- Joseph R. Biden, Jr. School of Public Policy and Administration, University of Delaware, Newark, DE, United States
Bioplastics have the potential to fill the role of conventional plastics but with lowered environmental and ecological impacts. But bioplastic production suffers from high production costs and as an immature technology, it proves less competitive than its petrol-based counterpart. Debates about the social versus private benefits of bioplastics are also cited. The literature argues that various bio-feedstock sources can produce high-quality drop-in plastics and that scaling up bioplastic production will provide the cost competitiveness needed to transition away from petroplastics. However, the market remains uncoordinated and lacks a strategic and comprehensive plan for the plastic transition. Moreover, the science-to-policy literature on bioplastics is very limited, providing scarce evidence or analysis to policymakers attempting to argue for bioplastics industrialization and integration. In this study we highlight this missing link particularly in the North American context in order to encourage further inquiry on these matters. Using Stern’s policy framework gap analysis approach, our evaluation identifies gaps in existing policy frameworks pertinent to bioplastics supply chains. On this basis we identify and prioritize five pointed areas for policy focus to advance bioplastics sector growth and integration. These are developing a strategy to sustainably coordinate and promote biomass production; incentivizing bioplastic investments and production; incentivizing bioplastic substitution; and enhancing the end-use management. Additionally, research is needed to support the technical performance of bioplastics, industrialization methods, supply chain integration, and the impact of exogenous factors.
1 Introduction
Plastics are one of the most versatile and useful materials in the world, and yet one of the most damaging materials. Not only does the existence of plastics impose significant damage to human and marine life (UNEP, 2018), but conventional plastics support our continued dependence on fossil fuels as they are derived from petroleum. Moreover, conventional plastics emit harmful greenhouse gases at every stage of their life cycle (Shen, et al., 2020). Bioplastics can potentially replace petroleum-based plastics (“petroplastics”) towards reducing the environmental footprint of plastics and the industries dependent on them. Moreover, some forms of bio-based plastics present lower carbon footprints of up to 80 percent (Suarez, et al., 2022). While bioplastics have successfully been established for packaging, automatic parts, toys, 3D printing, electronics, and agriculture (Jabeen, et al., 2015; Thakur, et al., 2018), the industry’s success is very much constrained. Limited technical substitution of biodegradable plastics, high production costs, and supply chain restrictions impede efforts to sustainably upscale production (Samer, et al., 2022). Efforts to transform the linear waste-producing business models into more green and circular models continue to emerge, but the actualization of circular supply chain designs is hindered by financial, technical, societal, and institutional barriers (Masi, et al., 2017). Bioplastics also fail to achieve cost competitiveness in the current conditions and will remain out unattractive relative to petroplastics (Horvat, et al., 2018).
The discussion becomes increasingly complex as we consider the variations in performance, environmental impact, and economic considerations across various feedstock. While conventional plastics supply chains are streamlined from petroleum-based chemicals, bio feedstock ranges from starch-based, protein-based, vegetable/seed oil-based, and those produced from biological waste (Sidek, et al., 2019). A few of the main plant-based feedstock are corn, sugar, and grains, with vegetable oils being sourced from soy, rapeseed, and jatropha. These crop-based sources impose heavy demand for agricultural land and require the use of pesticides and fertilizers (Bishop, et al., 2021). They are arguably the most economical biomass options, but they also compete for agricultural land and may potentially limit food security (Popp, et al., 2014). Emerging technologies expand the scope of possible biomass feedstock, including forest thinning, food waste, and municipal waste. Food and agricultural waste provide an alternative feedstock that does not impose social and environmental pressures. On the contrary, linking waste management with biomass supply supports the circular economy. However, this technology tends to be increasingly costly given its tendency to develop pathogens when transported over long distances (Bishop, et al., 2022). Other sources such as lignocellulosic biomass have strong potential to be both carbon-neutral and economically feasible though technologically immature. Fibrous plant material such as forest thinning, straw, hemp, etc. has significant advantages as they can be easily sourced, inexpensive, and has competitive technical properties (Abe, et al., 2021). While these solve the issues associated with crop-based sources, they impose the risk of incentivizing deforestation (Bishop, et al., 2021).
The term bioplastics has been used to address any biodegradable plastics regardless of their input material. For this study, we define bioplastics in alignment with modern convention as plastics made wholly or partially from biobased materials and can be both biodegradable and non-biodegradable (Shen, et al., 2009). The latter are biopolymer materials produced from bio-based degradable feedstock but converted into final polymer structures that are not biodegradable (Endres, 2017). Biomass farmers/suppliers can be independent of the companies that refine the raw materials or be merged into farmer-company models (Cai, et al., 2021). The attraction to bioplastics stems from its potential to remove the dependency on petroleum while addressing the mounting difficulties of managing non-biodegradable solid waste and reducing greenhouse gas emissions. Accordingly, bioplastics falls within the category of green technologies. Though there is no single agreed-upon definition of what constitutes “green technology,” the term is used when comparing the relationship between technology and its impact on ecology (Heng and Zou, 2010). Earlier work by Braun and Wield (1994) identified those product and process innovations that improve energy efficiency and reduce environmental impacts as those that are “green.” Others may broadly define green technologies as those that allow harmonious development between humans and their environment (Guo, et al., 2020). Given that no product exists with zero environmental impact, both biobased and biodegradable plastics arguably satisfy the green criteria (Moshood, et al., 2022).
Unfortunately, its cost structure does not allow for a seamless transition led by market forces. Bioplastics are more socially valuable than economically feasible. Moreover, bioplastics are not all biodegradable or without environmental impacts. The biobased forms of the most utilized conventional plastics like polyethylene (PE), polypropylene (PP), poly(vinyl chloride) (PVC), poly(ethylene terephthalate) (PET), and polyurethane (PUR) all remain non-biodegradable. Drop-in bioplastics, those which can immediately replace conventional without process disruptions, have the highest technical substitution potential (Brizga, et al., 2020). However, the forms of bioplastics which can induce sustainability need more policy support to increase viability.
Ultimately, the integration of bioplastics balances the need to achieve sustainability and meet the technical and economic requirements of the industry. There has been increasing research interest in bioplastics over the last decade. However, the policy requirements to accomplish its material substitution remain understudied (Shafqat, et al., 2020). Many publications on bioplastics are within the material sciences, engineering, chemistry, and biology fields, with almost nonexistent representation by policy-related journals (Garrido, et al., 2021). However, scientific discoveries require policy assistance for technology adaptation due to risk, constraints, support systems, and financial and non-financial returns (Foster and Rosenzweig, 2010). These questions are not adequately addressed from the point of view of hard sciences but require a more economic approach. This paper aims to understand the gaps in the literature that may infringe on, drive or hinder transitions. It does so to initiate a research agenda that allows reduced uncertainty and risk for investors and guidance for the strategic development of the industry. We present this in an effort to further the substitution of petroplastics for bioplastics in industries by assessing gaps within the supply chain and policy framework.
In the following sections we discuss the US plastics industry, outline the process of integrating bioplastics, and finally provide analyses of the supply chain and existing policies related to bioplastics. This paper adapts the approach outlined by Stern (2004b), to evaluate the gaps within the current US policy framework in accomplishing the integration of bioplastics. The successful integration of bioplastics requires higher production levels, sufficient supply of factors of production, investments into bioplastics and biomass production, and finally demand conditions necessary for material substitution. Given these conditions, the missing links were identified for research and policy attention.
2 Structure of the modern US plastics industry
Plastics make a substantial contribution to the US economy. Being the sixth largest industry in the USA, the plastics and resin industry earns $468 billion in shipments and employs almost one billion (Plastics, 2022). 25.8% of the final consumption of plastic products is absorbed by the service and wholesale and retail trade, and 89 percent of household consumption is made up of plastic-containing goods. Value-added production of plastics and rubber grew an average of 3.5 percent per year, while the average growth of the manufacturing output for plastic products was 5.4 percent from 2010 to2022.1 Therefore, both the direct and indirect importance and impact of plastics on the US economy are substantial.
The US plastics and resin industry is characterized by many producers in an unconcentrated market with moderate levels of competition, capital intensity, and barriers to entry (Berdousis, 2023). Plastic production activities (see Figure 1)2 are spread across the country with the greatest concentration of activity in the Midwest, Northeast, and Southeastern states, though Texas and California also fall within the top three states for value-added plastics product production. Facilities are typically established near oil refineries, hence the Southeast and Texas, or strategically aligned to access international markets, hence near the Great Lakes and the country’s largest port in California. The industry’s output consists of pipes, windows, moldings, and flooring supplied to the construction sector; dashboard panels, bumpers protective covers, and other components in automobiles; and bottles and packaging for food and beverages. As such, 50 percent of the industry output is supplied to five industries while the remaining 50 percent is split between 64 other industries (see Figure 2)3. Moreover, plastic production followed a generally upward trend over the past two decades, with a maximum output of over 40 million metric tons produced after 2020 (see Figure 3)4. As Figure 3 displays, the three forms of polyethylene account for more than 50 percent of the plastics produced. Linear low-density polyethylene (LLDPE) is typically used in plastic bags, thin plastic wrappings, and other products that require high levels of flexibility. Alternatively, high-density polyethylene (HDPE) is used more for its strength and durability in more industrial applications and containers (Milbrandt, et al., 2022). The plastic industry is deeply connected to the oil and gas sector, and the emergence of biofuel and bioplastics has brought linkages to the agricultural sectors as well. The Northeast region experiences a struggle for power over its economic and policy trajectory as social pressures arise to establish a bioeconomy and to deviate away from the dominance of corporations. As such, the conflicting environmental, social, and economic interests shape the industry’s development (Burnham, et al., 2017).
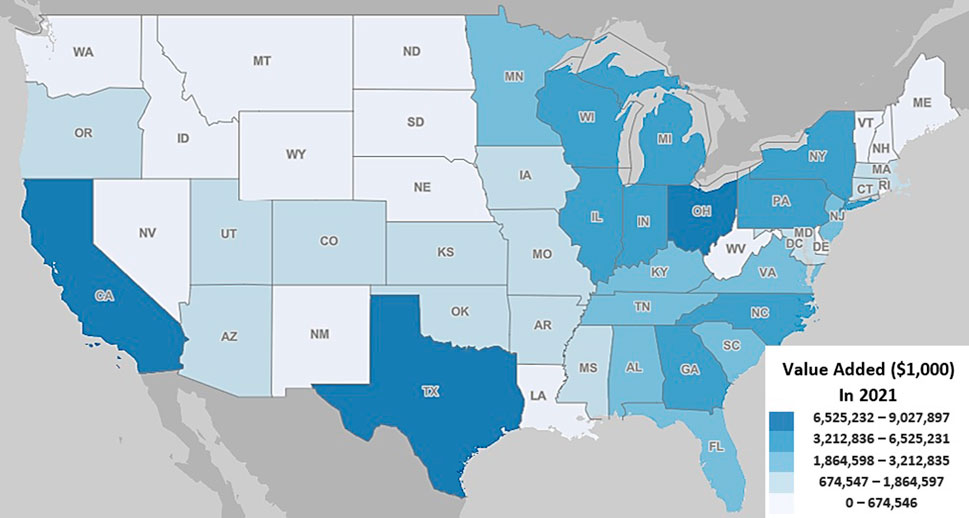
FIGURE 1. Value added production of plastics product manufacturing industry - adapted from the US Census Bureau database—industry NAICS 3261.
Plastics continue to be one of the most versatile materials providing inputs and products for almost all industries. The industry earned about $336.9 billion in revenue in 2021, with $225.8 billion earned by plastic production only.5 However, surging oil prices and inflation have driven up the cost of production, and the economic slowdown of the pandemic has produced a 10.3 percent decline in profits for the period 2017–2022 (Berdousis, 2023). Though profits are projected to rebound as input costs regularize, the contracting construction sector and greater public concerns against plastics suggest increasing risk and uncertainty in the industry.
3 Methodology
The goal of this study is to evaluate the gaps within the current US policy framework in accomplishing the supply chain integration of bioplastics. The dimensions of the study included the key players of the plastics industry, the legislative framework that influences the governance and activities within the industry, and the socioeconomic forces that influence decision-making. Evaluation theory is applied to design policy interventions, improve the efficiency of resource allocation, enhance intervention quality, and create accountability after implementation (European Commission, 2004). Evaluations are molded through a dynamic relationship between the theory, evidence/current events, and experience. Consequently, context generates the goals and challenges faced (Stern, 2004a).
This paper adapts the approach outlined by Stern (2004b), to evaluate the gaps within the current US policy framework in accomplishing the integration of bioplastics. Broadly, Stern’s simplified methodological approach emphasizes three stages of reasoning (see Figure 4). Firstly, the policy objective is identified, along with the necessary criteria to achieve success. Secondly, a causal link is made to connect the intervention to success. Finally, changes are identified for improving programs, processes and participants that activate those causal links (Kaczmarek and Romaniuk, 2020).
The steps taken in this study are outlined as follows. Firstly, a model of the plastic supply chain was outlined from individual bioplastics and conventional plastics supply chains. These supply chain models were published in industry reports obtained from the IBISWorld database (Bari, 2022; Berdousis, 2023). Next, official government websites were surveyed for reports using key terms such as “bioeconomy,” “bioplastics,” “biofuel,” and “biomass.” The main sources of information were the official websites of the US Department of Agriculture, the Department of Energy, the Federal Register, Congressional Research Services, and the National Center for Biotechnology Information Advanced BioFuels USA. These included websites that also fall under the USDA such as the official websites of the National Institute of Food and Agriculture, the Economic Research Service, BioPreferred, and the Farm Service Agency. Links to these sources are included in Table 1. The reports obtained from these sources were then analyzed for interactions between key stakeholders, policies implemented, details of government programs, and issues highlighted. These were then cross-referenced with the plastic supply chain to highlight the gaps needed for better integration. These gaps were compared to the existing bioplastics literature to identify where further research is required.
The existing policy framework is evaluated on its potential to stimulate growth in bioplastic production and competitive substitution into the plastic supply chain. The successful integration of bioplastics requires higher production levels, sufficient supply of factors of production, investments into bioplastics and biomass production, and finally demand conditions necessary for material substitution. Given these conditions, the missing links were identified for research and policy attention. Importantly, our approach focuses on historic and current sector activities to answer questions of “what exists” and “what is missing.” Consequently, it allocates less attention to the deeper dynamics within each existing feature, or factors exogenous to the industry and its supply chain. Instead, the goal is to draw attention to these factors for future research.
4 Bioplastic integration
To adequately address the environmental impact of the plastics industry, and to reduce its role in securing our petroleum dependence, conventional plastics must be replaced with more sustainable materials. However, the bioplastics industry cannot fully meet the capacity demanded, nor the technical specifications of all sectors. The task then is to outline the existing plastic supply chain and identify the weak points that constrain bioplastic integration.
Firstly, plastic manufacturers source their inputs from tier-one feedstock suppliers, who acquire their raw materials from extractive industries. Alternatively, bioplastic manufacturers source feedstock processed from biobased sources including oil-based crops, starch-based crops, sugar-based crops, lignocellulosic material, and algae (Wagemann and Tippkötter, 2017). The characteristics of biomass sources influence investment decisions, requiring policymakers to navigate the complexity of technical and economic options and their unique challenges. Agriculture-related biomass poses the risk of increasing land use and may require the use of fertilizers to meet suitable demand, while forest biomass can potentially incentivize deforestation (Bishop, et al., 2021). Biomass from municipal solid waste has a low yield and high cost of enzymes and labor. It is only economical if the production process incorporates energy generation, but this increases the environmental impacts as it requires the burning of fossil fuels (Izaguirre, et al., 2021). Moreover, the largest consumer of biomass feedstock is the biofuel industry, and there is insufficient supply to meet the current plastic demand (Bari, 2022). Hence, for bioplastics to match the output and demand in the existing industry, the supply of bio-feedstock must also be scaled up. Scaling production may imply encouraging new investments into bio-feedstock manufacturing as well as transitioning existing petrol-based suppliers. Biomass also relies on food-related crops such as corn, sugarcane, molasses, cassava, grains, and natural fats and oils (Bardhan, et al., 2015). Alternative high-yield sources of biomass, such as switchgrass, may be able to adjust the cost-benefit ratios as well as reduce the need to use food crops (Somleva, et al., 2008). Intrinsically, these decisions are also affected by the factors that constrain the supply of each feedstock type. Ultimately, bioplastic inputs can either increase their price or reduce their sustainability. As such, the most sustainable form of bioplastic product will most likely be constructed from a blend of biomass types with very heterogenous properties (Gerassimidou, et al., 2023). However, combining multiple biomass resources requires compatibilities of functionalities, structural features, and the dynamic changes within these compatibilities as other factors change (Ghodake, et al., 2021). Moreover, the final blend and its technical properties may not be easily predicted as it depends on the production capacity and economic viability of each biomass supplier, thus increasing the risk and uncertainty to investors.
Bioplastic manufacturers process the biobased feedstock into various forms required by tier-one buyers. Compared to the overall plastic and resin industry, bioplastic manufacturing falls short significantly in size with an estimated revenue of $864.7 million for 2022 (Bari, 2022). First and second-generation bioplastics, i.e., those sourced from crops and those sourced from lignocellulosic feedstocks, have achieved some degree of industrial scales, but incurred higher production costs (OECD, 2013; Gong, et al., 2023). True cost-saving economies of scale are yet to be achieved given technical and supply chain constraints sufficient, and an inability to attract sufficient investments. However, the lack of scale suggests that the industry has yet to attain the lower levels of the average cost associated with mass production (Rosenboom, et al., 2022). As such, there may be potential cost advantages of upscaling production leading to more competitive prices. Bioplastics have already successfully penetrated the market with the biobased polyethylene (PE), biobased poly (trimethylene terephthalate) (PTT), and biobased polyamides (PA) having the largest global bioplastic production capacities biobased (European Bioplastics, 2022).
The main cost drivers for bioplastics are the availability and cost of feedstock, the biochemical engineering technology, production scale, and policy support (Brizga, et al., 2020). It also depends on the technical substitutability which varies significantly across plastic types. Poly(lactide acid), biobased epoxy resin, and poly(ethylene) are estimated to have 90% technical substitutability for petroplastics (Shen, et al., 2010). Other literature argues that biobased polyamide and polyurethane have a substitutability of 80%, while some polyethylene only achieves 60% (Brizga, et al., 2020). However, the silver lining is that the highest rates of substitutability are found in the bio-based substitutes of conventional non-biodegradable plastics, allowing for the reduction of fossil fuel consumption at the very least. Evidence produced by Rhein (2020) suggests that in European markets, bioplastics with similar technical performance to their petroleum-based counterparts (drop-ins) were on average more likely to be adopted compared to bioplastics with new features. This suggests that companies may be more interested in replacing conventional plastics with bioplastics with the least amount of process disruptions. However, companies may not overlook the advantage of having greater control of composting conditions and shelf-life. This is the case as the choice of biomass inputs influences the speed of degradation (Karan, et al., 2019).
Low technical substitution of some bioplastics means fewer drop-in capabilities, more need to adjust production processes, and less efficient use of materials (Escobar, et al., 2018). Hence, advancing biotechnology and processing techniques are prerequisites to both performance and cost improvements (Karan, et al., 2019). Targeted research programs are required to improve the industrialization of bioplastics, improve its technical substitutability, as well as to advance the sustainability of the industry. Some bioplastics have a more harmful ecological impact than conventional bioplastics, while others promote increased use of land and water resources (Brizga, et al., 2020; Liu, et al., 2022).The inability to identify and separate bioplastics in end-use management undermines the sustainability objectives by limiting recyclability and increasing greenhouse gas (GHG) emissions. Hence, a revision of plastic identification standards and guidelines for life cycle assessment is necessary to guide converters, consumers, and recyclers to ensure a sustainable impact, (Rosenboom, et al., 2022). Currently, bioplastic standards are outlined by the International Organization for Standardization (ISO), European Committee for Standardization (CEN), and the American Society for Testing and Materials (ASTM). These address bioplastics concerning the marine environment, biobased content, environment, biodegradability, industrial composting and digestion, and communication of bioplastics (European Bioplastics, 2023).
The choice between conventional and bioplastics can either be influenced by restricting trade/use of petroplastics via policy or making bioplastics price competitive and allowing market forces to naturally execute the transition. However, the former would introduce inflationary pressures given the ubiquity of plastics in the economy. Hence, production costs must be addressed by scaling up production, supporting technical progress, and removing cost escalators. Average costs will fall as production is upscaled, however, as in the case of biofuel plants, investors are reluctant to invest in technologies that have not proven their commercial viability and scalability (CRS, 2015). Therefore, policies like public-private partnerships, production, and investment credits, or government pilot projects should be utilized to mitigate the risks and uncertainties of scaling up bioplastics technologies.
On the other hand, household consumption decisions may vary with income brackets and private companies based on their business model, i.e., product differentiation or price competition. Incorporating bioplastics, or biobased products in general, into ESG investment accounting allows tier-one buyers of industrial plastics to extract tax reliefs and other financial benefits from their more environmentally friendly purchases. Moreover, providing an investment or production tax credit for industries engaged in the sustainable production of renewable chemicals will also promote bioplastics industries (Singh, 2022). Heavy-handed policy options like banning conventional plastics would ensure the transition to bioplastics, however, with insufficient supply and technical substitutability this would introduce bottlenecks into the supply chain. Instead, akin to renewable fuel standards, bioplastic or broader biobased product quotas would lock in demand to potentially allow the industry to accelerate.
Conclusively, the successful integration of bioplastics requires attention at all points of the supply chain. The industry’s high costs, low substitutability, and low production of biomass constrain its ability to upscale production and attract investments. Moreover, attracting demand requires both cost improvements and performance improvements in bioplastics. Sustainability is also at risk given the existing technology, biomass sources, and standards for identifying plastics. As such, the growth of this industry will be limited without a comprehensive policy intervention.
5 The existing US policy environment for bioplastics
Policies already exist to develop the US bioeconomy by supporting biofuel and biomass production, although bioplastics are rarely addressed directly (OECD, 2013). However, they set both the foundation to understand what works for general biobased products, and what gaps remain for bioplastics. The National Biofuels Action Plan (NBAP) was developed in 2008 to ramp up biofuel production to help reduce US energy consumption and reliance on fuel imports (BRDB, 2008). The NBAP promoted biofuel production by addressing five aspects of the supply chain. Firstly, raw material inputs are supported to ensure a secured supply of biobased feedstock production. R&D activities were initiated and funded to promote the development and industrialization of biofuel conversion technology. Research activities were also initiated to reduce the costs associated with the collection and transportation of materials between facilities.
In 2012, the National Bioeconomy Blueprint (NBB) expanded on the NBAP intending to establish the US bioeconomy by removing barriers and developing a bioeconomy workforce (Frisvold, et al., 2021). The NBB outlaid strategies to initiate an integrated approach towards developing bioeconomy technologies, funding mechanisms, and partnerships from all angles. It coordinated the regulations and institutions needed for comprehensive R&D and for the transition of innovations from discovery to industry. As such, academic activities were incentivized towards supplying the indicative needs of the bioeconomy workforce and creating industry linkages for increased cooperation.
Nevertheless, the NBB had a strong emphasis on bioenergy. Coupled with the renewable fuel standard (RFS), established under the Energy Policy Act of 2005, it was able to facilitate the growth of biofuel production. The extension of the RFS under the Energy Independence and Security Act of 2007 brought higher prices for ethanol suppliers to incentivize higher production (McCarty and Sesmero, 2021). Figure 5 displays the production of biofuels in billions of gallons6. Biofuel production shows a gradual growth in the late 1990s and early 2000s, but following the implementation of the extended RFS, biofuel production experienced a massive expansion doubling its output levels by 2009. However, efforts to expand biofuel production were entirely streamlined in one industry, corn ethanol. Figure 6 shows the shares of various feedstocks used for biofuels for the period 2021 to October 20227. Although the biofuel industry had more than 15 years of experience with substantial government support, it remained mostly dependent on feedstock derived from corn.
These policies lay the foundation to be studied to guide the development of policies for a more comprehensive bioeconomy plan that puts greater emphasis on bioplastics. The outlook for bioplastics and the aim to replace conventional plastics requires exponential growth of the feedstock supply. Given the need to satisfy the RFS requirements with growing transportation demand, feedstock production capacity can become a binding constraint to bioplastic expansion. The experiences of the Biomass Crop Assistance Programme (BCAP) provide an essential pilot study to identify the economic factors surrounding the supply of biomass. The BCAP project was designed to transition agricultural operators and owners of non-industrial private forest land towards the production, and supply of biomass feedstocks through financial assistance. BCAP was implemented in two programs. Firstly, the project areas program aimed to incentivize biomass production with direct payments to establish energy crops and annual payments for any opportunity costs incurred by investing in energy crops (Endres, et al., 2010). The project was unable to motivate producers since the cost of transporting biomass to biofuel plants offset any potential gains from investing land and resources towards biomass crops (CRS, 2015). Plant operators also found that it was only economical to source biomass from suppliers within a 50-mile radius (Ekşioğlu, et al., 2009). Food waste biomass is particularly affected since they develop pathogens over long distances. The issue is alleviated by transporting in freezers; however, this increases the energy consumption and use of fossil fuels. (Bishop, et al., 2022). Alternatively, the recent acknowledgment of biomass as a source of both renewable energy and carbon neutrality allows the incorporation of forest biomass into government funding projects and tax relief schemes (H.R. 2617 - Consolidated Appropriations Act, 2023). Therefore, there is also potential to improve profitability through accounting for biomass as an environment and social governance (ESG) investment. The Trillion Trees Act (The Trillion Trees Act, 2023) was proposed to increase forest carbon stock and to provide market incentives to support bioplastics research and development.
The US Department of Agriculture initiated the Value-Added Producer Grants (VAPG) to encourage agricultural producers to transform their raw agricultural output to create broadly new products. The VAPG provided financial assistance to farmers, which provided the greatest impact on the fruits, nuts, and wine industries, and no significant impact on energy-related crops (Boland, et al., 2009). The USDA BioPreferred Program (BPP), first introduced by the Farm Bill of 2002, requires Federal agencies and contractors to purchase biobased products over others when available. It also increases public awareness through USDA-certified biobased product labeling (Pascoli, et al., 2022). This program provides demand-side support to both encourage production investments by securing markets for bioplastics and supporting successful marketing. However, a closer look at BPP category items shows great variation in the minimum required biobased content: packaging items at 25%, shopping, and trash bags at 22%, outdoor recreational items at 32%, plastic resins at 22%, plastic lumber at 23%, disposable Tableware 72%, plastic insulating foam 7%, etc. (USDA, 2022).
Finally, the September 2022 Executive Order geared toward advancing the US bioeconomy has outlined a strategy to consolidate and extend policy efforts in support of biotechnology and biomanufacturing (EOP, 2022). It expands existing support for biotechnology R&D, biobased procurement, and building a biomanufacturing ecosystem. In addition, it sets out plans for developing a more data-driven approach for measuring and centralizing information on the bioeconomy through its data initiative. Risks associated with the establishment of the bioeconomy are also targeted.
6 Discussion
Following the first step in the Stern (2004b) approach, the policy objective of our framework of policies, literature, and industry structures is to stimulate growth in bioplastic production and competitive substitution into the plastic supply chain. The causal linkages required for this to be accomplished is as follows. Firstly, each pillar of the bioplastic supply chain must be adequate to produce the outputs in their right quantities and qualities to meet the demands for vertical market linkages. Constructively, markets are centered around first satisfying their customer demand through the creation of desired value (Drucker, 1973) while balancing the supply-side activities to facilitate meeting those demands (Stank, et al., 2012). The same applies to the feedstock sub-industry that must meet their derived demand. Next, the successful integration of bioplastics requires higher production levels, sufficient supply of factors of production, investments into bioplastics and biomass production, and finally demand conditions necessary for material substitution. Moreover, the core driver for the bioplastics transition is its value of sustainability. Hence, the products to be supported are those that meet the criteria of facilitating decarbonization, reducing environmental and ecological loss, and being economically viable. The bioplastics supply chain was evaluated on these conditions and the gaps that require research and policy attention were highlighted.
At the outset, bioplastics have progressed into a well-established global industry that has been incorporated in many plastic-using sectors. From packaging to construction biobased plastics have been incorporated to varying degrees leading to the growing production of bioplastics. Biofuels have also laid a sufficient foundation for the entire bioeconomy, by connecting necessary supply chain linkages, coordinating investments, and facilitating national policy initiatives. The need to strengthen the supply of ethanol for biodiesel resulted in support programs like the BCAP, VAPG, and BPP. As such, suppliers of necessary crops and other sources of biomass were linked to biorefineries. Markets were further secured through fuel standards. The increased social awareness and demand for more sustainable action signals positive support to be expected from consumers and policymakers. However, bioplastic production remains only a fraction of the demand for plastics (Bari, 2022; Berdousis, 2023). The future of the industry and its ability to fully replace conventional plastics are at risk if the technical, economic, and sustainability issues are not adequately addressed.
Firstly, the social value of bioplastics outweighs its private value in its current state resulting in low investment attraction. Bioplastics have less environmental impact compared to conventional plastics (Spierling, et al., 2018; Atiwesh, et al., 2021) making it highly sought so sustainability purposes, but the industry is uncompetitive and lacks a plan to achieve that competitiveness (Wydra, 2019). Replacing conventional plastics with bioplastic hinges will require significant policy support to ensure its economic viability. From an investor’s perspective, the social interest forming climate-conscious consumers makes bioplastics marketable due to their biodegradability and role in potential GHG reduction. However, these remain niche markets given the cost competitiveness of conventional plastics (Rosenboom, et al., 2022). Nevertheless, the profitability of bioproducts can be improved by taking advantage of the green agenda which allows support for corporations that invest in activities that promote environmental and social governance (Singh, 2022). Accounting for bioplastics as an ESG investment allows cost reductions that can improve its competitiveness. Additionally, the benefits can be expanded upon given the potential for compounding environmental benefits throughout the supply chain. The carbon neutrality of some forms of biomass creates room for improved circularity and fossil fuel displacements that warrant institutional support. However, the development of these industry-specific policies requires further research on both technical and economic fronts, as well as a broader bioeconomy agenda.
Fortunately, the biofuel industry has developed the foundation for an expansive bioeconomy. Research in new sources of biomass and established supplier linkages have provided support for bioplastic production. Moreover, shocks in international oil prices provide incentives for investors to seek out fewer volatile inputs (Berdousis, 2023). The relative predictability of existing inputs and their prices may be able to mitigate some of the risks. However, investors are still faced with the uncertainty of industrializing technology that is still evolving and one that currently characterizes high cost, low technical substitutability of biodegradable materials, and supply constraints to upscaling. All things considered; the technology has grown substantially within the last two decades having over 900 published patents as of 2021.5 There is no consensus on the ideal source or sources of feedstock, though the more recent literature favors microalgae and lignocellulosic biomass. Complimentary research and development efforts are already underway for the development of the bioeconomy, particularly towards biofuels, and will continue to bridge the necessary gaps that hinder the industry’s expansion. Biomass constitutes a cross-cutting factor input into several emerging industries. Complementarities within the bioeconomy introduce the possibility of the co-products of multiple products, proving adequate incentives for investments and growth. Bioplastics can increase their competitiveness when combined with complementary policy actions to support biolubricants, aviation biofuel, and biofuel for road transport (Wydra, et al., 2021). Microalgae feedstock has significant gains through the co-production of biofuels and bioplastics (Beckstrom, et al., 2020). Bioplastics, biofuels, and biochemicals can be co-produced from the bacterium Halomonas bluephagenesis (Park, et al., 2023). Hence, such complementarities need to be investigated for their potential economies of scope with benefits not only limited to profitability but include carbon savings. Moreover, some biomass feedstock sources can be blended with conventional plastics as an intermediary step toward improving sustainability (Park and Lee, 2022). Such a proposal would be akin to the ethanol fuel blends and use of renewable fuel standards which has proved successful. The more similar the production activities and the greater degree of shared resources, the greater the possibilities of co-innovation in bioplastic production (Liliani, et al., 2020).
Biorefineries stand as a core component in the efforts to transform the state of the plastics industry. Their position in the supply chain allows successful integration and impact of circular economy principles, diversified feedstock sources, and other factors that support sustainability (Alibardi, et al., 2020). Hence, biorefineries should be one of the main targets for government intervention to support the plastic transition. Accordingly, biorefineries can become the target for the adoption of new technologies that improve the efficiency and sustainability of the industry. For instance, multiproduct operations can improve the energy intensity and water requirements in algae processing refineries (Subhadra and Edwards, 2011). Moreover, policy interventions can incentives traditional biorefineries to adopt new features such as the processing of biowaste (Alibardi, et al., 2020). Nonetheless, the design and planning of biorefineries require foreknowledge of the types of feedstocks being processed and polymers to be produced. Planning also must consider the refinery’s proximity to the feedstock suppliers becomes critical given the experience of agricultural waste but must consider the capacity constraints and constraints in the supply of feedstock resources (Patrik and Robert, 2009). Biorefineries require low-cost feedstock, and efficient technologies in order to supply competitive products to producers of plastics and plastic products. While economic and sustainability criteria can be met through varying combinations of co-production, the market structure can also facilitate economies of scale. Fewer numbers of large-capacity refineries can act as bulk buyers of raw materials which allows their cost of production and operational costs to be lowered (Patrik and Robert, 2009).
Addressing such shifts is reliant on the institutional and policy framework to improve investor confidence in the industry. Unfortunately, the current framework suffers from the absence of a clear approach and an outdated national strategy (PCAST, 2022). There is an absence of specific policies targeting bioplastics, but a sufficient starting point of related but uncoordinated policies. There is uncertainty in the ability of biomass supply to meet industry demands and still address biofuel and food needs. This includes the ability to secure a supply that remains both economic and sustainable. Low manufacturing capacity and high cost constrain product marketability, arguing the need for incentive and market-based policies to improve industry attractiveness (Hodgson, et al., 2022). The competitiveness and marketability of bioplastics would be supported through the inflow of investments and subsequent economies of scale in both feedstock and plastic production. Nevertheless, advancements in technology and industrialization methods are needed. As mentioned earlier, demand-side policies can follow that of the renewable energy standards which can secure markets and improve investment attractiveness.
Lastly, while the environmental sustainability of plastics rests heavily on the end-use processes for plastics, the complex and heterogeneous nature of plastics and plastic products requires the imposition of control and comparability through standards and regulations (Krzan, et al., 2006). While the ISO, CEN, and ASTM produce standards and guidelines for labeling and classification, the industry still lacks design guidelines that facilitate optimal recycling. Moreover, product-specific end-use guidelines can facilitate optimal material recovery and incentives are necessary to promote recycling and circularity (Watkins, et al., 2020). Cultural attitudes and behavior about the environment and recycling will also affect the sustainability of the industry since incorrect end-life treatment can lead to GHG emissions. The industry’s success hinges on the continuance of political, institutional, and societal support. Evidence suggests that support from the citizenry increase when persons are more engaged with the technology and can identify direct personal benefits and have reliable information on pros and cons from neutral sources (Lynch, et al., 2017).
The balancing act is exemplified by the substantial importance of the plastic industry to the US economy. Exports account for a third of the plastic industry’s revenues (Berdousis, 2023), and oil and gas revenues fund state, education, and local government spending (Newell and Raimi, 2018). Therefore, the support for bioplastics would depend on the extent to which it both displaces tax revenues and employment. State economies built around oil production such as Texas and California are consequently among the largest plastic-producing states. However, with rising oil prices exposing the nation’s vulnerability to shocks bioplastics are becoming more attractive. The new overarching objective is to attain energy security and reduced reliance on oil. Although bioplastics are far from the capacity needed to replace local petroplastics production, they can assist in weaning the nation off foreign oil imports allowing the preservation of local production while achieving energy security goals.
Conclusively, the systematic summary of this evaluation is illustrated in Figure 7.8 Improving the performance and competitiveness of the industry requires a cohesive development of both the supply and demand-side components. Notwithstanding these policy suggestions, some outstanding questions need to be addressed for the furtherance of bioplastics. Firstly, the expansion of bio-feedstock relies on its financial and environmental sustainability to hold when upscaled. Consequently, the technical, economic and policy needs to scale up production while maintaining viability are of high priority. The ability to blend feedstock sources can potentially alleviate the burden on any one source and limit its potential to infringe on its alternative uses. This is a technical issue with implications for the expansion of bioplastic supply, material substitution, drop-in potential, and the engineering of desirable features. Moreover, the extent to which biofuels and bioplastics are competing for resource inputs or potentially complementary ought to be examined given the risk of undermining one bioeconomy venture with another. Even so, the various combinations of co-production need to be extensively assessed. Central to creating investor confidence and market linkages, bioplastics must meet the quantitative and qualitative needs of the market. When this is guaranteed, policymakers will be more capable of executing bio-based standards in plastic products. Finally, the assessments made here were with the assumption that existing technology and markets will remain constant in the future. Forecasting and scenario analyses are required to assess the competitiveness under changing dynamics including the potential for oil price drops. Global trends of decarbonization can lead to falling demand for oil. The consequent falling oil prices can further improve the competitiveness of petroplastics, thus worsening the outlook for bioplastics. In this case, there will be an increasing need for government intervention in the industry. The policy gaps and research questions seen as having the greatest potential impact on the advancement of bioplastics are highlighted in Table 2.
7 Conclusion
This study outlays the supply chain of the US plastics industry and assesses the factors needed for the transition toward bioplastics. The sheer size of the plastics industry imposes the need for a gradual transition, though the pace of transition is constrained by the economic and environmental milestones needed to be met. The current state of technology and the capacity of bio feedstock supply apply negative pressures to investments, while volatile oil prices are increasing the industry’s attractiveness. Substantial research has been done on bioplastics substantial gaps remain regarding technical, economic, and supply chain issues. Existing policies either broadly address the bioeconomy at large or biofuels. The interconnectedness of bioeconomy has promising benefits and provides foundational work that can be built upon. Materializing meaningful transition of petroplastics requires an intentional and strategic approach to develop policies across each stage of the bioplastics supply chain. This requires an initial understanding of the feedstock needs, material blends, and capacity requirements. Existing legislation and national policies in support of bioeconomy development lay a sufficient foundation, however, bioplastics require industry-specific interventions. As such, this study highlights the need for a research agenda to fill the gaps necessary to ensure the sustainability of the industry and reduce the factors that deter investment. Of these gaps, the questions below were highlighted but do not represent an exhaustive list.
The discussions within this study are based on the available data and on the assumption that the existing trends economic and environmental trends will persist into the future. Firstly, quantifying the US plastics industry is difficult due to the lack of a single comprehensive database of plastics data, and the incoherence of measurement categories across available sources. Secondly, the need to intervene in the bioplastics industry will depend on the economic and political factors surrounding petroleum and petroleum-based products. A positive outlook would have improvements in technology facilitating a market-led growth of bioplastics. Additionally, improvements in sustainable agriculture can improve the environmental impact of bioplastics. Conversely, changes in the future projections of oil prices can make petroplastics even more competitive.
Finally, the furtherance of bioplastics requires future technical and economic research across all points of the supply chain. Promoting bio-feedstock relies on the sustainability at scaled economies, the potential for blending and co-production, and the extent to which the newly engineered features meet market demand. Conclusively, the competitiveness of bioplastics hinges on potential market outcomes, particularly the price of oil. As such, the success of decarbonization can paradoxically cause bio-based products to be less competitive since the reduction in oil consumption can also reduce oil prices. This underscores the need for extensive research on the technical performance of bioplastics, industrialization methods, supply chain integration, and the impact of exogenous factors. These factors have the potential to both advance and undermine the competitiveness of bioplastics.
Author contributions
All authors listed have made a substantial, direct, and intellectual contribution to the work and approved it for publication.
Funding
IG is a graduate research assistant funded under the National Science Foundation’s Established Program to Stimulate Competitive Research (EPSCoR) Renewable & Recyclable Polymers (R2P) Track 2, Award Number 2119754.
Conflict of interest
The authors declare that the research was conducted in the absence of any commercial or financial relationships that could be construed as a potential conflict of interest.
Publisher’s note
All claims expressed in this article are solely those of the authors and do not necessarily represent those of their affiliated organizations, or those of the publisher, the editors and the reviewers. Any product that may be evaluated in this article, or claim that may be made by its manufacturer, is not guaranteed or endorsed by the publisher.
Footnotes
1Federal Reserve Economic Data, Economic Research Division.
2Source: AM1831BASIC02 Annual Survey of Manufactures: Geographic Area Statistics: Summary Statistics for Industry Groups and Industries in the U.S. and States: 2018–2021.
3Source: Bureau of Economic Analysis—Use of Commodities by Industries - Summary.
4Source: American Chemistry Council; US Census Bureau; US International Trade Commission; ICIS; IHS; FEB; AFPM; TFI; Chlorine Institute; and Rubber Manufacturers Association.
5Source: Lens Patent Database—https://www.lens.org/.
6Source: US Energy Information Administration—Monthly Energy Review.
7Biofuels include fuel ethanol, biodiesel, renewable diesel, renewable heating oil, renewable jet fuel, renewable naphtha, renewable gasoline, biobutanol, and “other” biofuels and biointermediates.
8Source: Author’s depiction based on IBISWorld Industry Reports on Bioplastics (Bari, 2022) and Plastic & Resin (Berdousis, 2023) Manufacturing.
References
Abe, M. M., Martins, J. R., Sanvezzo, P. B., Macedo, J. V., Branciforti, M. C., Halley, P., et al. (2021). Advantages and disadvantages of bioplastics production from starch and lignocellulosic components. Polymers 13 (15), 2484. doi:10.3390/polym13152484
Alibardi, L., Astrup, T. F., Asunis, F., Clarke, W. P., De Gioannis, G., Dessì, P., et al. (2020). Organic waste biorefineries: Looking towards implementation. Waste Manag. 114 (1), 274–286. doi:10.1016/j.wasman.2020.07.010
Atiwesh, G., Mikhael, A., Parrish, C. C., Banoub, J., and Le, T. A. T. (2021). Environmental impact of bioplastic use: A review. Heliyon 7 (9), e07918. doi:10.1016/j.heliyon.2021.e07918
Bardhan, S. K., Gupta, S., Gorman, M. E., and Haider, M. A. (2015). Biorenewable chemicals: Feedstocks, technologies and the conflict with food production. Renew. Sustain. Energy Rev. 51, 506–520. doi:10.1016/j.rser.2015.06.013
Bari, S. A. (2022). Industry report Od4512 bioplastics manufacturing in the us S.L., Manhattan, New York, United States: IBISWorld.
Beckstrom, B. D., Wilson, M. H., Crocker, M., and Quinn, J. C. (2020). Bioplastic feedstock production from microalgae with fuel co-products: A techno-economic and life cycle impact assessment. Algal Res. 46, 101769. doi:10.1016/j.algal.2019.101769
Berdousis, D. (2023). INDUSTRY REPORT 32521 plastic & resin manufacturing in the US, s.l., Manhattan, New York, United States, IBISWorld.
Bishop, G., Styles, D., and Lens, P. N. (2021). Environmental performance of bioplastic packaging on fresh food produce: A consequential life cycle assessment. J. Clean. Prod. 317 (128377), 128377. doi:10.1016/j.jclepro.2021.128377
Bishop, G., Styles, D., and Lens, P. N. (2022). Land-use change and valorisation of feedstock side-streams determine the climate mitigation potential of bioplastics. Resour. Conservation Recycl. 180, 106185. doi:10.1016/j.resconrec.2022.106185
Boland, M. A., Crespi, J. M., and Oswald, D. (2009). An analysis of the 2002 Farm bill’s value-added producer Grants program. J. Agribus. 27 (1/2), 107–123. doi:10.22004/ag.econ.90660
Braun, E., and Wield, D. (1994). Regulation as a means for the social control of technology. Technol. Analysis Strategic Manag. 6 (3), 259–272. doi:10.1080/09537329408524171
BRDB (2008). National biofuels action plan. Washington, DC, USA: Biomass Research and Development Board.
Brizga, J., Hubacek, K., and Feng, K. (2020). The unintended side effects of bioplastics: Carbon, land, and water footprints. One Earth 3 (1), 45–53. doi:10.1016/j.oneear.2020.06.016
Burnham, M., Eaton, W., Selfa, T., Hinrichs, C., and Feldpausch-Parker, A. (2017). The politics of imaginaries and bioenergy sub-niches in the emerging Northeast U.S. bioenergy economy. Geoforum 82, 66–76. doi:10.1016/j.geoforum.2017.03.022
Cai, Z., Ye, F., Xie, Z., Zhang, L., and Cui, T. (2021). The choice of cooperation mode in the bioenergy supply chain with random biomass feedstock yield. J. Clean. Prod. 311 (127587), 127587. doi:10.1016/j.jclepro.2021.127587
CRS (2015). Report R4129, biomass crop assistance program (BCAP): Status and issues. Washington, DC, USA: Congressional Research Service.
Ekşioğlu, S. D., Acharya, A., Leightley, L. E., and Arora, S. (2009). Analyzing the design and management of biomass-to-biorefinery supply chain. Comput. Industrial Eng. 57 (4), 1342–1352. doi:10.1016/j.cie.2009.07.003
Endres, H.-J. (2017). “Bioplastics,” in Biorefineries. Advances in biochemical engineering/biotechnology. Editors K. Wagemann, and N. Tippkötter (Switzerland: Springer), 427–468. doi:10.1007/10_2016_75
Endres, J. M., Slating, T. A., and Miller, C. J. (2010). The biomass crop assistance program: Orchestrating the government's first significant step to incentivize biomass production for renewable energy. Environ. Law Report. 40 (1).
EOP (2022). E.O. 14081 of Sep 12, 2022, s.l. White House, Washington, D.C., USA: Executive Office of the President.
Escobar, N., Haddad, S., Börner, J., and Britz, W. (2018). Land use mediated GHG emissions and spillovers from increased consumption of bioplastics. Environ. Res. Lett. 13 (125005), 125005. doi:10.1088/1748-9326/aaeafb
European Bioplastics, (2022). Bioplastics facts and Figures, Berlin: European bioplastics. Available at: https://docs.european-bioplastics.org/publications/EUBP_Facts_and_figures.pdf.
European Bioplastics, (2023). Bioplastics - industry standards & labels: Relevant standards and labels for biobased and biodegradable plastics. Berlin, Germany: European Bioplastics.
European Commission (2004). Evaluating eu activities A practical guide for the commission service. Luxembourg: Office for Official Publications of the European Communities: Directorate-General for the Budget.
Foster, A. D., and Rosenzweig, M. R. (2010). Microeconomics of technology adoption. Annu. Rev. Econ. 2, 395–424. doi:10.1146/annurev.economics.102308.124433
Frisvold, G. B., Moss, S. M., Hodgson, A., and Maxon, M. E. (2021). Understanding the U.S. Bioeconomy: A new definition and landscape. Sustainability 13 (4), 1627. doi:10.3390/su13041627
Garrido, R., Cabeza, L. F., and Falguera, V. (2021). An overview of bioplastic research on its relation to national policies. Sustainability 13 (7848), 7848. doi:10.3390/su13147848
Gerassimidou, S., Martin, O. V., Diaz, G. Y. F., Wan, C., Komilis, D., and Iacovidou, E. (2023). Systematic evidence mapping to assess the sustainability of bioplastics derived from food waste: Do we know enough? Sustainability 15 (611), 611. doi:10.3390/su15010611
Ghodake, G. S., Shinde, S. K., Kadam, A. A., Saratale, R. G., Saratale, G. D., Kumar, M., et al. (2021). Review on biomass feedstocks, pyrolysis mechanism and physicochemical properties of biochar: State-of-the-art framework to speed up vision of circular bioeconomy. J. Clean. Prod. 297 (126645), 126645. doi:10.1016/j.jclepro.2021.126645
Gong, L., Passari, A. K., Yin, C., Kumar Thakur, V., Newbold, J., Clark, W., et al. (2023). Sustainable utilization of fruit and vegetable waste bioresources for bioplastics production. Crit. Rev. Biotechnol., 1–19. doi:10.1080/07388551.2022.2157241
Guo, R., Lv, S., Liao, T., Xi, F., Zhang, J., Zuo, X., et al. (2020). Classifying green technologies for sustainable innovation and investment. Resour. Conservation Recycl. 153, 104580. doi:10.1016/j.resconrec.2019.104580
Heng, X., and Zou, C. (2010). How can green technology Be possible. Asian Soc. Sci. 6 (5), 110–114. doi:10.5539/ASS.V6N5P110
Hodgson, A., Maxon, M. E., and Alper, J. (2022). The U.S. Bioeconomy: Charting a course for a resilient and competitive future. Ind. Biotechnol. 18 (3), 115–136. doi:10.1089/ind.2022.29283.aho
Horvat, D., Wydra, S., and Lerch, C. M. (2018). Modelling and simulating the dynamics of the European demand for bio-based plastics. Int. J. Simul. Model. 17 (3), 419–430. doi:10.2507/IJSIMM17(3)435
H.R. 2617 - Consolidated Appropriations Act, (2023). An Act Making consolidated appropriations for the fiscal year ending September 30, 2023, and for providing emergency assistance for the situation in Ukraine, and for other purposes. Washington, DC, USA: Consolidated Appropriations Act.
Izaguirre, J. K., Barañano, L., Castañón, S., Santos, J. A. L., Cesário, M. T., da Fonseca, M. M. R., et al. (2021). Economic and environmental assessment of bacterial poly(3-hydroxybutyrate) production from the organic fraction of municipal solid waste. Bioresour. Bioprocess. 8 (39), 39. doi:10.1186/s40643-021-00392-4
Jabeen, N., Majid, I., and Nayik, G. A. (2015). Bioplastics and food packaging: A review. Cogent Food & Agric. 1 (1117749), 1117749. doi:10.1080/23311932.2015.1117749
Kaczmarek, K., and Romaniuk, P. (2020). The use of evaluation methods for the overall assessment of health policy: Potential and limitations. Cost Eff. Resour. Allocation 18 (43), 43. doi:10.1186/s12962-020-00238-4
Karan, H., Funk, C., Grabert, M., Oey, M., and Hankamer, B. (2019). Green bioplastics as part of a circular bioeconomy. Trends Plant Sci. 24 (3), 237–249. doi:10.1016/j.tplants.2018.11.010
Krzan, A., Hemjinda, S., Miertus, S., Corti, A., and Chiellini, E. (2006). Standardization and certification in the area of environmentally degradable plastics. Polym. Degrad. Stab. 91 (12), 2819–2833. doi:10.1016/j.polymdegradstab.2006.04.034
Liliani, , Tjahjono, B., and Cao, D. (2020). Advancing bioplastic packaging products through co-innovation: A conceptual framework for supplier-customer collaboration. J. Clean. Prod. 252, 119861. doi:10.1016/j.jclepro.2019.119861
Liu, J., Wang, P., Wang, Y., Zhang, Y., Xu, T., Zhang, Y., et al. (2022). Negative effects of poly(butylene adipate-co-terephthalate) microplastics on Arabidopsis and its root-associated microbiome. J. Hazard. Material 437 (129294), 129294. doi:10.1016/j.jhazmat.2022.129294
Lynch, D. H., Klaassen, P., and Broerse, J. E. (2017). Unraveling Dutch citizens’ perceptions on the bio-based economy: The case of bioplastics, bio-jetfuels and small-scale bio-refineries. Industrial Crops Prod. 106, 130–137. doi:10.1016/j.indcrop.2016.10.035
Masi, D., Day, S., and Godsell, J. (2017). Supply chain configurations in the circular economy: A systematic literature review. Sustainability 9 (9), 1602. doi:10.3390/su9091602
McCarty, T., and Sesmero, J. (2021). Contracting for perennial energy crops and the cost-effectiveness of the biomass crop assistance program. Energy Policy 149, 112018. doi:10.1016/j.enpol.2020.112018
Milbrandt, A., Coney, K., Badgett, A., and Beckham, G. T. (2022). Quantification and evaluation of plastic waste in the United States. Resour. Conservation Recycl. 183, 106363. doi:10.1016/j.resconrec.2022.106363
Moshood, T. D., Nawanir, G., Mahmud, F., Mohamad, F., Ahmad, M. H., AbdulGhani, A., et al. (2022). Green product innovation: A means towards achieving global sustainable product within biodegradable plastic industry. J. Clean. Prod. 363, 132506. doi:10.1016/j.jclepro.2022.132506
Newell, R. G., and Raimi, D. (2018). US state and local oil and gas revenue sources and uses. Energy Policy 112, 12–18. doi:10.1016/j.enpol.2017.10.002
OECD (2013). Policies for bioplastics in the context of a bioeconomy. OECD science. Paris: OECD Publishing. doi:10.1787/5k3xpf9rrw6d-en
Park, H., Toogood, S. H., Chen, G.-Q., and Scrutton, N. S. (2023). Co-production of biofuel, bioplastics and biochemicals during extended fermentation of Halomonas bluephagenesis. Microb. Biotechnol. 16, 307–321. doi:10.1111/1751-7915.14158
Park, Y.-K., and Lee, J. (2022). Achievements in the production of bioplastics from microalgae. Phytochem. Rev. 22, 1147–1165. doi:10.1007/s11101-021-09788-8
Pascoli, D. U., Aui, A., Frank, J., Therasme, O., Dixon, K., Gustafson, R., et al. (2022). The US bioeconomy at the intersection of technology, policy, and education. Biofuels, Bioprod. Biorefining 16 (1), 9–26. doi:10.1002/bbb.2302
Patrik, S., and Robert, L. (2009). The development of forest-based biorefineries: Implications for market behavior and policy. For. Prod. J. 59 (1/2), 6–16.
Pcast, (2022). REPORT to the president biomanufacturing to advance the bioeconomy. Washington, D.C, USA: President’s Council of Advisors on Science and Technology.
Plastics, (2022). Size and impact: Executive summary, s.l.: Plastics industry association. Received from https://e.plasticsindustry.org/hubfs/Executive%20Summary_2022-Size%20%20Impact%20Report_9.13.pdf.
Popp, J., Lakner, Z., Rakos, M., and Fári, M. G. (2014). The effect of bioenergy expansion: Food, energy, and environment. Renew. Sustain. Energy Rev. 32, 559–578. doi:10.1016/j.rser.2014.01.056
Rhein, F. E. (2020). The dynamics of green innovation in B2B industries: A systems approach to explain the diffusion of bioplastics. Bensheim, Germany: Springer Nature. doi:10.1007/978-3-658-32148-2
Rosenboom, J.-G., Langer, R., and Traverso, G. (2022). Bioplastics for a circular economy. Nat. Rev. Mater. 7, 117–137. doi:10.1038/s41578-021-00407-8
Samer, M., Hijazi, O., Mohamed, B. A., Abdelsalam, E. M., Amer, M. A., Yacoub, I. H., et al. (2022). Environmental impact assessment of bioplastics production from agricultural crop residues. Clean Technol. Environ. Policy 24, 815–827. doi:10.1007/s10098-021-02145-5
Shafqat, A., Tahir, A., Mahmood, A., Tabinda, A. B., Yasar, A., and Pugazhendhi, A. (2020). A review on environmental significance carbon foot prints of starch based bio-plastic: A substitute of conventional plastics. Biocatal. Agric. Biotechnol. 27, 101540. doi:10.1016/j.bcab.2020.101540
Shen, L., Haufe, J., and Patel, M. K. (2009). Product overview and market projection of emerging bio-based plastics PRO-BIP 2009. Utrecht, Netherlands: Copernic Institute for Sustainable Development and Innovation - Utrecht University.
Shen, L., Worrell, E., and Patel, M. (2010). Present and future development in plastics from biomass. Biofuels, Bioprod. Bioref 4, 25–40. doi:10.1002/bbb.189
Shen, M., Huang, W., Chen, M., Song, B., Zeng, G., and Zhang, Y. (2020). (Micro)plastic crisis: Un-ignorable contribution to global greenhouse gas emissions and climate change. J. Clean. Prod. 254, 120138. doi:10.1016/j.jclepro.2020.120138
Sidek, I. S., Draman, S. F. S., Abdullah, S. R. S., and Anuar, N. (2019). Current development on bioplastics and its future prospects: An introductory review. i Tech. Mag. 1, 03–08. doi:10.26480/itechmag.01.2019.03.08
Singh, R. (2022). Defining sustainable chemistry. Ind. Biotechnol. 18 (5), 281–285. doi:10.1089/ind.2022.29288.rsi
Somleva, M. N., Snell, K. D., Beaulieu, J. J., Peoples, O. P., Garrison, B. R., and Patterson, N. A. (2008). Production of polyhydroxybutyrate in switchgrass, a value-added co-product in an important lignocellulosic biomass crop. Plant Biotechnol. J. 6 (7), 663–678. doi:10.1111/j.1467-7652.2008.00350.x
Spierling, S., Knüpffer, E., Behnsen, H., Mudersbach, M., Krieg, H., Springer, S., et al. (2018). Bio-based plastics - a review of environmental, social and economic impact assessments. J. Clean. Prod. 185 (1), 476–491. doi:10.1016/j.jclepro.2018.03.014
Stank, T. P., Esper, T. L., Crook, T. R., and Autry, C. W. (2012). Creating relevant value through demand and supply integration. J. Bus. Logist. 33 (2), 167–172. doi:10.1111/j.0000-0000.2012.01049.x
Stern, E. (2004b). “Philosophies and types of evaluation research,” in The foundations of evaluation and impact research. Third report on vocational training research in europe: Background report. Editors P. Descy, and M. Tessaring (Luxembourg: Office for Official Publications of the European Communities), 9–50.
Stern, E. (2004a). What shapes European evaluation? A personal reflection. Evaluation 10 (1), 7–15. doi:10.1177/1356389004044411
Suarez, A., Ford, E., Venditti, R., Kelley, S., Saloni, D., and Gonzalez, R. (2022). Rethinking the use of bio-based plastics to accelerate the decarbonization of our society. Resour. Conservation Recycl. 186, 106593. doi:10.1016/j.resconrec.2022.106593
Subhadra, B. G., and Edwards, M. (2011). Coproduct market analysis and water footprint of simulated commercial algal biorefineries. Appl. Energy 88 (10), 3515–3523. doi:10.1016/j.apenergy.2010.12.051
Thakur, S., Chaudhary, J., Sharma, B., Verma, A., Tamulevicius, S., and Thakur, V. K. (2018). Sustainability of bioplastics: Opportunities and challenges. Curr. Opin. Green Sustain. Chem. 13, 68–75. doi:10.1016/j.cogsc.2018.04.013
The Trillion Trees Act, (2023). H.R.2639 117th congress, introduced 04/19/2021. Washington, DC, USA: The Trillion Trees Act.
UNEP (2018). Mapping of global plastics value chain and plastics losses to the environment: With a particular focus on marine environment. Lyngby, Denmark: United Nations Environment Programme.
USDA (2022). BioPreferred. Available at: https://www.biopreferred.gov/BPResources/infographics/Categories_Infographic.pdf (Accessed March 11, 2023).
Wagemann, K., and Tippkötter, N. (2017). “Biorefineries: A short introduction,” in Biorefineries. Advances in biochemical engineering/biotechnology. Editors K. Wagemann, N. Tippkötter, K. Wagemann, and N. Tippkötter (Switzerland: Springer), 1–13. doi:10.1007/10_2016_75
Watkins, E., Romagnoli, V., Kirhensteine, I., Ruckley, F., Kreißig, J., Mitsios, A., et al. (2020). Support to the Circular Plastics Alliance in establishing a work plan to develop guidelines and standards on design-for recycling of plastic products. Luxembourg: Publications Office of the European Union.
Wydra, S., Hüsing, B., Köhler, J., Schwarz, A., Schirrmeister, E., and Voglhuber-Slavinsky, A. (2021). Transition to the bioeconomy - analysis and scenarios for selected niches. J. Clean. Prod. 294 (1), 126092. doi:10.1016/j.jclepro.2021.126092
Keywords: circular economy, plastics transition, bioeconomy, bioplastics, supply chain
Citation: Shah KU and Gangadeen I (2023) Integrating bioplastics into the US plastics supply chain: towards a policy research agenda for the bioplastic transition. Front. Environ. Sci. 11:1245846. doi: 10.3389/fenvs.2023.1245846
Received: 23 June 2023; Accepted: 20 September 2023;
Published: 16 October 2023.
Edited by:
May Massoud, American University of Beirut, LebanonReviewed by:
Manish Kumar, University of Palermo, ItalyMartin Koller, University of Graz, Austria
Fatimah Mahmud, Universiti Malaysia Pahang, Malaysia
Copyright © 2023 Shah and Gangadeen. This is an open-access article distributed under the terms of the Creative Commons Attribution License (CC BY). The use, distribution or reproduction in other forums is permitted, provided the original author(s) and the copyright owner(s) are credited and that the original publication in this journal is cited, in accordance with accepted academic practice. No use, distribution or reproduction is permitted which does not comply with these terms.
*Correspondence: Isaiah Gangadeen, aXNhaWFoZ0B1ZGVsLmVkdQ==