- 1Biological Sciences Department, Environmental and Conservation Sciences Program, North Dakota State University, Fargo, ND, United States
- 2Department of Ecosystem Science and Management, The Pennsylvania State University, University Park, PA, United States
An increase in herbicide use is occurring due to a growing population and herbicide-resistant crops in agriculture, which has resulted in more herbicide tolerant target species. Glyphosate and 2,4-Dichlorophenoxyacetic acid (2,4-D) are two of the most commonly used herbicides worldwide and are more recently being used in combination in pre-mixed commercial formulas. Subsequently, herbicide contamination of wetlands will increase exposure of microorganisms to multiple chemical stressors. Methane is a potent greenhouse gas naturally emitted from wetlands, but herbicides may disrupt biogeochemical processes leading to an unbalanced methane cycle. We review the impacts of these herbicides on aquatic microbial communities from glyphosate-derived nutrient enrichment and 2,4-D inhibition of methane oxidation, and examine how these altered metabolic processes may lead to increased methane production in wetlands. The response of wetland ecosystems to herbicide contamination will vary across regions, in part due to the complexity of microbial communities, however, this perspective gives a glimpse into the potential global implications of continuing herbicide use on wetlands and demonstrates the importance for research on ecosystem-level co-stressors.
1 Introduction
Climate change is an ongoing global concern as greenhouse gas (GHG) emissions continue to increase (IPCC, 2021). Methane (CH4) is the second most abundant GHG, after carbon dioxide (CO2), but is about 25 times more potent (Islam et al., 2018; EPA, 2023). Wetlands are a large natural source of CH4 as they play a significant role in carbon (C) sequestration and cycling (Andresen et al., 2017), and it has recently been suggested that agrochemicals may impact GHG emissions from freshwater ecosystems (Stehle and Schulz, 2015). In particular, herbicide use has substantially increased over the past 30 years due to the introduction and rapid adoption of herbicide-resistant crops worldwide (Bai and Ogbourne, 2016; Coupe and Capel, 2016; Peterson et al., 2018). As a result, target plants have developed herbicide-resistance and the use of pre-mixed formulas that contain multiple active ingredients (i.e., multiple modes of action) has become more common (Freydier and Lundgren, 2016; Schütte et al., 2017). Herbicide use is also projected to increase due to ongoing climatic change (Delcour et al., 2015), where higher temperatures can enhance toxicity and alter biodegradation processes (Noyes et al., 2009; Koleva and Schneider, 2010; Matzrafi, 2019). Subsequently, wetland biota are subjected to combinations of more severe physical and chemical stressors.
Glyphosate and 2,4-D are two of the most commonly used herbicides globally, and are also used in pre-mixed formulas such as Enlist Duo® (1:0.95 glyphosate:2,4-D) and Landmaster™ II (1:0.83 glyphosate:2,4-D) (Benbrook, 2016; Zuanazzi et al., 2020; EPA, 2022). Their extensive use is cause for environmental concern within aquatic ecosystems as herbicides are already substantial contributors to wetland pollution (Casado et al., 2019). Wetlands are often located in the lowest drainage points of agriculture fields, where they can serve as critical sinks for herbicides transported through spray drift, runoff, groundwater leaching, and wind and sediment erosion (Annett et al., 2014; Bento et al., 2017). However, their fate is dependent on many landscape- and ecosystem-level components such as, precipitation patterns, herbicide application dates, surrounding land use, and plant and animal composition. For example, glyphosate and 2,4-D are highly water soluble, thus a rainfall shortly after application would rapidly transport substantial amounts of these herbicides into nearby wetlands (Bertuzzo et al., 2013). These agrochemicals often persist in sediments of temperate and northern climates (Helander et al., 2012; Mierzejewska and Urbaniak, 2022), can bioaccumulate in organisms such as biofilms (Beecraft and Rooney, 2021), and can be transported between habitats via emerging insects (Roodt et al., 2023). Herbicides are frequently detected within aquatic ecosystems around the world, where they have even been found in protected conservation areas (Wolfram et al., 2023).
Consequently, “pesticide cocktails” can effect microorganisms that are important contributors to wetland biogeochemical cycling and overall ecosystem function (Aparicio et al., 2013; Sun et al., 2013; Islam et al., 2018; Baker et al., 2020). Microorganisms are sensitive to disturbances (Sun et al., 2013), thus as both herbicide use and climate change intensifies it is critical to assess the potential effects of herbicides on GHG emissions. Methanogens (i.e., CH4 producers) and methanotrophs (i.e., CH4 consumers), in addition to plant and algal-mediated transport, play a critical role in the global CH4 budget of wetlands, which may be impacted by glyphosate and 2,4-D. In this article we highlight previous research on glyphosate-derived nutrient enrichment and 2,4-D inhibited CH4 oxidation to suggest that herbicides entering wetlands could alter CH4 production via synergistic effects on microbial communities and consequently impact climate change.
1.1 Glyphosate
Glyphosate’s impacts on wetland microorganisms are often dose- and species-dependent (Bai and Ogbourne, 2016), therefore it can be detrimental or advantageous to different microbial species. While glyphosate’s mode of action was developed to target the shikimate pathway in higher plants (Hetrick and Blankinship, 2015), many archaea and bacteria also utilize this pathway resulting in non-target effects (Herrmann and Weaver, 1999). Despite the potential negative impacts on microorganisms, in many instances increased growth, respiration, and enhanced metabolism in wetland microbial communities have been observed as a result of glyphosate biodegradation (Vera et al., 2012; Lu et al., 2020) and linked with the use of glyphosate as a nutritive source (Saxton et al., 2011; Wang et al., 2016). Due to glyphosate’s chemical structure, its degradation often contributes substantial amounts of phosphorus (P), which has been found to be favored and more rapidly utilized by microorganisms compared to other sources of soil P (Hébert et al., 2019; Sun et al., 2019). Specifically, stimulated cyanobacterial growth and cyanotoxin production has been recorded from glyphosate-derived P enrichment (Vera et al., 2010; Qiu et al., 2013; Zhang et al., 2016; Hernández-García and Martínez-Jerónimo, 2020; Wang et al., 2021; Lin et al., 2023). Glyphosate degradation was found to be positively correlated with total P concentrations in surface waters (Carles et al., 2019). Glyphosate additions to aquatic ecosystems can contribute to water quality issues, such as eutrophication, which has been demonstrated to be an important driver of CH4 emissions (Sepulveda-Jauregui et al., 2018; Beaulieu et al., 2019; Yang et al., 2019; Bertolet et al., 2020). Ultimately, increased glyphosate use could shift microbial community dynamics towards copiotrophs and algae, altering important C biogeochemical processes and resulting in an indirect increase in CH4 production in wetlands (Figures 1A, B).
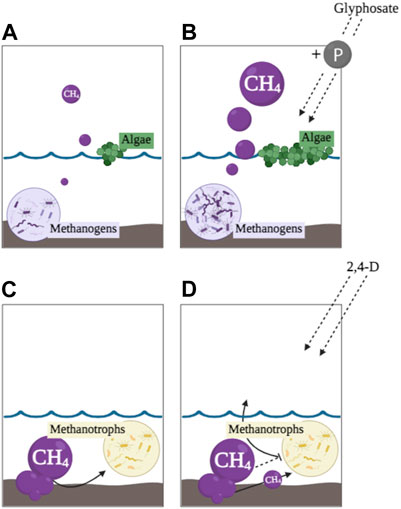
FIGURE 1. Conceptual diagrams of a wetland with methanogens, methanotrophs, and algae. (A) represents balanced CH4 production; (B) represents glyphosate contamination stimulating methanogens and algae causing higher CH4 emissions; (C) represents balanced CH4 oxidation; (D) represents 2,4-D contamination inhibiting oxidation (i.e., removal) of CH4 by methanotrophs causing higher CH4 emissions.
1.2 2,4-D
Despite 2,4-D being the first synthetic herbicide, compared to glyphosate, relatively little research has been conducted on its effects on aquatic microorganisms (Donald et al., 2018; Malaj et al., 2020). However, similar to glyphosate, 2,4-D can have a variety of impacts on wetland microbial communities. It targets broadleaf plants through mimicking the plant growth hormone, indol-3-yl-acetic acid (IAA or auxin), resulting in plant overgrowth (Cobb and Reade, 2010), but auxin synthesis and usage in microorganisms is also well known making them vulnerable non-target organisms (Spaepen and Vanderleyden, 2011). In addition, 2,4-D can be applied as an aquatic herbicide resulting in species being exposed to higher concentrations compared to terrestrial transport (Mierzejewska and Urbaniak, 2022). While 2,4-D is already a widespread environmental contaminant frequently detected in aquatic ecosystems (Malaj et al., 2020) and its use has also increased in recent decades with the development of herbicide-resistant crops, its use will likely continue to increase in the future (Freydier and Lundgren, 2016). Consequently, wetland microorganisms could be highly susceptible to its toxic effects with limited capacity to degrade it. Previous research has found some species use 2,4-D as a C source, whereas other species are toxicologically inhibited (Benndorf et al., 2004; Zabaloy et al., 2008; Sachu et al., 2022). Research in microcosms has also found that increased 2,4-D concentrations resulted in inhibition of CH4 oxidation, decreases in CH4 removal time, and increased CH4 emissions (Syamsul Arif et al., 1996; Kumaraswamy et al., 1997; Top et al., 1999). Where studies from Top et al. (1999) and Seghers et al. (2005) suggested decreases in CH4 removal could be due to 2,4-D inhibition of methanotroph-mediated oxidative metabolism. Research on the effects of 2,4-D on CH4 oxidation is extremely limited, however these studies do indicate that 2,4-D loading into wetlands could potentially alter the CH4 cycle by suppressing the removal of CH4 via the food web, resulting in greater concentrations within the water column and higher emissions (Figures 1C, D).
1.3 Pesticide cocktails: Glyphosate plus 2,4-D
The increased use of pre-mixed glyphosate and 2,4-D herbicides further exposes wetland microorganisms to combinations of chemical stressors, which could lead to unforeseen long-term effects. Research on the combined effects of pesticides has been conducted since the 1970’s, but the majority of the focus has been on the direct toxicological impacts to aquatic flora and fauna (Lichtenstein et al., 1973; Faust et al., 1994; Gardner and Grue, 1996; Hayes et al., 2006; Relyea, 2009; Moreira et al., 2020). These studies included compounds such as atrazine, chlorpyrifos, fipronil, etc., whereas research on the combined effects of glyphosate and 2,4-D is limited, especially at the aquatic microbial level. Additive and/or synergistic effects of glyphosate and 2,4-D have been found on fish and amphibian growth, fertilization, survival, and behavior (Carvalho et al., 2020; Pavan et al., 2021; Bernardi et al., 2022; Peluso et al., 2022), and zooplankton emergence (Portinho et al., 2018). Lozano et al. (2018) found additive impacts of glyphosate and 2,4-D on phytoplankton composition, abundance, and chlorophyll a after 7 days in microcosms, but also found an antagonist effect on total and live abundance of Staurastrum spp. In outdoor mesocosms Lozano et al. (2018) found a decrease in phytoplankton respiration and gross primary production from a high glyphosate (applied as Roundup MaxⓇ), low 2,4-D (applied as AsiMax 50Ⓡ) treatment after 4 h. Additionally, after 7 days in mesocosms with high glyphosate, an increase in primary production, chlorophyll a, and micro- and nanophytoplankton was observed (Lozano et al., 2020). Sura et al. (2015) researched the effects of a herbicide mixture including glyphosate, 2,4-D, MCPA, clopyralid, dicamba, dichlorprop, mecoprop, and bromoxynil on pelagic and benthic communities in nutrient-sufficient and nutrient-deficient wetlands. They found pelagic bacterial productivity significantly increased after treatment in the nutrient-sufficient wetland, but benthic bacterial productivity did not change, which suggests the stimulatory effect of these herbicides may be related to nutrient bioavailability. These results demonstrate the complexity of the direct effects of herbicide mixtures on aquatic microorganisms, but the potential indirect effects are still poorly understood. As pre-mixed glyphosate and 2,4-D herbicides become more common it is important to consider the extent of their effects on aquatic ecosystems. Glyphosate can easily be used as a nutrient source stimulating microbial activity, specifically algal communities, whereas 2,4-D may inhibit methanotrophic communities from oxidizing CH4. As these compounds enter aquatic ecosystems their impacts on microorganisms may become synergistic and/or additive resulting in eutrophication and inhibition of methanotrophs from glyphosate and 2,4-D, respectively. Subsequently, eutrophic conditions and decreased CH4 removal could cause increased CH4 production via an unbalanced CH4 cycle (Figure 2).
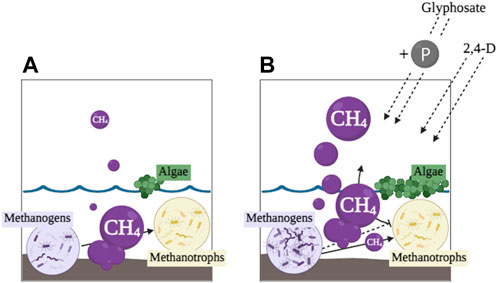
FIGURE 2. Conceptual diagrams of a wetland with methanogens, methanotrophs, and algae. (A) represents a balanced CH4 cycle, where algae and methanogens produce CH4, methanotrophs oxidize CH4; (B) represents an unbalanced CH4 cycle where glyphosate and 2,4-D contamination stimulate methanogens and algae and inhibit CH4 oxidation, respectively, and thus result in higher CH4 emissions.
2 Pollution-Induced Community Tolerance (PICT)
Aquatic ecosystems are subjected to year-round herbicide contamination, where herbicide use differs across crop, season, habitat, and region. Microorganism structure and function can be impacted by herbicides, but toxicity is often dependent on the mode of action, concentration, and duration of exposure, as well as microbial species and environmental factors (DeLorenzo et al., 2001). For example, glyphosate stimulated Chlorella vulgaris growth 24-h after exposure, but then inhibited growth after 48-h at the same concentrations (i.e., hormesis) (Reno et al., 2014). In addition to the duration of exposure, the exposure to a different mode of action could also impact microorganisms by causing a community shift often appearing as changes in gene expression or diversity (Feld et al., 2015). Pollution-Induced Community Tolerance (PICT) refers to the response of a community to a pollutant, which results in an increased tolerance to that pollutant (Blanck, 2002). The use of PICT analysis is extensive in the toxicology literature, especially on phototrophic microorganisms, which are often more susceptible to herbicidal effects due to their similarities with target species (DeLorenzo et al., 2001; Larras et al., 2016). Bérard and Benninghoff (2001) found phytoplankton were significantly more sensitive to atrazine after 1 day, but then significantly less sensitive after at least 11 days. Phototrophic biofilms were found to be increasingly more sensitive to diuron as contamination levels decreased 1–3 years after its ban in the European Union (Pesce et al., 2016). It has also been shown that selection pressure from multiple stressors can lead to more opportunistic species and higher tolerances (Rotter et al., 2013). Ultimately, PICT results suggest that more sensitive species are being replaced by less sensitive species creating a more tolerant community (Blanck, 2002). This has also been seen with both glyphosate and 2,4-D. Microbial communities from sediments with high glyphosate exposure were able to degrade glyphosate faster and had higher diversity compared to sediments with low to no previous exposure (Tang et al., 2019). Zabaloy et al. (2008) saw an increase in a 2,4-D degrading population in soils for approximately 1 month after treatment and found that agricultural soils had higher 2,4-D tolerance compared to reference soils via PICT analysis. In a study by de Lipthay et al. (2002) 2,4-D treatment induced transcription of the gene responsible for 2,4-D degradation (tfdA) which demonstrates a survival response from the microbial community. These studies demonstrate PICT can occur when communities are exposed to a herbicide, therefore contamination by an additional herbicide could further alter communities that have not been exposed before. It could be presumed that wetland microbial communities within a glyphosate-dominant region may substantially change when 2,4-D is introduced in combination with glyphosate and species are replaced. This potential shift would impact the biogeochemical functions of the community, subsequently altering herbicide degradation or metabolism.
3 Conclusion
Glyphosate and 2,4-D are frequently cited as having minimal to no environmental impacts (Peterson et al., 2016; Duke, 2020; Singh et al., 2020), however there is increasing evidence that their indirect effects may be of more substantial global concern. Wetlands naturally emit CH4 via diffusion, ebullition (i.e., bubbles), and plant-mediated transport, and are the highest natural sources of CH4 in the environment (Aben et al., 2017; Andresen et al., 2017), but emissions may be increasing due to agrochemical use adversely impacting CH4 sink potential (Seghers et al., 2005). Glyphosate could stimulate microbial processes resulting in increased CH4 production, in addition to 2,4-D inhibiting CH4 oxidation further resulting in increased CH4 production. Ultimately, this would lead to higher CH4 production versus removal from freshwater creating elevated CH4 in the atmosphere. Due to the widespread and extensive use of glyphosate and 2,4-D, these herbicides are frequently found in wetlands (Islam et al., 2018; Malaj et al., 2020). To our knowledge there has been no research investigating the combined impacts of glyphosate and 2,4-D on wetland microbial communities. The potential bottom-up effects of glyphosate and 2,4-D could be detrimental to a changing climate, thus improving our understanding of how these herbicides can impact GHG emissions is crucial.
3.1 Future research
To investigate the effects of glyphosate and 2,4-D on CH4 emissions from freshwater ecosystems, micro- or mesocosm experiments could be conducted. Experiments under controlled conditions could help determine how wetland microbial communities are affected by glyphosate and 2,4-D. Specifically, this research would give insight into the CH4-related mechanisms that may be enhanced or disrupted in microorganisms. In addition to in-lab research, pesticide loading data could be incorporated into GHG models. These data are currently not included in estimations of CH4 emissions from wetlands, but could be an important source of variation, and could be useful for future climate modeling. These potential impacts are crucial to research as herbicide use is only expected to increase over time, where chemical selection pressure on microbial communities could contribute to climate change.
Data availability statement
The original contributions presented in the study are included in the article/Supplementary Material, further inquiries can be directed to the corresponding author.
Author contributions
CC: Writing–original draft. JS: Writing–review and editing.
Funding
The authors declare that no financial support was received for the research, authorship, and/or publication of this article.
Acknowledgments
Thank you to Dr. Ted Harris for reviewing this manuscript, and for his ongoing support as a committee member to CC.
Conflict of interest
The authors declare that the research was conducted in the absence of any commercial or financial relationships that could be construed as a potential conflict of interest.
Publisher’s note
All claims expressed in this article are solely those of the authors and do not necessarily represent those of their affiliated organizations, or those of the publisher, the editors and the reviewers. Any product that may be evaluated in this article, or claim that may be made by its manufacturer, is not guaranteed or endorsed by the publisher.
References
Aben, R. C. H., Barros, N., van Donk, E., Frenken, T., Hilt, S., Kazanjian, G., et al. (2017). Cross continental increase in methane ebullition under climate change. Nat. Commun. 8, 1682. doi:10.1038/s41467-017-01535-y
Andresen, C. G., Lara, M. J., Tweedie, C. E., and Lougheed, V. L. (2017). Rising plant-mediated methane emissions from arctic wetlands. Glob. Change Biol. 23, 1128–1139. doi:10.1111/gcb.13469
Annett, R., Habibi, H. R., and Hontela, A. (2014). Impact of glyphosate and glyphosate-based herbicides on the freshwater environment: impact of glyphosate-based herbicides. J. Appl. Toxicol. 34, 458–479. doi:10.1002/jat.2997
Aparicio, V. C., De Gerónimo, E., Marino, D., Primost, J., Carriquiriborde, P., and Costa, J. L. (2013). Environmental fate of glyphosate and aminomethylphosphonic acid in surface waters and soil of agricultural basins. Chemosphere 93, 1866–1873. doi:10.1016/j.chemosphere.2013.06.041
Bai, S. H., and Ogbourne, S. M. (2016). Glyphosate: environmental contamination, toxicity and potential risks to human health via food contamination. Environ. Sci. Pollut. Res. 23, 18988–19001. doi:10.1007/s11356-016-7425-3
Baker, B. J., De Anda, V., Seitz, K. W., Dombrowski, N., Santoro, A. E., and Lloyd, K. G. (2020). Diversity, ecology and evolution of Archaea. Nat. Microbiol. 5, 887–900. doi:10.1038/s41564-020-0715-z
Beaulieu, J. J., DelSontro, T., and Downing, J. A. (2019). Eutrophication will increase methane emissions from lakes and impoundments during the 21st century. Nat. Commun. 10, 1375. doi:10.1038/s41467-019-09100-5
Beecraft, L., and Rooney, R. (2021). Bioconcentration of glyphosate in wetland biofilms. Sci. Total Environ. 756, 143993. doi:10.1016/j.scitotenv.2020.143993
Benbrook, C. M. (2016). Trends in glyphosate herbicide use in the United States and globally. Environ. Sci. Eur. 28, 3. doi:10.1186/s12302-016-0070-0
Benndorf, D., Davidson, I., and Babel, W. (2004). Regulation of catabolic enzymes during long-term exposure of Delftia acidovorans MC1 to chlorophenoxy herbicides. Microbiology 150, 1005–1014. doi:10.1099/mic.0.26774-0
Bento, C. P. M., Goossens, D., Rezaei, M., Riksen, M., Mol, H. G. J., Ritsema, C. J., et al. (2017). Glyphosate and AMPA distribution in wind-eroded sediment derived from loess soil. Environ. Pollut. 220, 1079–1089. doi:10.1016/j.envpol.2016.11.033
Bérard, A., and Benninghoff, C. (2001). Pollution-induced community tolerance (PICT) and seasonal variations in the sensitivity of phytoplankton to atrazine in nanocosms. Chemosphere 45, 427–437. doi:10.1016/S0045-6535(01)00063-7
Bernardi, F., Lirola, J. R., Cestari, M. M., and Bombardelli, R. A. (2022). Effects on reproductive, biochemical and genotoxic parameters of herbicides 2,4-D and glyphosate in silver catfish (Rhamdia quelen). Environ. Toxicol. Pharmacol. 89, 103787. doi:10.1016/j.etap.2021.103787
Bertolet, B. L., Olson, C. R., Szydlowski, D. K., Solomon, C. T., and Jones, S. E. (2020). Methane and primary productivity in lakes: divergence of temporal and spatial relationships. J. Geophys. Res. Biogeosci. 125. doi:10.1029/2020JG005864
Bertuzzo, E., Thomet, M., Botter, G., and Rinaldo, A. (2013). Catchment-scale herbicides transport: theory and application. Adv. Water Resour. 52, 232–242. doi:10.1016/j.advwatres.2012.11.007
Blanck, H. (2002). A critical review of procedures and approaches used for assessing pollution-induced community tolerance (PICT) in biotic communities. Hum. Ecol. Risk Assess. Int. J. 8, 1003–1034. doi:10.1080/1080-700291905792
Carles, L., Gardon, H., Joseph, L., Sanchís, J., Farré, M., and Artigas, J. (2019). Meta-analysis of glyphosate contamination in surface waters and dissipation by biofilms. Environ. Int. 124, 284–293. doi:10.1016/j.envint.2018.12.064
Carvalho, W. F., Ruiz De Arcaute, C., Torres, L., De Melo E Silva, D., Soloneski, S., and Larramendy, M. L. (2020). Genotoxicity of mixtures of glyphosate with 2,4-dichlorophenoxyacetic acid chemical forms towards Cnesterodon decemmaculatus (Pisces, Poeciliidae). Environ. Sci. Pollut. Res. 27, 6515–6525. doi:10.1007/s11356-019-07379-x
Casado, J., Brigden, K., Santillo, D., and Johnston, P. (2019). Screening of pesticides and veterinary drugs in small streams in the European Union by liquid chromatography high resolution mass spectrometry. Sci. Total Environ. 670, 1204–1225. doi:10.1016/j.scitotenv.2019.03.207
Cobb, A. H., and Reade, J. P. H. (2010). Herbicides and plant physiology: cobb/herbicides and plant physiology. Oxford, UK: Wiley-Blackwell. Available at: http://doi.wiley.com/10.1002/9781444327793 (Accessed June 14, 2022).
Coupe, R. H., and Capel, P. D. (2016). Trends in pesticide use on soybean, corn and cotton since the introduction of major genetically modified crops in the United States: pesticide use on US soybean, corn and cotton since the introduction of GM crops. Pest. Manag. Sci. 72, 1013–1022. doi:10.1002/ps.4082
Delcour, I., Spanoghe, P., and Uyttendaele, M. (2015). Literature review: impact of climate change on pesticide use. Food Res. Int. 68, 7–15. doi:10.1016/j.foodres.2014.09.030
de Lipthay, J. R., Aamand, J., and Barkay, T. (2002). Expression of tfdA genes in aquatic microbial communities during acclimation to 2,4-dichlorophenoxyacetic acid. FEMS Microbiol. Ecol. 40, 205–214. doi:10.1111/j.1574-6941.2002.tb00953.x
DeLorenzo, M. E., Scott, G. I., and Ross, P. E. (2001). Toxicity of pesticides to aquatic microorganisms: a review. Environ. Toxicol. Chem. 20, 84–98. doi:10.1002/etc.5620200108
Donald, D. B., Cessna, A. J., and Farenhorst, A. (2018). Concentrations of herbicides in wetlands on organic and minimum-tillage farms. J. Environ. Qual. 47, 1554–1565. doi:10.2134/jeq2018.03.0100
Duke, S. O. (2020). Glyphosate: environmental fate and impact. Weed Sci. 68, 201–207. doi:10.1017/wsc.2019.28
EPA (2022). Registration of enlist one and enlist Duo. Available at: https://www.epa.gov/ingredients-used-pesticide-products/registration-enlist-one-and-enlist-duo.
EPA (2023). Overview of greenhouse gases greenhouse gas emissions. Available at: https://www.epa.gov/ghgemissions/overview-greenhouse-gases.
Faust, M., Altenburger, R., Boedeker, W., and Grimme, L. H. (1994). Algal toxicity of binary combinations of pesticides. Bull. Environ. Contam. Toxicol. 53, 134–141. doi:10.1007/BF00205150
Feld, L., Hjelmsø, M. H., Nielsen, M. S., Jacobsen, A. D., Rønn, R., Ekelund, F., et al. (2015). Pesticide side effects in an agricultural soil ecosystem as measured by amoA expression quantification and bacterial diversity changes. PLoS ONE 10, e0126080. doi:10.1371/journal.pone.0126080
Freydier, L., and Lundgren, J. G. (2016). Unintended effects of the herbicides 2,4-D and dicamba on lady beetles. Ecotoxicology 25, 1270–1277. doi:10.1007/s10646-016-1680-4
Gardner, S. C., and Grue, C. E. (1996). Effects of rodeo® and garlon® 3A on nontarget wetland species in central Washington. Environ. Toxicol. Chem. 15, 441–451. doi:10.1002/etc.5620150406
Hayes, T. B., Case, P., Chui, S., Chung, D., Haeffele, C., Haston, K., et al. (2006). Pesticide mixtures, endocrine disruption, and Amphibian declines: are we underestimating the impact? Environ. Health Perspect. 114, 40–50. doi:10.1289/ehp.8051
Hébert, M. P., Fugère, V., and Gonzalez, A. (2019). The overlooked impact of rising glyphosate use on phosphorus loading in agricultural watersheds. Front. Ecol. Environ. 17, 48–56. doi:10.1002/fee.1985
Helander, M., Saloniemi, I., and Saikkonen, K. (2012). Glyphosate in northern ecosystems. Trends Plant Sci. 17, 569–574. doi:10.1016/j.tplants.2012.05.008
Hernández-García, C. I., and Martínez-Jerónimo, F. (2020). Multistressor negative effects on an experimental phytoplankton community. The case of glyphosate and one toxigenic cyanobacterium on Chlorophycean microalgae. Sci. Total Environ. 717, 137186. doi:10.1016/j.scitotenv.2020.137186
Herrmann, K. M., and Weaver, L. M. (1999). THE SHIKIMATE PATHWAY. Annu. Rev. Plant Physiol. Plant Mol. Biol. 50, 473–503. doi:10.1146/annurev.arplant.50.1.473
Hetrick, J., and Blankinship, A. (2015). Preliminary ecological risk assessment in support of the registration review of glyphosate and its salts. USA: U.S. Environmental Protection Agency: Office of Chemical Safety and Pollution Prevention.
IPCC (2021). IPCC, 2021: climate change 2021: the physical science basis, the working group I contribution to the sixth assesment report. Cambridge, United Kingdom and New York, NY, USA: Cambridge University Press.
Islam, F., Wang, J., Farooq, M. A., Khan, M. S. S., Xu, L., Zhu, J., et al. (2018). Potential impact of the herbicide 2,4-dichlorophenoxyacetic acid on human and ecosystems. Environ. Int. 111, 332–351. doi:10.1016/j.envint.2017.10.020
Koleva, N. G., and Schneider, U. >A. (2010). The impact of climate change on aquatic risk from agricultural pesticides in the US. Int. J. Environ. Stud. 67, 677–704. doi:10.1080/00207233.2010.507477
Kumaraswamy, S., Ramakrishnan, B., Satpathy, S. N., Rath, A. K., Misra, S., Rao, V. R., et al. (1997). Spatial distribution of methane-oxidizing activity in a flooded rice soil. Plant Soil 191, 241–248. doi:10.1023/A:1004274302326
Larras, F., Rimet, F., Gregorio, V., Bérard, A., Leboulanger, C., Montuelle, B., et al. (2016). Pollution-induced community tolerance (PICT) as a tool for monitoring Lake Geneva long-term in situ ecotoxic restoration from herbicide contamination. Environ. Sci. Pollut. Res. 23, 4301–4311. doi:10.1007/s11356-015-5302-0
Lichtenstein, E. P., Liang, T. T., and Anderegg, B. N. (1973). Synergism of insecticides by herbicides. Science 181, 847–849. doi:10.1126/science.181.4102.847
Lin, W., Zhang, Z., Chen, Y., Zhang, Q., Ke, M., Lu, T., et al. (2023). The mechanism of different cyanobacterial responses to glyphosate. J. Environ. Sci. 125, 258–265. doi:10.1016/j.jes.2021.11.039
Lozano, V. L., Allen Dohle, S., Vera, M. S., Torremorell, A., and Pizarro, H. N. (2020). Primary production of freshwater microbial communities is affected by a cocktail of herbicides in an outdoor experiment. Ecotoxicol. Environ. Saf. 201, 110821. doi:10.1016/j.ecoenv.2020.110821
Lozano, V. L., Vinocur, A., Sabio y García, C. A., Allende, L., Cristos, D. S., Rojas, D., et al. (2018). Effects of glyphosate and 2,4-D mixture on freshwater phytoplankton and periphyton communities: a microcosms approach. Ecotoxicol. Environ. Saf. 148, 1010–1019. doi:10.1016/j.ecoenv.2017.12.006
Lu, T., Xu, N., Zhang, Q., Zhang, Z., Debognies, A., Zhou, Z., et al. (2020). Understanding the influence of glyphosate on the structure and function of freshwater microbial community in a microcosm. Environ. Pollut. 260, 114012. doi:10.1016/j.envpol.2020.114012
Malaj, E., Liber, K., and Morrissey, C. A. (2020). Spatial distribution of agricultural pesticide use and predicted wetland exposure in the Canadian Prairie Pothole Region. Sci. Total Environ. 718, 134765. doi:10.1016/j.scitotenv.2019.134765
Matzrafi, M. (2019). Climate change exacerbates pest damage through reduced pesticide efficacy. Pest Manag. Sci. 75, 9–13. doi:10.1002/ps.5121
Mierzejewska, E., and Urbaniak, M. (2022). “Phenoxy herbicides in aquatic ecosystems: environmental levels, toxicological effects, and remediation methods,” in Legacy and emerging contaminants in water and wastewater emerging contaminants and associated treatment technologies. Editors P. Chakraborty, and D. Snow (Cham: Springer International Publishing), 361–388. doi:10.1007/978-3-030-95443-7_16
Moreira, R. A., Rocha, G. S., Da Silva, L. C. M., Goulart, B. V., Montagner, C. C., Melão, M. D. G. G., et al. (2020). Exposure to environmental concentrations of fipronil and 2,4-D mixtures causes physiological, morphological and biochemical changes in Raphidocelis subcapitata. Ecotoxicol. Environ. Saf. 206, 111180. doi:10.1016/j.ecoenv.2020.111180
Noyes, P. D., McElwee, M. K., Miller, H. D., Clark, B. W., Van Tiem, L. A., Walcott, K. C., et al. (2009). The toxicology of climate change: environmental contaminants in a warming world. Environ. Int. 35, 971–986. doi:10.1016/j.envint.2009.02.006
Pavan, F. A., Samojeden, C. G., Rutkoski, C. F., Folador, A., Da Fré, S. P., Müller, C., et al. (2021). Morphological, behavioral and genotoxic effects of glyphosate and 2,4-D mixture in tadpoles of two native species of South American amphibians. Environ. Toxicol. Pharmacol. 85, 103637. doi:10.1016/j.etap.2021.103637
Peluso, J., Furió Lanuza, A., Pérez Coll, C. S., and Aronzon, C. M. (2022). Synergistic effects of glyphosate- and 2,4-D-based pesticides mixtures on Rhinella arenarum larvae. Environ. Sci. Pollut. Res. 29, 14443–14452. doi:10.1007/s11356-021-16784-0
Pesce, S., Margoum, C., and Foulquier, A. (2016). Pollution-induced community tolerance for in situ assessment of recovery in river microbial communities following the ban of the herbicide diuron. Agric. Ecosyst. Environ. 221, 79–86. doi:10.1016/j.agee.2016.01.009
Peterson, M. A., Collavo, A., Ovejero, R., Shivrain, V., and Walsh, M. J. (2018). The challenge of herbicide resistance around the world: a current summary. Pest Manag. Sci. 74, 2246–2259. doi:10.1002/ps.4821
Peterson, M. A., McMaster, S. A., Riechers, D. E., Skelton, J., and Stahlman, P. W. (2016). 2,4-D past, present, and future: a review. Weed Technol. 30, 303–345. doi:10.1614/WT-D-15-00131.1
Portinho, J. L., Nielsen, D. L., Daré, L., Henry, R., Oliveira, R. C., and Branco, C. C. Z. (2018). Mixture of commercial herbicides based on 2,4-D and glyphosate mixture can suppress the emergence of zooplankton from sediments. Chemosphere 203, 151–159. doi:10.1016/j.chemosphere.2018.03.156
Qiu, H., Geng, J., Ren, H., Xia, X., Wang, X., and Yu, Y. (2013). Physiological and biochemical responses of Microcystis aeruginosa to glyphosate and its Roundup® formulation. J. Hazard. Mater. 248–249, 172–176. doi:10.1016/j.jhazmat.2012.12.033
Relyea, R. A. (2009). A cocktail of contaminants: how mixtures of pesticides at low concentrations affect aquatic communities. Oecologia 159, 363–376. doi:10.1007/s00442-008-1213-9
Reno, U., Gutierrez, M. F., Regaldo, L., and Gagneten, A. M. (2014). The impact of Eskoba®, a glyphosate formulation, on the freshwater plankton community. water Environ. Res. 86, 2294–2300. doi:10.2175/106143014X13896437493580
Roodt, A. P., Huszarik, M., Entling, M. H., and Schulz, R. (2023). Aquatic-terrestrial transfer of neonicotinoid insecticides in riparian food webs. J. Hazard. Mater. 455, 131635. doi:10.1016/j.jhazmat.2023.131635
Rotter, S., Heilmeier, H., Altenburger, R., and Schmitt-Jansen, M. (2013). Multiple stressors in periphyton - comparison of observed and predicted tolerance responses to high ionic loads and herbicide exposure. J. Appl. Ecol. 50, 1459–1468. doi:10.1111/1365-2664.12146
Sachu, M., Kynshi, B. L., and Syiem, M. B. (2022). A biochemical, physiological and molecular evaluation of how the herbicide 2, 4-dichlorophenoxyacetic acid intercedes photosynthesis and diazotrophy in the cyanobacterium Nostoc muscorum Meg 1. Environ. Sci. Pollut. Res. 29, 36684–36698. doi:10.1007/s11356-021-18000-5
Saxton, M. A., Morrow, E. A., Bourbonniere, R. A., and Wilhelm, S. W. (2011). Glyphosate influence on phytoplankton community structure in Lake Erie. J. Gt. Lakes. Res. 37, 683–690. doi:10.1016/j.jglr.2011.07.004
Schütte, G., Eckerstorfer, M., Rastelli, V., Reichenbecher, W., Restrepo-Vassalli, S., Ruohonen-Lehto, M., et al. (2017). Herbicide resistance and biodiversity: agronomic and environmental aspects of genetically modified herbicide-resistant plants. Environ. Sci. Eur. 29, 5. doi:10.1186/s12302-016-0100-y
Seghers, D., Siciliano, S. D., Top, E. M., and Verstraete, W. (2005). Combined effect of fertilizer and herbicide applications on the abundance, community structure and performance of the soil methanotrophic community. Soil Biol. Biochem. 37, 187–193. doi:10.1016/j.soilbio.2004.05.025
Sepulveda-Jauregui, A., Hoyos-Santillan, J., Martinez-Cruz, K., Walter Anthony, K. M., Casper, P., Belmonte-Izquierdo, Y., et al. (2018). Eutrophication exacerbates the impact of climate warming on lake methane emission. Sci. Total Environ. 636, 411–419. doi:10.1016/j.scitotenv.2018.04.283
Singh, S., Kumar, V., Gill, J. P. K., Datta, S., Singh, S., Dhaka, V., et al. (2020). Herbicide glyphosate: toxicity and microbial degradation. IJERPH 17, 7519. doi:10.3390/ijerph17207519
Spaepen, S., and Vanderleyden, J. (2011). Auxin and plant-microbe interactions. Cold Spring Harb. Perspect. Biol. 3, a001438. doi:10.1101/cshperspect.a001438
Stehle, S., and Schulz, R. (2015). Agricultural insecticides threaten surface waters at the global scale. Proc. Natl. Acad. Sci. U. S. A. 112, 5750–5755. doi:10.1073/pnas.1500232112
Sun, K., Liu, W., Liu, L., Wang, N., and Duan, S. (2013). Ecological risks assessment of organophosphorus pesticides on bloom of Microcystis wesenbergii. Int. Biodeterior. Biodegrad. 77, 98–105. doi:10.1016/j.ibiod.2012.11.010
Sun, M., Li, H., and Jaisi, D. P. (2019). Degradation of glyphosate and bioavailability of phosphorus derived from glyphosate in a soil-water system. Water Res. 163, 114840. doi:10.1016/j.watres.2019.07.007
Sura, S., Waiser, M. J., Tumber, V., Raina-Fulton, R., and Cessna, A. J. (2015). Effects of a herbicide mixture on primary and bacterial productivity in four prairie wetlands with varying salinities: an enclosure approach. Sci. Total Environ. 512 (513), 526–539. doi:10.1016/j.scitotenv.2015.01.064
Syamsul Arif, M. A., Houwen, F., and Verstraete, W. (1996). Agricultural factors affecting methane oxidation in arable soil. Biol. Fertil. Soils 21, 95–102. doi:10.1007/BF00335999
Tang, F. H. M., Jeffries, T. C., Vervoort, R. W., Conoley, C., Coleman, N. V., and Maggi, F. (2019). Microcosm experiments and kinetic modeling of glyphosate biodegradation in soils and sediments. Sci. Total Environ. 658, 105–115. doi:10.1016/j.scitotenv.2018.12.179
Top, E. M., Maila, M. P., Clerinx, M., Goris, J., Vos, P., and Verstraete, W. (1999). Methane oxidation as a method to evaluate the removal of 2,4-dichlorophenoxyactic acid (2,4-D) from soil by plasmid-mediated bioaugmentation. FEMS Microbiol. Ecol. 28, 203–213. doi:10.1111/j.1574-6941.1999.tb00576.x
Vera, M. S., Di Fiori, E., Lagomarsino, L., Sinistro, R., Escaray, R., Iummato, M. M., et al. (2012). Direct and indirect effects of the glyphosate formulation Glifosato Atanor® on freshwater microbial communities. Ecotoxicology 21, 1805–1816. doi:10.1007/s10646-012-0915-2
Vera, M. S., Lagomarsino, L., Sylvester, M., Pérez, G. L., Rodríguez, P., Mugni, H., et al. (2010). New evidences of Roundup® (glyphosate formulation) impact on the periphyton community and the water quality of freshwater ecosystems. Ecotoxicology 19, 710–721. doi:10.1007/s10646-009-0446-7
Wang, S., Seiwert, B., Kästner, M., Miltner, A., Schäffer, A., Reemtsma, T., et al. (2016). Bio)degradation of glyphosate in water-sediment microcosms – a stable isotope co-labeling approach. Water Res. 99, 91–100. doi:10.1016/j.watres.2016.04.041
Wang, W., Jiang, M., and Sheng, Y. (2021). Glyphosate accelerates the proliferation of microcystis aeruginosa, a dominant species in cyanobacterial blooms. Environ. Toxicol. Chem. 40, 342–351. doi:10.1002/etc.4942
Wolfram, J., Bub, S., Petschick, L. L., Schemmer, A., Stehle, S., and Schulz, R. (2023). Pesticide occurrence in protected surface waters in nature conservation areas of Germany. Sci. Total Environ. 3, 858doi:10.1016/j.scitotenv.2022.160074
Yang, Y., Chen, J., Tong, T., Li, B., He, T., Liu, Y., et al. (2019). Eutrophication influences methanotrophic activity, abundance and community structure in freshwater lakes. Sci. Total Environ. 662, 863–872. doi:10.1016/j.scitotenv.2019.01.307
Zabaloy, M. C., Garland, J. L., and Gómez, M. A. (2008). An integrated approach to evaluate the impacts of the herbicides glyphosate, 2,4-D and metsulfuron-methyl on soil microbial communities in the Pampas region, Argentina. Appl. Soil Ecol. 40, 1–12. doi:10.1016/j.apsoil.2008.02.004
Zhang, Q., Zhou, H., Li, Z., Zhu, J., Zhou, C., and Zhao, M. (2016). Effects of glyphosate at environmentally relevant concentrations on the growth of and microcystin production by Microcystis aeruginosa. Environ. Monit. Assess. 188, 632. doi:10.1007/s10661-016-5627-2
Keywords: glyphosate, 2,4-D, wetlands, methane, climate change
Citation: Cornish CM and Sweetman JN (2023) A perspective on how glyphosate and 2,4-D in wetlands may impact climate change. Front. Environ. Sci. 11:1282821. doi: 10.3389/fenvs.2023.1282821
Received: 24 August 2023; Accepted: 24 October 2023;
Published: 06 November 2023.
Edited by:
Rajeev Pratap Singh, Banaras Hindu University, IndiaReviewed by:
Simone Jaqueline Cardoso, Juiz de Fora Federal University, BrazilCopyright © 2023 Cornish and Sweetman. This is an open-access article distributed under the terms of the Creative Commons Attribution License (CC BY). The use, distribution or reproduction in other forums is permitted, provided the original author(s) and the copyright owner(s) are credited and that the original publication in this journal is cited, in accordance with accepted academic practice. No use, distribution or reproduction is permitted which does not comply with these terms.
*Correspondence: Christine M. Cornish, christine.cornish@ndsu.edu