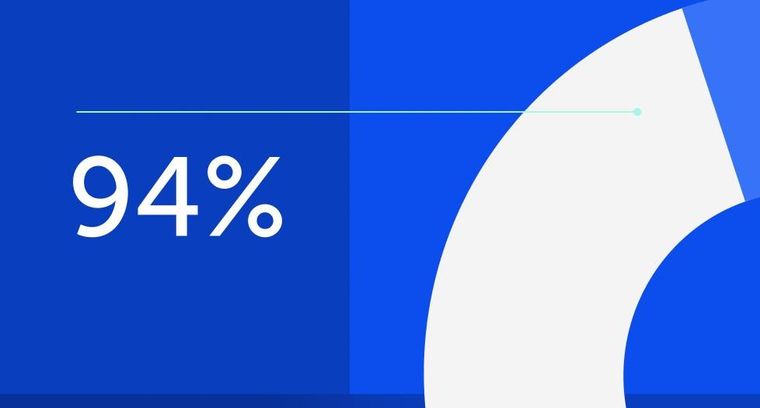
94% of researchers rate our articles as excellent or good
Learn more about the work of our research integrity team to safeguard the quality of each article we publish.
Find out more
ORIGINAL RESEARCH article
Front. Environ. Sci., 29 February 2024
Sec. Toxicology, Pollution and the Environment
Volume 12 - 2024 | https://doi.org/10.3389/fenvs.2024.1310310
This article is part of the Research TopicTextile Fiber Pollution from Source to SinkView all 6 articles
Microplastics are found in agricultural soils worldwide; however, little is known about the impacts of this ubiquitous pollutant on the growth and development of crops. Microfibres are one of the dominant microplastic types found in agricultural soils. Sources of microfibres in the agricultural environment are multiple, including soil amendment applications, wastewater irrigation and atmospheric deposition, with agricultural soils subsequently acting as an accumulating sink for plastics. A key consideration in an agricultural setting is yield; the seed yield is critical, as this is the part with economic value. This study investigates the influence of polyester microfibres, a prevalent type of microplastic in agricultural soils, on the growth and seed yield of Sinapis alba (white mustard). Polyester microfibres were added to soil samples at concentrations of 0.1% and 1% w/w, simulating environmental exposure levels. The study evaluated flower production, seed yield, pod-to-seed ratio, and chlorophyll fluorescence as indicators of plant health and reproductive success. Results revealed significant changes in chlorophyll fluorescence values (Fv/Fm), reduced flower production control (74 ± 37 flowers in the control, 31 ± 27 in the 0.1 treatment and 44 ± 31 in the 1 % treatment), and changes in pod-to-seed ratio (3.5 ± 0.65 seeds per pod in the control, 2.76 ± 0.5 seeds per pod in the 0.1% treatment and 2.83 ± 0.81 seeds per pod in the 1% treatment) in response to polyester microfibre exposure. Polyester microfibres were demonstrated to act as a stressor to S. alba, changing the chlorophyll fluorescence values, reducing the flower number, and in turn, reducing the pod-to-seed ratio. This study provides evidence that microfibres could reduce seed yield but that further research is required to elucidate the mechanisms by which these changes are occurring.
Plastic is an almost ubiquitous manufacturing material, providing significant benefits to many industries (Lusher et al., 2017; Rochman, 2018; De Sousa Machado, 2021); despite the societal benefits being vast, there are no doubts that management of plastic waste and byproducts has developed into a considerable environmental problem (Ilyas et al., 2018). Microplastics are defined as being smaller than 5 mm in size and may be primary microplastics manufactured in this size range (Auta et al., 2017) or secondary, formed via degradation from larger macroplastics (Cole et al., 2011). Research into microplastics has been extensively conducted in the marine environment (Cole et al., 2013; Auta et al., 2017; Sharma and Chatterjee, 2017; Barboza et al., 2018), and studies have more recently explored terrestrial and soil systems (Corradini et al., 2019; Yu et al., 2021; Sajjad et al., 2022; Kedzierski et al., 2023), with excellent progress in the understanding of sources and fate of microplastics. Agricultural soils are recognized as having the potential to be a major sink for microplastics (Nizzetto et al., 2016; Büks and Kaupenjohann, 2020), with estimates suggesting there are between 1.5 and 6.6 million tons of microplastics in agricultural soils globally (Kedzierski et al., 2023).
Microplastic fibres (microfibres) are a prevalent type of microplastic pollution, ubiquitous in aquatic and terrestrial environments (Acharya, 2021). Sources of microfibres are multiple, including being released from textile garments during domestic laundering (Hernandez et al., 2017), cigarette butts and fishing nets (Mishra et al., 2020). In the marine environment, microfibres comprise around 91% of all surface water pollution (Barrows et al., 2018). In soils, the use of biosolids may lead to increased accumulation of microfibres, which have been found to account for 97% of the plastics found in agricultural soils with biosolid application (Corradini et al., 2019). Given the prevalence of microfibres in the environment, research has increasingly begun to investigate the effects of this pollutant in a range of environments (Gago et al., 2018; Lozano and Rillig, 2020; Acharya, 2021).
The existing studies have shown that plastic microfibres in soils cause changes to soil properties (De Souza Machado et al., 2019; Kwak et al., 2022) and microbial communities (Guo et al., 2021; Liang et al., 2021; Zeb et al., 2022). The addition of microfibres in soil impacts the bulk density (De Souza Machado et al., 2019; Liang et al., 2021), the total porosity (Selonen et al., 2020) and the hydraulic conductivity of soil (De Souza Machado et al., 2019; Zhang et al., 2019), which can affect the root growth of plants and can have knock-on effects on the uptake of nutrients and water (De Souza Machado et al., 2019; Lozano and Rillig, 2020). Changes in nutrient and water availability can ultimately lead to plant stress (defined as external conditions that adversely affect plants’ development and productivity), which impacts survival and reproduction (Shao et al., 2008; Jia, 2023). These factors may mean that microfibres can act as abiotic stressors to plants (Ullah et al., 2021) potentially leading to negative effects on agriculture and food security; thus, an understanding of how microfibres may act as stressors to plants is of key importance.
The germination and reproductive stages of plant development are more sensitive to stress than the vegetative phase (Zinn et al., 2010); in the reproductive stage, stress can result in fewer flowers or decreased seed numbers (Rering et al., 2020). Fertilisation, gametogenesis and embryogenesis are impacted, limiting seed development and lowering crop yields (Begcy and Dresselhaus, 2018). Both biotic and abiotic stresses have been noted to interfere with several key reproductive processes, such as germination, vegetative growth, tiller production, reproductive organ development, reproduction and grain filling (Sehgal et al., 2019). Abiotic stressors such as drought and heat have been noted to cause decreases in seed yield in legumes (Farooq et al., 2017), cereal crops (Dias and Lidon, 2009), and in oil seed rape and in oil seed rape (Hatzig et al., 2018). Stress has been noted to cause changes to photosynthetic tissues, leading to the inhibition of photosynthesis. Stressors such as high temperature have been demonstrated to damage the oxygen-evolving complex of photosystem II (PSII), resulting in disorganisation of the thylakoid membranes (Yamashita et al., 2008). A reduction in photosynthesis ultimately reduces parental resources available for reproduction, subsequently impacting seed yield (Zinn et al., 2010).
Sinapis alba is a cool season crop (being able to tolerate cold temperatures (Baumbauer and Burgess, 2020), with seed maturity being obtained within 80–90 days (Kokotkiewicz and Luczkiewicz, 2015). It is primarily grown in agriculture for the seeds of the crop, which are used in the condiment industry and as a feedstock for biodiesel production (Mitrović et al., 2020). S. alba is cultivated on 60,000–80,000 ha annually, producing 685,000 t of seed (Mitrović et al., 2020). The species is insect and wind-pollinated (Hemingway, 1976) and is a non-mycorrhizal plant which tends to grow in moderately fertile soils (Lambers and Teste, 2013). Non-mycorrhizal plants have evolved specialist root structures which make them capable of extracting all of the required nutrients from soils without the use of mycorrhiza, using a carboxylate-relating phosphorus mining strategy to enable adequate phosphorus for development (Shane and Lambers, 2005). Due to the fact that S. alba has a short cropping cycle and can be grown in a range of soils, it is now a widespread commercial crop globally (Ekanayake et al., 2016). S. alba goes through nine life cycle stages (germination, leaf development, side shoot development, stem elongation, vegetable plant development, flowering, fruit development, ripening and senescence) (Saskatchewan Mustard Commission, 2019). In the current experiment, the S. alba were grown through to senescence to investigate the effects on the full life cycle of the plants, particularly reviewing the effects on seed yield, as with oilseed crops, which is the most important part of the crop.
This study aims investigated the impacts of polyester microfibres on the yield of the oilseed plant S. alba reviewing any potential changes to the reproductive cycle of the plant. It is hypothesised that microfibres may result in plant stress, resulting in changes to the reproductive capacity of the mustard plants. The current study was conducted to assess whether microfibres could result in changes to the reproductive stage of plant development, a key outcome for agriculture. In this study, mustard was grown in soils contaminated with polyester microfibres to assess whether changes occurred to seed number and seed-to-pod ratio.
Three treatments were conducted for this experiment, a control, 0.1% w/w and 1% w/w polyester fibres. Polyester fibres were sourced from The Flocking shop (England); fibres were measured using a Leica stereo microscope (DM2500P) with a magnification of ×100 and photographed using a Leica Pixel Shift Camera (DMC6200). Measurements were calculated using Leica application suite X (version 3.0.14.23224); the fibres had an average length of 519.34 ± 66.67 μm (min = 402.83 μm, max = 1016.02 μm, n = 100) and an average thickness of 15.5 ± 3.69 μm (min = 8.82 μm, max = 27.35 μm, n = 100).
To negate any losses from germinating plants in the plastic-contaminated soil, seeds were germinated in trays with cotton wool and water and planted once the first leaves had appeared, using metal tweezers to transfer them to pots. A total of 200 S. alba seedlings were planted per treatment (with one seed per pot).
Seedlings were planted into polythene plastic pots (Elixir Garden supplies, England; diameter 11 cm, depth 12 cm) with a volume of 1 L. The plant pots were filled with 500 g of John Innes compost No.2 (Westland, UK–pH 6.5). One seedling was planted per pot in one of three concentrations: 0 (control), 0.1% (0.5 g w/w of polyester fibres) or 1% (5 g w/w of plastic fibres) after germinating in cotton wool, giving a total of 200 pots per treatment. The concentrations of polyester microfibres were similar to those which reported noticeable changes in plant responses (Yu et al., 2021; Jia, 2023; Mészáros et al., 2023). As plastic pollution continues, investigating high concentrations could help to represent future scenarios (Ingraffia, Amato, Iovino, et al., 2022). For each pot, the appropriate amount of polyester microfibers for the treatment was weighed and homogenised into the 500 g of soil, the pots were stirred using a clean glass rod and manually shaken; 10 mL of water was added to help homogenise the plastic and soil. As noted by Machado (De Souza Machado et al., 2019), due to the density of the fibres, true homogenisation of plastics into the soil is difficult but was achieved after approximately 5 min of stirring per pot.
Seedlings were transplanted using tweezers into each pot. Plants were kept thoroughly watered throughout the experimental period, with plants being bottom watered by filling the trays with 5 L twice per week. To mitigate the shared effects of planting multiple plants per pot, plants were potted individually. Pots were then placed into trays, which contained 24 pots of one of the treatments (0%, 0.1% or 1%), to avoid any leachates passing to controls or lower concentrations. Therefore, it is not possible to rule out a tray-based effect, however, tray positions were shifted monthly to mitigate a location-based effect. Plants were grown under natural light conditions between April and August, with an average greenhouse temperature of 23.5°C ± 9°C. During the flowering period, fans were placed on timers for 4 hours per day to enable wind pollination of the plants. Due to the location of the greenhouses, it was not possible to encourage insect pollination.
Chlorophyll fluorescence is a measurement to gain detailed information on the state of photosystem II (PSII) (Murchie and Lawson, 2013), which is the protein super complex that executes the initial reaction of photosynthesis in higher plants (Coe et al., 2015). Chlorophyll fluorescence measurements are commonly applied in plant stress studies, providing rapid insight into the PSII system (Reiling and Davison, 1992; Maxwell and Johnson, 2000). Chlorophyll fluorescence of PSII was recorded every 2 weeks using a modulated fluorescence system. Thirty fully expanded leaves were selected from thirty plants (one leaf per plant) for each treatment (control, 0.1% w/w, and 1% w/w), and fast fluorescence kinetics were recorded after a 20-min dark adaption period using an Opti-Sciences OS1P chlorophyll fluorometer and dark adaption clips (Opti-Sciences, Inc, Hudson, NH, USA). The dark adaption phase is needed to reverse non-photochemical quenching fluorescence before fluorescence can be measured (Maxwell and Johnson, 2000). In the dark-adapted state, it is possible to measure initial fluorescence (Fo) when all PSII reaction centres are open. Maximal fluorescence (Fm), when all PSII reaction centres are closed, is then assessed with saturating light (Murchie and Lawson, 2013). Fv is variable fluorescence which is the rise from the Fo to the Fm measurement (Ritchie, 2006). The maximal quantum yield for electron transport by open PSII centres is calculated as Fv/Fm = (Fm–Fo)/Fm (Murchie and Lawson, 2013). The optimal Fv/Fm measurements in most plant species are between 0.79–0.83, with significantly lower values indicating photoinhibition, which can indicate stress (Maxwell and Johnson, 2000). Fv/Fo measurements can be used to assess the number and size of the active photosynthetic reaction centres, and changes to this can indicate a change in the rate of electron transport from PSII to the primary electron acceptors. This has been reported in plants exposed to different environmental stressors (Kumar et al., 2020).
Before the experiment, a preliminary study was performed to determine the optimal protocol for chlorophyll fluorescence image acquisition; the dark-adapted time was determined by checking the value of maximum PSII. It was observed that after a 20-min dark adaption period, the Fv/Fm values were stable, which was considered the optimal dark adaption period.
The number of flowers were measured from the first flower emergence at 8 weeks for S. alba until senescence had occurred. A maximum of thirty flowering plants per treatment were counted for their number of flowers, and the total number of flowering plants was also recorded for each treatment. A random number generator app was used to determine which flowering plants were counted for their flower number, with plants randomly selected based on the number generated to measure.
Seeds were harvested once the plant had gone through senescence and the pods had turned brown. The seed pods from thirty plants in each treatment were harvested, with the pods for each plant added to a separate envelope. The thirty plants harvested were determined by a random number generator app (Random Number Generator Plus). Subsequently, each of the 30 selected plants total number of pods per plant was recorded, along with the number of seeds per pod and the individual seed weight. Finally, the seeds were weighed using Mettler Toledo, Pl303.
After the seed pods were harvested, plants were removed from their individual pots, the shoots were measured using a measuring tape, and the lengths of the individual plant were recorded. Root lengths were not recorded in this study because once plants had gone through senescence, the root system was extremely fragile; thus, accurate measurement was not possible.
Statistical analysis was conducted in R version 4.1.2 (R Core Team, 2022), and data were screened for normality using s Shapiro Wilk test and homogeneity of variance using Levene’s test from the car package v3.0.12 (Fox and Weisberg, 2021). Differences in Fv/Fm measurements and Fv/Fo of thirty plants, and the shoot height measurements of all surviving plants were analysed at the final time period using a Kruskal Wallis test considering the differences between concentrations. The number of plants flowering and the number of flowers at the full flowering stage were analysed using a Kruskal Wallis test investigating the difference between concentrations. Where significance was demonstrated, a Dunn’s post hoc test was used to further explore the responses using the dunn. test package v1.3.5 (Dinno, 2017) with an alpha value of 0.05.
The pod-to-seed ratio, mean individual seed weight, number of pods and number of seeds of thirty plants were analysed using a one-way ANOVA, and where significance was demonstrated, a Tukey’s HSD test was used to test differences in the response variable versus the concentration. The pod number and seed number were tested using a Kruskal Wallis test with a Dunn’s post hoc test with an alpha value of 0.05. Data were visualised using ggplot2 (Wickham, 2016) and displayed as the mean ± the standard error of the mean.
A generalized estimating equation (GEE) with a gamma family was used to analyse Fv/Fm, Fv/Fo and flower number by concentration over time using the package geepack v1.3.9 (Halekoh et al., 2006). GEE was used to account for the correlated nature of the data due to the repeated measures, and an exchangeable correlation structure was assumed. GEE also enables an estimate of population-averaged effects, which can be interpreted as the average effect of the concentrations on the selected variables. A gamma family was selected due to the over-dispersed nature of the results, which meant the data did not conform to the assumptions for Poisson distribution.
There was a statistically significant difference in Fv/Fm measurements for S. alba at the final timepoint (χ2 (2) = 7.62, p = 0.02, n = 30), with the 1% treatment being significantly different to both the control (p = 0.007) and the 0.1% (p = 0.011). No significant difference was demonstrated between the control and the 0.1% treatments (p = 0.432) (see Figure 1). On the final week of measurement (week 16), the Fv/Fm mean measurements for the 1% treatment were 0.741 ± 0.061, compared to the control (0.772 ± 0.052) and the 0.1% (0.773 ± 0.036) (see Figure 2). The results of the GEE model showed significant estimates for S. alba for the concentration (estimate = 0.019, standard error = 0.0009, Wald = 4.35, p = 0.037) and time (estimate = 0.007, standard error = 0.002, Wald = 9.94, p = 0.002). The scale parameter for the model was (estimate = 0.0029, standard error = 0.0006).
FIGURE 1. The Fv/Fm values of thirty leaves from thirty different S. alba plants across three concentrations of polyester microfibres (0% w/w represented by , 0.1% w/w by
, and 1% w/w by
) at various time points, measured bi-weekly. Data are presented as the mean ± standard error of the mean, with n = 30. Jitter has been incorporated at each sampling point to enhance readability.
FIGURE 2. The Fv/Fo values of thirty leaves from different Sinapis alba plants across the three concentrations of polyester microfibres (0% w/w represented by , 0.1% w/w by
, and 1% w/w by
) at different time periods, measured every 2 weeks. Data are displayed as the mean ± the standard error of the mean, n = 30. Jitter has been added to each sampling point to ease readability.
The Fv/Fo measurements demonstrated a significant difference (χ2 (2) = 6.27, p = 0.044, n = 30), and Dunn’s post hoc test indicated that the 1% treatment (mean = 3.07 ± 0.9) was significantly different from the control (p = 0.015, mean = 3.56 ± 1.05). However, there were no significant differences between the 0.1% (mean = 3.49 ± 0.67) and the control (p = 0.49) (see Figure 2). The results of the GEE model showed significant estimates for S. alba for the concentration (estimate = 0.015, standard error = 0.007, Wald = 4.47, p = 0.03) and for time (estimate = 0.0056, standard error = 0.002, Wald = 7.24, p = 0.0071). The scale parameter for the model was (estimate = 0.04, standard error = 0.0085).
There were no statistically significant differences in the total number of plants flowering (χ2 (2) = 0.05, p = 0.98, n = 30) as determined by a Kruskal Wallis test. However, at week 11, when plants were in the full flowering stage (defined as 50% of the flowers on the main raceme open (Sasketchewan Mustard Development Commission, 2019), the control had a total of 104 plants flowering, the 0.1% had 84 plants in flower and the 1% had 100 plants flowering (see supporting information Figure 3).
FIGURE 3. The total number of flowering Sinapis alba plants measured weekly. The three concentrations of polyester microfibres are represented as follows; 0% w/w represented by , 0.1% w/w by
, and 1% w/w by
. Jitter has been added to each sampling point to ease readability.
There was a statistically significant difference between the number of flowers produced between treatments at week 11 (Full flowering) (χ2 (2) = 15.526, p = 0.0005) as determined by a Kruskal Wallis test (see Figure 4). The 0.1% and the 1% treatments were significantly different compared to the control (p = 0.0009, p < 0.0001, respectively), but there were no significant differences between the 0.1% and the 1% treatments (p = 0.3). The flowers reached the full flowering stage as of week 11, where the control had an average of 74 ± 37 flowers. The control flower number was 43% higher than the 0.1% treatment (31 ± 27 flowers) and 30% higher than the 1% treatment (44 ± 31 flowers). The control and the 0.1% treatments began flowering as of week eight, but the 1% treatment did not begin until 1 week later, in week nine. The GEE model found that concentration was the only factor that had a significant negative effect on flower numbers (estimate = −0.25, standard error = 0.043, p < 0.001). The estimated scale parameter was 26.4 (standard error = 5.4), and an estimated correlation parameter of 0.245 (standard error = 0.1).
FIGURE 4. The average flower number of thirty Sinapis alba plants, measured weekly, Data are displayed as the mean ± the standard error of the mean, n = 30 plants. The three concentrations of polyester microfibres are represented as follows; 0% w/w represented by , 0.1% w/w by
, and 1% w/w by
. A jitter has been added to each sampling point to ease readability.
There were no statistically significant differences in shoot height for S. alba as determined by a Kruskal Wallis test (χ2 (2) = 2.205, p = 0.3). The mean shoot height for the control was 209 ± 61 cm, the 0.1% w/w 198 ± 45 cm, and the 1% w/w treatment 203 ± 51 cm (see supporting information Figure 5).
FIGURE 5. displays the shoot heights of Sinapis alba grown in the three treatments (% w/w polyester microfibres), n = 30 plants. Each box represents the interquartile range (IQR) of the data, with the median indicated by the bold horizontal line. Whiskers extend to 1.5 times the IQR, and individual data points beyond this range are plotted as circles.
There were no significant differences in the number of pods per plant for either the 0.1 or 1% treatments compared to the control (χ2 (2) = 3.346, p = 0.19) for the S. alba; however, the average number of pods per plant was higher in the control (mean = 120 ± SD = 100 pods), in the 0.1% the average pod number was 22% lower (0.1%, mean = 94 ± SD = 113) and 19% lower in the 1% treatments (1%, mean = 97 ± SD = 103 pods per plant) (see supporting information Figure 6). No significant differences were demonstrated in the total seed number (χ2 (2) = 5.579, p = 0.06); however, the average number of seeds was higher for the control (432 ± 371 seeds), the 0.1% was 32% lower for the average seed number (294 ± 403 seeds) and 29% lower in the 1% treatment (308 ± 354 seeds) (see supporting information Figure 7).
FIGURE 6. Displays the total pod numbers of Sinapis alba grown in the three treatments (% w/w polyester microfibres), n = 30 plants. Each box represents the interquartile range (IQR) of the data, with the median indicated by the bold horizontal line. Whiskers extend to 1.5 times the IQR, and individual data points beyond this range are plotted as circles. Black points represent outliers within the data.
FIGURE 7. Displays the total seed numbers for Sinapis alba in the three treatments (% w/w polyester microfibres), n = 30 plants. Each box represents the interquartile range (IQR) of the data, with the median indicated by the bold horizontal line. Whiskers extend to 1.5 times the IQR, and individual data points beyond this range are plotted as circles.
There was a significant difference in the seed-to-pod ratio (F (2,87) = 11.25, p < 0.0001) (see Figure 8), with the control having an average of 3.5 ± 0.65 seeds per pod. The 0.1% seed-to-pod ratio was decreased by 21.15% (2.76 ± 0.5 seeds per pod, p = 0.0001), and the 1% treatment decreased by 19.14% (2.83 ± 0.81 seeds per pod, p = 0.0006). No significant differences were demonstrated between the 0.1% w/w and the 1% w/w treatments (p = 0.9).
FIGURE 8. shows the seed/pod ratio of Sinapis alba grown in the three treatments (% w/w polyester microfibres), n = 30 plants total seed pods collected. Each box represents the interquartile range (IQR) of the data, with the median indicated by the bold horizontal line. Whiskers extend to 1.5 times the IQR, and individual data points beyond this range are plotted as circles.
The per 100 seed weight was not significantly different (F (2, 87) = 0.151, p = 0.86), with the control having an average seed weight of 0.462 ± 0.197 g, the 0.1% 0.482 ± 0.176 g and the 1% 0.485 ± 0.14 g (See supporting information Figure 9).
FIGURE 9. Displays the seed weight per 100 seeds in the three treatments (% w/w polyester microfibres), n = 30 plants. Each box represents the interquartile range (IQR) of the data, with the median indicated by the bold horizontal line. Whiskers extend to 1.5 times the IQR, and individual data points beyond this range are plotted as circles.
Microplastics and microfibres have been extensively incorporated into agricultural landscapes, either intentionally through agricultural practices such as the application of mulch films or through pollution (Huerta-Lwanga et al., 2022). In this study, microfibres have been demonstrated to show impacts on the reproductive phase of S. alba’s life cycle, from flower number to seed-to-pod ratio. The results from the chlorophyll fluorescence testing indicate that the maximum efficiency of PSII was reduced in the 1% treatment. Fv/Fm can indicate stress, photoinhibition, and the downregulation of photosynthesis (Jägerbrand and Kudo, 2016); thus, the lowered values for the 1% treatment indicate that microfibres were acting as an abiotic stressor for S. alba. Research into terrestrial plants has found a reduction in the Fm/Fv values when microplastics are added to soils. Colzi et al. (2022) assessed the impacts of different types of microplastics on the growth of Cucurbita pepo (field pumpkin), finding that PVC and PE induced a significant decrease in Fv/Fm measurements from concentrations of 0.02%, noting that this response was dose dependant. However, in the current research, 0.1% w/w of polyester microfibres in soil did not induce any changes to the Fv/Fm measurements, which could be linked to the type of plastics used, as Colzi et al. (2022) noted that of the four plastics tested, only two (polyethylene and polyvinyl chloride) had impacts at the concentrations tested (0.02, 0.1% and 0.2% w/w).
The study conducted by Gao et al. (2018) clearly demonstrated that exposure to polyethylene microplastics (0.25, 0.5, and 1 mg mL-1, size = ∼ 23 μm) in a hydroponic system caused a significant decrease in Fv/Fm measurements in Lactuca sativa (lettuce) plants. Their results strongly suggest that effective pigments involved in photosynthesis were reduced, and the PSII reaction centres were damaged due to microplastic exposure. However, it is worth noting that the concentrations and sizes of the plastic used in their study were much higher and plastic sizes smaller, than those used in the current study. Gao et al. (2018) suggested that the microplastics attached to the roots of the lettuce caused changes in photosynthetic activity. However, this is unlikely to occur in the current study due to the sizes of the plastics used. For the S. alba in this study, it appears that polyester microfibres impacted the efficacy of photosystem II at the highest concentration. This study and the ones discussed above demonstrate that microplastics can act as a stressor on plants, causing effects on PSII. Changes to PSII change nutrient availability for plants; thus, this will likely impact the nutrient availability for seed production, which could help to explain the impacts on the pod-to-seed ratio demonstrated in this study. Teng et al. (2022) found that Nicotiana tabacum (tobacco) seedlings exposed to low-density polyethylene at concentrations of 0.1% had reduced photosynthetic activity after 48 days of culture. The authors suggested that when the microplastics were too large to be absorbed by the plants, indirect factors such as changes to soil properties or reduced nutrient transport could explain the changes demonstrated to the photosynthetic performance. Additionally, the authors suggested that accumulation of reactive oxygen species, inhibition of leaf pigment synthesis and the prevention of electron transport between PSII and PSI occurred when exposed to polyethylene microplastics. Wu et al. (2019) hypothesised that stress from nanoplastics slows down the PSII electron transport rate, resulting in a build-up of electrons which amplifies photoinhibition resulting in a rise in reactive oxygen species. Thus, similar interactions could be occurring for S. alba, though further testing would be needed to confirm whether this is the mechanism by which changes to PSII are occurring. It is considered here that changes to the soil properties could have resulted in the changes to PSII, by altering nutrient availability, however, further research is needed to confirm this.
Shoot lengths were not significantly different for the S. alba grown in the different treatments, which concurs with research by Qi et al. (2018), who found no significant differences in shoot height for Triticum aestivum (wheat) grown in soil contaminated with LDPE microplastic films harvested at 4 months. Ohashi et al. (2009) found that although seed parameters for Glycine max (soybean) were changed during drought stress, such as decreased pod thickness, no significant differences were demonstrated in stem growth. Due to the difficulties in measuring the roots post senescence, root measurements were not taken during this study, however, research has suggested that changes to rooting structures do occur (Bosker et al., 2019; Lozano and Rillig, 2020). However, this was not measured in the current study.
When considering floral development, the total flower number for S. alba was significantly different when comparing those grown in plastic-contaminated soils versus the control, with a decrease of 43% and 40% for the 0.1% and 1% treatments. There were, however, no significant changes to the total number of flowering plants. Abiotic stresses can cause floral bud abortion and reduce the flower number, leading to decreased reproductive success (Smith and Zhao, 2016). Stress-related changes can also result in reductions in nectar production, which can result in changes to plant-pollinator interactions (Descamps et al., 2021). Reduced flower numbers have been demonstrated when considering other stressors, such as drought and temperature stress. Su et al. (2013) found that drought stress reduced flower number in Arabidopsis thailiana (Thale cress), and Descamps et al. (2018) found similar results when Borago officinalis (borage) were grown in drought conditions, demonstrated that stress responses can result in reduced flower numbers. Barnabás et al. (2008) suggested that stressful environments limit cell division in the meristem and reduce the transport of nutrients to different plant tissues, thus changing overall resource partitioning, which could explain the reduction in flower number. Another strategy that Descamps et al. (2021) suggested is that plants may sacrifice young floral buds and reduce new bud formation to enable nutrients to support the continued development of older flowers and immature seeds. Further research is needed to understand the mechanisms that plants employ in microplastic contaminated soils, which results in the reduction of the total flower number. Nevertheless, it is clear that microfibres are acting as a stressor on S. alba plants, as demonstrated by the changes to the PSII and flower number.
The reduction in flower number is likely to have further impacts on total yield (Pang et al., 2017), which correlates with the results of this study finding a reduction in seed-to-pod ratio. Additionally, although not statistically significant, the pod number and total seed number per plant were lower in the 0.1% and 1% treatments compared to the control. As initially hypothesised, the addition of polyester microfibres resulted in changes to the pod-to-seed ratio. Seed filling is the final stage of growth, involving diverse biochemical processes and is impart regulated by leaf photo assimilation processes, delivering the nutrients needed for seed development, such as sucrose, starch, proteins, and fats (Sehgal et al., 2018). This may explain changes demonstrated in the pod and seed number and the seed/pod ratio for the 1% treatment, where PSII was disrupted compared to the control (Sehgal et al., 2019). However, as changes were also demonstrated to the 0.1%, where no changes were shown in PSII, other factors not measured in this study, such as stomatal conductance, chlorophyll content, and inhibited carbon fixation enzymes, may be important (Kaushal et al., 2013).
Conversely, if changes were demonstrated in the root biomass, a reduction in vital minerals such as potassium, calcium, iron, or magnesium would result in reduced seed production (Marles, 2017). This could occur due to changes to the soil structure as a result of the addition of polyester microfibres, as demonstrated by Machado et al. (2019), who found an increase in root biomass but a decrease in the root diameter when 2% w/w polyester microfibres were added to soils.
While this study provides valuable insights into the potential effects of polyester microfibres on S. alba, there is a need for further research to fully understand their impact. Future research should consider how significant the effects demonstrated to the seed/pod ratio would be in a typical agricultural landscape. This could enable an understanding of the economic losses that could result from adding microplastics to agricultural lands. However, field trials are difficult with emerging pollutants, and modelling based studies looking at reduction in crop yields would be beneficial.
In addition, further research is needed into different crops to review whether polyester microfibres have similar effects on crops as on S. alba. This is important because crops differ in their growth and development patterns. Understanding how microfibres affect different crops could help to develop strategies to mitigate their impact on agriculture and food production.
Overall, the study on S. alba highlights the potential risks associated with microfibre pollution, and the need for further research to assess their impact on agricultural ecosystems. By investigating the effects of microplastics on different crops and their mechanisms of action, we can develop strategies to minimize their impact and ensure the sustainability of our food production systems.
In conclusion, the findings of this study suggest that polyester microfibers can have a significant impact on S. alba, an important agricultural crop. Our research has shown that these microfibers can act as an abiotic stressor, negatively affecting the plant’s reproductive capacity and seed yield. Additionally, changes to photosystem II, a key protein complex involved in photosynthesis, have been observed, with potential impacts on crop yield.
To further understand the mechanisms behind these changes, future research should examine seed filling, nutrient status, and root development to understand the role that these play in the reproductive capacity of the plant when exposed to microfibres. Further investigation into the effects of polyester microfibers on other plant species and agricultural crops can help us better understand the scope of this issue and develop strategies to reduce its impact.
The raw data supporting the conclusion of this article will be made available by the authors, without undue reservation.
EH: Conceptualization, Data curation, Formal Analysis, Methodology, Writing–original draft, Writing–review and editing. KR: Supervision, Writing–review and editing. RH: Formal Analysis, Supervision, Writing–review and editing. CG: Supervision, Writing–review and editing.
The author(s) declare that no financial support was received for the research, authorship, and/or publication of this article.
The authors declare that the research was conducted in the absence of any commercial or financial relationships that could be construed as a potential conflict of interest.
The handling editor TS declared a past co-authorship with the authors EH and CG.
All claims expressed in this article are solely those of the authors and do not necessarily represent those of their affiliated organizations, or those of the publisher, the editors and the reviewers. Any product that may be evaluated in this article, or claim that may be made by its manufacturer, is not guaranteed or endorsed by the publisher.
Acharya, S., Rumi, S. S., Hu, Y., and Abidi, N. (2021). Microfibers from synthetic textiles as a major source of microplastics in the environment: a review. Text. Res. J. 91 (17), 2136–2156. doi:10.1177/0040517521991244
Auta, H. S., Emenike, C. U., and Fauziah, S. H. (2017). Distribution and importance of microplastics in the marine environment: a review of the sources, fate, effects, and potential solutions. Environ. Int. 102, 165–176. doi:10.1016/J.ENVINT.2017.02.013
Barboza, L. G. A., Dick Vethaak, A., Lavorante, B. R., Lundebye, A. K., and Guilhermino, L. (2018). Marine microplastic debris: an emerging issue for food security, food safety and human health. Mar. Pollut. Bull. 133, 336–348. doi:10.1016/J.MARPOLBUL.2018.05.047
Barnabás, B., Jäger, K., and Fehér, A. (2008). The effect of drought and heat stress on reproductive processes in cereals. Plant Cell Environ. 31 (1), 11–38. doi:10.1111/J.1365-3040.2007.01727.X
Barrows, A. P. W., Cathey, S. E., and Petersen, C. W. (2018). Marine environment microfiber contamination: global patterns and the diversity of microparticle origins. Environ. Pollut. 237, 275–284. doi:10.1016/J.ENVPOL.2018.02.062
Baumbauer, D. A., and Burgess, M. H. (2020). Season, sowing date, and row cover influences the production of cool season vegetables in movable high tunnels. Can. J. Plant Sci. 100 (5), 528–536. doi:10.1139/cjps-2019-0150
Begcy, K., and Dresselhaus, T. (2018). Epigenetic responses to abiotic stresses during reproductive development in cereals. Plant Reprod. 31, 343–355. doi:10.1007/s00497-018-0343-4
Bosker, T., Bouwman, L. J., Brun, N. R., Behrens, P., and Vijver, M. G. (2019). Microplastics accumulate on pores in seed capsule and delay germination and root growth of the terrestrial vascular plant Lepidium sativum. Chemosphere 226, 774–781. doi:10.1016/j.chemosphere.2019.03.163
Büks, F., and Kaupenjohann, M. (2020). Global concentrations of microplastics in soils - a review. SOIL 6 (2), 649–662. doi:10.5194/SOIL-6-649-2020
Coe, J., Kupitz, C., Basu, S., Conrad, C. E., Roy-Chowdhury, S., Fromme, R., et al. (2015). Crystallization of photosystem II for time-resolved structural studies using an X-ray free electron laser. Methods Enzym. 557, 459–482. doi:10.1016/BS.MIE.2015.01.011
Cole, M., Lindeque, P., Fileman, E., Halsband, C., Goodhead, R., Moger, J., et al. (2013). Microplastic ingestion by zooplankton. Environ. Sci. Technol. 47, 6646–6655. doi:10.1021/es400663f
Cole, M., Lindeque, P., Halsband, C., and Galloway, T. S. (2011). Microplastics as contaminants in the marine environment: a review. Mar. Pollut. Bull. 62, 2588–2597. doi:10.1016/j.marpolbul.2011.09.025
Colzi, I., Renna, L., Bianchi, E., and Castellani, M. B. (2022). Impact of microplastics on growth, photosynthesis and essential elements in Cucurbita pepo L. J. Hazard. Mater. 423 (7), 127238. doi:10.1016/J.JHAZMAT.2021.127238
Corradini, F., Meza, P., Eguiluz, R., Casado, F., Huerta-Lwanga, E., and Geissen, V. (2019). Evidence of microplastic accumulation in agricultural soils from sewage sludge disposal. Sci. Total Environ. 671, 411–420. doi:10.1016/J.SCITOTENV.2019.03.368
Descamps, C., Quinet, M., and Jacquemart, A. L. (2021). Climate change–induced stress reduce quantity and alter composition of nectar and pollen from a bee-pollinated species (Borago officinalis, Boraginaceae). Front. Plant Sci. 12, 2264. doi:10.3389/FPLS.2021.755843/BIBTEX
Descamps, C., Quinet, M., Baijot, A., and Jacquemart, A. L. (2018). Temperature and water stress affect plant–pollinator interactions in Borago officinalis (Boraginaceae). Ecol. Evol. 8 (6), 3443–3456. doi:10.1002/ECE3.3914
de Sousa, F. D. B. (2021). The role of plastic concerning the sustainable development goals: the literature point of view. Clean. Responsible Consum. 3, 100020. doi:10.1016/j.clrc.2021.100020
De Souza Machado, A. A., Lau, C. W., Till, J., Kloas, W., Lehmann, A., Becker, R., et al. (2018). Impacts of microplastics on the soil biophysical environment. Environ. Sci. Technol. 52 (17), 9656–9665. doi:10.1021/acs.est.8b02212
De Souza Machado, A. A., Lau, C. W., Kloas, W., Bergmann, J., Bachelier, J. B., Faltin, E., et al. (2019). Microplastics can change soil properties and affect plant performance. Environ. Sci. Technol. 53 (10), 6044–6052. doi:10.1021/acs.est.9b01339
Dias, A. S., and Lidon, F. C. (2009). Evaluation of grain filling rate and duration in bread and durum wheat, under heat stress after anthesis. J. Agron. Crop Sci. 195 (2), 137–147. doi:10.1111/J.1439-037X.2008.00347.X
Ekanayake, A., Strife, R. J., Zehentbauer, G. N., and David, J. R. (2016). Yellow or white mustard (Sinapis alba L.) oils. Essent. Oils Food Preserv. Flavor Saf., 857–863. doi:10.1016/B978-0-12-416641-7.00098-5
Farooq, M., Gogoi, N., Barthakur, S., Baroowa, B., Bharadwaj, N., Alghamdi, S. S., et al. (2017). Drought stress in grain legumes during reproduction and grain filling. J. Agron. Crop Sci. 203 (2), 81–102. doi:10.1111/JAC.12169
Gago, J., Carretero, O., Filgueiras, A., and Viñas, L. (2018). Synthetic microfibers in the marine environment: a review on their occurrence in seawater and sediments. Mar. Pollut. Bull. 127, 365–376. doi:10.1016/J.MARPOLBUL.2017.11.070
Guo, Q. Q., Xiao, M., Ma, Y., Niu, H., and Zhang, G. (2021). Polyester microfiber and natural organic matter impact microbial communities, carbon-degraded enzymes, and carbon accumulation in a clayey soil. J. Hazard. Mater. 405, 124701. doi:10.1016/J.JHAZMAT.2020.124701
Guo, Z. Q., Li, P., Yang, X., Wang, Z., Lu, B., Chen, W., et al. (2022). Soil texture is an important factor determining how microplastics affect soil hydraulic characteristics. Environ. Int. 165, 107293. doi:10.1016/J.ENVINT.2022.107293
Halekoh, U., Højsgaard, S., and Yan, J. (2006). The R package geepack for generalized estimating equations. JSS J. Stat. Softw. 15. doi:10.18637/jss.v015.i02
Hatzig, S. V., Nuppenau, J. N., Snowdon, R. J., and Schießl, S. V. (2018). Drought stress has transgenerational effects on seeds and seedlings in winter oilseed rape (Brassica napus L.). BMC Plant Biol. 18 (1), 297–313. doi:10.1186/s12870-018-1531-y
Hemingway, J. S. (1976). in ‘Mustards: Brassica spp. and Sinapis alba (cruciferae)’, Evolution of crop plants. Editor N. W. Simmonds [Preprint]. doi:10.3/JQUERY-UI.JS
Hernandez, E., Nowack, B., and Mitrano, D. M. (2017). Polyester textiles as a source of microplastics from households: a mechanistic study to understand microfiber release during washing. Environ. Sci. Technol. 51 (12), 7036–7046. doi:10.1021/acs.est.7b01750
Ilyas, M., Ahmad, W., Khan, H., Yousaf, S., Khan, K., and Nazir, S. (2018). Plastic waste as a significant threat to environment - a systematic literature review. Rev. Environ. Health 33 (4), 383–406. doi:10.1515/reveh-2017-0035
Ingraffia, R., Amato, G., Bagarello, V., Carollo, F. G., Giambalvo, D., Iovino, M., et al. (2022). Polyester microplastic fibers affect soil physical properties and erosion as a function of soil type. SOIL 8 (1), 421–435. doi:10.5194/SOIL-8-421-2022
Ingraffia, R., Amato, G., Iovino, M., Rillig, M. C., Giambalvo, D., and Frenda, A. S. (2022). Polyester microplastic fibers in soil increase nitrogen loss via leaching and decrease plant biomass production and N uptake. Environ. Res. Lett. 17 (5), 054012. doi:10.1088/1748-9326/AC652D
Jägerbrand, A. K., and Kudo, G. (2016). Short-term responses in maximum quantum yield of PSII (Fv/Fm) to ex situ temperature treatment of populations of bryophytes originating from different sites in Hokkaido, Northern Japan. Plants 5 (2), 455–465. doi:10.3390/PLANTS5020022
Jia, L., Liu, L., Zhang, Y., Fu, W., Liu, X., Wang, Q., et al. (2023). Microplastic stress in plants: effects on plant growth and their remediations. Front. Plant Sci. 14, 1226484. doi:10.3389/FPLS.2023.1226484
Kaushal, N., Awasthi, R., and Siddique, K. H. M. (2013). Heat-stress-induced reproductive failures in chickpea (Cicer arietinum) are associated with impaired sucrose metabolism in leaves and anthers allelopathy in agriculture view project functional genomics for enhanced drought tolerance in chickpea. view project. Funct. Plant Biol. doi:10.1071/FP13082
Kedzierski, M., Cirederf-Boulant, D., Palazot, M., Yvin, M., and Bruzaud, S. (2023). Continents of plastics: an estimate of the stock of microplastics in agricultural soils. Sci. Total Environ. 880, 163294. doi:10.1016/J.SCITOTENV.2023.163294
Kokotkiewicz, A., and Luczkiewicz, M. (2015). “Celery (Apium graveolens var. dulce (mill.) pers.) oils,” in Essential oils in food preservation, flavor and safety (Elsevier), 325–338. doi:10.1016/B978-0-12-416641-7.00037-7
Kumar, D., Singh, H., Raj, S., and Soni, V. (2020). Chlorophyll a fluorescence kinetics of mung bean (Vigna radiata L.) grown under artificial continuous light. Biochem. Biophysics Rep. 24, 100813. doi:10.1016/J.BBREP.2020.100813
Kwak, J., Liu, H., Wang, D., Lee, Y. H., and Lee, J. S. (2022). Critical review of environmental impacts of microfibers in different environmental matrices. Comp. Biochem. Physiology Part C Toxicol. Pharmacol. 251, 109196. doi:10.1016/J.CBPC.2021.109196
Lambers, H., and Teste, F. P. (2013). Interactions between arbuscular mycorrhizal and non-mycorrhizal plants: do non-mycorrhizal species at both extremes of nutrient availability play the same game? Plant, Cell. and Environ. 36 (11), 1911–1915. doi:10.1111/PCE.12117
Liang, Y., Lehmann, A., Yang, G., Leifheit, E. F., and Rillig, M. C. (2021). Effects of microplastic fibers on soil aggregation and enzyme activities are organic matter dependent. Front. Environ. Sci. 9, 650155. doi:10.3389/fenvs.2021.650155
Lozano, Y. M., and Rillig, M. C. (2020a). Effects of microplastic fibers and drought on plant communities. Environ. Sci. Technol. 54 (10), 6166–6173. doi:10.1021/acs.est.0c01051
Lusher, A. L., Jambeck, J. R., and Law, K. L. (2017). Production, use, and fate of all plastics ever made. Sci. Adv. 3 (7), e1700782. doi:10.1126/sciadv.1700782
Lwanga, E. H., Beriot, N., Corradini, F., Silva, V., Yang, X., Baartman, J., et al. (2022). Review of microplastic sources, transport pathways and correlations with other soil stressors: a journey from agricultural sites into the environment. Chem. Biol. Technol. Agric. 9 (1), 20. doi:10.1186/S40538-021-00278-9
Marles, R. J. (2017). Mineral nutrient composition of vegetables, fruits and grains: the context of reports of apparent historical declines. J. Food Compost. Anal. 56, 93–103. doi:10.1016/J.JFCA.2016.11.012
Maxwell, K., and Johnson, G. N. (2000). Chlorophyll fluorescence—a practical guide. J. Exp. Bot. 51 (345), 659–668. doi:10.1093/JEXBOT/51.345.659
Mészáros, E., Bodor, A., Kovács, E., Papp, S., Kovács, K., Perei, K., et al. (2023). Impacts of plastics on plant development: recent advances and future research directions. Plants 12 (18), 3282. doi:10.3390/PLANTS12183282
Mishra, S., Singh, R. P., Rath, C. C., and Das, A. K. (2020). Synthetic microfibers: source, transport and their remediation Chandi charan Rath Synthetic microfibers: source, transport and their remediation. Article J. Water Process Eng. 38, 2214–7144. doi:10.1016/j.wpe.2020.101612
Mitrović, P. M., Stamenković, O. S., Banković-Ilić, I., Djalović, I. G., Nježić, Z. B., Farooq, M., et al. (2020). White mustard (Sinapis alba L.) oil in biodiesel production: a review. Front. Plant Sci. 11, 299. doi:10.3389/fpls.2020.00299
Murchie, E. H., and Lawson, T. (2013). Chlorophyll fluorescence analysis: a guide to good practice and understanding some new applications. J. Exp. Bot. 64 (13), 3983–3998. doi:10.1093/JXB/ERT208
Nizzetto, L., Bussi, G., Futter, M. N., Butterfield, D., and Whitehead, P. G. (2016). A theoretical assessment of microplastic transport in river catchments and their retention by soils and river sediments. Environ. Sci. Process. Impacts 18 (8), 1050–1059. doi:10.1039/C6EM00206D
Ohashi, Y., Saneoka, H., Mohapatra, P. K., and Fujita, K. (2009). Differences in the responses of stem diameter and pod thickness to drought stress during the grain filling stage in soybean plants. Acta Physiol. Plant 31 (2), 271–277. doi:10.1007/S11738-008-0229-4/TABLES/3
Pang, J., Du, Y. -T., Colmer, T. D, and Siddique, K. H. M. (2017). Pattern of water use and seed yield under terminal drought in chickpea genotypes. Front. Plant Sci. 8, 1375. doi:10.3389/FPLS.2017.01375/BIBTEX
Qi, Y., Yang, X., Pelaez, A. M., Lwanga, E. H., Beriot, N., Gertsen, H., et al. (2018). Macro- and micro- plastics in soil-plant system: effects of plastic mulch film residues on wheat (Triticum aestivum) growth. Sci. Total Environ. doi:10.1016/j.scitotenv.2018.07.229
Reiling, K., and Davison, A. W. (1992). The response of native, herbaceous species to ozone: growth and fluorescence screening. New Phytol. 120 (1), 29–37. doi:10.1111/j.1469-8137.1992.tb01055.x
Rering, C. C., Franco, J. G., Yeater, K. M., and Mallinger, R. E. (2020). Drought stress alters floral volatiles and reduces floral rewards, pollinator activity, and seed set in a global plant. Ecosphere 11 (9), e03254. doi:10.1002/ECS2.3254
Ritchie, G. A. (2006). National proceedings: forest and conservation nursery associations - 2005. Available at: http://www.rngr.net/nurseries/publications/proceedings.
Rochman, C. M. (2018). Microplastics research-from sink to source. Sci. (New York, N.Y.) 360 (6384), 28–29. doi:10.1126/science.aar7734
Sajjad, M., Huang, Q., Khan, S., Khan, M. A., Liu, Y., Wang, J., et al. (2022). Microplastics in the soil environment: a critical review. Environ. Technol. Innovation 27, 102408. doi:10.1016/J.ETI.2022.102408
Sehgal, A., Sita, K., Siddique, K. H. M., Kumar, R., Bhogireddy, S., Varshney, R. K., et al. (2018). Drought or/and heat-stress effects on seed filling in food crops: impacts on functional biochemistry, seed yields, and nutritional quality. Front. Plant Sci. 9. doi:10.3389/FPLS.2018.01705
Sehgal, A., Sita, K., Bhandari, K., Kumar, S., Kumar, J., Vara Prasad, P., et al. (2019). Influence of drought and heat stress, applied independently or in combination during seed development, on qualitative and quantitative aspects of seeds of lentil (Lens culinaris Medikus) genotypes, differing in drought sensitivity. Plant, Cell. and Environ. 42 (1), 198–211. doi:10.1111/PCE.13328
Selonen, S., Dolar, A., Jemec Kokalj, A., Skalar, T., Parramon Dolcet, L., Hurley, R., et al. (2020). ‘Exploring the impacts of plastics in soil – the effects of polyester textile fibers on soil invertebrates. Sci. Total Environ. 700, 134451. doi:10.1016/j.scitotenv.2019.134451
Shane, M. W., and Lambers, H. (2005). Cluster roots: a curiosity in context. Plant Soil 274 (1), 101–125. doi:10.1007/S11104-004-2725-7
Shao, H. B., Chu, L. Y., Jaleel, C. A., and Zhao, C. X. (2008). Water-deficit stress-induced anatomical changes in higher plants. Comptes Rendus Biol. 331 (3), 215–225. doi:10.1016/J.CRVI.2008.01.002
Sharma, S., and Chatterjee, S. (2017). Microplastic pollution, a threat to marine ecosystem and human health: a short review. Environ. Sci. Pollut. Res. 24, 21530–21547. doi:10.1007/s11356-017-9910-8
Smith, A. R., and Zhao, D. (2016). Sterility caused by floral organ degeneration and abiotic stresses in arabidopsis and cereal grains, Front. Plant Sci. 7. doi:10.3389/FPLS.2016.01503
Su, Z., Ma, X., Guo, H., Sukiran, N. L., Guo, B., Assmann, S. M., et al. (2013). Flower development under drought stress: morphological and transcriptomic analyses reveal acute responses and long-term acclimation in arabidopsis, The Plant Cell, 25 (10), 3785–3807. doi:10.1105/TPC.113.115428
R Core Team (2022). R: A language and environment for statistical computing. Vienna, Austria: R Foundation for Statistical Computing.
Teng, L., Zhu, Y., Li, H., Song, X., and Shi, L. (2022). The phytotoxicity of microplastics to the photosynthetic performance and transcriptome profiling of Nicotiana tabacum seedlings. Ecotoxicol. Environ. Saf. 231, 113155. doi:10.1016/J.ECOENV.2021.113155
Ullah, R., Tsui, M. T., Chen, H., Chow, A., Williams, C., and Ligaba-Osena, A. (2021). Microplastics interaction with terrestrial plants and their impacts on agriculture. J. Environ. Qual. 50 (5), 1024–1041. doi:10.1002/JEQ2.20264
Wu, Y., Guo, P., Zhang, X., Zhang, Y., Xie, S., Deng, J., et al. (2019). Effect of microplastics exposure on the photosynthesis system of freshwater algae. J. Hazard. Mater. 374, 219–227. doi:10.1016/J.JHAZMAT.2019.04.039
Yu, Z., Song, S., Xu, X. l., Ma, Q., and Lu, Y. (2021). Sources, migration, accumulation and influence of microplastics in terrestrial plant communities. Environ. Exp. Bot. 192, 104635. doi:10.1016/J.ENVEXPBOT.2021.104635
Yamashita, A., Nijo, N., Pospísil, P., Morita, N., Takenaka, D., Aminaka, R., et al. (2008). Quality Control of Photosystem II: reactive oxygen species are responsible for the damage to photosystem II under moderate heat stress, J. Biol. Chem. 283 (42), 28380. doi:10.1074/JBC.M710465200
Zeb, A., Liu, W., Meng, L., Lian, J., Wang, Q., Lian, Y., et al. (2022). Effects of polyester microfibers (PMFs) and cadmium on lettuce (Lactuca sativa) and the rhizospheric microbial communities: a study involving physio-biochemical properties and metabolomic profiles. J. Hazard. Mater. 424, 127405. doi:10.1016/J.JHAZMAT.2021.127405
Zhang, G. S., Zhang, F. X., and Li, X. T. (2019). Effects of polyester microfibers on soil physical properties: perception from a field and a pot experiment. Sci. Total Environ. 670, 1–7. doi:10.1016/J.SCITOTENV.2019.03.149
Keywords: microplastics, fibers, plastics, soils, plant development
Citation: Harrison EG, Reiling K, Halfpenny RK and Gwinnett C (2024) The effects of polyester microfibres on the development and seed yield of white mustard (Sinapis alba L.). Front. Environ. Sci. 12:1310310. doi: 10.3389/fenvs.2024.1310310
Received: 09 October 2023; Accepted: 05 February 2024;
Published: 29 February 2024.
Edited by:
Thomas Stanton, Loughborough University, United KingdomReviewed by:
Miranda Prendergast-Miller, Northumbria University, United KingdomCopyright © 2024 Harrison, Reiling, Halfpenny and Gwinnett. This is an open-access article distributed under the terms of the Creative Commons Attribution License (CC BY). The use, distribution or reproduction in other forums is permitted, provided the original author(s) and the copyright owner(s) are credited and that the original publication in this journal is cited, in accordance with accepted academic practice. No use, distribution or reproduction is permitted which does not comply with these terms.
*Correspondence: Eleanor Grace Harrison, RS5IYXJyaXNvbkBrZWVsZS5hYy51aw==
Disclaimer: All claims expressed in this article are solely those of the authors and do not necessarily represent those of their affiliated organizations, or those of the publisher, the editors and the reviewers. Any product that may be evaluated in this article or claim that may be made by its manufacturer is not guaranteed or endorsed by the publisher.
Research integrity at Frontiers
Learn more about the work of our research integrity team to safeguard the quality of each article we publish.