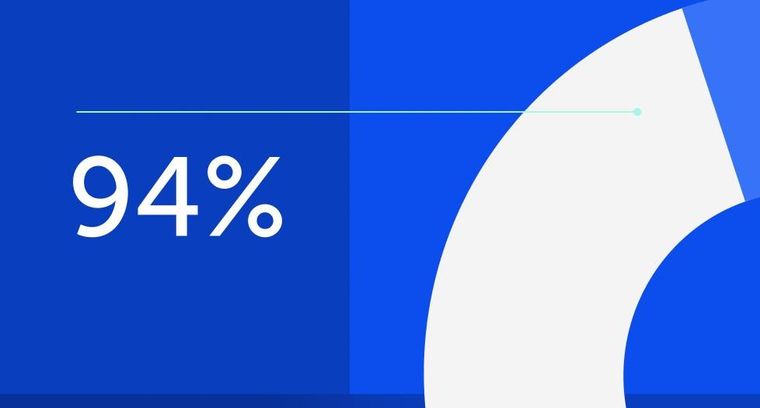
94% of researchers rate our articles as excellent or good
Learn more about the work of our research integrity team to safeguard the quality of each article we publish.
Find out more
ORIGINAL RESEARCH article
Front. Environ. Sci., 16 November 2023
Sec. Soil Processes
Volume 11 - 2023 | https://doi.org/10.3389/fenvs.2023.1309106
This article is part of the Research TopicEffects and Challenges of Ecological Protection and Restoration Strategies on Elemental CyclesView all 8 articles
Although agro-geotextile (AGT) emplacement shows potential to mitigate soil loss and, thus, increase carbon sequestration, comprehensive information is scanty on the impact of using agro-geotextiles on soil organic carbon (SOC) sequestration, aggregate-associated C, and soil loss in the foothills of the Indian Himalayan Region. We evaluated the impacts of Arundo donax AGT in different configurations on SOC sequestration, aggregate stability, and carbon management index (CMI) since 2017 under maize-based cropping systems on a 4% land slope, where eight treatment procedures were adopted. The results revealed that A. donax placement at 0.5-m vertical-interval pea–wheat (M + AD10G0.5-P-W) treatment had ∼23% increase in SOC stock (27.87 Mg·ha−1) compared to the maize–wheat (M-W) system in the 0–30-cm soil layer. M + AD10G0.5-P-W and maize–pea–wheat treatments under bench terracing (M-P-W)BT had similar impacts on SOC stocks in that layer after 5 years of cropping. The total SOC values in bulk soils, macroaggregates, and microaggregates were ∼24, 20, and 31% higher, respectively, in plots under M + AD10G0.5-P-W treatment than M-W in the topsoil (0–5 cm). The inclusion of post-rainy season vegetable pea in the maize–wheat cropping system, along with AGT application and crop residue management, generated additional biomass and enhanced CMI by ∼60% in the plots under M + AD10G0.5-P-W treatment over M-W, although M + AD10G0.5-P-W and (M-P-W)BT had similar effects in the topsoil. In the 5–15-cm layer, there was no significant effect of soil conservation practices on CMI values. Under the M + AD10G0.5-P-W treatment, the annual mean soil loss decreased by ∼92% over M-W treatment. We observed that CMI, proportion of macroaggregates, aggregate-associated C, labile C, total SOC concentration (thus, SOC accumulation rate), and mean annual C input were strongly correlated with the mean annual soil loss from 2017 to 2021. The study revealed that the emplacement of an A. donax mat and incorporation of a legume in a cropping system (M-W), conservation tillage, and crop residue retention not only prevented soil loss but also enhanced C sequestration compared to farmers’ practice (M-W) in the Indian Himalayas. The significance of this study is soil conservation, recycling of residues and weeds, and climate change adaptation and mitigation, as well as increasing farmers’ income.
Under a changing climate scenario, the Indian Himalayan Region (IHR) is projected to face an increase in the frequency of extreme rainfall events, characterized by precipitation rates exceeding 80–100 mm·h−1. This results in accelerated soil erosion in the region (Mandal and Sharda, 2011). The Himalayas are reported to have extraordinarily high soil erosion rates among the different regions of India, ranging from 20 to 25 Mg·ha−1·yr−1 to even 92 Mg·ha−1·yr−1, based on multiple field-based investigations at different locations and river basins (Sharda and Ojasvi, 2016; Swarnkar et al., 2018).
Soil erosion by water is directly related to the intensity of rainfall. Soil erosion is a naturally occurring process that results in the degradation of landforms and, in particular, results in topsoil loss by water flow. Along with topsoil, clay content and organic matter are also lost, which leads to degradation of cultivable and arable lands (Mandal et al., 2020). This leads to loss of soil organic carbon (SOC) and poor soil fertility in the Indian Himalayas (Bhattacharyya et al., 2009a). Soil conservation practices improve soil health by improving soil aggregation, reduce the risks of soil compaction, increase surface soil organic matter (SOM) and SOC contents, retain moderate soil temperature, and suppress weeds and pathogens (Somasundaram et al., 2020). Although soil properties have decreased over the years due to improper management practices (Singh et al., 2019), the adoption of the best management practices (conservation tillage, nutrient management, etc.) enhanced SOC, available N, P, and K contents of the Himalayan soils (Gogoi et al., 2021).
A potential method to prevent soil loss and degradation is the use of agro-geotextiles (AGTs). Using AGTs can increase the infiltration of water in soil and slow down runoff generation due to increased surface roughness of soil, directly impacting surface water flow velocity and decreasing the splash water erosion rate. It also improves SOM by improving the soil structure and aggregate stability (Bhattacharyya et al., 2010b). Bhattacharyya et al. (2009b)proposed that biological geotextiles could be a very successful and low-cost soil conservation technology with great worldwide potential. Soil erosion can be effectively controlled using vegetation strips. Geotextiles stretch over the soil surface as they become wet, improving their drapability (adherence to surface micro-topography) and, consequently, the ability to limit runoff and erosion (Bhattacharyya et al., 2010a).
In the foothills of the Indian Himalayas, AGT mats can be prepared from the robust stem and stiff leaves of Arundo donax when the culms reach a height of 15–20 feet, coupled with green compact leaf biomass and locally available sources, such as rope and plant fibers, at an economically viable price. A. donax as AGT, having rough texture, high water-holding capacity, and increased weight when wet, has significant characteristics that ensure physical contact between the soil and geotextiles, which can control soil erosion (Bhattacharyya et al., 2015a; Singh et al., 2019). Visconti et al. (2020)noted that the constant release of organic wastes by A. donax creates a mulching effect that may lessen the impact of severe rainfall contributing to soil erosion. Compared to fallow, A. donax drastically reduced the total runoff and sediment concentration by 65%, 78%, and 34% (63 m3·ha−1 vs. 181 m3·ha−1, 0.05 Mg·ha−1 vs. 0.21 Mg·ha−1, and 0.71 g·L−1 vs. 1.08 g·L−1, respectively) (Visconti et al., 2020). It also increased the SOC concentration (Fagnano et al., 2015). A. donax emplacement on soil is a low-cost practice as it is naturally grown, beside the main cropping field, only in torrent beds. When the culms of this perennial grass attain a height of 15–20 ft, along with green compact leaf biomass, it is harvested, and mats are prepared using its sturdy stem and stiff leaves by using some locally available sources (Singh et al., 2019). It is an extremely fast-growing plant with a higher density, which helps increase surface roughness and reduce soil loss, and after biodegradation, it adds nutrients to the soil. This enhances vegetative growth and root growth of test crops, resulting in higher soil aggregate stability. Reduced soil erosion in the plots under AGT further contributes to increased productivity and SOC sequestration. For soils in the Himalayas, minimum and zero tillage also lower cultivation costs, and increase soil water retention and physical protection of SOC (Bhattacharyya et al., 2009c; Bhattacharyya et al., 2012). On croplands with slopes of 2%–8%, conservation methods for soil and water, like contour farming, zero tillage, mulch application, and legume incorporation, not only decreased runoff by 8%–40% and soil loss by 6%–35% but also increased productivity by 3%–28% (Ghosh et al., 2012; Singh et al., 2019).
The application of AGT and adoption of a soil conservation method are helpful ameliorative measures to decrease soil erosion, crop production constraints associated with the soil, and carbon sequestration in high-rainfall areas. Although biological mat emplacement has positive impacts on SOC sequestration, the literature is scanty on its effects on SOC dynamics (C pools and soil aggregation). In conservation tillage, C sequestration increases and more C stability occurs, which increases the carbon management index (CMI) as well. Generally, an increase in CMI decreases soil loss (Ghosh et al., 2016). As a preliminary indicator of soil deterioration or improvement in response to various management practices, the computation of SOC lability within each management practice or land cover type can be used. The development of CMI has been applied to various land uses or management practices in order to employ more sensitive indicators to assess the ability of a land use or practice to improve the soil quality (Maini et al., 2020). Chemical extractants enable the classification of SOC into very labile C, labile C, less labile C, and non-labile C pools. Very labile and labile C pools constitute labile C, and recalcitrant C is the sum of less labile and non-labile C pools (Bongiorno et al., 2019). The labile and recalcitrant pools make up the total SOC. CMI could be calculated from these C pools. The physical fraction, which includes particle organic matter, the chemical fraction (KMnO4–C), and the biological fraction (which includes microbial biomass C), make up the labile organic carbon (LOC) fraction (Mandal et al., 2013). In order to understand soil C dynamics, it is important to understand the relative proportion of these fractions, which impacts the soil quality. LOC changes over a short period of time (1–2 years), and this pool can be used to evaluate the effects of land management (Jat et al., 2019).
Very little research on soil and water conservation has been conducted in the context of the concurrent use of conservation tillage and A. donax AGT in a rainfed maize–pea–wheat cropping system (Singh et al., 2019). Information on the impact of using AGT under conservation-tilled cropping systems on the depth distribution of SOC is scanty. We hypothesized that the use of different configurations of AGT in the no-till rainy season maize has a positive effect on carbon pools within different soil depths, aggregate stability, and CMI to enhance carbon sequestration and reduce soil loss. Thus, two post-monsoon rainfed crops (vegetable pea, followed by wheat) could be effectively raised that are also cultivated under conservation tillage. The aim of this study was to identify an appropriate soil conservation practice for improving soil carbon sequestration, aggregate stability, and CMI under maize-based cropping systems in the foothills of the Indian Himalayas.
The present experiment was carried out at the experimental farm at Selakui of the ICAR-Indian Institute of Soil and Water Conservation, Dehradun, India (Figure 1). The slope of the experimental site was 4%. The site comprises fine loamy mixed hyperthermic Udic Haplustalf soil (United States Department of Agriculture Natural Resource Conservation Service, 1996). The climate in the study region is subtropical, with hot summers and freezing winters. January is the coldest month with mean daily minimum temperatures ranging from 4°C to 5°C, whereas May and June are the hottest months with mean daily maximum temperatures ranging from 36°C to 37°C. The average annual rainfall is 1,615 mm, of which the southwest monsoon from July to September accounts for 80%.
Experiments were carried out on zero-tilled maize and minimum-tilled vegetable pea and wheat, planted in rainy (mid-June to mid-September), autumn (October to mid-December), and winter seasons (mid-December to April) from 2017 with eight treatment combinations. The crops, tillage, residue management, and details of emplacement of the Arundo donax mats are given in Table 1. The individual plot size was 100 m × 20 m, and there were four replications laid out in a randomized block design. The recommended amounts of N were applied to the maize crop in three equal splits at the seedling (10–15 days after sowing), knee height (30–35 days after sowing), and tasseling (45 days after sowing) stages of crop growth, respectively. Only 100 kg·ha−1 of di-ammonium phosphate (18-46-0) was used as a basal dose on vegetable pea. In a manner similar to that described previously, P and K were applied as a basal dose, along with 50% of N using a seed cum fertilizer drill, while the remaining N was top-dressed in two equal splits (at crown root initiation and booting stages that generally coincide with the first and second winter rains). Tank-mixed treatments of atrazine (1 kg a.i. ha−1), pendimethalin (1.5 kg a.i.·ha−1), and glyphosate (1.5 kg a.i.·ha−1) were used as pre-emergence herbicides to control weeds in zero-tilled maize. Handweeding was also carried out in maize 20 days after sowing (DAS). In all treatments, maize was given one tembotrione post-emergence spray between 20 and 25 DAS. One spray of Coragen® was used between 30 and 45 DAS to suppress autumn army worm in maize. After the pea pods were harvested, a disc harrow was used to fully incorporate the above-ground biomass (between 0.1 and 0.3 t·ha−1) into the soil. A tank mixture of metsulfuron–methyl (4.0 g a.i.·ha−1) and clodinafop–propargyl (60.0 g a.i.·ha−1) was sprayed at 35–40 DAS of wheat to control weeds in the crop. At the flowering stage in both years, a preventive spray of propicanazole (Tilt) was used to prevent fungal diseases (yellow rust and bunts) in the wheat crop. The treatment layout is given in Figure 2, and treatment-wise tillage and residue management practices are given in Table 1.
TABLE 1. Cropping systems and treatment details of soil conservation practices in the Indian Himalayas on a 4% slope.
FIGURE 2. (A) Plot without A. donax mat emplacement. (B) Plot with A. donax mat emplacement (5/10 cm thick) on a 1-m vertical interval. (C) Plot with A. donax mat emplacement (5/10 cm thick) on a 0.5-m vertical interval. (D) Plot with bench terrace practices.
After vegetable pea was harvested, two sets of undisturbed soil samples were collected from the 0–5, 5–15, 15–30, and 30–60-cm soil layers using a core sampler (5 cm diameter and 5.9 cm height). Samples from each soil depth were taken from each plot. One sample set was used to calculate the bulk density (BD) as the oven-dried core mass divided by the core volume (three cores). The second set of samples (three cores) was obtained from individual plots and completely combined before being air-dried and put through an 8-mm filter for aggregate separation. After processing, soil samples were utilized to determine certain soil properties. The values of the initial soil parameters were obtained from the study by Singh et al. (2019).
The aggregates were separated by size in several sieves using a wet sieving process adopted from the study by Elliott (1986). Using a series of three sieves (2, 0.25, and 0.053 mm), four aggregate fractions were obtained: 1) >2-mm (large macroaggregates (MAs)); 2) 0.25–2-mm (small macroaggregates); 3) 0.053–0.25-mm (microaggregates); and 4) <0.053-mm (silt plus clay-sized particles). Soil aggregate fractions that were retained on various sieves were dried in an oven at 40°C to determine the constant weight and then stored for total SOC analysis. The proportions of large macroaggregates (>2 mm) recovered after wet sieving weighed too little; thus, the large and small macroaggregates were combined to obtain the macroaggregates (Joseph et al., 2023).
Using a CHN analyzer (Foss Heraeus Elemental Analyzer CHN-O-RAPID, Anau, Germany), the total C concentrations were determined in bulk soils and aggregates. Since there were no inorganic carbonates in the samples, the total soil C measurement was interpreted to be similar to the total SOC value.
The protocol proposed by Chan et al. (2001) was used to divide the SOC concentration into separate pools using 12.0, 18.0, and 24.0 N H2SO4. This resulted in the division of the whole SOC into four distinct pools in the decreasing order of stability: pool 1, pool 2, pool 3, and pool 4. In contrast to pools 3 and 4, which together make up the recalcitrant pool, pools 1 and 2 are the labile pools (Chan et al., 2001). The method used by Tirol-Padre and Ladha (2004) was used to determine KMnO4–C.
Since the size of the overall pool and the lability (an estimation of the turnover rate) both need to be taken into account in order to develop a CMI, the consistency of the C supply is dependent on labile and recalcitrant C pools. The labile fraction of carbon can be estimated by first oxidizing some of the carbon in a soil sample by treating it with 333 mM KMnO4 (Blair et al., 1995).
CMI can then be calculated as (Blair et al., 1995)
where X is oxidized C by KMnO4–C, Y is remaining C un-oxidized by KMnO4, A is lability of C in the sample soil, B is lability of C in the reference soil, T is sample total C (mg g−l)/R = reference total C (mg g−l), P is the C pool index, and I is the lability index.
The mean annual total above-biomass residue addition (grain + straw) was estimated during 2017–2021. The average residue (kg·ha−1) added every year was estimated for root and crop stubble of wheat, vegetable pea, and maize; Arundo donax mat residue input; and weed biomass input. Replicated fresh samples of crop residues (stubble) and weed biomass were taken from the quadrats of 1.0 × 1.0 m2 in size, and AGT biomass was taken from the running length of 0.5 m (0.5 m × 0.5 m) at the time of maize harvest and then placed in an oven for 48 h to obtain the constant weight. The oven-dried biomass weight of residues was then converted to kg·ha−1.
The root biomass was calculated by multiplying the above-ground biomass of maize, vegetable pea, and wheat by the root-to-- shoot ratio, which was 0.13, 0.19, and 0.14 (Williams et al., 2013; Zhao et al., 2021), respectively. C concentrations of all residues/roots were analyzed using a CHN analyzer (Foss Heraeus Elemental Analyzer CHN-O-RAPID, Anau, Germany). The sum of biomass C inputs through stubble and root, as well as C input through residue retention, was used to calculate the cumulative C inputs to the soil (Kundu et al., 2007).
Soil organic carbon concentrations were converted to total SOC stocks in the corresponding soil layers as follows (Ellert and Bettany, 1995):
[where C is the total SOC concentration (g kg−1), D = BD (Mg m−3), and E is the depth (m)]
Soil organic carbon stock on an equivalent soil mass (ESM) basis.
The equivalent soil mass (ESM) method is described by Ellert and Bettany (1995) and Wendt and Hauser (2013), among others. We designated the original soil mass as ESM, and we evaluated soil C stocks using ESM as a base in order to account for the overestimation of the overall SOC stock in the soil with higher BD than the soil with smaller BD. The calculation procedure is as follows (Ellert and Bettany, 1995):
where Msoil is the soil mass and ESM is the equivalent soil.
where a is the total SOC concentration (g kg−1), b = (M soil–ESM), c is initial BD (Mg m−3), d is the depth (m), e represents treatment BD (Mg m−3), and f represents total SOC on a soil depth basis.
The following formula was used to determine the SOC accumulation rate (Bhattacharyya et al., 2015b):
where M and N denote the SOC stock (Mg ha−1) of a particular treatment and control plots, respectively. Here, the number of years of experiment (n) is 5 years. The M-W system is considered the control plot or farmers’ practice.
After each rainfall event during the maize growing season in all the years, runoff data from each plot were recorded at 8:00 a.m. (local time) using a stage-level recorder by measuring the hydrograph coupled with a Coshocton wheel (Sharma et al., 2017). In order to calculate soil loss, the collected runoff water was thoroughly stirred, and 1 L was withdrawn from each tank to estimate the amount of accumulated sediment in each plot’s run-off tank. Using Whatman 42 filter paper, the resultant suspensions were filtered. To determine the amount of soil lost, the sediment in the filter paper was oven-dried for 24 h at 105°C and weighed (Singh et al., 2019).
The data from the soil analyses were processed for analysis of variance (ANOVA) using SAS 9.3 with the methodology suggested by Gomez and Gomez (1984) using a randomized block design to examine differences among the treatment means (SAS Institute, Cary, North Carolina, United States). The significance level for Tukey’s HSD test was set at p < 0.05.
In the 0–5-cm soil layer, the bulk density varied from 1.32 to 1.36 Mg·m−3 (Figure 3). According to BD values from the topsoil (0–5 cm), M + AD10G0.5-P-W plots exhibited the lowest BD (1.32 Mg·m−3). Overall, it was observed that plots emplaced with A. donax mats had lower BD than those without A. donax mats, which can be attributed to the adoption of zero tillage, minimum tillage, and residue retention practices. These practices led to minimal soil disturbance, increased organic matter content, and improved soil structural characteristics, resulting in lower bulk density values than those in the control plots (Solomon et al., 2017; Worku, 2017; Meena et al., 2018). There were no significant differences in BD values in different treatments at lower depths (Figure 3).
FIGURE 3. Effect of different conservation practices on soil bulk density at different depths. Treatment details are given in Table 1. Tukey’s HSD test indicates that means with different lowercase letters within a soil depth are significantly different at p < 0.05.
Plots under M + AD10G0.5-P-W treatment had ∼34 and ∼37% higher MA than M-W plots in the topsoil and 5–15 cm soil layers, respectively (Figure 4). The M + AD10G0.5-P-W and (M-P-W)BT treatments had similar effects on the proportion of MA in the topsoil. There was ∼21% higher MA in plots emplaced with A. donax mats than in those without mats. Crop residue management considerably increased the proportion of macroaggregates in the residue-added plots due to the release of polysaccharides, formation of organic acids during degradation of crop residues, and increased concentration of glomalin (Singh et al., 2018).
FIGURE 4. Distribution (%) of soil aggregates in the (A) 0–5-cm and (B) 5–15-cm layers as affected by soil conservation practices. Treatment details are given in Table 1. Tukey’s HSD test indicates that means with different lowercase letters within an aggregate size are significantly different at p < 0.05.
Generally, conservation tillage in all treatments resulted in better soil aggregation. However, A. donax mat emplacement, residue retention, and cultivation of a cover crop caused improved soil macroaggregates (MAs), both in the topsoil and 5–15-cm layer. The higher proportion of MA in M + AD10G0.5-P-W treatment than M-W (Figure 4) was probably due to higher C input, slope protection, and root mass in surface soils, all of which resulted in decreased soil loss. Increased proportion of MA and decreased MI proportion with soil conservation practices enhanced soil aggregation due to zero tillage practiced in maize, minimum tillage in vegetable pea and wheat, and residue retention.
Across all soil depths, total SOC concentrations were considerably higher in M + AD10G0.5-P-W plots than in M-W plots. Legume incorporation in the M-W cropping system had a higher total SOC concentration in the topsoil than M-W plots (Table 3). The highest total SOC concentration was found in the maize + A. donax mat (10 cm thick) on 0.5-m vertical-interval vegetable pea–wheat (M + AD10G0.5-P-W) plots. In that depth, plots under M + AD10G0.5-P-W and (M-P-W)BT treatments had ∼24 and ∼21% more total SOC concentrations, respectively, than control (M-W) plots. In the 5–15-cm layer, M + AD10G0.5-P-W and (M-P-W)BT plots had ∼22 and ∼20% higher total SOC concentrations than M-W plots, respectively (Tables 2, 3). Plots under M + AD10G0.5-P-W treatment had ∼20 and ∼21% more total SOC concentrations than those of M-P-W plots in the topsoil and 5–15-cm soil layers, respectively (Tables 2, 3). In the 15–30 and 30–60-cm soil depths, there was no significant effect of soil conservation practices on total SOC concentrations (Table 4).
TABLE 4. Impacts of 5 years of different conservation practices on the total SOC and KMnO4−C concentrations (g kg-1) at 15–30-cm and 30–60-cm depths.
In the topsoil, plots under M + AD10G0.5-P-W and (M-P-W)BT treatments had ∼20 and ∼19% higher total SOC within MA, ∼31 and ∼29% higher total SOC within MI, and ∼35 and ∼27% higher total SOC within the SC fraction, respectively, than M-W plots (Table 3). In the 5–15-cm layer, M + AD10G0.5-P-W plots had ∼25, ∼31, and ∼48% higher total SOC concentrations within MA, MI, and SC fractions, respectively, than M-W plots (Table 3).
Presence of crop residues, decomposing root biomass, and hyphae within MA might be the reason for the higher SOC concentration in MA than in MI (Bhattacharyya et al., 2013). Due to less soil disturbance (conservation tillage), a long lasting turn-over rate was observed within MA, especially in the 5–15-cm soil layer. Thus, SOC concentrations were higher in MA for this obvious reason. Conservation-till farming enhanced soil aggregation by reducing soil disturbance, increasing SOM content, and possibly increasing the growth of fungi that bind to soil particles and MI (Helgason et al., 2010; Sithole et al., 2019).
The labile C-pool concentration was the highest in M + AD10G0.5-P-W plots (6.42 g·kg−1) and least in M-W plots (2.86 g·kg−1) in the topsoil. In that layer, M-W and M + AD5G1-W (non-legume treatments) plots had ∼55% and ∼36% lower labile C concentrations, respectively, than M + AD10G0.5-P-W in bulk soils (Table 2). In the 5–15-cm layer, plots under M + AD10G0.5-P-W and (M-P-W)BT treatments had ∼47% and 40% higher labile C, respectively, than M-W plots in bulk soils (Table 3). The M-W and M-P-W plots had ∼33% and ∼28% lower macroaggregate-associated labile C pools, respectively (Tables 2, 3), than M + AD10G0.5-P-W plots in the topsoil. In the 5–15-cm layer, M + AD10G0.5-P-W and (M-P-W)BT plots had ∼54% and ∼57% higher labile C values within MA, respectively (Tables 2, 3), than the control plots.
A higher concentration of glomalin in maize–vegetable pea–wheat crop rotation may have contributed to higher levels of very-labile C in soil layers. Increased microbial activity in soil may accelerate the conversion of organic matter in plant litter to labile forms of organic carbon (Poirier et al., 2013; Whalen et al., 2014). Emplacement of agro-geotextiles, legume incorporation, and residue management significantly increased labile C within MA compared to the control plots. Short-term residue management and legume-based crop rotation increased C inputs that improved the very labile and labile C concentrations in the soil surface (Bhattacharyya et al., 2009a).
In the 5–15-cm soil layer, M-W plots had ∼24% and ∼20% lower recalcitrant C pools than plots under M + AD10G0.5-P-W and (M-P-W)BT, respectively (Table 3). In the topsoil, M-W plots had ∼11% higher recalcitrant C within MA than M + AD10G0.5-P-W. The M + AD10G0.5-P-W plots had ∼13% and ∼30% higher recalcitrant C pools within MA and MI, respectively, than M-W plots in the 5–15-cm soil layer (Table 3). In the topsoil and 5–15-cm soil layers, M + AD10G0.5-P-W plots had ∼44% and ∼52% higher KMnO4–C, respectively, than control plots. In the topsoil, incorporation of a legume crop (vegetable pea) in the M-W system increased KMnO4–C by ∼17% compared to plots without the legume crop (Table 2). Distribution of recalcitrant C with respect to the total C content within macroaggregates was higher due to the fact that C accumulation was associated with macroaggregate formation, which forms a physical barrier between the microbes and substrates and, in turn, physically protect SOC from microbial decomposition (Zhou et al., 2009; Tripathi et al., 2014).
The M-W plot was considered the reference soil for the calculation of CMI. The lability index (LI) of C was not significantly affected by any treatments in the topsoil and 5–15-cm soil depths (Table 5). The carbon pool index (CPI) values in both depths were significantly affected due to the application of A. donax mats and vegetable pea incorporation in the M-W system (Table 5). In the topsoil, M + AD10G0.5-P-W plots had ∼60% more CMI than the control treatment, and A. donax mat-treated plots had ∼9% higher CMI than those without A. donax mat-emplaced plots. In the 5–15-cm layer, soil conservation practices had no impact on CMI values (Table 5).
TABLE 5. Lability index (LI), C pool index (CPI), and C management index (CMI) in bulk soils, as affected by 5 years of different soil conservation practices at the 0–5-cm and 5–15-cm depths in the foothills of the Indian Himalayas.
The sensitive estimate of the rate of changes in the system’s soil C dynamics relative to the more stable reference soil was provided by CMI. With a focus on labile carbon changes rather than non-labile C in SOM, the CMI investigates changes in the total and labile carbon as a function of agricultural practices. As a result, CMI can be used to measure the management systems’ ability to increase soil quality by incorporating both the SOC pool and C lability. Treatments with the application of A. donax mats showed significantly higher CMI than conventional treatments (Table 5). Compared to ZT alone, ZT treatments with cover-crop mixtures showed significantly higher values of CMI (Islam and Reeder, 2014). It was observed that the lability of C was higher in the 0–5-cm soil, and lability of plots emplaced with A. donax mats and residue-added plots was higher, so, CPI was higher. This might be the probable reason of a higher CMI in topsoil (0–5 cm) as well as agro-geotextile and residue-retained plots. The labile carbon pool was higher (Table 2) in the topsoil due to the addition of A. donax mats, causing contact of the mats with organic matter addition.
In surface soil, microbial activity was higher due to residue retention, resulting in the translocation of soluble organic compounds. Higher proportion of MA in plots with A. donax mat application and residue management led to a higher amount of the labile C pool in surface layers. Rhizodeposits that improved the labile C concentrations in the soil surface were boosted by short-term residue management and legume-based crop rotation (Bhattacharyya et al., 2009a). Because more residue biomass was added under A. donax mat-emplaced plots than in those without mat-emplaced plots, total SOC, and labile carbon and recalcitrant carbon fractions were considerably higher (p < 0.05). The most likely causes were increased rhizodeposition, microbial biomass, and changes in crop residue quality, all of which altered the lability of SOC by KMnO4 oxidation (Rudrappa et al., 2006; Purakayastha et al., 2008).
It was observed that the maximum amount of crop residue carbon input was by maize, followed by wheat and vegetable pea. The root portion of the crops had the highest C input to soil. In the case of wheat, C input by root and shoot ranged from 46% to 48% and 44% to 47%, respectively. C input by vegetable pea root and shoot ranged from 35% to 38% and 33% to 36%, respectively. In the case of maize, C input in soil ranged from 37% to 40% and 36% to 39% by root and shoot, respectively (Table 6). A. donax contributed 0.24–0.90 Mg·C·ha−1 yr-1 to the soil over different treatments. Total C inputs of eight different treatments ranged from 2.63 to 4.23 Mg·ha−1 yr−1 (Table 6). Plots under M + AD10G0.5-P-W treatment had the highest C input over other treatments, followed by M + AD5G0.5-P-W and M + AD10G1-P-W plots.
TABLE 6. Estimated total organic carbon inputs (Mg ha-1 yr-1) to soil under various soil conservation practices.
Agro-geotextiles with legume integration and soil conservation practices under an M-W cropping system significantly increased the total SOC stock and carbon sequestration rate in the 0–30-cm soil layer. In that layer, the SOC stock ranged from 22.74 Mg ha−1 to 27.87 Mg ha−1. In the 0–30-cm depth, M + AD10G0.5-P-W and (M-P-W)BT plots had ∼23 and 20% higher SOC stocks, respectively, than M-W plots (Table 7). In the deep soil layer (30–60 cm), soil conservation practices had no impact on SOC stocks (Table 7). In the M + AD10G0.5-P-W plots, the C accumulation rate was 1.03 Mg·ha−1 yr−1.
TABLE 7. Total SOC stock (Mg ha−1) on an equivalent mass basis and C accumulation rate (Mg ha−1 yr-1) in bulk soils, as affected by 5 years of different conservation practices in the foothills of the Indian Himalayas.
There were significantly higher total SOC stocks in treatments emplaced with A. donax mats (M + AD5G1-W, M + AD5G1-P-W, M + AD10G1-P-W, M + AD5G0.5-P-W, and M + AD10G0.5-P-W) than those in M-W and M-P-W plots in the topsoil and 5–15-cm soil depths (Tables 2, 3). This was due to the use of A. donax mats, which were mixed into the field after maize harvest, and higher rates of the addition of crop roots, crop residues (due to higher crop productivities), and weed biomass in the soil conservation plots than those in the control plots, resulting in improved soil aggregation (Bhattacharyya et al., 2011). In the case of bench terrace treatment [(M-P-W)BT], soil loss was reduced due to improved soil water conservation. Vegetable pea incorporation also contributed to a higher SOC concentration than a non-legume cropping system (M-W and M + AD5G1-W). The C accumulation rate in the 0–30-cm soil layer in the M-W-P plots (over the M-W plots) was only 0.1 Mg·ha−1·yr−1, implying the insignificant role of legume addition on C accumulation in the M-W system. However, this C accumulation, along with improved soil aggregation, resulted in decreased soil loss due to cover crop addition in the M-W system. A. donax mats contributed to higher C stock by reducing soil loss due to splash erosion (Bhattacharyya et al., 2010a). A. donax biomass C was higher in the 0.5-m vertical interval than the 1-m vertical interval plots due to more number of mat strips, which resulted in higher biomass addition. Retention of crop residues of maize, wheat and vegetable pea, and A. donax mat emplacement and weed biomass C addition increased the C input value in the study area (Table 6). The slow breakdown of the residue on the surface in zero-tillage (ZT) plots causes a slower rate of incorporation, resulting in increased SOC sequestration in surface soil (Jat et al., 2019).
From 2017 to 2021, the mean annual soil loss from the study area ranged between 0.78 t·ha−1 yr−1 and 10.39 t·ha−1 yr−1 in different treatments. The highest average soil loss occurred in the control treatment plot (M-W), i.e., 10.39 t·ha−1·yr−1, and the least soil loss was observed in the M + AD10G0.5-P-W plot (0.78 t ha−1 yr−1). The M + AD10G0.5-P-W plots had ∼92% reduction in the mean annual soil loss over M-W plots. A. donax mat emplacement had ∼65% mean annual soil loss reduction compared to plots without mats (Figure 5). Total SOC concentrations, proportion of MA, labile C concentrations, macroaggregate-associated C concentrations, CMI values, and thus, C accumulation rates were strongly correlated with soil loss in the topsoil and in the 5–15-cm soil layer (Table 8). In topsoil, the SOC accumulation rate had a significantly high correlation with soil loss rates (R2 = 0.8432, p < 0.05; n = 7).
TABLE 8. Results of regression analysis among different soil parameters with the mean annual soil loss (n = 8).
Thus, it was evident that A. donax mat emplacement and residue input can improve soil aggregation, aggregate-associated C, and, hence, enhance the total SOC and C accumulation rate, which controls soil loss. The correlation analysis showed that the mean estimated annual C input was significantly (p < 0.05) related to the mean annual soil loss (Table 8). This implies soil aggregation and C accumulation in surface soil as the major driver for reducing soil loss. The cover of AGT mats and legumes (as a cover crop) might be the reasons for a better soil structure and increased infiltration rate, which resulted in reduced runoff and soil loss. From the significant (p < 0.01; n = 7) relationship between mean SOC accumulation in the 0–30-cm layer and mean annual soil loss, it was observed that for an attainable SOC accumulation rate of 0.5 Mg·ha−1·yr−1 by agricultural management practices, the soil loss rate could be reduced from 10.39 to 3.75 Mg·ha−1 yr−1 in the study site (Figure 6).
FIGURE 6. Relationship between the soil organic carbon (SOC) accumulation rate in the 0–30-cm layer and the mean annual soil loss under 5 years of soil conservation practices in the Indian Himalayas.
A. donax mat emplacement had ∼65% mean annual soil loss reduction compared to plots without mats. The plots under M + AD10G0.5-P-W treatment had only a mean annual soil loss of 0.78 t·ha−1 yr−1 , whereas that of the M-W plot was 10.39 t·ha−1 yr−1. The highest carbon accumulation rate in the 0–30-cm depth was under the M + AD10G0.5-P-W treatment (1.03 Mg·ha−1 yr−1), which accumulated 1.20 Mg·ha−1 yr−1 more C than non-agro-geotextile-treated plots (farmers’ practice). For an attainable SOC accumulation rate of 0.5 Mg·ha−1·yr−1 by appropriate agricultural management practice in this region, the soil loss rate could be reduced by ∼64% (from 10.39 to 3.75 Mg·ha−1 yr−1). Thus, the use of A. donax mats as agro-geotextiles in soil has a significant effect on soil loss control and SOC sequestration and, hence, could be adopted in the foothills of the Indian Himalayas and similar agro-ecologies. Limitations to the study are as follows:
1. Preparation of agro-geotextiles on a large scale may be difficult due to labor scarcity.
2. Farmers’ perception is not to leave an inch of land for other purposes, such as emplacement of mats, and to use 100% land for cultivation.
The original contributions presented in the study are included in the article/Supplementary Material; further inquiries can be directed to the corresponding author.
PR: Writing–original draft, Writing–review and editing. RB: Writing–original draft, Writing–review and editing. RS: Writing–review and editing. NS: Writing–review and editing. GK: Writing–review and editing. MM: Writing–review and editing. DB: Writing–review and editing. AG: Writing–review and editing. SD: Writing–review and editing. AJ: Writing–review and editing. TD: Writing–review and editing. SK: Writing–review and editing. SJ: Writing–review and editing. YSS: Writing–review and editing. PJ: Writing–review and editing.
The authors declare financial support was received for the research, authorship, and/or publication of this article. The work was supported by the Department of Science and Technology, Government of India, through the National Mission for Sustaining Himalayan Ecosystem Taskforce on Himalayan Agriculture being implemented by the ICAR.
The authors acknowledge the Indian Council of Agricultural Research (ICAR) for providing facilities to carry out this work. The first author acknowledges the UGC-NFSC fellowship during her Ph.D.
The authors declare that the research was conducted in the absence of any commercial or financial relationships that could be construed as a potential conflict of interest.
All claims expressed in this article are solely those of the authors and do not necessarily represent those of their affiliated organizations, or those of the publisher, the editors, and the reviewers. Any product that may be evaluated in this article, or claim that may be made by its manufacturer, is not guaranteed or endorsed by the publisher.
Bhattacharyya, R., Das, T. K., Pramanik, P., Ganeshan, V., Saad, A. A., and Sharma, A. R. (2013). Impacts of conservation agriculture on soil aggregation and aggregate-associated N under an irrigated agroecosystem of the Indo-Gangetic Plains. Nutr. Cycl. Agroecosyst. 96, 185–202. doi:10.1007/s10705-013-9585-6
Bhattacharyya, R., Das, T. K., Sudhishri, S., Dudwal, B., Sharma, A. R., Bhatia, A., et al. (2015b). Conservation agriculture effects on soil organic carbon accumulation and crop productivity under a rice-–wheat cropping system in the western Indo-Gangetic Plains. Eur. J. Agron. 70, 11–21. doi:10.1016/j.eja.2015.06.006
Bhattacharyya, R., Fullen, M. A., Davies, K., and Booth, C. A. (2009c). Utilizing palm-leaf geotextile mats to conserve loamy sand soil in the United Kingdom. Agric. Ecosyst. Environ. 130, 50–58. doi:10.1016/j.agee.2008.11.015
Bhattacharyya, R., Fullen, M. A., Davies, K., and Booth, C. A. (2010a). Use of palm-mat geotextiles for rainsplash erosion control. Geomorph 119, 52–61. doi:10.1016/j.geomorph.2010.02.018
Bhattacharyya, R., Ghosh, B. N., Mishra, P. K., Mandal, B., Rao, C. S., Sarkar, D., et al. (2015a). Soil degradation in India: challenges and potential solutions. Sustainability 7, 3528–3570. doi:10.3390/su7043528
Bhattacharyya, R., Kundu, S., Srivastva, A. K., Gupta, H. S., Prakash, V., and Bhatt, J. C. (2011). Long term fertilization effects on soil organic carbon pools in a sandy loam soil of the Indian sub-Himalayas. Plant Soil 341, 109–124. doi:10.1007/s11104-010-0627-4
Bhattacharyya, R., Prakash, V., Kundu, S., Srivastva, A. K., and Gupta, H. S. (2009a). Soil properties and their relationships with crop productivity after 30 years of different fertilization in the Indian Himalayas. Arch. Agron. Soil Sci. 55, 641–661. doi:10.1080/03650340902718615
Bhattacharyya, R., Prakash, V., Kundu, S., Srivastva, A. K., and Gupta, H. S. (2009b). Soil aggregation and organic matter in a sandy clay loam soil of the Indian Himalayas under different tillage and crop regimes. Agric. Ecosyst. Environ. 132, 126–134. doi:10.1016/j.agee.2009.03.007
Bhattacharyya, R., Prakash, V., Kundu, S., Srivastva, A. K., Gupta, H. S., and Mitra, S. (2010b). Long term effects of fertilization on carbon and nitrogen sequestration and aggregate associated carbon and nitrogen in the Indian sub-Himalayas. Nutr. Cycl. Agroecosyst. 86, 1–16. doi:10.1007/s10705-009-9270-y
Bhattacharyya, R., Tuti, M. D., Bisht, J. K., Bhatt, J. C., and Gupta, H. S. (2012). Conservation tillage and fertilization impact on soil aggregation and carbon pools in the Indian Himalayas under an irrigated rice-wheat rotation. Soil Sci. 177, 218–228. doi:10.1097/ss.0b013e3182408f1e
Blair, G. J., Lefroy, R. D., and Lisle, L. (1995). Soil carbon fractions based on their degree of oxidation, and the development of a carbon management index for agricultural systems. Aust. J. Agric. Res. 46, 1459–1466. doi:10.1071/ar9951459
Bongiorno, G., Bunemann, E. K., Oguejiofor, C. U., Meier, J., Gort, G., Comans, R., et al. (2019). Sensitivity of labile carbon fractions to tillage and organic matter management and their potential as comprehensive soil quality indicators across pedoclimatic conditions in Europe. Ecol. Indic. 99, 38–50. doi:10.1016/j.ecolind.2018.12.008
Chan, K. Y., Bowman, A., and Oates, A. (2001). Oxidizible organic carbon fractions and soil quality changes in an oxic paleustalf under different pasture leys. Soil Sci. 166, 61–67. doi:10.1097/00010694-200101000-00009
Ellert, B. H., and Bettany, J. R. (1995). Calculation of organic matter and nutrients stored in soils under contrasting management regimes. Can. J. Soil Sci. 75, 529–538. doi:10.4141/cjss95-075
Elliott, E. T. (1986). Aggregate structure and carbon, nitrogen, and phosphorus in native and cultivated soils. Soil Sci. Soc. Am. J. 50, 627–633. doi:10.2136/sssaj1986.03615995005000030017x
Fagnano, M., Impagliazzo, A., Mori, M., and Fiorentino, N. (2015). Agronomic and environmental impacts of giant reed (Arundo donax L.): results from a long-term field experiment in hilly areas subject to soil erosion. Bioenergy Res. 8, 415–422. doi:10.1007/s12155-014-9532-7
Ghosh, B. N., Meena, V. S., Alam, N. M., Dogra, P., Bhattacharyya, R., Sharma, N. K., et al. (2016). Impact of conservation practices on soil aggregation and the carbon management index after seven years of maize-wheat cropping system in the Indian Himalayas. Agric. Ecosyst. Environ. 216, 247–257. doi:10.1016/j.agee.2015.09.038
Ghosh, P. K., Venkatesh, M. S., Hazra, K. K., and Kumar, N. (2012). Long-term effect of pulses and nutrient management on soil organic carbon dynamics and sustainability on an inceptisol of indo-gangetic plains of India. Exp. Agric. 48, 473–487. doi:10.1017/s0014479712000130
Gogoi, B., Borah, N., Baishya, A., Dutta, S., Nath, D. J., Das, R., et al. (2021). Yield trends, soil carbon fractions and sequestration in a rice-rice system of North-East India: effect of 32 years of INM practices. Field Crops Res. 272, 108289. doi:10.1016/j.fcr.2021.108289
Gomez, K. A., and Gomez, A. A. (1984). Statistical procedures for agricultural research. New Jersey, United States: John Wiley and Sons.
Helgason, B. L., Walley, F. L., and Germida, J. J. (2010). No-till soil management increases microbial biomass and alters community profiles in soil aggregates. Appl. Soil Ecol. 46, 390–397. doi:10.1016/j.apsoil.2010.10.002
Islam, R., and Reeder, R. (2014). No-till and conservation agriculture in the United States: an example from the David Brandt farm, Carroll, Ohio. Int. Soil Water Conserv. Res. 2, 97–107. doi:10.1016/s2095-6339(15)30017-4
Jat, S. L., Parihar, C. M., Singh, A. K., Kumar, B., Choudhary, M., Nayak, H. S., et al. (2019). Energy auditing and carbon footprint under long-term conservation agriculture-based intensive maize systems with diverse inorganic nitrogen management options. Sci. Total Environ. 664, 659–668. doi:10.1016/j.scitotenv.2019.01.425
Joseph, A., Bhattacharyya, R., Biswas, D. R., Das, T. K., Bandyopadhyay, K. K., Dey, A., et al. (2023). Long-term adoption of bed planted conservation agriculture based maize/cotton-wheat system enhances soil organic carbon stabilization within aggregates in the Indo-Gangetic Plains. Front. Environ. Sci. 11, 1216242. doi:10.3389/fenvs.2023.1216242
Kundu, S., Bhattacharyya, R., Prakash, V., Ghosh, B. N., and Gupta, H. S. (2007). Carbon sequestration and relationship between carbon addition and storage under rainfed soybean–wheat rotation in a sandy loam soil of the Indian Himalayas. Soil tillage Res. 92, 87–95. doi:10.1016/j.still.2006.01.009
Maini, A., Sharma, V., and Sharma, S. (2020). Assessment of soil carbon and biochemical indicators of soil quality under rainfed land use systems in North Eastern region of Punjab, India. Carbon Manage 11, 169–182. doi:10.1080/17583004.2020.1721976
Mandal, D., Chandrakala, M., Alam, N. M., Roy, T., and Mandal, U. (2021). Assessment of soil quality and productivity in different phases of soil erosion with the focus on land degradation neutrality in tropical humid region of India. Catena 204, 105440. doi:10.1016/j.catena.2021.105440
Mandal, D., Giri, N., and Srivastava, P. (2020). The magnitude of erosion-induced carbon (C) flux and C-sequestration potential of eroded lands in India. Eur. J. Soil Sci. 71, 151–168. doi:10.1111/ejss.12886
Mandal, D., and Sharda, V. N. (2011). Assessment of permissible soil loss in India employing a quantitative bio-physical model. Curr. Sci., 383–390.
Mandal, N., Dwivedi, B. S., Meena, M. C., Singh, D., Datta, S. P., Tomar, R. K., et al. (2013). Effect of induced defoliation in pigeonpea, farmyard manure and sulphitation pressmud on soil organic carbon fractions, mineral nitrogen and crop yields in a pigeonpea–wheat cropping system. Field Crops Res. 154, 178–187. doi:10.1016/j.fcr.2013.08.007
Meena, V. S., Mondal, T., Pandey, B. M., Mukherjee, A., Yadav, R. P., Choudhary, M., et al. (2018). Land use changes: strategies to improve soil carbon and nitrogen storage pattern in the mid-Himalaya ecosystem, India. Geoderma 321, 69–78. doi:10.1016/j.geoderma.2018.02.002
Poirier, V., Angers, D. A., Rochette, P., and Whalen, J. K. (2013). Initial soil organic carbon concentration influences the short-term retention of crop-residue carbon in the fine fraction of a heavy clay soil. Biol. Fertil. Soils 49, 527–535. doi:10.1007/s00374-013-0794-6
Purakayastha, T. J., Rudrappa, L., Singh, D., Swarup, A., and Bhadraray, S. (2008). Long-term impact of fertilizers on soil organic carbon pools and sequestration rates in maize–wheat– cowpea cropping system. Geoderma 144, 370–378. doi:10.1016/j.geoderma.2007.12.006
Rudrappa, L., Purakayastha, T. J., Singh, D., and Bhadraray, S. (2006). Long-term manuring and fertilization effects on soil organic carbon pools in a Typic Haplustept of semi-arid subtropical India. Soil tillage Res. 88, 180–192. doi:10.1016/j.still.2005.05.008
Sharda, V. N., and Ojasvi, P. R. (2016). A revised soil erosion budget for India: role of reservoir sedimentation and land-use protection measures. Earth Surf. Process Landf. 41, 2007–2023. doi:10.1002/esp.3965
Sharma, N. K., Singh, R. J., Mandal, D., Kumar, A., Alam, N. M., and Keesstra, S. (2017). Increasing farmer’s income and reducing soil erosion using intercropping in rainfed maize-wheat rotation of Himalaya, India. Agric. Ecosyst. Environ. 247, 43–53. doi:10.1016/j.agee.2017.06.026
Singh, M., Sarkar, B., Sarkar, S., Churchman, J., Bolan, N., Mandal, S., et al. (2018). Stabilization of soil organic carbon as influenced by clay mineralogy. Adv. Agron. 148, 33–84.
Singh, R. J., Deshwal, J. S., Sharma, N. K., Ghosh, B. N., and Bhattacharyya, R. (2019). Effects of conservation tillage based agro-geo-textiles on resource conservation in sloping croplands of Indian Himalayan Region. Soil Tillage Res. 191, 37–47. doi:10.1016/j.still.2019.03.012
Sithole, N. J., Magwaza, L. S., and Thibaud, G. R. (2019). Long-term impact of no-till conservation agriculture and N-fertilizer on soil aggregate stability, infiltration and distribution of C in different size fractions. Soil Tillage Res. 190, 147–156. doi:10.1016/j.still.2019.03.004
Solomon, H., James, L., and Woldeamilak, B. (2017). Soil and water conservation effect on soil properties in the Middle Silluh Valley, northern Ethiopia. Int. Soil Water Conserv. Res. 5, 231–240.
Somasundaram, J., Sinha, N. K., Dalal, R. C., Lal, R., Mohanty, M., Naorem, A. K., et al. (2020). No-till farming and conservation agriculture in South Asia–issues, challenges, prospects and benefits. Crit. Rev. Plant Sci. 39, 236–279. doi:10.1080/07352689.2020.1782069
Swarnkar, S., Malini, A., Tripathi, S., and Sinha, R. (2018). Assessment of uncertainties in soil erosion and sediment yield estimates at ungauged basins: an application to the Garra River basin, India. Hydrol. Earth Syst. Sci. 22, 2471–2485. doi:10.5194/hess-22-2471-2018
Tirol-Padre, A., and Ladha, J. K. (2004). Assessing the reliability of permanganate-oxidizable carbon as an index of soil labile carbon. Soil. Sci. Soc. Am. J. 68, 969–978. doi:10.2136/sssaj2004.9690
Tripathi, R., Nayak, A. K., Bhattacharyya, P., Shukla, A. K., Shahid, M., Raja, R., et al. (2014). Soil aggregation and distribution of carbon and nitrogen in different fractions after 41 years long-term fertilizer experiment in tropical rice–rice system. Geoderma 213, 280–286. doi:10.1016/j.geoderma.2013.08.031
USDA (1996). “Natural resources conservation services, nation soil survey center,” in Soil survey laboratory manual. Soil survey investigation report no. 1 (Washington, D.C: US Dept. Agric).
Visconti, D., Fiorentino, N., Cozzolino, E., Di Mola, I., Ottaiano, L., Mori, M., et al. (2020). Use of giant reed (Arundo donax L) to control soil erosion and improve soil quality in a marginal degraded area. Ital. J. Agron. 15, 332–338. doi:10.4081/ija.2020.1764
Wendt, J. W., and Hauser, S. (2013). An equivalent soil mass procedure for monitoring soil organic carbon in multiple soil layers. Eur. J. Soil Sci. 64, 58–65. doi:10.1111/ejss.12002
Whalen, J. K., Gul, S., Poirier, V., Yanni, S. F., Simpson, M. J., Clemente, J. S., et al. (2014). Transforming plant carbon into soil carbon: process-level controls on carbon sequestration. Can. J. Plant Sci. 94, 1065–1073. doi:10.4141/cjps2013-145
Williams, J. D., McCool, D. K., Reardon, C. L., Douglas, C. L., Albrecht, S. L., and Rickman, R. W. (2013). Root: shoot ratios and belowground biomass distribution for Pacific Northwest dryland crops. J. Soil Water Conserv. 68, 349–360. doi:10.2489/jswc.68.5.349
Worku, H. (2017). Impact of physical soil and water conservation structure on selected soil physicochemical properties in Gondar Zuriya Woreda. Resour. Environ. Sustain. 7, 40–48.
Zhao, G., Zhou, B., Mu, Y., Wang, Y., Liu, Y., and Wang, L. (2021). Irrigation with activated water promotes root growth and improves water use of winter wheat. Agronomy 11, 2459. doi:10.3390/agronomy11122459
Zhou, Z., Lai, C., Zhang, L., Qian, Y., Hou, H., Reneker, D. H., et al. (2009). Development of carbon nanofibers from aligned electrospun polyacrylonitrile nanofiber bundles and characterization of their microstructural, electrical, and mechanical properties. Polym 50, 2999–3006. doi:10.1016/j.polymer.2009.04.058
Keywords: soil conservation, agro-geotextiles, Arundo donax, carbon management index, carbon accumulation rate, soil aggregation
Citation: Roy P, Bhattacharyya R, Singh RJ, Sharma NK, Kumar G, Madhu M, Biswas DR, Ghosh A, Das S, Joseph AM, Das TK, Kumar SN, Jat SL, Saharawat YS and Jha P (2023) Impact of agro-geotextiles on soil aggregation and organic carbon sequestration under a conservation-tilled maize-based cropping system in the Indian Himalayas. Front. Environ. Sci. 11:1309106. doi: 10.3389/fenvs.2023.1309106
Received: 07 October 2023; Accepted: 30 October 2023;
Published: 16 November 2023.
Edited by:
Kaibo Wang, Chinese Academy of Sciences (CAS), ChinaReviewed by:
Ashim Datta, Central Soil Salinity Research Institute (ICAR), IndiaCopyright © 2023 Roy, Bhattacharyya, Singh, Sharma, Kumar, Madhu, Biswas, Ghosh, Das, Joseph, Das, Kumar, Jat, Saharawat and Jha. This is an open-access article distributed under the terms of the Creative Commons Attribution License (CC BY). The use, distribution or reproduction in other forums is permitted, provided the original author(s) and the copyright owner(s) are credited and that the original publication in this journal is cited, in accordance with accepted academic practice. No use, distribution or reproduction is permitted which does not comply with these terms.
*Correspondence: Ranjan Bhattacharyya, cmFuamFudnBrYXNAZ21haWwuY29t; Raman Jeet Singh, cmR4c2luZ2hAZ21haWwuY29t
Disclaimer: All claims expressed in this article are solely those of the authors and do not necessarily represent those of their affiliated organizations, or those of the publisher, the editors and the reviewers. Any product that may be evaluated in this article or claim that may be made by its manufacturer is not guaranteed or endorsed by the publisher.
Research integrity at Frontiers
Learn more about the work of our research integrity team to safeguard the quality of each article we publish.