationships that could be construed as a potential conflict of interest.
Publisher’s note
All claims expressed in this article are solely those of the authors and do not necessarily represent those of their affiliated organizations, or those of the publisher, the editors, and the reviewers. Any product that may be evaluated in this article, or claim that may be made by its manufacturer, is not guaranteed or endorsed by the publisher.
Supplementary material
The Supplementary Material for this article can be found online at: https://www.frontiersin.org/articles/10.3389/fenvs.2024.1358561/full#supplementary-material
References
Aredes, S., Klein, B., and Pawlik, M. (2013). The removal of arsenic from water using natural iron oxide minerals. J. Clean. Prod. 60, 71–76. doi:10.1016/j.jclepro.2012.10.035
Barrow, N. J. (2017). The effects of pH on phosphate uptake from the soil. Plant soil 410 (1-2), 401–410. doi:10.1007/s11104-016-3008-9
Bissen, M., and Frimmel, F. H. (2003). Arsenic—a review. Part I: occurrence, toxicity, speciation, mobility. Acta hydrochimica hydrobiologica 31 (1), 9–18. doi:10.1002/aheh.200390025
Bolan, N., Kunhikrishnan, A., Thangarajan, R., Kumpiene, J., Park, J., Makino, T., et al. (2014). Remediation of heavy metal (loid) s contaminated soils–to mobilize or to immobilize? J. Hazard. Mater. 266, 141–166. doi:10.1016/j.jhazmat.2013.12.018
Borgnino, L., Avena, M., and De Pauli, C. (2006). Surface properties of sediments from two Argentinean reservoirs and the rate of phosphate release. Water Res. 40 (14), 2659–2666. doi:10.1016/j.watres.2006.05.007
Cao, X., Ma, L. Q., and Shiralipour, A. (2003). Effects of compost and phosphate amendments on arsenic mobility in soils and arsenic uptake by the hyperaccumulator, Pteris vittata L. Environ. Pollut. 126 (2), 157–167. doi:10.1016/s0269-7491(03)00208-2
Chikkanna, A., Mehan, L., Sarath, P. K., and Ghosh, D. (2019). “Arsenic exposures, poisoning, and threat to human health: arsenic affecting human health,” in Environmental exposures and human health challenges Pennsylvania, PA, USA (IGI Global), 86–105.
Cortes-Arriagada, D., and Ortega, D. E. (2020). Removal of arsenic from water using iron-doped phosphorene nanoadsorbents: a theoretical DFT study with solvent effects. J. Mol. Liq. 307, 112958. doi:10.1016/j.molliq.2020.112958
Cui, Y., Du, X., Weng, L., and Van Riemsdijk, W. H. (2010). Assessment of in situ immobilization of lead (Pb) and arsenic (As) in contaminated soils with phosphate and iron: solubility and bioaccessibility. Water, Air, & Soil Pollut. 213 (1), 95–104. doi:10.1007/s11270-010-0370-8
Deng, Y., Li, Y., Li, X., Sun, Y., Ma, J., Lei, M., et al. (2018). Influence of calcium and phosphate on pH dependency of arsenite and arsenate adsorption to goethite. Chemosphere 199, 617–624. doi:10.1016/j.chemosphere.2018.02.018
Esshaimi, M., Ouazzani, N., Avila, M., Perez, G., Valiente, M., and Mandi, L. (2012). Heavy metal contamination of soils and water resources Kettara abandoned mine. Am. J. Environ. Sci. 8 (3), 253–261. doi:10.3844/ajessp.2012.253.261
Fytas, K., and Evangelou, B. (1998). Phosphate coating on pyrite to prevent acid mine drainage. Int. J. Surf. Min. Reclam. Environ. 12 (3), 101–104. doi:10.1080/09208118908944031
Gallegos-Garcia, M., Ramírez-Muñiz, K., and Song, S. (2012). Arsenic removal from water by adsorption using iron oxide minerals as adsorbents: a review. Mineral Process. Extr. Metallurgy Rev. 33 (5), 301–315. doi:10.1080/08827508.2011.584219
Gamble, A. V., Givens, A. K., and Sparks, D. L. (2018). Arsenic speciation and availability in orchard soils historically contaminated with lead arsenate. J. Environ. Qual. 47 (1), 121–128. doi:10.2134/jeq2017.07.0264
Han, F. X., Banin, A., Su, Y., Monts, D. L., Plodinec, J. M., Kingery, W. L., et al. (2002). Industrial age anthropogenic inputs of heavy metals into the pedosphere. Naturwissenschaften 89 (11), 497–504. doi:10.1007/s00114-002-0373-4
Hughes, M. F. (2002). Arsenic toxicity and potential mechanisms of action. Toxicol. Lett. 133 (1), 1–16. doi:10.1016/s0378-4274(02)00084-x
Kaur, S., Kamli, M. R., and Ali, A. (2011). Role of arsenic and its resistance in nature. Can. J. Microbiol. 57 (10), 769–774. doi:10.1139/w11-062
Kim, H. C., Lee, J. U., Roh, Y., and Shim, Y. S. (2011). Column experiments on removal of dissolved arsenic using microorganism and nano-sized pd-akaganeite particles. J. of Korean Society for Geosystem Engineering. 48. 613-622 .
Kim, H. N., and Park, J. H. (2023). Concurrent sorption of antimony and lead by iron phosphate and its possible application for multi-oxyanion contaminated soil. Environ. Sci. Pollut. Res. 30 (9), 22835–22842. doi:10.1007/s11356-022-23800-4
Kim, Y. J., Choi, S. Y., and Kim, Y. H. (2019). Synthesis of iron oxide and adsorption of arsenic on iron oxide. J. Environ. Sci. Int. 28 (1), 99–106. doi:10.5322/jesi.2019.28.1.99
Kocabaş-Ataklı, Z. Ö., and Yürüm, Y. (2013). Synthesis and characterization of anatase nanoadsorbent and application in removal of lead, copper and arsenic from water. Chem. Eng. J. 225, 625–635. doi:10.1016/j.cej.2013.03.106
Lambkin, D. C., and Alloway, B. J. (2003). Arsenate-induced phosphate release from soils and its effect on plant phosphorus. Water, Air, Soil Pollut. 144, 41–56. doi:10.1023/a:1022949015848
Lenoble, V., Laclautre, C., Deluchat, V., Serpaud, B., and Bollinger, J. C. (2005). Arsenic removal by adsorption on iron(III) phosphate. J. Hazard. Mater. 123 (1-3), 262–268. doi:10.1016/j.jhazmat.2005.04.005
Li, H. B., Chen, X. Q., Wang, J. Y., Li, M. Y., Zhao, D., Luo, X. S., et al. (2019). Antagonistic interactions between arsenic, lead, and cadmium in the mouse gastrointestinal tract and their influences on metal relative bioavailability in contaminated soils. Environ. Sci. Technol. 53 (24), 14264–14272. doi:10.1021/acs.est.9b03656
Liang, Y., Cao, X., Zhao, L., and Arellano, E. (2014). Biochar-and phosphate-induced immobilization of heavy metals in contaminated soil and water: implication on simultaneous remediation of contaminated soil and groundwater. Environ. Sci. Pollut. Res. 21 (6), 4665–4674. doi:10.1007/s11356-013-2423-1
Liu, H., Wei, Y., Sun, Y., and Wei, W. (2005). Dependence of the mechanism of phase transformation of Fe (III) hydroxide on pH. Colloids Surfaces A Physicochem. Eng. Aspects 252 (2-3), 201–205. doi:10.1016/j.colsurfa.2004.10.105
Mamindy-Pajany, Y., Hurel, C., Marmier, N., and Roméo, M. (2011). Arsenic (V) adsorption from aqueous solution onto goethite, hematite, magnetite and zero-valent iron: effects of pH, concentration and reversibility. Desalination 281, 93–99. doi:10.1016/j.desal.2011.07.046
Martin, M. H. (2012). Biological monitoring of heavy metal pollution: land and air. Berlin, Germany Springer Science & Business Media.
Moon, D. H., Dermatas, D., and Menounou, N. (2004). Arsenic immobilization by calcium–arsenic precipitates in lime treated soils. Sci. Total Environ. 330 (1-3), 171–185. doi:10.1016/j.scitotenv.2004.03.016
Nagajyoti, P. C., Lee, K. D., and Sreekanth, T. V. M. (2010). Heavy metals, occurrence and toxicity for plants: a review. Environ. Chem. Lett. 8 (3), 199–216. doi:10.1007/s10311-010-0297-8
Olsen, S. R., and Watanabe, F. S. (1957). A method to determine a phosphorus adsorption maximum of soils as measured by the Langmuir isotherm. Soil Sci. Soc. Am. J. 21 (2), 144–149. doi:10.2136/sssaj1957.03615995002100020004x
Oremland, R. S., and Stolz, J. F. (2003). The ecology of arsenic. Science 300 (5621), 939–944. doi:10.1126/science.1081903
Park, J. H., Han, Y. S., and Ahn, J. S. (2016). Comparison of arsenic co-precipitation and adsorption by iron minerals and the mechanism of arsenic natural attenuation in a mine stream. Water Res. 106, 295–303. doi:10.1016/j.watres.2016.10.006
Payne, K. B., and Abdel-Fattah, T. M. (2004). Adsorption of divalent lead ions by zeolites and activated carbon: effects of pH, temperature, and ionic strength. J. Environ. Sci. Health, Part A 39 (9), 2275–2291. doi:10.1081/lesa-200026265
Priya, V. N., Rajkumar, M., Mobika, J., and Sibi, S. L. (2022). Adsorption of as (v) ions from aqueous solution by carboxymethyl cellulose incorporated layered double hydroxide/reduced graphene oxide nanocomposites: isotherm and kinetic studies. Environmental Technology & Innovation. 26 102268. doi:10.1016/j.eti.2022.102268
Raven, K. P., Jain, A., and Loeppert, R. H. (1998). Arsenite and arsenate adsorption on ferrihydrite: kinetics, equilibrium, and adsorption envelopes. Environ. Sci. Technol. 32 (3), 344–349. doi:10.1021/es970421p
Sayadi, M. H. (2014). Impact of land use on the distribution of toxic metals in surface soils in Birjand city, Iran. Proc. Int. Acad. Ecol. Environ. Sci. 4 (1), 18. doi:10.5277/epe180301
Sazakli, E., Zouvelou, S. V., Kalavrouziotis, I., and Leotsinidis, M. (2015). Arsenic and antimony removal from drinking water by adsorption on granular ferric oxide. Water Sci. Technol. 71 (4), 622–629. doi:10.2166/wst.2014.460
Schock, M. R., Scheckel, K. G., DeSantis, M., and Gerke, T. L. (2005). Mode of occurrence, treatment, and monitoring significance of tetravalent lead. Proc. 2005 AWWA WQTC, Quebec City, Quebec, 1–15.
Sharma, V. K., Filip, J., Zboril, R., and Varma, R. S. (2015). Natural inorganic nanoparticles–formation, fate, and toxicity in the environment. Chem. Soc. Rev. 44 (23), 8410–8423. doi:10.1039/c5cs00236b
Smedley, P. L., and Kinniburgh, D. G. (2002). A review of the source, behaviour and distribution of arsenic in natural waters. Appl. Geochem. 17 (5), 517–568. doi:10.1016/s0883-2927(02)00018-5
Soares, C. R., and Siqueira, J. O. (2008). Mycorrhiza and phosphate protection of tropical grass species against heavy metal toxicity in multi-contaminated soil. Biol. Fertil. Soils 44 (6), 833–841. doi:10.1007/s00374-007-0265-z
Stollenwerk, K. G. (2003). “Geochemical processes controlling transport of arsenic in groundwater: a review of adsorption,” in Arsenic in ground water Berlin, Germany (Springer), 67–100.
Strawn, D. G. (2018). Review of interactions between phosphorus and arsenic in soils from four case studies. Geochem. Trans. 19 (1), 10–13. doi:10.1186/s12932-018-0055-6
Sundar, S., and Chakravarty, J. (2010). Antimony toxicity. Int. J. Environ. Res. Public Health 7 (12), 4267–4277. doi:10.3390/ijerph7124267
Tabelin, C. B., Corpuz, R. D., Igarashi, T., Villacorte-Tabelin, M., Alorro, R. D., Yoo, K., et al. (2020). Acid mine drainage formation and arsenic mobility under strongly acidic conditions: importance of soluble phases, iron oxyhydroxides/oxides and nature of oxidation layer on pyrite. J. Hazard. Mater. 399, 122844. doi:10.1016/j.jhazmat.2020.122844
Taghizadeh-Toosi, A., Clough, T. J., Sherlock, R. R., and Condron, L. M. (2012). Biochar adsorbed ammonia is bioavailable. Plant soil 350 (1), 57–69. doi:10.1007/s11104-011-0870-3
Tawfik, D. S., and Viola, R. E. (2011). Arsenate replacing phosphate: alternative life chemistries and ion promiscuity. Biochemistry 50 (7), 1128–1134. doi:10.1021/bi200002a
Theodoratos, P., Papassiopi, N., and Xenidis, A. (2002). Evaluation of monobasic calcium phosphate for the immobilization of heavy metals in contaminated soils from Lavrion. J. Hazard. Mater. 94 (2), 135–146. doi:10.1016/s0304-3894(02)00061-4
Tiwari, S. K., and Pandey, V. K. (2013). Removal of arsenic from drinking water by precipitation and adsorption or cementation: an environmental prospective. Recent Res. Sci. Technol. 5 (5), 88–91.
Walkley, A., and Black, I. A. (1934). An examination of the Degtjareff method for determining soil organic matter, and a proposed modification of the chromic acid titration method. Soil Sci. 37 (1), 29–38. doi:10.1097/00010694-193401000-00003
Wang, Y. M., Chen, T. C., Yeh, K. J., and Shue, M. F. (2001). Stabilization of an elevated heavy metal contaminated site. J. Hazard. Mater. 88 (1), 63–74. doi:10.1016/s0304-3894(01)00289-8
Weng, L., Vega, F. A., and Van Riemsdijk, W. H. (2011). Competitive and synergistic effects in pH dependent phosphate adsorption in soils: LCD modeling. Environ. Sci. Technol. 45 (19), 8420–8428. doi:10.1021/es201844d
Yang, J., Guo, Z., Jiang, L., Sarkodie, E. K., Li, K., Shi, J., et al. (2022). Cadmium, lead and arsenic contamination in an abandoned nonferrous metal smelting site in southern China: chemical speciation and mobility. Ecotoxicol. Environ. Saf. 239, 113617. doi:10.1016/j.ecoenv.2022.113617
Yu, W. H., Harvey, C. M., and Harvey, C. F. (2003). Arsenic in groundwater in Bangladesh: a geostatistical and epidemiological framework for evaluating health effects and potential remedies. Water Resour. Res. 39 (6), 1146–1163. doi:10.1029/2002wr001327
Yuan, Y., Chai, L., Yang, Z., and Yang, W. (2017). Simultaneous immobilization of lead, cadmium, and arsenic in combined contaminated soil with iron hydroxyl phosphate. J. Soils Sediments 17 (2), 432–439. doi:10.1007/s11368-016-1540-0
Zhang, T., Zeng, X., Zhang, H., Lin, Q., Su, S., Wang, Y., et al. (2019). The effect of the ferrihydrite dissolution/transformation process on mobility of arsenic in soils: investigated by coupling a two-step sequential extraction with the diffusive gradient in the thin films (DGT) technique. Geoderma 352, 22–32. doi:10.1016/j.geoderma.2019.05.042
Zhao, Z., Meng, Y., Yuan, Q., Wang, Y., Lin, L., Liu, W., et al. (2021). Microbial mobilization of arsenic from iron-bearing clay mineral through iron, arsenate, and simultaneous iron-arsenate reduction pathways. Sci. Total Environ. 763, 144613. doi:10.1016/j.scitotenv.2020.144613
Keywords: adsorption, precipitation, stabilization, oxyanions, metals
Citation: Kim HN and Park JH (2024) Simultaneous removal of arsenic and lead by iron phosphate and its potential for immobilization in mixed-contaminated soil. Front. Environ. Sci. 12:1358561. doi: 10.3389/fenvs.2024.1358561
Received: 20 December 2023; Accepted: 11 March 2024;
Published: 18 April 2024.
Edited by:
Qi Liao, Central South University, ChinaReviewed by:
Ksenija Jakovljevic, University of Belgrade, SerbiaSudipta Rakshit, Tennessee State University, United States
Copyright © 2024 Kim and Park. This is an open-access article distributed under the terms of the Creative Commons Attribution License (CC BY). The use, distribution or reproduction in other forums is permitted, provided the original author(s) and the copyright owner(s) are credited and that the original publication in this journal is cited, in accordance with accepted academic practice. No use, distribution or reproduction is permitted which does not comply with these terms.
*Correspondence: Jin Hee Park, cGppbmhAY2h1bmdidWsuYWMua3I=