- 1Date Palm Research Center of Excellence, King Faisal University, Al-Hasa, Saudi Arabia
- 2Central Laboratory for Date Palm Research and Development, Agriculture Research Center, Giza, Egypt
- 3Environment and Bio-agriculture Department, Faculty of Agriculture, Al-Azhar University, Cairo, Egypt
- 4Animal and Fish Production Department, College of Agricultural and Food Sciences, King Faisal University, Al-Hasa, Saudi Arabia
- 5Fish and Animal Production Department, Faculty of Agriculture (Saba Basha), Alexandria University, Alexandria, Egypt
- 6National Institute of Oceanography and Fisheries (NIOF), Cairo, Egypt
Due to the progressive climate change on our planet, scientists are interested in solving this issue since it threatens not only certain regions or countries but also the world’s ecosystems and economies. Therefore, minimizing carbon dioxide (CO2) emissions and reducing atmospheric levels are global priorities. Thus, it is necessary at this moment to develop an appropriate approach to reduce or stabilize CO2 levels in the atmosphere. However, CO2 capture projects are long-term, low-profitable, and high-risk environmental projects. Consequently, it is necessary to find an appropriate and sustainable CO2 capture approach that is efficient in reducing atmospheric CO2 levels while having a safe impact on the environment. Although carbon (C) is the key basic component used to produce biological compounds by photosynthetic organisms in terrestrial plants, the C pathway is a key factor affecting the capture of CO2 by photosynthetic organisms. Among photosynthetic organisms, Paulownia, a multipurpose tree, is popular around the world for its timber and its potential role in CO2 sequestration. Paulownia spp. belongs to the Paulowniaceae family and comprises a group of trees. These trees are primarily found in southeastern Asia, particularly in China, and have been intentionally grown for more than two millennia due to their ornamental, cultural, and medicinal value. The number of Paulownia species varies depending on taxonomic classification, ranging from 6 to 17. Among them, Paulownia tomentosa, Paulownia elongata, Paulownia fortunei, and Paulownia catalpifolia are the most widely recognized and favored species. The present review provides a comprehensive technical-economic scenario for the capture of one million tons of CO2 by Paulownia trees (as a terrestrial plant model, grown on 2,400 ha−1). P. tomentosa can be utilized in agroforestry systems to mitigate greenhouse gas (GHG) emissions within urban cities and emphasize the carbon storage potential of agroforestry. In conclusion, Paulownia trees as an environmental mass project showed great encouragement to investors and governments to expand these types of projects to achieve global climate goals by 2050.
1 Introduction
The climate change challenge is a global issue affecting many species of plants and animals, as well as human civilization and the health of the earth. The continued increase in greenhouse gas (GHG) emissions, such as CO2, CH4, N2O, and fluorinated gases, has only served to worsen this situation (Adams and Engel, 2021). Among greenhouse gases, carbon dioxide (CO2) is the most important and essential for photosynthesis, which sustains the life of plants. However, the concentration of CO2 can vary, with natural gas power plants emitting CO2 at a rate of 3%–4%, while coal power plants release it at a rate of 10%–13%. Conversely, bio-refineries can have a CO2 concentration of up to 80%. Generally, the amount of atmospheric CO2 globally has risen significantly, from 313 ppm in 1960 to 411 ppm in 2020, and is projected to reach 450 ppm by 2035 (Santori et al., 2018). This could result in a 2°C increase in global warming and have a major impact on the global economy, with a 99% chance of this outcome (Santori et al., 2018; Bushing, 2021). However, increased atmospheric CO2 is considered the predominant cause of global climate change (Shreyash et al., 2021).
The reduction of CO2 emissions is a pressing global concern, and a strategy must be put in place to lower or maintain CO2 levels in the atmosphere. Despite extensive research on reducing CO2 emissions by physical and chemical methods, there are several environmental, technical, and economic challenges. Therefore, it is crucial to find a sustainable, profitable, and effective approach for capturing CO2 that reduces atmospheric CO2 levels better than physical and chemical methods (Kadlec et al., 2021). A study conducted by Prasad et al. (2021) found that there are two crucial approaches for reducing CO2 emissions: 1) reducing dependence on fossil fuels and increasing the use of renewable energy sources and 2) capturing and storing CO2 through biological, chemical, or physical methods (Shreyash et al., 2021). Osman et al. (2021) have identified three primary methods for CO2 capture, storage, and utilization: pre-combustion, post-combustion, and oxyfuel combustion.
Among CO2 capture and storage (CCS) technologies, biological CCS is the most cost-efficient and environmentally sound option, relying primarily on photosynthetic organisms such as terrestrial and aquatic plants (Chu and Majumdar, 2012; Benedetti et al., 2018). Through photosynthesis, photoautotrophic organisms, including terrestrial and aquatic plants, can convert CO2 into carbon-based products such as sugars, proteins, and lipids. Globally, these organisms can store solar energy at a rate of 120 TW y−1 (Zhu et al., 2010). This means that photoautotrophic organisms can cover the global energy demand by 800%. Therefore, the widespread cultivation of these organisms is a promising solution for meeting a significant portion of the world’s energy needs (Stephenson et al., 2011).
Several published studies have reported that urban green areas can play a crucial role in reducing the carbon footprint of cities. These areas include trees, parks, gardens, and canals and provide several benefits, such as improved air quality, reduced noise, preservation of biodiversity, mitigation of urban heat islands, management of microclimate, soil stability, groundwater recharge, avoidance of soil erosion, and CO2 capture (Strohbach et al., 2012; Singh et al., 2018). Such urban green spaces, along with vegetation, green areas, and soils, have the potential to lower atmospheric CO2 levels and influence the CO2 cycle (Chang et al., 2017; Roeland et al., 2019). In another work, Chia et al. (2016) reported that forests are seen as a way to mitigate the effects of climate change, given that it is a global issue.
Forests play a critical role in carbon sequestration, storing carbon in trees and soils. They also provide numerous other ecosystem services that are essential for human wellbeing and the functioning of the planet. Forests provide a habitat for countless species of plants and animals, many of which are essential for pollination, pest control, and nutrient cycling. They also play a critical role in regulating the water cycle, helping prevent erosion and flooding, and providing clean drinking water to downstream communities (Martínez Pastur et al., 2018; Chaudhry et al., 2021). For a long time, forest CO2 capture projects were considered high-risk investments due to the long time frames involved. Thus, they have been adopted relatively slowly or excluded from international carbon markets, such as those established by the Kyoto Protocol and the EU Emissions Trading Scheme (ETS). Recently, over 25 public funds have provided incentives for forest operations related to carbon rather than relying on carbon markets. This helps governments better manage their forests (van der Gaast et al., 2018). The findings of Chia et al. (2016) align with those of Osman et al. (2021), who stated that carbon pricing is an effective approach to encourage investment in the carbon sequestration and storage industries. Regarding this point, terrestrial plants have attractive CO2 capture potential and high biomass productivity. Trees have an average CO2 capture potential of 1.78 tons CO2 tons biomass−1 y−1 and an average biomass productivity of 2.6–3.9 tons ha−1 y−1 (Fuhrer and Molnar, 2003; Khan and Ansari, 2005).
Investigating the C pathways in terrestrial plants is crucial to assessing their ability to absorb atmospheric CO2 and produce oxygen (O2) through photosynthesis, as well as their contribution to the ecosystem. The exchange of CO2 and O2 by photosynthetic cells through their cell walls plays a crucial role in this process. However, understanding the unique carbon pathways in terrestrial plants can provide valuable information about their potential as a tool for CO2 capture (Kheyrodin and Kheyrodin, 2017). When the stomata of a plant are open, CO2 enters and is utilized in the photosynthesis process. At the same time, O2, a byproduct of photosynthesis, can escape. However, in hot and dry conditions, this problem is amplified because, while the stomata are open, the plant also loses water through transpiration. As a result, the efficiency of a plant’s CO2 fixation can vary. Plants fix CO2 in the atmosphere through one of three pathways: the C3, C4, and Crassulacean acid metabolism (CAM) pathways (Winter and Holtum, 2017).
C3 plants, which make up more than 85% of plants on Earth (Kheyrodin and Kheyrodin, 2017), are referred to as the “C3 pathway” because the first molecule created in the cycle is a 3-carbon molecule called 3-phosphoglyceric acid. Although C3 plants are the most common on the planet, C4 plants are estimated to be twice as efficient at photosynthesizing as C3 plants, although this difference becomes less noticeable in high CO2 environments (Mondal et al., 2017). This increased efficiency is because C4 plants concentrate carbon and reduce carbon loss during the fixation process. In contrast, C3 plants fix CO2 through the Calvin cycle, where the RuBisCO enzyme causes an oxidation reaction that accounts for part of the energy utilized in photosynthesis being lost through photorespiration. As a result, the amount of carbon that the plant fixes and releases back into the environment as CO2 has decreased by approximately 25%. In C3 plants, the main carboxylase is RuBisCO, and the main product of RuBP’s carboxylation is a three-carbon sugar. Additionally, RuBP’s oxygenation, which is the first stage of photorespiration, is catalyzed by RuBisCO in C3 plants (Zhu et al., 2010). Figure 1 shows the carbon pathways in plant cells. The C4 pathway was unknown until the 1960s, when scientists discovered the C4 pathway in sugarcane. The C4 pathway, also known as the Hatch–Slack cycle, is named for the 4-carbon intermediate molecules that were generated (malic or aspartic acid).
In C4 plants, the PEPCase enzyme is the main carboxylase, a 4-carbon molecule is the main carboxylation product in light, and a secondary carboxylase is RuBisCO, which works under high CO2 conditions to limit oxygenation and photorespiration. C4 plants have an additional step in their pathway before starting the Calvin cycle, which decreases the amount of carbon lost in the CO2 fixation process (Santos et al., 2022; Silva Araújo et al., 2022). In C4 plants, CO2 reacts with phosphoenolpyruvate to produce 4-carbon acids (malate), which are transported to bundle sheath cells where CO2 is liberated and used in the Calvin cycle. The typical carbon isotope composition in C4 plants ranges from 10% to 14%. In CAM plants, which are found in deserts and shallow bodies of water, the stomata close during the day to conserve water and open at night to absorb CO2, which is stored as malate. During daylight, photosynthesis starts through the Calvin cycle. Table 1 summarizes the differences between the three pathways, as described in various studies (Hatfield et al., 2009; Carvajal, 2010; Kheyrodin and Kheyrodin, 2017; Guidi et al., 2019).
Paulownia trees, also known as the “princess tree,” are part of the Paulowniaceae family and have attracted attention for their potential to capture CO2 from the atmosphere. These trees have a fast growth rate and are known for their exceptional carbon sequestration abilities. When grown in large quantities, they can absorb substantial amounts of CO2, making them a promising solution for combating the impacts of climate change (Janjić and Janjić, 2019).
Several studies have indicated that Paulownia trees can absorb up to twice as much CO2 compared to other tree species. The CO2 absorbed by Paulownia trees is stored in their wood and soil, making them effective long-term carbon sinks. In addition, these trees are resistant to pests and require minimal input of water, fertilizer, and pesticides (Magar et al., 2018; Jakubowski, 2022; Testa et al., 2022). The popularity of Paulownia trees has skyrocketed due to their remarkable CO2 capture and storage capabilities (Icka et al., 2016b; Magar et al., 2018). They are known for their high productivity and carbon sequestration potential and are widely considered valuable assets in the fight against climate change (Dong et al., 2014). Furthermore, they are versatile and can be utilized for a range of purposes, such as lumber, construction materials, and musical instruments. With their ability to absorb and store significant amounts of CO2, Paulownia trees have become a popular choice for reforestation and carbon offset projects. The effectiveness of these trees in capturing CO2 is contingent upon various factors, such as location, growth conditions, and management practices (Jakubowski, 2022).
A comprehensive evaluation of the entire life cycle of Paulownia trees, including their harvesting and processing, is crucial to determining their actual carbon footprint. The potential of Paulownia trees to capture CO2 from the atmosphere is substantial, but more research and analysis are required to understand its effectiveness and limitations regarding C sequestration value and information about P. tomentosa and its implementation for CO2 mitigation. This work offers a review of carbon pathways in terrestrial photosynthetic plants as well as an in-depth assessment of the ability of terrestrial plants, particularly the Paulownia genus, to capture CO2 from the atmosphere. The study also contains a detailed techno-economic scenario aimed at capturing one million tons of CO2 using the Paulownia species. The expected results from using the Paulownia are carefully evaluated and discussed. Finally, the work gives a comprehensive overview of ongoing carbon credit projects and assesses the prospects of achieving global climate objectives by 2050.
2 Role of terrestrial plants in CO2 capture for biomass production
During biophysical processes, trees absorb and release CO2 into the atmosphere. In the process of photosynthesis, leaves capture CO2 through their stomata and utilize the energy from the sun to transform it into O2, carbohydrates, and water. These substances are then used to create the structures of wood, as well as the vitamins, resins, and hormones required for tree development, growth, and health. Trees get their energy from the carbohydrates that are produced during photosynthesis. The net storage of CO2 by the tree is the result of the interaction between photosynthesis and respiration (Aguaron and McPherson, 2012). Indeed, urban green areas, especially those with trees, have a great potential to capture CO2 from the atmosphere and reduce the effects of climate change in urban areas. However, several studies have reported that urban green areas can be critical to reducing carbon footprints (Strohbach et al., 2012; Nouri et al., 2019; Sharma et al., 2020). As previously reported by Sharma et al. (2020), there are three ways to reduce CO2 levels naturally in the atmosphere: (I) increase atmospheric CO2 capture rates through tree planting; (II) reduce energy demand; and (III) increase bioenergy demand and utilization of bioenergy.
The expression “atmospheric CO2 storage” describes the accumulation of woody biomass that accumulates over time as plants grow. The annual rate of CO2 uptake in biomass for one growing season is called “atmospheric CO2 sequestration.” Sequestration relies on tree growth and death, which is strongly dependent on species diversity and demographic factors such as the age of the urban forest. Carbon stored in one location at a specific moment is referred to as “carbon stock.” Carbon stocks in forests include live and standing dead plants, wood waste and litter, organic matter present in the soil, and harvested stocks like timber for wood products and fuel (Robards, 2008). According to the Intergovernmental Panel on Climate Change (IPCC) report (Pedersen et al., 2022), the plant ecosystem involves five major carbon pools, namely, 1) above-ground biomass (AGB), 2) below-ground biomass (BGB), 3) dead wood, 4) detritus, and 5) soil organic matter. When trees die, the biomass becomes part of the food chain or becomes soil carbon (Suryawanshi et al., 2014).
However, the average rate of CO2 sequestration in trees is mainly influenced by factors such as the size of the tree at maturity, the lifespan, and the growth rate (Nowak and Crane, 2002). To determine the amount of CO2 stored in trees, Aguaron and McPherson (2012) used allometric formulas that consider several characteristics, including diameter at breast height (DBH), site index, height, moisture content, wood density, and overall tree conditions. These characteristics can vary between species and even within individual trees, making it difficult to determine an accurate average. The allometric biomass equations used for this calculation come in two forms: volumetric and direct. The volumetric equation calculates the above-ground volume of a tree using DBH and height, while the direct equation determines the above-ground dry weight using the same variables (Domec and Gartner, 2002). The study conducted by MacDicken (1997) concluded that tree biomass, including AGB, BGB, total biomass (TB), carbon content (CC), and equivalent CO2 (CO2-Eq.), can be estimated by measuring the diameter at breast height (DBH, cm) of the tree using morphometric equations. These equations were designed specifically for a dry climate with an average seasonal rainfall of up to 1,500 mm.
As described in the study by Sharma et al. (2020), AGB, BGB, TB, CC, and CO2-Eq. were calculated for several trees commonly used in the construction of landscapes and green belts. Table 2 presents the mean values (based on 10 trees) of AGB, BGB, TB, CC, and CO2-Eq. found in the Godavari Botanical Garden in Nepal (Magar et al., 2018) and at the Amity University Campus in India (Sharma et al., 2020).
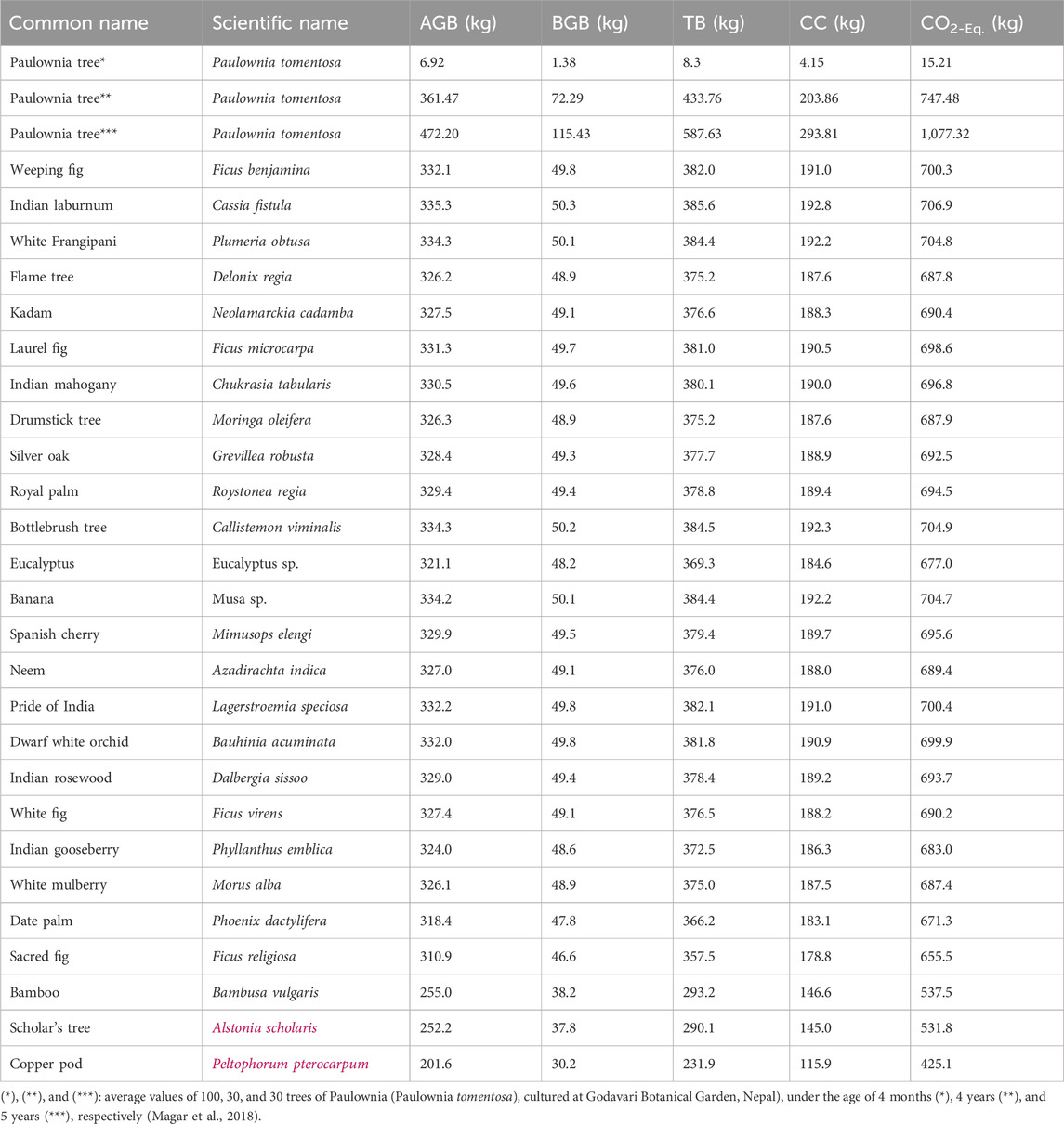
TABLE 2. CO2 equivalent content of some tree species used in the construction of CO2 capture, landscape, and green belts.
The IPCC report demonstrated that urban green areas (green belts) may reduce atmospheric carbon in three primary ways (Pörtner et al., 2022). First, atmospheric CO2 is absorbed by the leaves, and a portion of this adsorbed CO2 is then released into the atmosphere. The remaining portion is stored in the plant tissues, both AGB and BGB (total biomass), leading to plant growth in the form of biomass. Second, soils are considered one of the major contributors to carbon stocks because they make up only a small portion of the overall carbon stocks; litter and dead wood are not significant sources of carbon. Third, urban areas minimize the need for heating by lowering wind speed and the need to cool infrastructure by offering shade and evaporation. This significantly lowers the need to use fossil fuels to generate energy, which balances out carbon emissions (Jo, 2002).
Most published studies on carbon have focused on AGB because BGB assessments are inherently more expensive and time-consuming. There is still a demand for reliable BGB equations, although very few studies have concentrated on creating equations to predict BGB based on straightforward tree variables (Hertel et al., 2009; Ziegler et al., 2012; Yuen et al., 2013; Kralicek et al., 2017). The amount of CO2 captured per hectare using the formula based on plant photosynthesis and wood chemical composition can be estimated by calculating the average yearly increase of the trees, and this value was estimated to be 981 kg m−3 (Fuhrer and Molnar, 2003).
Intelligent selection of effective and suitable species, as well as their proper management in urban spaces, are vital in increasing the potential and success of these areas (Bhalla and Bhattacharya, 2015; Ram et al., 2015). Therefore, to guarantee the success of any green belt project, the specific tree species must be identified before starting the project planning. The study by Alotaibi et al. (2020) investigated which specific, effective, and tolerant tree species must be planted and used within the frame of the “Green Riyadh Project,” one of the limited greening-belt projects. This study aimed to assess the air pollution tolerance index (APTI) associated with the anticipated performance index (API) for five tree species (Ficus altissima, Eucalyptus camaldulensis, Ziziphus spina-christi, Albizia lebbeck, and Prosopis juliflora), which are usually planted and used along roadsides and around industrial and residential spaces. Four different Riyadh sites were used to collect leaf samples: a residential area, a busy intersection, an industrial area, and a reference site that was approximately 20 km outside the city. Based on the APTI and API performance data, they concluded that the green belt planning in the “Green Riyadh Project” must include growing Ficus altissima on roadsides and heavy industrial locations, followed by Z. spina-christi and A. lebbeck.
3 Current carbon credit industries and projects
To reach the goal of reducing global CO2 emissions by 2050, there is a growing need for projects that capture CO2. Various strategies have been proposed and implemented throughout the world, including improving energy efficiency, implementing a carbon tax, increasing the production of renewable energy, planting trees, and capturing CO2 from the atmosphere in power plants (Nunez, 2019). Carbon capture and storage (CCS) and carbon capture, utilization, and storage (CCUS) are considered effective solutions for addressing climate change. As the climate change crisis intensifies, CCS/CCUS projects are becoming increasingly common. In a comprehensive study of CCUS systems, Hong (2022) reviewed technologies for CO2 capture, separation, transport, utilization, and storage. The study indicated various methods for CO2 capture, such as industrial separation, pre- and post-combustion, oxyfuel combustion, chemical looping combustion, and direct air capture (DAC).
Therefore, the current study specifically focuses on DAC technology using biological adsorption through trees and microalgae. Studies by Deutz and Bardow (2021), Keith et al. (2018), and Abanades et al. (2020) have shown that the efficiency of CO2 removal, energy consumption, and cost for the DAC technology are 85%–93% vol, 5.25 GJ tons−1 CO2, and USD 140–USD 340 tons−1 CO2, respectively. Carbon credit pricing is a crucial aspect in promoting the development and growth of CO2 capture technologies (Lefvert et al., 2022). To make these technologies commercially viable, it is important to have attractive carbon pricing mechanisms, such as carbon taxes or allowances. The value of carbon taxes varies among countries, ranging from a few US $ to 100 US $ per ton of CO2. In 2017, the carbon allowance equivalent was valued at 5.17 dollars per ton of CO2, and it is projected to increase to 47.25 USD per ton of CO2 by 2023 (Chen et al., 2020; Osman et al., 2021). In 2020, there were 22 demo CO2 capture projects around the world, with the United States and China being the main contributors with seven and five projects, respectively (Vega et al., 2020). The number of commercial CO2 capture projects has been steadily increasing, with a reported increase from 51 projects in 2019 to 135 projects in 2021 (Turan et al., 2021). In September 2021, the global CO2 capture capacity was estimated at 49.4 million tons per year.
The Americas region had the highest CO2 capture capacity, contributing 58.5% of all global projects. Europe ranked second with a total of 38 projects and 28.1% of global projects (E Silva and Costa, 2021), while the Asia–Pacific region ranked third with 14 projects (Steyn and Havercroft, 2021). The Middle East has the lowest number of commercial CCS/CCUS projects, representing only 10% of the global CO2 captured (Turan et al., 2021), with a total CO2 capture capacity reached in September 2021 of 3.8 million tons CO2 y−1. In the Middle East region, the total number of CCS/CCUS commercial projects was four, coming from three countries (one in Qatar, one in Saudi Arabia, and two in the United Arab Emirates) (Steyn and Havercroft, 2021). The European Union aims to achieve net-zero CO2 emissions by 2050, leading to an increase in CCS/CCUS projects and facilities in the region. The United Kingdom government has invested 1 billion GBP in CCS/CCUS facilities to establish four industrial clusters that will be able to capture 10 million metric tons of CO2 per year by 2030 (Turner et al., 2021). This investment has contributed to the growth of CCS/CCUS projects and facilities in the EU, leading to a 32% increase in the maximum CO2 capture capacity. This capacity increased to 37.4 million tons of CO2 per year in September 2021, up from 28.4 million metric tons of CO2 in 2020 (Turan et al., 2021; E Silva and Costa, 2021).
In response to the global increase in carbon emissions and the decline in the carbon budget, the use of CCS technology has become increasingly critical in addressing climate change. Therefore, the use of CCS technology has become increasingly crucial in mitigating the impact of rising global carbon emissions. The IEA has outlined a Sustainable Development Scenario (IEA-SDS) that calls for CCS to reduce global emissions by 9%. The IEA-SDS aims to reduce the world’s annual CO2 emissions from 33 to 10 gigatons by 2050, which requires the development of 2,000 commercial CO2 capture projects. This means that an estimated 70 to 100 new projects need to be built each year, requiring a total capital investment of between 655 and 1,280 billion USD (Rassool, 2021; Yan et al., 2021). On the other hand, scientists are incorporating CCS into their scenario models as a means of effectively capturing and storing CO2 in geological formations. This aligns with the goals outlined in the Paris Agreement and is reflected in the Sustainable Development Scenario (IEA-SDS) (Newell et al., 2021; Berrada et al., 2022).
Due to the high cost of implementation, private sector investment is crucial to financing CCS projects. The majority of funding is expected to come from debt, financial markets, and sovereign wealth funds, as governments may not be able to provide the necessary capital within the required time frame. According to the Global CCS Institute Report 2021 (Rassool, 2021), the prices of CCS systems are projected to decrease as more projects are implemented, but the rate of decrease depends on several variables, such as geography and industry. The CCS learning rate predicts a cost reduction of 10%–25% for every doubling of installed capacity, leading to an estimated total capital need of 655–1,280 billion US $.
Developing nations still lack sufficient government-led programs that recognize the value of CO2. However, programs that incentivize CO2 capture investment have been implemented successfully in developed countries in the form of carbon credits. These credits are used to offset emissions and finance mitigation projects in less developed countries. The most well-known example of a crediting system is the Clean Development Mechanism (CDM) under the Kyoto Protocol (Bajaj, 2022). The report by Doda et al. (2021) found that the voluntary carbon market (VCM) is rapidly growing in the carbon credit industry, but they also noted that these credits alone will not be enough to address all climate risks. They concluded that investment in CO2 capture through both natural and technical means, including CCS, is necessary. Additionally, investment in CO2 capture can be made through VCMs as the need for compensation becomes increasingly important. More than 612 million USD of carbon credits were granted through VCM programs between 2007 and 2019, including 142 million USD in 2019 (Doda et al., 2021).
4 Taxonomy, characteristics, and cultivation of Paulownia
4.1 Paulownia classification and botanical description
In the past, Paulownia was classified as a member of the Scrophulariaceae family before its current classification as a member of the Paulowniaceae family (Schneiderová and Šmejkal, 2015). There is a lack of consensus on the exact number of Paulownia species, as taxonomical classifications vary. Depending on the classification, the number of species ranges from 6 to 17 (Kadlec et al., 2021). In the study conducted by Li et al. (2020), eight species of Paulownia have been defined, namely, P. catalpifolia, P. tomentosa, P. australis, P. kawakamii, P. coreana, P. fortune, P. fargesii, and P. elongata. However, the Chinese Flora Editorial Committee differs in its classification, as it does not recognize P. coreana but instead includes two additional variations of P. tomentosa: P. tomentosa var. tomentosa and P. tomentosa var. tsinlingensis (Cheng et al., 2019). In addition, other studies have recognized P. albiflora, P. taiwaniana, and P. glabrata (Yadav et al., 2013; He et al., 2016).
Typically, a mature Paulownia tree reaches a height ranging from 20 to 30 m; the tallest registered specimen was 50 m (Icka et al., 2016a; Yi et al., 2020). The trunk is typically approximately 1 m thick but can reach 2 m under suitable environmental conditions. Under normal environmental conditions, the trunk of a mature Paulownia tree generally has a diameter of approximately 1 m. However, under favorable environmental conditions, the trunk can grow even thicker, reaching up to 2 m in diameter (Kadlec et al., 2021). Paulownia trees possess extensive and well-developed root systems that can extend up to a depth of 8 m in the soil. The upper part of the roots is densely packed, exhibiting branching and dichotomous growth patterns. The bark of the tree is typically brown or black. In young Paulownia trees, lenticels begin to form, and as the tree matures, these lenticels expand, eventually developing into vertical cracks on the bark’s surface (Jakubowski et al., 2018).
Mature Paulownia trees have umbrella-shaped leaves that measure approximately 10–12 cm in width and 15–30 cm in length. The leaves have smooth, undulating edges. It is worth noting that younger trees have even larger leaves, with a width that can reach up to 80 cm (Woods, 2008b). The flowering period for Paulownia occurs in May and June, with flowers displaying five petals that range in color from white to light purple. The fruits of the Paulownia tree are approximately 4 cm long and 2.5 cm wide. They mature in the autumn season, and each fruit can release up to 2,000 winged seeds (Šmejkal et al., 2007). Many different substances are secreted by glandular trichomes covering the surfaces of leaves, fruits, and flowers (Asai et al., 2008; Kobayashi et al., 2008).
4.2 Cultivation and growth conditions of Paulownia
Paulownia trees have the ability to reproduce both generatively and vegetatively, although vegetative reproduction is predominantly employed in industrial settings. Traditional methods of reproduction, such as root-splitting, which is also utilized for natural species, have been historically employed (Yi et al., 2020). Additionally, techniques like mini-cuttings at an early developmental stage (Stuepp et al., 2015) or stimulating rooting in green cuttings (Temirov et al., 2021) have been utilized. However, in vitro propagation serves as the primary means of propagation for many clones (Gyuleva, 2010; Magar et al., 2016). The production of a robust and well-developed root system is a critical aspect of the reproduction phase, leading to extensive research focused on addressing this matter (Pożoga et al., 2019; Mohamad et al., 2022).
Among the most commonly cultivated species of paulownias are P. tomentosa, P. catalpifolia, P. elongata, P. taiwaniana, P. fortunei, P. glabrata, and P. fargesii (Woods, 2008b). During the initial global introduction of paulownias, pure botanical species were used predominantly. The United States was one of the early adopters, importing Paulownias (specifically P. tomentosa) around 1840. Due to its rapid growth, it earned the nickname “the tree of the future.” Over the past 150 years, it has spread across various states, causing significant problems and sparking heated debates concerning all species of Paulownia. P. tomentosa has been officially recognized as an invasive species, leading to its eradication in many states. In the United States, Paulownia has garnered both opponents and proponents, and discussions surrounding the genus are contentious due to the substantial profits generated by existing crops (Snow, 2015).
Recent research indicates that P. tomentosa has the ability to spread in various areas where forests have been damaged by several disturbances (Chongpinitchai and Williams, 2021). In certain countries, specific Paulownia species like P. tomentosa have been identified as hazardous and recognized as invasive, as seen in Austria (Franz, 2007). Although natural Paulownia species are still cultivated in Asia, including Turkey, there is a growing shift toward hybrid varieties. In Bulgaria, for instance, hybrids have gained importance after unsuccessful attempts to cultivate pure species (Gyuleva, 2010).
4.3 Paulownia as a sustainable model for CO2 mitigation
The study by Sage and Sultmanis (2016) highlighted an important issue to consider: why are C3 trees more suitable for forests and carbon sequestration than C4 trees? Most C4 species are associated with harsh habitats, such as deserts and salty areas, where arborescence is not feasible. Most C4 species are grasses and sedges that lack the meristems required for tree growth. Only seven species of Hawaiian Euphorbia and a few desert plants that become arborescent with age exhibit C4 photosynthesis. Therefore, wherever C3 trees can grow, they have a competitive advantage over C4 plants due to their height (Sage and Sultmanis, 2016). Recently, published research in the field of reducing climate change has increased rapidly, particularly in the area of biomass production as a renewable energy resource (Jamil et al., 2021; Sikkema et al., 2021; Kirikkaleli et al., 2022). Numerous reports predict that the demand for wood and wood-based products will continue to increase until at least 2050 (Haldar and Sethi, 2021; Kircher, 2022). The production of trees and timber species for biomass use is also increasing worldwide (Ols and Bontemps, 2021; Hamdan and Houri, 2022). They are also considered one of the most promising C4 trees, known for their air-purifying properties (Magar et al., 2018; Jakubowski, 2022; Testa et al., 2022).
One notable characteristic of Paulownia is its remarkable ability to grow to enormous sizes within a remarkably short period. In China, it is often said that Paulownia “shoots up like a pole in 1 year, transforms into an umbrella in 3 years, and can be harvested for boards in 5 years” (Zhu et al., 1986). China has witnessed the existence of extraordinary specimens, such as an 80-year-old P. fortunei tree in Kweichow Province, which soared to a towering height of 49.5 m, possessed a DBH of 202 cm, and yielded a wood volume of 34 m3. Another striking example was a 90-year-old Paulownia with a DBH of 224 cm and a wood volume of 44 m3. Even younger trees demonstrated impressive dimensions, such as an 11-year-old P. fortunei tree in the Guangxi Zhuang Autonomous Region of southern China, which was 22 m tall, had a DBH of 75.1 cm and produced a wood volume of 3.69 m3. P. elongata also achieved similar sizes. In their native habitats in China, Paulownia typically attains a DBH of 30–40 cm within a decade and produces approximately 0.3–0.5 m3 of wood. However, under ideal conditions, valuable timber can be obtained in just 5–6 years (Zhu et al., 1986; Yi et al., 2020).
As reported in the study conducted by Kozakiewicz et al. (2020), the environmental and growth conditions of Paulownia vary between different species, such as P. tomentosa, P. fortunei, and P. elongata, which consequentially contribute to the variation in the density of Paulownia wood. The density of Paulownia wood ranges from 220 to 400 kg m─3, with an average of approximately 270 kg m─3 (Akyildiz and Kol Sahin, 2010; Madhoushi and Boskabadi, 2019; Lachowicz and Giedrowicz, 2020a). Paulownia trees have a high growth rate and low wood density, but they do not efficiently produce biofuel (Jakubowski, 2022). Recently, using Paulownia for the production of biomass has gained popularity, especially as a way to mitigate the harmful effects of CO2 (Magar et al., 2018). These trees can produce more biomass per year than other trees can produce in several seasons. However, the region in which trees are grown can limit biomass production (Zuazo et al., 2013). In a study by Magar et al. (2018), P. tomentosa trees were planted in the Godavari Botanical Garden in Nepal at a density of 2000 plants ha−1. The researchers assessed the carbon content of the total biomass of 5-year-old, 1-year-old, and newly planted 4-month-old P. tomentosa trees. They found that the 5-year-old trees had a carbon content of 4.52 kg C y─1 tree─1, yielding 9 tons of C ha─1 y─1. The 1-year-old trees had a carbon content of 18.21 kg C y─1 tree─1, resulting in 0.36 tons of C y─1 ha─1. The newly planted 4-month-old P. tomentosa trees in a remote village in Nepal had a carbon content of 6.07 kg of C tree─1.
In a study conducted by Gyuleva et al. (2021), they estimated the productivity (dried biomass content) of two cultivated Paulownia species (P. tomentosa and the hybrid species P. elongata × P. fortunei) after 2 and 4 years of planting in southwestern Bulgaria. They found that after 2 or 4 years, P. tomentosa showed higher productivity (3.47 and 36.99 tons ha─1, respectively) than the hybrid species (2.73 and 19.96 tons ha−1, respectively). The carbon content of P. tomentosa was also higher after 2 or 4 years (1.73 and 18.49 tons ha─1, respectively) than that of the hybrid species (1.15 and 9.98 tons ha─1, respectively). Similarly, the equivalent capture of CO2 (CO2-Eq.) of P. tomentosa was also higher after 2 or 4 years (6.34 and 67.79 tons ha─1, respectively) than that of the hybrid species (4.21 and 36.59 tons ha─1, respectively).
In another study, Joshi (2015) reported that a 16-year-old trial of P. tomentosa in Asia yielded 38.8 tons of C y─1 ha─1, while a 21-year-old trial yielded more than 105 tons C y─1 ha─1. Paulownia can have reduced growth rates when grown in poor soil conditions. In a study by Madejón et al. (2016), the biomass of P. fortune cultivated in Spain for 3 years was 3.34 tons ha─1 (Madejón et al., 2016), compared to the symmetrically grown Eucalyptus globules, which had a biomass of 40.4 tons ha─1. In a related study, Stankova et al. (2019) used a model to demonstrate how the crop species had an impact on the differences in biomass production, which ranged from 0.3 to 4.5 tons ha─1 of dry matter. Although modeling is continually improving, it still has several issues because of how complicated the factors that might affect prediction are, such as different areas and their local conditions. This is particularly true for the prediction of wood characteristics and biomass production (Abbasi et al., 2020; Lachowicz and Giedrowicz, 2020b; Palma et al., 2021). Therefore, globally, using Paulownia as a significant component of biomass production requires special attention in nations where the growth of hybrids that could compete with native species is encouraged. In recent reports, Iran, due to its field experience, has reported its ability to plant Paulownia in an area of approximately 16 × 104 km2 (Galán-Martín et al., 2015; Abbasi et al., 2020). More importantly, several nations are beginning to advance in this area, such as Portugal (Abreu et al., 2020), Iran (Abbasi et al., 2020), Spain (Parra-Lopez et al., 2015; Pleguezuelo et al., 2015), Romania (BUZAN et al., 2018), Italy (Testa et al., 2022), Serbia (Janjić and Janjić, 2019), Ukraine (Morozova et al., 2020; Kaletnik et al., 2021), Northern Ireland (Woods, 2008a; Olave et al., 2015), and Kyrgyzstan (Thevs et al., 2021).
Compared to the production and utilization of fossil fuels, the production and utilization of bioenergy are considered more environmentally benign (Pieratti, 2020). Globally, concerned parties are increasingly understanding that implementing strategies to combat climate change reduces environmental risks, increases production efficiency, and increases profits (Hiloidhari et al., 2019; Secinaro et al., 2020). According to the literature, numerous studies have shown that farmers, especially in the EU, are motivated by their position toward adopting cleaner production methods that can reduce the harmful effects of climate change (Sacchelli et al., 2017; Boyer and Touzard, 2021). Globally, Paulownia culture is a trend that has been pervasive in recent years, in addition to their environmental tasks, such as fighting climate change. As a primary key to the sustainable production of biomass crops, Paulownia farms powerfully achieve economic sustainability for farmers (Magar et al., 2018).
A recent study conducted by Testa et al. (2022) evaluated the economic profitability of the Paulownia farming that replaced a vineyard. The study was conducted on a farm located in southern Italy. They reported that Paulownia farming for wood and woodchip production generated an annual overall margin of approximately 357.91 € ha−1, compared to the annual overall margin of wine grapes of approximately 237.41 € ha−1, yielding 150% annual profit ha−1, whereas Paulownia farming for biomass production only has roughly zero profitability (4.22 € ha−1). Finally, they concluded that profitability relies not only on the product type but also on future price variations, public funding, rewards, and the appropriate decisions made by entrepreneurs for the sustainable development of supply chains from an environmental and social perspective.
5 Investment opportunities and risks in Paulownia and other carbon sequestration-based projects
Forestry-based projects have struggled to take off in compliance and voluntary carbon markets due to various reasons, including investment risks related to non-permanence and leakage (Verma and Ghosh, 2023). Non-permanence refers to the risk that carbon stored in forests will be released back into the atmosphere before the end of the project’s crediting period, while leakage refers to the risk that emission reductions achieved in one area are offset by increased emissions in another area. These risks have led to uncertainty in the carbon market and a lack of investor confidence in forestry-based projects (Henry, 2023; Wang et al., 2023). Additionally, there have been challenges in accurately measuring carbon sequestration in forests and ensuring the permanence of carbon storage over time. As a result, there have been relatively few forestry-based projects in the compliance market, with most focused on afforestation and reforestation. In the voluntary market, forestry projects have also faced challenges in attracting buyers due to the perception that they are less credible than other types of carbon credits (Chen et al., 2023).
These challenges have been exacerbated by the limited availability of funding for forest projects and a lack of standardized methodologies for measuring and verifying carbon sequestration. However, recent developments in measurement and verification technologies, as well as the emergence of new voluntary carbon markets and standards, may provide opportunities for forestry-based projects to overcome these challenges and play a more significant role in global efforts to mitigate climate change (Ince, 2022).
On the other hand, Paulownia, also known as the “empress tree,” is a fast-growing hardwood species that has become increasingly popular as an investment opportunity in recent years. The tree’s ability to grow rapidly, even on poor soils, makes it an attractive option for timber production as well as for carbon sequestration and ecosystem restoration projects. However, as with any investment opportunity, there are both potential rewards and risks associated with investing in Paulownia projects (Yadav et al., 2013). One of the primary benefits of investing in Paulownia projects is the fast growth rate, which allows for relatively quick returns on investment. Additionally, the high-quality wood of the tree is in demand for a variety of uses, including furniture, flooring, and musical instruments, further increasing the potential for profitability (Fos et al., 2023).
Another potential benefit of Paulownia projects is their ability to sequester carbon. As trees grow, they absorb CO2 from the atmosphere, helping mitigate climate change. In addition, Paulownia can be planted on degraded or marginal land, helping restore ecosystems and providing a variety of additional benefits, such as improved soil health and increased biodiversity (Marana, 2018). In addition to the importance of Paulownia culture in the fields of CO2 mitigation, biomass, and wood production, this tree has great commercial potential due to the bioproducts it could produce. Paulownia wood can be used in the production of wood plastics and their composites (Khanjanzadeh et al., 2012; Ebrahimi et al., 2021), blackboards (Nelis et al., 2019), low-density woods (Li et al., 2018; Yu et al., 2018), lightweight particleboards (Nelis et al., 2018; Nelis and Mai, 2021), biopolymers (Rodríguez-Seoane et al., 2020), as well as energy sources (Zhang et al., 2017) such as bioethanol (Zhang et al., 2017; Kirikkaleli et al., 2022), biomethane (Janjić and Janjić, 2019), and biohydrogen (Zhang et al., 2022). In addition to the applications of Paulownia wood, the Paulownia flowers, leaves, and their remains can be utilized for medicinal (Yang et al., 2019; Adach et al., 2021; Dżugan et al., 2021; Huang et al., 2021; Nowak et al., 2022), animal feed (Al-Sagheer et al., 2019; Ganchev et al., 2019; Alagawany et al., 2022), and bioremediation applications (Tzvetkova et al., 2015; Miladinova-Georgieva et al., 2018a; Miladinova-Georgieva et al., 2018b). Despite these potential benefits, there are also several risks associated with investing in Paulownia projects (Ferguson et al., 2010).
One of the main risks is the potential for crop failure. Although Paulownia is known for its fast growth, it is also susceptible to disease, pests, and other environmental factors that can impact growth rates and yield. Additionally, the tree’s rapid growth can make it more vulnerable to wind damage, which can result in significant losses. In addition to these risks, there are also several regulatory and legal considerations that investors in Paulownia projects should be aware of. Depending on the location of the plantation, there may be specific regulations related to forest management practices, land use, and environmental impacts that must be complied with. Failure to comply with these regulations can result in fines or legal action, which can have a significant impact on the profitability of the project (de Deus Ribeiro et al., 2021). Despite these risks, several strategies can be employed to minimize potential losses and maximize returns on investment in Paulownia projects. One approach is to diversify investments across multiple projects or regions, reducing the impact of any individual crop failure or market downturn. Additionally, investors can work with experienced plantation management teams that have a proven track record of success in Paulownia projects. This can help ensure that best practices are followed and that risks are minimized (Zhao et al., 2019; Oliveira et al., 2020).
6 Techno-economic scenario for Paulownia CO2 capture
To achieve global climate targets by 2050, there will be greater demand for approximately 2000 commercial CO2 capture projects and a rate of 70–100 commercial CO2 capture projects y−1, with a total capital investment ranging from 655 to 1,280 billion USD. This number clearly shows that these projects required large investments, which governments (Alprol et al., 2021; Ashour and Omran, 2022) have not been prepared to make in the required time (Bajaj, 2022). Biological CO2 capture projects are the most sustainable, safe, and attractive solution that can overcome and mitigate high levels of atmospheric CO2. Although the Paulownia tree, a terrestrial C4 plant, differs in its nature and habitats, they are both photosynthetic organisms and have a greater potential for biological CO2 fixation than other terrestrial and aquatic plants (Mansour et al., 2022). Although biological CO2 capture projects are seen as long-term, low-profitability, and high-risk environmental projects, recent microalgae-based CO2 capture projects have proven otherwise. They are commercial, short-term, highly profitable, and low-risk. Additionally, these types of projects have a significant environmental impact by reducing high levels of atmospheric CO2. However, to capture 1 million tons of atmospheric CO2 over 10 years as a part of a megaproject, a technical and economic scenario for Paulownia trees is presented below. The cost of the used land is not included.
For wood production, Paulownia hybrid trees (Wu et al., 2014; Huseinovic et al., 2017) are mainly planted primarily at a distance of 4 m2 × 4 m2 (Zhao et al., 2019), with approximately 625 trees ha-1 (Icka et al., 2016b; Berdón Berdón et al., 2017). As reported by several studies (Newman et al., 1997; Popescu and Sabau, 2016; Zhao et al., 2019), the standard cycle duration for Paulownia trees’ roundwood is 10 years. Based on this fact, the current study has selected 10 years as the ideal cycle duration for Paulownia trees. The study conducted by Jakubowski (Zhao et al., 2019) reported that there are ideal growing conditions for Paulownia trees for wood production in southern Europe and the Middle East. However, the crop yield of Paulownia trees varies based on several parameters, such as climate change, soil type, culture conditions, age, species, and cultivation regions. In Asia, at 16 and 21 years of age, P. tomentosa yields approximately 382.6 and 223 tons y─1 ha─1, respectively (Joshi, 2015). In Bulgaria, after 2 and 4 years, P. tomentosa showed higher productivity (3.47 and 36.99 tons ha─1, respectively) than the hybrid species (P. elongata × P. fortunei), (2.73 and 19.96 tons ha─1, respectively) (Gyuleva et al., 2021). In China, at 80 and 90 years of age, Paulownia trees reached a wood volume of 34 m3 and 44 m3, respectively. In Spain, after 3 years, the total biomass of P. fortune was 3.34 tons ha─1 (Madejón et al., 2016). In Nepal, the average total biomass of P. tomentosa after 5 years was 19.50 tons ha─1 y─1 (Magar et al., 2018).
Based on the literature, proposed model, and calculations, in the best-case scenario to capture 1 million tons of CO2 over 10 years using Paulownia trees, 1.5 million trees will be planted in approximately 2,400 ha─1 with a planting density of 625 trees ha─1 (the distance needed for each tree is approximately 4 m2 × 4 m2). Based on the literature, proposed model, calculations, and the best-case scenario, each Paulownia tree will gradually capture CO2 (CO2-Eq.) in the 1st, 2nd, 3rd, 4th, 5th, 6th, 7th, 8th, 9th, and 10th years as follows: 6.25, 12.50, 25.00, 27.50, 33.00, 42.90, 60.06, 93.09, 144.29, and 250 kg CO2 tree─1, respectively. For 1.5 million trees in 10 years, it will be calculated that all 1.5 M trees will gradually capture CO2 as follows: 9,375; 18,750; 37,500; 41,250; 49,500; 64,350; 90,090; 139,635; 216,435; and 375,000 tons CO2 1.5 million trees at 2,400 ha─1.
After 10 years, the CO2 captured by 1.5 million trees on 2,400 ha─1 is approximately 1.04 million tons of CO2. According to Equations 6 and 5, to convert CO2-Eq. to CC (eq. 6) and then to TB (eq. 5), the constant factors 0.27273 and 0.5, respectively, should be used. Therefore, the total biomass of Paulownia trees yearly was calculated as follows: 5,114; 10,227; 20,455; 22,500; 27,000; 35,100; 49,140; 76,165; 118,055; and 204,545 tons, respectively. As a result, after 10 years, the total crop biomass (wood) of 1.5 million trees is approximately 568,301 tons (Sharma et al., 2020).
To establish a mass project over 10 years to capture 1.04 million tons of atmospheric CO2 using Paulownia trees, 1.5 million trees will be planted on 2,400 ha─1 to produce 568,301 tons of total crop biomass (wood). The total cost (capital and operating costs) of the investment required for the planting of 1.5 million tons in 2,400 ha─1 over 10 years is approximately 1,128 billion USD. The total marketing value of Paulownia’s wood (568,301 tons) is estimated at 1,136.6 billion USD (based on 2,000 USD ton─1 wood).
Our proposed scenario is based on the study by Testa et al. (2022), who evaluated the economic profitability of Paulownia and concluded that the highest profit of Paulownia is approximately USD 358 ha−1 y−1, while the lowest profit is approximately USD 5 ha−1 y−1. Furthermore, as described previously (Chen et al., 2020; Osman et al., 2021), the carbon allowance in 2023 is equivalent to approximately USD 47.25 tons of CO2, which means that the equivalent carbon allowance of 1.04 million tons of CO2 (captured by 1.5 million trees on 2,400 ha─1 10-year─1) is approximately 49.7 billion USD (based on the lowest estimated value reported for 2023). In conclusion, Table 3 shows a technical and economical comparison between Paulownia trees with respect to the capture of 1 million tons of atmospheric CO2 over 10 years.
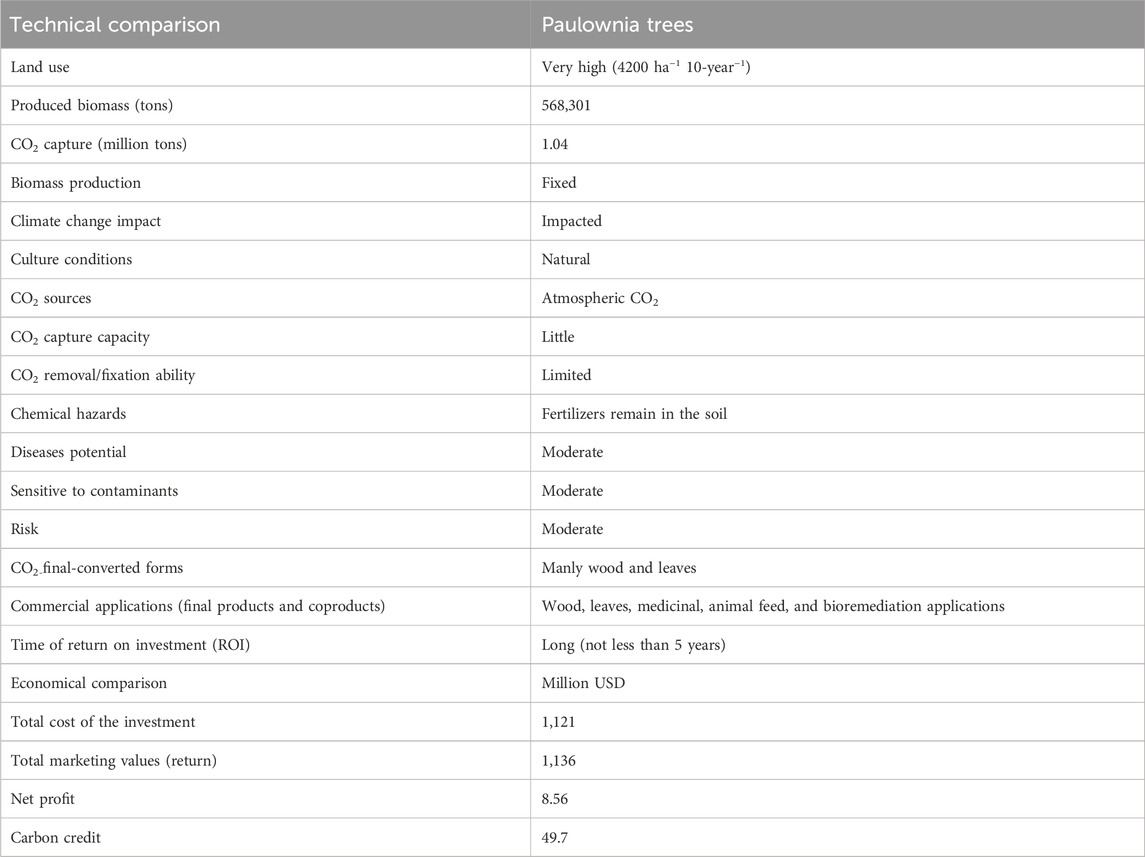
TABLE 3. Technical and economic comparison of Paulownia trees, concerning the capture of 1 million tons of CO2 over 10 years.
7 Conclusion and future perspectives
Reducing CO2 emissions is a global priority. Thus, it is necessary to develop an appropriate approach to reduce or stabilize CO2 levels in the atmosphere. First, CO2 capture projects are long-term, low-profitable, and high-risk environmental projects. To achieve global climate goals by 2050, there is a greater demand for approximately 2000 commercial CO2 capture projects, with an average of 70–100 commercial CO2 capture projects per year, with a total capital investment ranging from 655 to 1,280 billion USD (Rassool, 2021). This figure clearly shows that the majority of CO2 capture project funding will come from debt, financial markets, and sovereign wealth funds. Therefore, it is necessary to provide direct support for CO2 capture projects on a large scale, especially in developing countries. In addition, this number clearly shows that these projects require high investments that governments were not prepared to spend in the required time. Therefore, the private sector should be encouraged to participate in this type of investment. Carbon credit pricing is the most effective way to encourage investors to expand and develop CO2 capture technologies. It is essential to have enough attractive carbon pricing for CO2 capture technology to become commercially viable, such as a carbon tax or carbon allowances, especially for investors. Private sector encouragement may include tax exemption and providing technologies with low facility prices. On the other hand, requiring flue and power plants to participate in financing such projects increases the carbon tax on the companies and factories that emit high CO2 levels into the atmosphere, as well as on transport, shipping, and aviation companies (Rassool, 2021). These challenges make investors, as well as governments, not prefer this type of project. Therefore, it is necessary to find appropriate, sustainable, and profitable CO2 capture projects that are efficient in reducing atmospheric CO2 levels. Previously, many scientists focused on capturing atmospheric CO2.
Today, scientists around the world are proposing a completely different new strategy. Scientists try not only to capture atmospheric CO2 but also to use it straightaway for energy generation alongside other vital commercial applications by converting atmospheric CO2 into several biologically bioactive carbonic compounds such as proteins, carbohydrates, and lipids. Therefore, the regeneration of bioenergy alongside the capture of atmospheric CO2, in addition to the benefit of other carbonic bioactive compounds, is a wonderful concept and a likely solution to the global warming issue (Doda et al., 2021).
Based on the literature, recommended calculations, and equations, this review presents the latest developments in the use of Paulownia trees as a biological solution for capturing CO2, with a focus on technical and economic aspects. The study provides a scenario for the implementation of a million ton CO2 capture project using C4 Paulownia trees grown on 2,400 ha. The results demonstrate the profitability and feasibility of Paulownia trees as a large-scale CO2 capture project and provide insights into the potential for investment and government support toward achieving global climate goals by 2050.
Author contributions
HG: conceptualization, funding acquisition, investigation, methodology, writing–original draft, and writing–review and editing. AB: investigation, writing–original draft, and writing–review and editing. ATM: investigation and writing–original draft. MA: investigation, writing–original draft, conceptualization, methodology, and writing–review and editing.
Funding
The author(s) declare that financial support was received for the research, authorship, and/or publication of this article. The authors extend their appreciation to the Deputyship for Research and Innovation, Ministry of Education in Saudi Arabia for funding this research work through the project number INSTV007.
Acknowledgments
The authors would like to acknowledge the Deanship of Scientific Research, Vice Presidency for Graduate Studies and Scientific Research, King Faisal University, Saudi Arabia.
Conflict of interest
The authors declare that the research was conducted in the absence of any commercial or financial relationships that could be construed as a potential conflict of interest.
The author(s) declared that they were an editorial board member of Frontiers, at the time of submission. This had no impact on the peer review process and the final decision.
Publisher’s note
All claims expressed in this article are solely those of the authors and do not necessarily represent those of their affiliated organizations, or those of the publisher, the editors, and the reviewers. Any product that may be evaluated in this article, or claim that may be made by its manufacturer, is not guaranteed or endorsed by the publisher.
References
Abanades, J. C., Criado, Y. A., and Fernández, J. R. (2020). An air CO 2 capture system based on the passive carbonation of large Ca (OH) 2 structures. Sustain. Energy and Fuels 4, 3409–3417. doi:10.1039/d0se00094a
Abbasi, M., Pishvaee, M. S., and Bairamzadeh, S. (2020). Land suitability assessment for Paulownia cultivation using combined GIS and Z-number DEA: a case study. Comput. Electron. Agric. 176, 105666. doi:10.1016/j.compag.2020.105666
Abreu, M., Reis, A., Moura, P., Fernando, A. L., Luís, A., Quental, L., et al. (2020). Evaluation of the potential of biomass to energy in Portugal—conclusions from the CONVERTE project. Energies 13, 937. doi:10.3390/en13040937
Adach, W., Żuchowski, J., Moniuszko-Szajwaj, B., Szumacher-Strabel, M., Stochmal, A., Olas, B., et al. (2021). In vitro antiplatelet activity of extract and its fractions of Paulownia Clone in vitro 112 leaves. Biomed. Pharmacother. 137, 111301. doi:10.1016/j.biopha.2021.111301
Adams, J. C., and Engel, J. (2021). “Human-made risks and climate change with global heating,” in Life and its future (Springer).
Aguaron, E., and Mcpherson, E. G. (2012). “Comparison of methods for estimating carbon dioxide storage by Sacramento’s urban forest,” in Carbon sequestration in urban ecosystems (Springer).
Akyildiz, M. H., and Kol Sahin, H. (2010). Some technological properties and uses of paulownia. wood: Paulownia tomentosa Steud.
Alagawany, M., Farag, M. R., Sahfi, M. E., Elnesr, S. S., Alqaisi, O., El-Kassas, S., et al. (2022). Phytochemical characteristics of Paulownia trees wastes and its use as unconventional feedstuff in animal feed. Anim. Biotechnol. 33, 586–593. doi:10.1080/10495398.2020.1806074
Alotaibi, M. D., Alharbi, B. H., Al-Shamsi, M. A., Alshahrani, T. S., Al-Namazi, A. A., Alharbi, S. F., et al. (2020). Assessing the response of five tree species to air pollution in Riyadh City, Saudi Arabia, for potential green belt application. Environ. Sci. Pollut. Res. 27, 29156–29170. doi:10.1007/s11356-020-09226-w
Alprol, A. E., Ashour, M., Mansour, A. T., Alzahrani, O. M., Mahmoud, S. F., and Gharib, S. M. (2021). Assessment of water quality and phytoplankton structure of eight alexandria beaches, southeastern mediterranean sea, Egypt. J. Mar. Sci. Eng. 9, 1328. doi:10.3390/jmse9121328
Al-Sagheer, A. A., Abd El-Hack, M. E., Alagawany, M., Naiel, M. A., Mahgoub, S. A., Badr, M. M., et al. (2019). Paulownia leaves as a new feed resource: chemical composition and effects on growth, carcasses, digestibility, blood biochemistry, and intestinal bacterial populations of growing rabbits. Animals 9, 95. doi:10.3390/ani9030095
Asai, T., Hara, N., Kobayashi, S., Kohshima, S., and Fujimoto, Y. (2008). Geranylated flavanones from the secretion on the surface of the immature fruits of Paulownia tomentosa. Phytochemistry 69, 1234–1241. doi:10.1016/j.phytochem.2007.11.011
Ashour, M., and Omran, A. M. (2022). Recent advances in marine microalgae production: highlighting human health products from microalgae in view of the coronavirus pandemic (COVID-19). Fermentation 8, 466. doi:10.3390/fermentation8090466
Benedetti, M., Vecchi, V., Barera, S., and Dall’osto, L. (2018). Biomass from microalgae: the potential of domestication towards sustainable biofactories. Microb. Cell Factories 17, 173–218. doi:10.1186/s12934-018-1019-3
Berdón Berdón, J., Montero Calvo, A., Royano Barroso, L., Parralejo Alcobendas, A., and González Cortés, J. (2017). Study of Paulownia’s biomass production in mérida (badajoz), southwestern Spain. Environ. Ecol. Res. 5, 521–527. doi:10.13189/eer.2017.050709
Berrada, T., Engelhardt, L., Gibson, R., and Krueger, P. (2022). The economics of sustainability linked bonds. Swiss Finance Institute Research Paper.
Bhalla, P., and Bhattacharya, P. (2015). Urban biodiversity and green spaces in Delhi: a case study of new settlement and lutyens’ Delhi. J. Hum. Ecol. 52, 83–96. doi:10.1080/09709274.2015.11906933
Boyer, J., and Touzard, J.-M. (2021). To what extent do an innovation system and cleaner technological regime affect the decision-making process of climate change adaptation? Evidence from wine producers in three wine clusters in France. J. Clean. Prod. 315, 128218. doi:10.1016/j.jclepro.2021.128218
Buzan, R. L., Maxim, A., Odagiu, A., Balint, C., and Hartagan, R. M. (2018). Paulownia sp. used as an energetic plant, for the phytoremediation of soils and in agroforestry systems. ProEnvironment Promediu 11.
Carvajal, M. (2010). Investigation into CO2 absorption of the most representative agricultural crops of the region of murcia. Madrid: CSIC (Consejo Superior de Investigaciones Cientificas.
Chang, J., Qu, Z., Xu, R., Pan, K., Xu, B., Min, Y., et al. (2017). Assessing the ecosystem services provided by urban green spaces along urban center-edge gradients. Sci. Rep. 7, 11226–11229. doi:10.1038/s41598-017-11559-5
Chaudhry, S., Sidhu, G. P. S., and Paliwal, R. (2021). Restoring ecosystem services of degraded forests in a changing climate. Handb. Ecol. Ecosyst. Eng., 353–375. doi:10.1002/9781119678595.ch19
Chen, D., Cheng, Y., Zhou, N., Chen, P., Wang, Y., Li, K., et al. (2020). Photocatalytic degradation of organic pollutants using TiO2-based photocatalysts: a review. J. Clean. Prod. 268, 121725. doi:10.1016/j.jclepro.2020.121725
Chen, J., Hu, S., Chen, L., Gu, J., Ge, F., and Wang, S. (2023). Optimal forest rotation from the viewpoint of carbon sequestration efficiency based on the gilpin-ayala model. IAENG Int. J. Appl. Math. 53.
Cheng, C.-l., Jia, X.-h., Xiao, C.-m., and Tang, W.-z. (2019). Paulownia C-geranylated flavonoids: their structural variety, biological activity and application prospects. Phytochem. Rev. 18, 549–570. doi:10.1007/s11101-019-09614-2
Chia, E. L., Fobissie, K., and Kanninen, M. (2016). Exploring opportunities for promoting synergies between climate change adaptation and mitigation in forest carbon initiatives. Forests 7, 24. doi:10.3390/f7010024
Chongpinitchai, A. R., and Williams, R. A. (2021). The response of the invasive princess tree (Paulownia tomentosa) to wildland fire and other disturbances in an Appalachian hardwood forest. Glob. Ecol. Conservation 29, e01734. doi:10.1016/j.gecco.2021.e01734
Chu, S., and Majumdar, A. (2012). Opportunities and challenges for a sustainable energy future. nature 488, 294–303. doi:10.1038/nature11475
De Deus Ribeiro, G. B., Batista, F. R. S., De Magalhães, M. A., Valverde, S. R., Carneiro, A. d. C. O., and Amaral, D. H. (2021). Techno-economic feasibility analysis of a eucalyptus-based power plant using woodchips. Biomass Bioenergy 153, 106218. doi:10.1016/j.biombioe.2021.106218
Deutz, S., and Bardow, A. (2021). Life-cycle assessment of an industrial direct air capture process based on temperature–vacuum swing adsorption. Nat. Energy 6, 203–213. doi:10.1038/s41560-020-00771-9
Doda, B., Theuer, S. L. H., Cames, M., Healy, S., and Schneider, L. (2021). Voluntary offsetting: credits and allowances. Clim. Change 4, 2021.
Domec, J. C., and Gartner, B. L. (2002). How do water transport and water storage differ in coniferous earlywood and latewood? J. Exp. Bot. 53, 2369–2379. doi:10.1093/jxb/erf100
Dong, Y., Fan, G., Zhao, Z., and Deng, M. (2014). Compatible solute, transporter protein, transcription factor, and hormone-related gene expression provides an indicator of drought stress in Paulownia fortunei. Funct. Integr. genomics 14, 479–491. doi:10.1007/s10142-014-0373-4
Dżugan, M., Miłek, M., Grabek-Lejko, D., Hęclik, J., Jacek, B., and Litwińczuk, W. (2021). Antioxidant activity, polyphenolic profiles and antibacterial properties of leaf extract of various paulownia spp. clones. Agronomy 11, 2001. doi:10.3390/agronomy11102001
Ebrahimi, H., Vaziri, V., Faraji, F., Aminian, H., and Jamalirad, L. (2021). The effect of using PET to Paulownia strands on physical and mechanical properties of OSB. For. Wood Prod. 74, 371–382.
E Silva, I. M. M., and Costa, H. K. M. (2021). The United Kingdom’s experience in Carbon Capture and Storage projects: the current regulatory framework and related challenges. Carbon Capture Storage Int. Energy Policy Law, 141–153. doi:10.1016/B978-0-323-85250-0.00001-3
Ferguson, I., Bull, L., and Gorrie, G. (2010). Improving the investment climate for Australian forest industries. Growing green assets: Removing constraints to private sector investment in forestry in Asia and the Pacific, 27.
Fos, M., Oliver-Villanueva, J.-V., and Vazquez, M. (2023). Radial variation in anatomical wood characteristics and physical properties of Paulownia elongata x Paulownia fortunei hybrid Cotevisa 2 from fast-growing plantations. Eur. J. Wood Wood Prod. 81, 819–831. doi:10.1007/s00107-023-01941-8
Franz, E. (2007). From ornamental to detrimental? The incipient invasion of Central Europe by Paulownia tomentosa. Preslia 79, 377–389.
Fuhrer, E., and Molnar, S. (2003). The amount of carbon stored in the live matter in Hungarian forests. Faipar (Hungary).
Galán-Martín, Á., Pozo, C., Guillén-Gosálbez, G., Vallejo, A. A., and Esteller, L. J. (2015). Multi-stage linear programming model for optimizing cropping plan decisions under the new Common Agricultural Policy. Land use policy 48, 515–524. doi:10.1016/j.landusepol.2015.06.022
Ganchev, G., Ilchev, A., and Koleva, A. (2019). Digestibility and energy content of Paulownia (Paulownia elongata SY Hu) leaves. Agric. Sci. Technol. (1313-8820), 11.
Guidi, L., Lo Piccolo, E., and Landi, M. (2019). Chlorophyll fluorescence, photoinhibition and abiotic stress: does it make any difference the fact to be a C3 or C4 species? Front. plant Sci. 10, 174. doi:10.3389/fpls.2019.00174
Gyuleva, V. (2010). Micropropagation of hybryd paulownia from long-term preserved seeds. Silva Balcan 11, 45–58.
Gyuleva, V., Stankova, T., Zhiyanski, M., and Andonova, E. (2021). Five years growth of paulownia on two sites in Bulgaria. For. Sci 1, 11–22.
Haldar, A., and Sethi, N. (2021). Effect of institutional quality and renewable energy consumption on CO2 emissions− an empirical investigation for developing countries. Environ. Sci. Pollut. Res. 28, 15485–15503. doi:10.1007/s11356-020-11532-2
Hamdan, H. Z., and Houri, A. F. (2022). CO2 sequestration by propagation of the fast-growing Azolla spp. Environ. Sci. Pollut. Res. 29, 16912–16924. doi:10.1007/s11356-021-16986-6
Hatfield, R. D., Marita, J. M., Frost, K., Grabber, J., Ralph, J., Lu, F., et al. (2009). Grass lignin acylation: p-coumaroyl transferase activity and cell wall characteristics of C3 and C4 grasses. Planta 229, 1253–1267. doi:10.1007/s00425-009-0900-z
He, T., Vaidya, B., Perry, Z., Parajuli, P., and Joshee, N. (2016). Paulownia as a medicinal tree: traditional uses and current advances. Eur. J. Med. plants 14, 1–15. doi:10.9734/ejmp/2016/25170
Henry, B. (2023). Potential for soil carbon sequestration in Northern Australian grazing lands: a review of the evidence.
Hertel, D., Moser, G., Culmsee, H., Erasmi, S., Horna, V., Schuldt, B., et al. (2009). Below-and above-ground biomass and net primary production in a paleotropical natural forest (Sulawesi, Indonesia) as compared to neotropical forests. For. Ecol. Manag. 258, 1904–1912. doi:10.1016/j.foreco.2009.07.019
Hiloidhari, M., Baruah, D., Kumari, M., Kumari, S., and Thakur, I. (2019). Prospect and potential of biomass power to mitigate climate change: a case study in India. J. Clean. Prod. 220, 931–944. doi:10.1016/j.jclepro.2019.02.194
Hong, W. Y. (2022). A techno-economic review on carbon capture, utilisation and storage systems for achieving a net-zero CO2 emissions future. Carbon Capture Sci. Technol. 3, 100044. doi:10.1016/j.ccst.2022.100044
Huang, H., Szumacher-Strabel, M., Patra, A. K., Ślusarczyk, S., Lechniak, D., Vazirigohar, M., et al. (2021). Chemical and phytochemical composition, in vitro ruminal fermentation, methane production, and nutrient degradability of fresh and ensiled Paulownia hybrid leaves. Animal Feed Sci. Technol. 279, 115038. doi:10.1016/j.anifeedsci.2021.115038
Huseinovic, S., Osmanović, Z., Bektić, S., and Ahmetbegović, S. (2017). Paulownia elongata sy hu in function of improving the quality of the environment. Periodicals Eng. Nat. Sci. (PEN) 5. doi:10.21533/pen.v5i2.83
Icka, P., Damo, R., and Icka, E. (2016a). Paulownia tomentosa, a fast growing timber. Ann. Valahia Univ. Targoviste Agric. 10, 14–19. doi:10.1515/agr-2016-0003
Icka, P., Damo, R., and Icka, E. (2016b). Paulownia tomentosa, a fast growing timber. Ann. Valahia Univ. Targoviste, Agric. 10, 14–19. doi:10.1515/agr-2016-0003
Ince, O. F. (2022). Economic and environmental effects of improved forest management and loblolly pine genotypes on carbon sequestration in the southeast US. University of Georgia.
Jakubowski, M. (2022). Cultivation potential and uses of paulownia wood: a review. Forests 13, 668. doi:10.3390/f13050668
Jakubowski, M., Tomczak, A., Jelonek, T., and Grzywiński, W. (2018). Wykorzystanie drewna i możliwości uprawy drzew z rodzaju paulownia. Acta Sci. Polonorum Silvarum Colendarum Ratio Industria Lignaria 17.
Jamil, K., Liu, D., Gul, R. F., Hussain, Z., Mohsin, M., Qin, G., et al. (2021). Do remittance and renewable energy affect CO2 emissions? An empirical evidence from selected G-20 countries. Energy and Environ., 0958305X211029636.
Janjić, Z., and Janjić, M. (2019). Paulownia, characteristics and perspectives of its exploitation. Innovation Woodwork. Industry Eng. Des., 34–41.
Jo, H.-K. (2002). Impacts of urban greenspace on offsetting carbon emissions for middle Korea. J. Environ. Manag. 64, 115–126. doi:10.1006/jema.2001.0491
Joshi, N. R. (2015). Development of allometric equations for Paulownia tomentosa (Thunb.) to estimate biomass and carbon stocks: an assessment from the ICIMOD Knowledge Park, Godavari, Nepal. Int. Centre Integr. Mt. Dev.
Kadlec, J., Novosadová, K., and Pokorný, R. (2021). Preliminary results from a plantation of semi-arid hybrid of Paulownia Clone in vitro 112® under conditions of the Czech Republic from the first two years. Balt. 27, 18–25. doi:10.46490/bf477
Kaletnik, G., Pryshliak, N., and Tokarchuk, D. (2021). Potential of production of energy crops in Ukraine and their processing on solid biofuels. Ecol. Engeneering Environ. Technol. 22. 3, 59–70. doi:10.12912/27197050/135447
Keith, D. W., Holmes, G., Angelo, D. S., and Heidel, K. (2018). A process for capturing CO2 from the atmosphere. Joule 2, 1573–1594. doi:10.1016/j.joule.2018.05.006
Khan, F. A., and Ansari, A. A. (2005). Eutrophication: an ecological vision. botanical Rev. 71, 449–482. doi:10.1663/0006-8101(2005)071[0449:eaev]2.0.co;2
Khanjanzadeh, H., Bahmani, A. A., Rafighi, A., and Tabarsa, T. (2012). Utilization of bio-waste cotton (Gossypium hirsutum L.) stalks and underutilized paulownia (paulownia fortunie) in wood-based composite particleboard. Afr. J. Biotechnol. 11, 8045–8050. doi:10.5897/ajb12.288
Kheyrodin, H., and Kheyrodin, S. (2017). CO2 gas exchange in Crassulacean acid metabolism and C3 and C4 plants. Int. J. Adv. Res. Biol. Sci. 4, 36–43.
Kircher, M. (2022). Economic trends in the transition into a circular bioeconomy. J. Risk Financial Manag. 15, 44. doi:10.3390/jrfm15020044
Kirikkaleli, D., Güngör, H., and Adebayo, T. S. (2022). Consumption-based carbon emissions, renewable energy consumption, financial development and economic growth in Chile. Bus. Strategy Environ. 31, 1123–1137. doi:10.1002/bse.2945
Kobayashi, S., Asai, T., Fujimoto, Y., and Kohshima, S. (2008). Anti-herbivore structures of Paulownia tomentosa: morphology, distribution, chemical constituents and changes during shoot and leaf development. Ann. Bot. 101, 1035–1047. doi:10.1093/aob/mcn033
Kozakiewicz, P., Laskowka, A., and Ciołek, S. (2020). A study of selected features of Shan Tong variety of plantation paulownia and its wood properties. Ann. Wars. Univ. Life Sci. SGGW For. Wood Technol 111, 116–123. doi:10.5604/01.3001.0014.6904
Kralicek, K., Huy, B., Poudel, K. P., Temesgen, H., and Salas, C. (2017). Simultaneous estimation of above-and below-ground biomass in tropical forests of Viet Nam. For. Ecol. Manag. 390, 147–156. doi:10.1016/j.foreco.2017.01.030
Lachowicz, H., and Giedrowicz, A. (2020a). Characteristics of the technical properties of Paulownia COTE-2 wood. Sylwan 164, 414–423.
Lachowicz, H., and Giedrowicz, A. (2020b). Charakterystyka jakości technicznej drewna paulowni COTE− 2. Sylwan 164, 414–423.
Lefvert, A., Rodriguez, E., Fridahl, M., Grönkvist, S., Haikola, S., and Hansson, A. (2022). What are the potential paths for carbon capture and storage in Sweden? A multi-level assessment of historical and current developments. Energy Res. Soc. Sci. 87, 102452. doi:10.1016/j.erss.2021.102452
Li, H., Jiang, X., Ramaswamy, H. S., Zhu, S., and Yu, Y. (2018). High-pressure treatment effects on density profile, surface roughness, hardness, and abrasion resistance of paulownia wood boards. Trans. ASABE 61, 1181–1188. doi:10.13031/trans.12718
Li, P., Lou, G., Cai, X., Zhang, B., Cheng, Y., and Wang, H. (2020). Comparison of the complete plastomes and the phylogenetic analysis of Paulownia species. Sci. Rep. 10, 2225. doi:10.1038/s41598-020-59204-y
Macdicken, K. G. (1997). A guide to monitoring carbon storage in forestry and agroforestry projects.
Madejón, P., Alaejos, J., García-Álbala, J., Fernández, M., and Madejón, E. (2016). Three-year study of fast-growing trees in degraded soils amended with composts: effects on soil fertility and productivity. J. Environ. Manag. 169, 18–26. doi:10.1016/j.jenvman.2015.11.050
Madhoushi, M., and Boskabadi, Z. (2019). Relationship between the dynamic and static modulus of elasticity in standing trees and sawn lumbers of Paulownia fortune planted in Iran. Maderas. Cienc. Tecnol. 21, 35–44.
Magar, L. B., Khadka, S., Joshi, J. R. R., Pokharel, U., Rana, N., Thapa, P., et al. (2018). Total biomass carbon sequestration ability under the changing climatic condition by Paulownia tomentosa Steud. Int. J. Appl. Sci. Biotechnol. 6, 220–226. doi:10.3126/ijasbt.v6i3.20772
Magar, L. B., Shrestha, N., Khadka, S., Joshi, J. R., Acharya, J., Gyanwali, G. C., et al. (2016). Challenges and opportunity of in vitro propagation of Paulownia tomentosa Steud for commercial production in Nepal. Int. J. Appl. Sci. Biotechnol. 4, 155–160. doi:10.3126/ijasbt.v4i2.14752
Mansour, A. T., Ashour, M., Alprol, A. E., and Alsaqufi, A. S. (2022). Aquatic plants and aquatic animals in the context of sustainability: cultivation techniques, integration, and blue revolution. Sustainability 14, 3257. doi:10.3390/su14063257
Marana, B. (2018). A green GIS solution against air pollution in the Province of bergamo: the paulownia tree. J. Geogr. Inf. Syst. 10, 193–218. doi:10.4236/jgis.2018.102010
Martínez Pastur, G., Perera, A. H., Peterson, U., and Iverson, L. R. (2018). Ecosystem services from forest landscapes: an overview. Ecosyst. Serv. For. landscapes broadscale considerations, 1–10.
Miladinova-Georgieva, K., Geneva, M., and Markovska, Y. (2018a). Effects of EDTA and citrate addition to the soil on C4 photosynthetic enzymes and biochemical indicators for heavy metal tolerance in two paulownia hybrids. Plant Physiol. 8, 68–81.
Miladinova-Georgieva, K., Ivanova, K., Georgieva, T., Geneva, M., Petrov, P., Stancheva, I., et al. (2018b). EDTA and citrate impact on heavy metals phytoremediation using paulownia hybrids. Int. J. Environ. Pollut. 63, 31–46. doi:10.1504/ijep.2018.092985
Mohamad, M. E., Awad, A., Majrashi, A., Abd Esadek, O., El-Saadony, M. T., Saad, A. M., et al. (2022). In vitro study on the effect of cytokines and auxins addition to growth medium on the micropropagation and rooting of Paulownia species (Paulownia hybrid and Paulownia tomentosa). Saudi J. Biol. Sci. 29, 1598–1603. doi:10.1016/j.sjbs.2021.11.003
Mondal, M., Goswami, S., Ghosh, A., Oinam, G., Tiwari, O., Das, P., et al. (2017). Production of biodiesel from microalgae through biological carbon capture: a review. 3 Biotech. 7, 99–21. doi:10.1007/s13205-017-0727-4
Morozova, I., Oechsner, H., Roik, M., Hülsemann, B., and Lemmer, A. (2020). Assessment of areal methane yields from energy crops in Ukraine, best practices. Appl. Sci. 10, 4431. doi:10.3390/app10134431
Nelis, P. A., Henke, O., and Mai, C. (2019). Comparison of blockboards with core layers made of kiri (Paulownia spp.) and of spruce (Picea abies) regarding mechanical properties. Eur. J. Wood Wood Prod. 77, 323–326. doi:10.1007/s00107-019-01381-3
Nelis, P. A., and Mai, C. (2021). The influence of low-density (Paulownia spp.) and high-density (Fagus sylvatica L.) wood species on various characteristics of light and medium-density three-layered particleboards. Wood Material Sci. Eng. 16, 21–26. doi:10.1080/17480272.2019.1659850
Nelis, P. A., Michaelis, F., Krause, K. C., and Mai, C. (2018). Kiri wood (Paulownia tomentosa): can it improve the performance of particleboards? Eur. J. wood wood Prod. 76, 445–453. doi:10.1007/s00107-017-1222-7
Newell, R., Raimi, D., Villanueva, S., and Prest, B. (2021). Global energy outlook 2021: pathways from Paris. Resour. Future 8.
Newman, S., Bennett, K., and Wu, Y. (1997). Performance of maize, beans and ginger as intercrops in Paulownia plantations in China. Agrofor. Syst. 39, 23–30. doi:10.1023/a:1005938310106
Nouri, H., Borujeni, S. C., and Hoekstra, A. Y. (2019). The blue water footprint of urban green spaces: an example for Adelaide, Australia. Landsc. urban Plan. 190, 103613. doi:10.1016/j.landurbplan.2019.103613
Nowak, B., Moniuszko-Szajwaj, B., Skorupka, M., Puchalska, J., Kozłowska, M., Bocianowski, J., et al. (2022). Effect of paulownia leaves extract levels on in vitro ruminal fermentation, microbial population, methane production, and fatty acid biohydrogenation. Molecules 27, 4288. doi:10.3390/molecules27134288
Nowak, D. J., and Crane, D. E. (2002). Carbon storage and sequestration by urban trees in the USA. Environ. Pollut. 116, 381–389. doi:10.1016/s0269-7491(01)00214-7
Nunez, C. (2019). Carbon dioxide levels are at a record high. National geographic, 13. Here’s what you need to know.
Olave, R., Forbes, G., Muñoz, F., and Lyons, G. (2015). Survival, early growth and chemical characteristics of Paulownia trees for potential biomass production in a cool temperate climate. Ir. For.
Oliveira, N., Pérez-Cruzado, C., Cañellas, I., Rodríguez-Soalleiro, R., and Sixto, H. (2020). Poplar short rotation coppice plantations under Mediterranean conditions: the case of Spain. Forests 11, 1352. doi:10.3390/f11121352
Ols, C., and Bontemps, J.-D. (2021). Pure and even-aged forestry of fast-growing conifers under climate change: on the need for a silvicultural paradigm shift. Environ. Res. Lett. 16, 024030. doi:10.1088/1748-9326/abd6a7
Osman, A. I., Hefny, M., Abdel Maksoud, M., Elgarahy, A. M., and Rooney, D. W. (2021). Recent advances in carbon capture storage and utilisation technologies: a review. Environ. Chem. Lett. 19, 797–849. doi:10.1007/s10311-020-01133-3
Palma, A., Loaiza, J. M., Díaz, M. J., García, J. C., Giráldez, I., and López, F. (2021). Tagasaste, leucaena and paulownia: three industrial crops for energy and hemicelluloses production. Biotechnol. Biofuels 14, 89–14. doi:10.1186/s13068-021-01930-0
Parra-Lopez, C., Sayadi-Gmada, S., and Duran-Zuazo, V. H. (2015). Production and use of biomass from short-rotation plantations in Andalusia, southern Spain: limitations and opportunities. New Medit. 14, 40–49.
Pedersen, J. T. S., Van Vuuren, D., Gupta, J., Santos, F. D., Edmonds, J., and Swart, R. (2022). IPCC emission scenarios: how did critiques affect their quality and relevance 1990–2022? Glob. Environ. Change 75, 102538. doi:10.1016/j.gloenvcha.2022.102538
Pieratti, D. (2020). Published. Writing like a reader: the power of imitation in writing instruction. MLA Annual Convention. MLA.
Pleguezuelo, C. R. R., Zuazo, V. H. D., Bielders, C., Bocanegra, J. A. J., Pereatorres, F., and Martínez, J. R. F. (2015). Bioenergy farming using woody crops. A review. Agron. Sustain. Dev. 35, 95–119. doi:10.1007/s13593-014-0262-1
Popescu, A., and Sabau, L. (2016). “Paulownia Species” growing for saplings in pots in Romania: technological aspects and comparative expenses, incomes and profit. Sci. Pap. Ser. Manag. Econ. Eng. Agric. Rural. Dev. 16, 255–265.
Pörtner, H.-O., Roberts, D. C., Adams, H., Adler, C., Aldunce, P., Ali, E., et al. (2022). Climate change 2022: impacts, adaptation and vulnerability. IPCC Sixth Assessment Report.
Pożoga, M., Olewnicki, D., and Jabłońska, L. (2019). In vitro propagation protocols and variable cost comparison in commercial production for paulownia tomentosa × paulownia fortunei hybrid as a renewable energy source. Appl. Sci. 9, 2272. doi:10.3390/app9112272
Prasad, R., Gupta, S. K., Shabnam, N., Oliveira, C. Y. B., Nema, A. K., Ansari, F. A., et al. (2021). Role of microalgae in global CO2 sequestration: physiological mechanism, recent development, challenges, and future prospective. Sustainability 13, 13061. doi:10.3390/su132313061
Ram, S., Majumder, S., Chaudhuri, P., Chanda, S., Santra, S., Chakraborty, A., et al. (2015). A review on air pollution monitoring and management using plants with special reference to foliar dust adsorption and physiological stress responses. Crit. Rev. Environ. Sci. Technol. 45, 2489–2522. doi:10.1080/10643389.2015.1046775
Robards, T. (2008). A review of the accuracy of urban forestry biomass functions: utility for the California Climate Action Registry Protocol.
Rodríguez-Seoane, P., Domínguez, H., and Torres, M. D. (2020). Mechanical characterization of biopolymer-based hydrogels enriched with Paulownia extracts recovered using a green technique. Appl. Sci. 10, 8439. doi:10.3390/app10238439
Roeland, S., Moretti, M., Amorim, J. H., Branquinho, C., Fares, S., Morelli, F., et al. (2019). Towards an integrative approach to evaluate the environmental ecosystem services provided by urban forest. J. For. Res. 30, 1981–1996. doi:10.1007/s11676-019-00916-x
Sacchelli, S., Fabbrizzi, S., Bertocci, M., Marone, E., Menghini, S., and Bernetti, I. (2017). A mix-method model for adaptation to climate change in the agricultural sector: a case study for Italian wine farms. J. Clean. Prod. 166, 891–900. doi:10.1016/j.jclepro.2017.08.095
Sage, R. F., and Sultmanis, S. (2016). Why are there no C4 forests? J. plant physiology 203, 55–68. doi:10.1016/j.jplph.2016.06.009
Santori, G., Charalambous, C., Ferrari, M.-C., and Brandani, S. (2018). Adsorption artificial tree for atmospheric carbon dioxide capture, purification and compression. Energy 162, 1158–1168. doi:10.1016/j.energy.2018.08.090
Santos, M. G., Davey, P. A., Hofmann, T. A., Borland, A., Hartwell, J., and Lawson, T. (2022). Stomatal and mesophyll responses tissue to in light, Vicia faba CO2, and kalanchoë fedtschenkoi. Stomatal Biol. Beyond.
Schneiderová, K., and Šmejkal, K. (2015). Phytochemical profile of paulownia tomentosa (thunb). Steud. Phytochem. Rev. 14, 799–833. doi:10.1007/s11101-014-9376-y
Secinaro, S., Brescia, V., Calandra, D., and Biancone, P. (2020). Employing bibliometric analysis to identify suitable business models for electric cars. J. Clean. Prod. 264, 121503. doi:10.1016/j.jclepro.2020.121503
Sharma, R., Pradhan, L., Kumari, M., and Bhattacharya, P. (2020). Assessment of carbon sequestration potential of tree species in amity university campus Noida. Environ. Sci. Proc. 3, 52.
Shreyash, N., Sonker, M., Bajpai, S., Tiwary, S. K., Khan, M. A., Raj, S., et al. (2021). The review of carbon capture-storage technologies and developing fuel cells for enhancing utilization. Energies 14, 4978. doi:10.3390/en14164978
Sikkema, R., Proskurina, S., Banja, M., and Vakkilainen, E. (2021). How can solid biomass contribute to the EU’s renewable energy targets in 2020, 2030 and what are the GHG drivers and safeguards in energy-and forestry sectors? Renew. Energy 165, 758–772. doi:10.1016/j.renene.2020.11.047
Silva Araújo, J., Aires Souza, J. T., Dos Santos Félix, E., De Cássia Alves, R., De Oliveira Filho, T. J., and Cunha De Lira, E. (2022). Gas exchange in genotypes of Nopalea cochenillifera in different seasons and evaluations times. Acta Bot. Croat. 81, 177–184. doi:10.37427/botcro-2022-015
Singh, S., Bhattacharya, P., and Gupta, N. (2018). Dust particles characterization and innate resistance for Thevetia peruviana in different land-use pattern of urban area. Int. J. Environ. Sci. Technol. 15, 1061–1072. doi:10.1007/s13762-017-1461-5
Šmejkal, K., Grycová, L., Marek, R., Lemiere, F., Jankovská, D., Forejtníková, H., et al. (2007). C-geranyl compounds from Paulownia tomentosa fruits. J. Nat. Prod. 70, 1244–1248. doi:10.1021/np070063w
Snow, W. A. (2015). Ornamental, crop, or invasive? The history of the Empress tree (Paulownia) in the USA. For. Trees Livelihoods 24, 85–96. doi:10.1080/14728028.2014.952353
Stankova, T., Gyuleva, V., Dimitrov, D., and Popov, E. (2019). Allometric relationships for estimation of aboveground woody biomass of two clones Paulownia at juvenile age. Nauka za gorata 55, 43–54.
Stephenson, P. G., Moore, C. M., Terry, M. J., Zubkov, M. V., and Bibby, T. S. (2011). Improving photosynthesis for algal biofuels: toward a green revolution. Trends Biotechnol. 29, 615–623. doi:10.1016/j.tibtech.2011.06.005
Steyn, M., and Havercroft, I. (2021). Brief: inclusion of a CCS method under the emissions reduction fund.
Strohbach, M. W., Arnold, E., and Haase, D. (2012). The carbon footprint of urban green space—a life cycle approach. Landsc. Urban Plan. 104, 220–229. doi:10.1016/j.landurbplan.2011.10.013
Stuepp, C. A., Zuffellato-Ribas, K. C., Koehler, H. S., and Wendling, I. (2015). Rooting mini-cuttings of Paulownia fortunei var. mikado derived from clonal mini-garden. Rev. Árvore 39, 497–504. doi:10.1590/0100-67622015000300010
Suryawanshi, M., Patel, A., Kale, T., and Patil, P. (2014). Carbon sequestration potential of tree species in the environment of North Maharashtra University Campus, Jalgaon (MS) India. Biosci. Discov. 5, 175–179.
Temirov, J., Shukurova, G., and Klichov, I. (2021). “Published. Study on the influence of stimulants on the rooting of the paulownia (paulownia) and tulip (liriodendron tulipifera) trees during the propagation by cuttings,” in IOP Conference Series: Earth and Environmental Science (IOP Publishing).
Testa, R., Schifani, G., Rizzo, G., and Migliore, G. (2022). Assessing the economic profitability of Paulownia as a biomass crop in Southern Mediterranean area. J. Clean. Prod. 336, 130426. doi:10.1016/j.jclepro.2022.130426
Thevs, N., Baier, C., and Aliev, K. (2021). Water productivity of poplar and paulownia on two sites in Kyrgyzstan, central Asia. J. Water Resour. Prot. 13, 293–308. doi:10.4236/jwarp.2021.134018
Turan, G., Zapantis, A., Kearns, D., Tamme, E., Staib, C., Zhang, T., et al. (2021). Global status of CCS 2021. CCS Accelerating to net zero.
Turner, K., Race, J., Alabi, O., Katris, A., and Swales, J. K. (2021). Policy options for funding carbon capture in regional industrial clusters: what are the impacts and trade-offs involved in compensating industry competitiveness loss? Ecol. Econ. 184, 106978. doi:10.1016/j.ecolecon.2021.106978
Tzvetkova, N., Miladinova, K., Ivanova, K., Georgieva, T., Geneva, M., and Markovska, Y. (2015). Possibility for using of two Paulownia lines as a tool for remediation of heavy metal contaminated soil. J. Environ. Biol. 36, 145–151.
Van Der Gaast, W., Sikkema, R., and Vohrer, M. (2018). The contribution of forest carbon credit projects to addressing the climate change challenge. Clim. Policy 18, 42–48. doi:10.1080/14693062.2016.1242056
Vega, F., Baena-Moreno, F., Fernandez, L. M. G., Portillo, E., Navarrete, B., and Zhang, Z. (2020). Current status of CO2 chemical absorption research applied to CCS: towards full deployment at industrial scale. Appl. Energy 260, 114313. doi:10.1016/j.apenergy.2019.114313
Verma, P., and Ghosh, P. K. (2023). The economics of Forest carbon sequestration: a bibliometric analysis. Environ. Dev. Sustain. 26, 2989–3019. doi:10.1007/s10668-023-02922-w
Wang, T., Zhang, X., Ma, Y., and Wang, Y. (2023). Risk contagion and decision-making evolution of carbon market enterprises: comparisons with China, the United States, and the European Union. Environ. Impact Assess. Rev. 99, 107036. doi:10.1016/j.eiar.2023.107036
Winter, K., and Holtum, J. A. (2017). Facultative crassulacean acid metabolism (CAM) in four small C3 and C4 leaf-succulents. Aust. J. Bot. 65, 103–108. doi:10.1071/bt16015
Woods, V. (2008a). Paulownia as a novel biomass crop for northern Ireland? Agri-food and bioscience Institute. Occasional publication.
Woods, V. (2008b). “Paulownia as a novel biomass crop for Northern Ireland? a review of current knowledge,” in Hillsborough, Co. Down: agri-food and biosciences Institute, global research unit (Occasional Publication).
Wu, L., Wang, B., Qiao, J., Zhou, H., Wen, R., Xue, J., et al. (2014). Effects of trunk-extension pruning at different intensities on the growth and trunk form of Paulownia fortunei. For. Ecol. Manag. 327, 128–135. doi:10.1016/j.foreco.2014.05.008
Yadav, N. K., Vaidya, B. N., Henderson, K., Lee, J. F., Stewart, W. M., Dhekney, S. A., et al. (2013). A review of Paulownia biotechnology: a short rotation, fast growing multipurpose bioenergy tree. Am. J. Plant Sci. 4, 2070–2082. doi:10.4236/ajps.2013.411259
Yan, Y. H., Borhani, T., Subraveti, G., Pai, N., Prasad, V., Rajendran, A., et al. (2021). Harnessing the power of machine learning for carbon capture, utilisation, and storage (CCUS)–a state-of-the-art review. Energy and Environ. Sci. 14, 6122–6157. doi:10.1039/d1ee02395k
Yang, H., Zhang, P., Xu, X., Chen, X., Liu, Q., and Jiang, C. (2019). The enhanced immunological activity of Paulownia tomentosa flower polysaccharide on Newcastle disease vaccine in chicken. Biosci. Rep. 39. doi:10.1042/bsr20190224
Yi, W., Nadeem, F., Xu, G., Zhang, Q., Joshee, N., and Tahir, N. (2020). Modifying crystallinity, and thermo-optical characteristics of Paulownia biomass through ultrafine grinding and evaluation of biohydrogen production potential. J. Clean. Prod. 269, 122386. doi:10.1016/j.jclepro.2020.122386
Yu, Y., Jiang, X., Ramaswamy, H. S., Zhu, S., and Li, H. (2018). Effect of high-pressure densification on moisture sorption properties of Paulownia wood. BioResources 13, 2473–2486. doi:10.15376/biores.13.2.2473-2486
Yuen, J. Q., Ziegler, A. D., Webb, E. L., and Ryan, C. M. (2013). Uncertainty in below-ground carbon biomass for major land covers in Southeast Asia. For. Ecol. Manag. 310, 915–926. doi:10.1016/j.foreco.2013.09.042
Zhang, C., Chen, X., Wang, J., and Tan, L. (2017). Toxic effects of microplastic on marine microalgae Skeletonema costatum: interactions between microplastic and algae. Environ. Pollut. 220, 1282–1288. doi:10.1016/j.envpol.2016.11.005
Zhang, Q., Jin, P., Li, Y., Zhang, Z., Zhang, H., Ru, G., et al. (2022). Analysis of the characteristics of paulownia lignocellulose and hydrogen production potential via photo fermentation. Bioresour. Technol. 344, 126361. doi:10.1016/j.biortech.2021.126361
Zhao, Y., Qiao, J., Feng, Y., Wang, B., Duan, W., Zhou, H., et al. (2019). The optimal size of a Paulownia-crop agroforestry system for maximal economic return in North China Plain. Agric. For. Meteorology 269, 1–9. doi:10.1016/j.agrformet.2019.01.043
Zhu, X.-G., Long, S. P., and Ort, D. R. (2010). Improving photosynthetic efficiency for greater yield. Annu. Rev. plant Biol. 61, 235–261. doi:10.1146/annurev-arplant-042809-112206
Zhu, Z.-H., Chao, C.-J., Lu, X.-Y., and Xiong, Y. G. (1986). Paulownia in China: cultivation and utilization. International Development Research Centre.
Ziegler, A. D., Phelps, J., Yuen, J. Q., Webb, E. L., Lawrence, D., Fox, J. M., et al. (2012). Carbon outcomes of major land-cover transitions in SE Asia: great uncertainties and REDD+ policy implications. Glob. change Biol. 18, 3087–3099. doi:10.1111/j.1365-2486.2012.02747.x
Keywords: global warming, carbon concentrating mechanism, carbon pathways, Paulownia tree, Rubisco, PEPCase, carbon dioxide biofixation
Citation: Ghazzawy HS, Bakr A, Mansour AT and Ashour M (2024) Paulownia trees as a sustainable solution for CO2 mitigation: assessing progress toward 2050 climate goals. Front. Environ. Sci. 12:1307840. doi: 10.3389/fenvs.2024.1307840
Received: 05 October 2023; Accepted: 12 February 2024;
Published: 28 February 2024.
Edited by:
Haipeng Yu, Chinese Academy of Sciences (CAS), ChinaReviewed by:
Nadeem Tahir, Henan Agricultural University, ChinaManoj Kumar Jhariya, Sant Gahira Guru Vishwavidyalaya, India
Copyright © 2024 Ghazzawy, Bakr, Mansour and Ashour. This is an open-access article distributed under the terms of the Creative Commons Attribution License (CC BY). The use, distribution or reproduction in other forums is permitted, provided the original author(s) and the copyright owner(s) are credited and that the original publication in this journal is cited, in accordance with accepted academic practice. No use, distribution or reproduction is permitted which does not comply with these terms.
*Correspondence: Hesham S. Ghazzawy, aGdoYXp6YXd5QGtmdS5lZHUuc2E=; Mohamed Ashour, bWljcm9hbGdhZV9lZ3lwdEB5YWhvby5jb20=