- 1Akvaplan-niva, Tromsø, Norway
- 2The Climate and Environmental Research Institute NILU, Tromsø, Norway
- 3Institute for Arctic and Marine Biology, UiT The Arctic University of Norway, Tromsø, Norway
- 4SINTEF Ocean, Trondheim, Norway
Car tire rubber constitutes one of the largest fractions of microplastics emissions to the environment. The two main emission sources are tire wear particles (TWPs) formed through abrasion during driving and runoff of crumb rubber (CR) granulate produced from end-of-life tires that is used as infill on artificial sports fields. Both tire wear particles and crumb rubber contain a complex mixture of metal and organic chemical additives, and exposure to both the particulate forms and their leachates can cause adverse effects in aquatic species. An understanding of the exposure pathways and mechanisms of toxicity are, however, scarce. While the most abundant metals and organic chemicals in car tire rubber have multiple other applications, para-phenylenediamines (PDs) are primarily used as rubber antioxidants and were recently shown to cause negative effects in aquatic organisms. The present study investigated the responses of the marine lumpfish (Cyclopterus lumpus) to crumb rubber exposure in a controlled feeding experiment. Juvenile fish were offered crumb rubber particles with their feed for 1 week, followed by 2 weeks of depuration. Crumb rubber particle ingestion occurred in >75% of exposed individuals, with a maximum of 84 particles observed in one specimen. Gastrointestinal tract retention times varied, with some organisms having no crumb rubber particles and others still containing up to 33 crumb rubber particles at the end of the experiment. Blood samples were analyzed for metals and organic chemicals, with ICP-MS analysis revealing there was no uptake of metals by the exposed fish. Interestingly, high resolution GC-MS analysis indicated that uptake of PDs into lumpfish blood was proportionate to the number of ingested CR particles. Three of the PDs found in blood were the same as those identified in the additive mixture Vulkanox3100. N-(1,3-dimethylbutyl)-N′-phenyl-p-phenylenediamine (6PPD) was the most concentrated PD in both the crumb rubber and lumpfish blood. The transformation product 6PPD-quinone was detected in the rubber material, but not in the blood. This study demonstrates that PDs are specific and bioavailable chemicals in car tire rubber that have the potential to serve as biomarkers of recent exposure to tire chemicals, where simple blood samples could be used to assess recent tire chemical exposure in vertebrates, including humans.
1 Introduction
The global tire market produced over 2.36 billion units in 2019, and was expected to grow at a rate of 3% over the next 5 years (Smithers, 2019). There are currently more than 1.45 billion vehicles on the roads globally (Hedges and Company, 2021), with an average annual emission of 0.95 kg TWP/capita, ranging between 0.23 and 4.7 kg TWP/capita/year (Kole et al., 2017). Unsurprisingly, the regional and local emission levels and distribution of TWPs is strongly related to the degree of urbanization and traffic levels (Leads and Weinstein, 2019; Knight et al., 2020; Goßmann et al., 2021). Per capita emissions by country range between 0.23 and 1.9 kg per year, with a maximum of 4.7 kg per year in the United States of America (Kole et al., 2017). Owing to their polymer-based composition, TWP are typically categorized as a specific form of microplastic pollution and are considered one of the major contributors to total microplastic emissions. For example, TWP have been estimated to constitute the largest fraction (42%) of all microplastic emissions in Norway (Sundt et al., 2020). Similar emissions can be expected from other countries with comparable per capita car use.
TWP are also thought to represent one of the main sources of marine microplastics (Boucher and Friot, 2017; UNEP, 2018), with transport pathways from the terrestrial environment to marine ecosystems including surface runoff, wastewater effluents and wind-driven air transfer (Furuseth et al., 2020; Parker-Jurd et al., 2021). TWP impact natural systems in two ways: as anthropogenic particles and through the leaching of rubber additives into aqueous media. Once present in the natural environment, empirical and modeling studies suggest TWP can be transported regionally via air and water, as well as to remote regions (Evangeliou et al., 2020; Adams et al., 2021; Järlskog et al., 2022).
In addition to TWP being emitted when driving cars and other vehicles, end-of life tires (ELT) are often downcycled to crumb rubber (CR), which is widely used in artificial surfaces, such as rubber-modified asphalt, playgrounds, and sport fields due to its low price and physical properties (Erman et al., 2013; Magnusson et al., 2016; WBCSD and Tire Industry Project, 2019). In the form of loose granulate, CR is widely used as infill on artificial grass pitches and horse tracks and is easily lost to the surrounding environment. An average size football field covered with artificial turf contains between 60 and 100 tonnes of CR. These fields need to be regularly re-filled with several tonnes of CR due to continuous losses through attachment to clothing, cleaning and maintenance practices, run-off and snow clearing. CR loss may be significant over the 15–20-year lifespan of an artificial turf sports field, with estimates suggesting this could amount to >80 tonnes of CR material per field (Krång et al., 2019; Bø et al., 2020). CR particles have been estimated to be the second largest source of microplastic emissions in Norway (30%), where the cold and wet climate has result in a large shift to artificial sports fields (Sundt et al., 2020). CR may represent a significant microplastic emission source in other countries with widespread use of such artificial sports fields.
Car tire rubber contains numerous chemicals, such as stabilizers, vulcanizing agents, softeners, and others (Kreider et al., 2010). Common stabilizers include substituted para-phenylenediamines (PDs), which are primarily used as antioxidants and antiozonants, and where N-(1,3-dimethylbutyl)-N′-phenyl-p-phenylenediamine (6PPD) is one of the most commonly used PDs (Erman et al., 2013; Zhao et al., 2023). This organic chemical additive recently attracted attention as the precursor to the oxidation/transformation product 6PPD-quinone, which was associated with mass mortality events of Coho salmon (Oncorhynchus kisutch) in the United States of America (Tian et al., 2020). Although PD antioxidants are used in a few rubber consumer products, they are mainly related to tire rubber (Zhao et al., 2023). PD antioxidants have been found in urban dust, in particulate matter <2.5 μm (PM2.5; Wang et al., 2022; Huang et al., 2021), as well as in snow (Seiwert et al., 2022), indicating the widespread presence of TWP and TWP-derived chemicals in the environment.
Regulations for the handling of waste/ELT tires that prohibit their disposal in landfills (Directive 1999/31/EC), as well as regulations that define content thresholds for certain harmful chemicals (e.g., polycyclic aromatic hydrocarbons (PAHs) in extender oils used during tire production; Directive 2005/69/EC), have been endorsed in the EU and elsewhere (Trudsø et al., 2022). For example, a threshold for PAHs in CR granulate being used as infill on sports fields in the EU was set already in 2006 (EC 1907/2006). However, regulations do not cover all vehicle tire ingredients, partly due to the specific formulations used by individual tire manufacturers being classified information from competitors and partly because transformation products are harder to identify, and risk assess. This has led to certain harmful car tire ingredients being identified only recently, as evidenced by the recent work focused on 6PPD and 6PPD-quinone (Zhao et al., 2023 and references therein). Regulatory work addressing PDs remains scarce, but the growing concern and evidence of toxicity have increased the interest for regulating this group of substances, especially 6PPD. The California Department of Toxic Substances Control (DTSC) has taken the initiative to regulate 6PPD emissions by including motor vehicle tires containing 6PPD on the Safer Consumer Products list of priority products, effective 1 October 2023. This requires that both foreign and domestic producers of tires containing 6PPD that will be sold in California have to make a priority product notification followed by submission of a notification of removal of the chemical or the product, replacement of the chemical or an alternative analysis report (DTSC and CalEpa, 2022). Such regulatory work is important, but little is still known about tire particle ingestion by terrestrial and aquatic species exposed to TWP or CR residues in the environment, and even less about the extent of uptake of potentially harmful additives from ingested particles.
The present work examined the ingestion, gut retention, and excretion of CR particles exposed to a marine fish, the lumpfish Cyclopterus lumpus, in a 21-day laboratory feeding and depuration experiment. The uptake of vehicle tire rubber metal and organic chemical additives was studied by ICP-MS and GC-MS analysis, using a combination of target and non-target screening approaches. In addition to measuring the presence of the more well-known additives, specific focus was placed on studying the uptake of 6PPD, other PD derivatives and the transformation product 6PPD-quinone. For the first time, we report the ingestion of CR by marine vertebrates under experimental conditions, as well as the concentrations of organic chemicals and metals in lumpfish blood prior, during and after CR exposure.
2 Materials and methods
2.1 Exposure of lumpfish to tire rubber particles
Laboratory-reared juvenile lumpfish (Cyclopterus lumpus) were acclimated for 24 h to the experimental conditions (9 °C and 99.9% O2) in six incubation tanks (240 L) containing natural seawater collected from the local fjord. Each tank received thirty 11-month-old fish (180 fish in total), with an average weight of 137 g and an average body length of 15 cm. Wet fish feed (PTAqua) was mixed with pre-used CR granulate of 3–5 mm diameter that was sourced from a local football club (Halsband et al., 2020; their sample ‘TOS’ from a sports field in Tromsø, Norway). The spiked fish feed had an average concentration of 57 CR particles or 0.36 g of CR, per Gram food. This mixture was continuously supplied to three of the experimental fish tanks (Supplementary Table S1) via a conveyor belt feeding system for 7 days, while the other three tanks served as controls and received uncontaminated feed in the same way (Supplementary Figures S1A, B). The 7-day exposure was followed by a 14-day depuration period, where all lumpfish were fed uncontaminated feed. On ten selected days during the incubation period, three fish were collected from each tank (Figure 1), anesthetized with a lethal dose of Finquel (1600 mg/L), weighed, and blood samples taken. Then, they were either immediately dissected for the GI-tract, or frozen at −20°C for later removal of organs. Both GI-tracts and blood were frozen at −20°C until isolation of CR particles and analysis of chemicals, respectively.
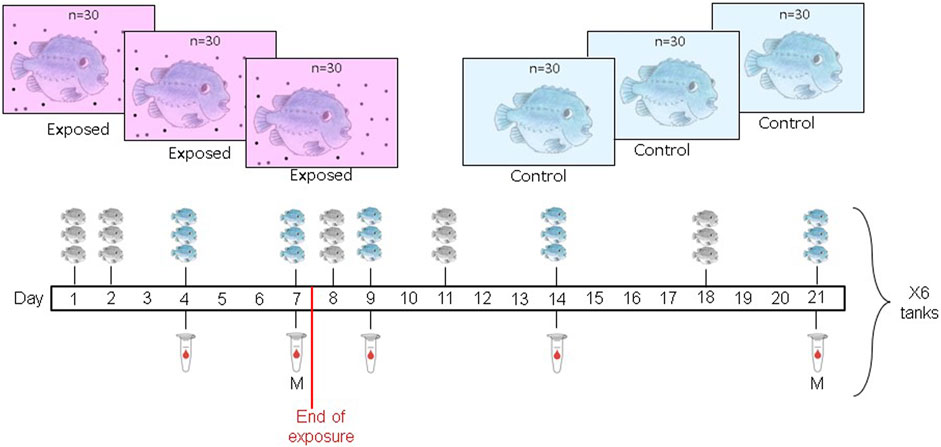
FIGURE 1. Experimental design of the lumpfish exposure. The fish (n = 180) were randomly allocated to six tanks (n = 30) divided in three exposed (pink) and three control (blue) tanks. Three fish per tank were dissected on 9 of the sampling days, of which 5 sample sets (blue) were analyzed for organic chemicals. On days 7 and 21, blood from 3 fish per tank/treatment were pooled for metal analysis (M).
2.2 Quantification of ingested crumb rubber particles
To count ingested CR particles, fish sampled on selected days during and after the exposure (Figure 1) were examined for their stomach and gut contents. GI-tracts were removed from the fish and opened with a scalpel. Stomach and gut contents were carefully dissected, rubber particles separated from the tissue and counted (Figure 2). The median number of CR particles present in the GI-tracts of exposed fish was determined per sampling day and tank.
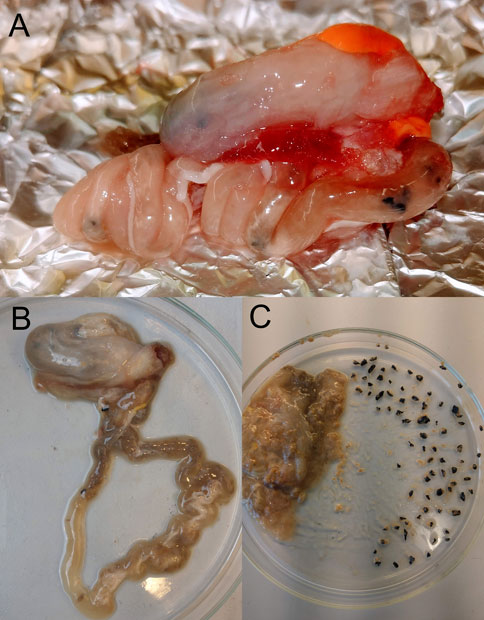
FIGURE 2. Gastrointestinal tract (GI-tract) of an exposed lumpfish. (A) Stomach, gut, and liver, (B) gut extended, and (C) opened with CR dissected.
2.3 Analysis of metals in lumpfish blood
Both CR and blood were analyzed using inductively coupled plasma mass spectroscopy (Agilent 7700x ICP-MS). The method for metal determination as well as metal concentrations in the CR material have previously been reported (Halsband et al., 2020). Lumpfish blood samples (n = 36) for both exposed (tanks 2, 4 and 6) and non-exposed (tanks 1, 3 and 5) fish were pooled per tank and day (3 fish/tank/day) for days 7 (last day of exposure) and 21 (last day of depuration) to give a total of 12 blood samples that amounted to a total sample mass of 1 g each. The blood was transferred to acid cleaned plastic tubes (15 mL) and the concentration of ten different metals known to be present in car tires was determined: Chromium (Cr), Manganese (Mn), Iron (Fe), Cobalt (Co), Nickel (Ni), Copper (Cu), Zink (Zn), Cadmium (Cd), Antimony (Sb), and Lead (Pb).
2.4 Organic chemicals in crumb rubber granulate and a commercial antiozonant mixture
A 20 mg sample of the same CR granulate used for the feeding study was extracted with 2 mL of ethylacetate for 24 h at room temperature. After filtration to remove the particulate material, subsamples were analyzed by gas chromatography-mass spectrometry (Q Exactive GC equipped with Trace 1310 Gas chromatograph, TriPlus RSH autosampler and TG-5SILMS column (30m, 0.25 mm i.d., film thickness—0.25 μm). The injector was operated in splitless mode at 280°C. The constant flow was set at 1.2 mL/min. The following temperature program was applied: 60°C for 2 min, increased by 20°C/min to 300°C, held for 8 min. Transfer lines were held at 280°C, the ion source at 250°C. The mass-spectrometer operated at 40 eV ionization energy in full-scan mode and the selected range of the mass to charge ratio (m/z) was 50–750. The mass resolution was set at 120000.
The same analytical chemical procedure was applied to a specimen of the commercially available PD antiozonant mixture Vulkanox 3100 (Alfa Chemistry, Cat. No. A-ACM-220830202612, Lot no. ALN6876), referred to hereafter as Vulkanox. The substance was dissolved in ethylacetate, and the stock solution diluted to 0.5 ng/mL prior to analysis. Analyses of the CR granulate extracts and the Vulkanox mixture were then used to identify CR-related chemicals that are not commercially available as standards, except for 6PPD (Fluorochem, Cat. No. 216709) and its degradation product 6PPD-quninone (ASCA GmbH, Cat. No. 10243). All other compounds are reported at semi-quantitative concentrations.
2.5 Analysis of organic chemicals in lumpfish blood
For extraction of organic chemicals blood samples (0.5 g) were mixed with 1.5 mL of hexane and 0.5 mL of acetonitrile. At the beginning of the extraction, 20 ng of an internal standard (6PPD-Quinone-D5; HPC GmbH, Cat. No. 688151, Lot no. 801289) was added as 50 μL of solution in acetonitrile at a concentration of 0.4 ng/μL. The samples were briefly vortexed prior to 30 min of sonication, the vortex-sonication cycle was repeated, followed by shaking in a horizontal position for 16 h. Finally, the blood samples were centrifuged at 1500 rpm for 10 min. A portion (100 μL) of the upper hexane layer was transferred to GC vials containing an insert and a recovery standard was added (20 μL of 6PPD-quinone-13C6 (CIL, inc., cat. no. CLM-12293-1.2) at 0.1 ng/μL prior to analysis by high-resolution GC-MS (HRGC/HRMS, Thermo Fisher Orbitrap). The laboratory blanks (0.25 mL Milli-Q water) were processed in the same way. For conducting an initial non-target screening, the full scan GC-MS method was identical to that used for the analysis of CR extract and Vulkanox samples described above. For the targeted analysis of five identified marker compounds, the method was optimized with a narrower mass range (180–330 m/z) to increase the probability of detection.
2.6 Quantification of 6PPD and other characteristic compounds in blood
The raw GC-MS data files were processed with the Software package Chromeleon 7 (Thermo Scientific). A single-point calibration was used for 6PPD, and the calculation of 6PPD concentrations in the blood samples was based on the relative response of the analyte to that of the isotope-labeled internal standard in a sample and in the calibration solution, respectively. Other organic chemicals, for which there were no available authentic standards at the time of analysis, were semi-quantified based on the assumption of an equal response to that of 6PPD. Thus, the true concentrations of the other organic chemicals present in the blood samples might be different from the ones presented due to their respective relative response factors. The instrumental limit of detection (LOD) for 6PPD and a selected number of identified compounds was determined using a serial dilution of reference solutions.
2.7 Statistical analyses
Shapiro-Wilks tests of normality showed that the data for length and weight of fish were normally distributed, while the data for number of CR found in the GI-tract and the concentrations of metals and organic chemicals in blood were not normally distributed. We thus applied parametric tests for the statistical analyses of body size (length and weight data) and non-parametric tests for the statistical analyses for the number of CR found in the GI-tract and the concentrations of metals and organic chemicals in blood. Statistical differences in the body size (length and weight) of fish between treatments were determined using t-tests and comparisons between tanks were determined using one-way ANOVA. Statistical differences in the number of CR particles in the GI-tract between sampling days and incubation tanks were determined with Kruskal–Wallis tests, followed by Dunn’s post hoc test corrected with Holm-Bonferroni were significant. Statistical differences in the concentrations of metals in blood between treatments and days were determined by applying Mann-Whitney U tests. Statistical differences in the concentrations of organic chemicals in blood between treatments were determined with the Mann-Whitney U test. Statistical differences in the concentration of organic chemicals in blood between days were determined using Kruskal–Wallis’s test, followed by Dunn’s post hoc test corrected with Holm-Bonferroni when significant. Correlations between the number of ingested CR particles and the concentrations of organic chemicals in blood and between body size and 1) the number of ingested CR, and 2) concentrations of organic chemicals in blood were determined with linear regression. All data analyses and visualizations were performed with RStudio (version 2022.07.1, R Core Team, 2022).
3 Results
3.1 Ingestion and egestion of car tire particles
The fish were randomly distributed to the tanks (Supplementary Figure S2). There was no statistical difference in length between exposed (15 ± 0.75 cm) and non-exposed fish (15 ± 0.94 cm; t-test, p-value >0.05), but there was a statistical difference in weight between non-exposed fish (143 ± 27 g) and exposed fish (132 ± 23 g; t-test, p-value <0.05). This had, however, no effect on statistical differences in length or weight between tanks (One-way ANOVA, p-value >0.05). All incubated fish survived the experimental period, i.e., no mortality was recorded. In the non-exposed fish serving as control groups (tanks 1, 3 and 5), no CR particles were found in their GI-tracts. Of the exposed fish (n = 90), a majority of 76% (69 fish) had CR particles in their GI-tract at the time of sampling, while the remaining 21 exposed fish did not. The amount of ingested CR varied significantly among individuals and within sampling days (Figure 3), but without any clear relationship to their body size (Supplementary Figure S3). A temporal trend in the abundance of CR particles present in the lumpfish GI-tracts was observed. CR was ingested from the first day of the experiment, with the median CR particle numbers increasing to a maximum of 28 particles per fish on day 8, at the beginning of the depuration phase. This was followed by a decrease from day 9–18, with a slight increase again on day 21 (Figure 3). The maximum number of CR particles present in an individual fish was recorded on day 9, with 84 CR particles (Figure 3). On the last day of the depuration phase (day 21), one lumpfish contained 33 particles, five individuals contained 10 particles or less, and the remaining three fish contained no observable CR.
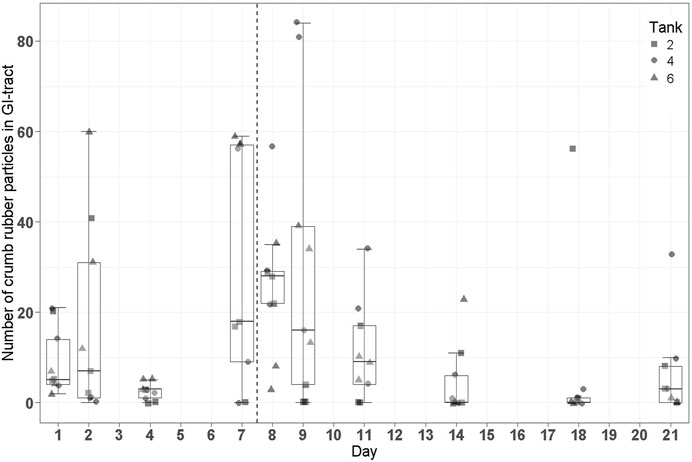
FIGURE 3. Number of CR particles (boxplots denote minimum, maximum, median, and upper/lower quartiles) in the GI-tract of exposed fish over time. The dotted line marks the end of the exposure.
3.2 Metals in blood
The metal concentrations in blood of individual fish varied widely. Fe was found in the highest concentrations (>100 mg/kg), followed by Zn concentrations between 6–12 mg/kg (ca. 10 time lower than Fe), and then Cu and Mn with concentrations between 0.5 and 1 mg/kg (Figure 4). Cr, Ni, Pb and Co had intermediate concentrations around 0.2 mg/kg, while the concentrations of the trace metals Cd and Sb were two to three orders of magnitude lower than those for the other metals (Figure 4). No significant differences were observed in the metal concentrations between controls and exposed fish, or between time points (days 7 and 21; Mann-Whitney U test, p > 0.05). The only exception was Cr, which had a higher concentration on day 7 than on day 21 in both controls and exposed fish (Mann-Whitney U-test, p < 0.05). Although the median concentrations of Cr, Fe, Co, Ni, and Cu (day 7), as well as Zn; days 7 and 21), were higher in exposed fish compared to controls, the differences were not significant (Figure 4). The opposite was found for Mn (days 7 and 21), as well as Fe, Cu and Pb (day 21), where the concentrations were higher in controls than exposed fish, although not significantly.
3.3 Organic chemicals in CR, the antiozonant mixture and blood
The CR granulate used in the current study has previously been analyzed by non-target GC-MS and found to contain a large number of organic chemicals (Halsband et al., 2020). Here, we focused on compounds that are suspected to be both bioavailable and cause toxicity, e.g., benzothiazole (Li et al., 2010; Halsband et al., 2020), 6PPD and its transformation product 6PPD-quinone (Tian et al., 2020), as well as example compounds that were detected in both CR and blood in the present study (Table 1). A comparison of the chromatograms corresponding to the solvent extracts from the lumpfish blood samples (Supplementary Figure S4) with the chromatogram of CR solvent extract (Supplementary Figure S5A) informed the search for and tentative identification of several phenylenediamines (PDs) present in both sample types. The identification of 3 PDs, diphenylphenylenediamine (DPPD), ditolylphenylenediamine (DTPD; C20H20N2), and tolylphenylphenylenediamine (TPPD; C19H18N2), was subsequently confirmed through later analysis of the commercially available Vulkanox mixture (Supplementary Figure S5B). In addition, two structurally unidentified PD chemicals with the chemical formulae C21H26N2 and C23H26N2 were detected in both the blood and CR (Table 1). The compound cyclohexylphenylphenylenediamine (CPPD), a constituent of some tire rubber materials (Galtung, 2023), was not found in any of the samples. High resolution mass-spectra of the five PD chemicals identified in both CR and lumpfish blood (6PPD, DTPD, TPPD, C21H26N2 and C23H26N2; Supplementary Figures S6–S10) agreed with the elemental composition and other literature data available for these compounds. It is important to note that the determined concentrations of the PD additives (except 6PPD-quinone) are only semi-quantitative and the calculated concentrations therefore represent approximate orders of magnitude, rather than true absolute concentrations (see Material and Methods). This reflects the uncertainty with respect to their extraction efficiency and the level of equivalence with the internal standard (6PPD-quinone-D5). The instrumental detection limits for 6PPD, 6PPD-quinone, DTPD, TPPD, DPPD, C21H26N2 and C23H26N2 were 0.1 pg, 0.5 pg, 0.05 pg, 0.05 pg, 0.05 pg, 0.1 pg, and 0.05 pg, respectively.
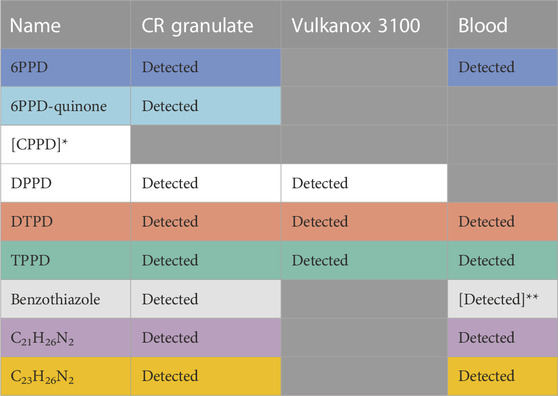
TABLE 1. Organic chemicals detected in CR by suspect screening, in the anti-ozonant Vulkanox, and in lumpfish blood. *CPPD (Cyclohexylphenylphenylenediamine) was not detected in any of our samples, although it is a constituent in some tire rubbers. **Benzothiazole was detected in blood, but without significant difference in concentrations between exposed and non-exposed fish.
While the CR samples were found to contain all three of the target chemicals (benzothiazole, 6PPD and 6PPD-quinone), only 6PPD and benzothiazole were subsequently detected in the lumpfish blood samples (Table 1). As 6PPD-quinone was detected in low concentrations in the CR, its absence in the blood samples may reflect it being below the instrumental LOD. Similarly, DPPD, DTPD, TPPD, C21H26N2 and C23H26N2 were found in the CR, but only DTPD, TPPD, C21H26N2 and C23H26N2 were found in the lumpfish blood samples. The five PDs detected in both CR and blood (6PPD, DTPD, TPPD, C21H26N2 and C23H26N2; Table 1) had significantly elevated concentrations in exposed lumpfish compared to control organisms (Mann-Whitney U-test, p < 0.05; Figure 5). The highest concentrations were recorded for 6PPD, which exhibited a maximum concentration of 1206 pg/g blood in one individual on day 9 (Figure 5A). This was followed by C21H26N2 and DTPD with up to 798 pg/g on days 4 and 9, as well as on day 7, respectively (Figures 5B,C). The compound C23H26N2 showed a lower average concentration of 111 ± 118 pg/g, with one fish peaking at 428 pg/g on day 7 (Figure 5D). Low concentrations, but still above the LOD, were observed for TPPD, with a maximum of 115 pg/g on day 7 (Figure 5E). Interestingly, two temporal patterns emerged. In the first trend, the two additives with highest maximum concentrations (6PPD and C21H26N2) increased from day 4 to day 7, peaked on day 9, decreased again by day 14, and then decreased further by day 21. Both chemicals still exhibited quite high concentrations on day 14, with one fish containing 1176 pg/g of 6PPD. In the second trend, the three other compounds (DTPD, TPPD and C23H26N2) had peaked already on day 7, observed to be decreasing by day 9, and already below the LOD by day 14 (Figures 5C–E). It should be noted that TPPD exhibited both trend types, with differences between individual fish (Figure 5E). DTPD concentrations were slightly elevated on the last day of depuration (day 21) compared with the low concentrations on day 14 (Figure 5C). Three of the chemicals, 6PPD, DTPD and C21H26N2, were still detected in some individuals on day 21 in various combinations, with 6PPD detected the most frequently (6 fish).
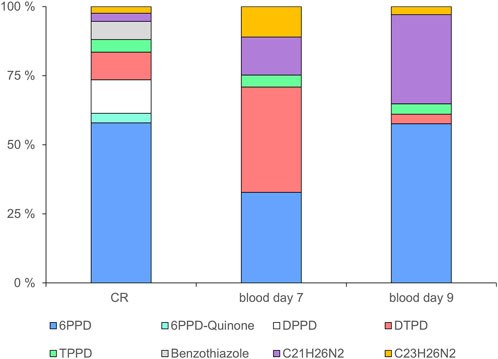
FIGURE 5. Relative composition of organic additives in CR and fish blood after 7 days of exposure (day 7) and after 2 days of depuration (day 9).
The PD concentrations in lumpfish blood were four to six orders of magnitude lower (tens to hundreds of pg/g) than those in the CR particles themselves (10–177 μg/g). Furthermore, the five PD additives detected in blood were in different relative proportions compared to their relative proportions in the CR (Figure 6). While 6PPD was the PD present at the highest concentration in both the CR and blood, no DPPD was detected in blood, even though it was the second most abundant PD in CR (Figure 6). In contrast, DTPD and one of the unidentified chemicals (C23H26N2) were proportionally more concentrated in the lumpfish blood relative to the other PDs compared to their relative contribution to the PD composition in the CR (Figure 6). The time points chosen for blood sampling showed that the PDs were taken up and processed in the blood within the time scale of a few days; the unidentified compound C21H26N2 was measured in higher concentrations later in the depuration phase (day 9) than, e.g., DTPD and C23H26N2 (day 7), similar to 6PPD and TPPD (Figure 6).
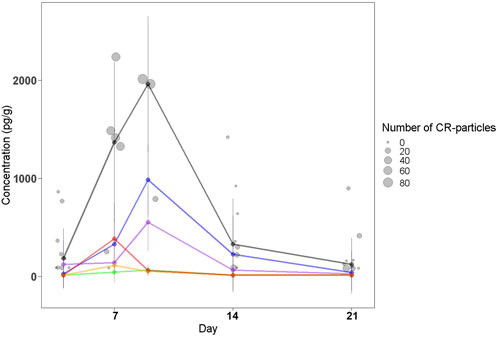
FIGURE 6. Concentrations of organic chemicals measured in lumpfish blood over time (mean concentration per day and fish). Blue = 6PPD, purple = C21H26N2, red = DTPD, yellow = C23H26N2, green = TPPD, and black = cumulative concentration and the number of CR particles present in the guts of individual fish (circles).
The concentrations of the individual CR additives present in the lumpfish blood were significantly positively correlated with the number of CR particles present in the GI-tract, with R2 values ranging from 0.4 for 6PPD, DTPD, C21H26N2 and C23H26N2 to 0.8 for TPPD (Supplementary Figure S11). Figure 7 shows the average body burden of additives together with the number of CR particles in GI-tracts of individual fish over time, and the cumulative median concentrations of the additives in blood for all exposed fish on a given sampling day. A clear temporal pattern of additive uptake into and elimination from the blood is evident, as well as differing contributions of the 5 PDs to additive body burden, where 6PPD had the highest peak around 1000 pg/g, followed by C21H26N2 and DTPD with around half that concentration, and much lower concentrations of C23H26N2 and TPPD (Figure 7). PD concentrations in blood were unrelated to body length or weight (Supplementary Figure S12).
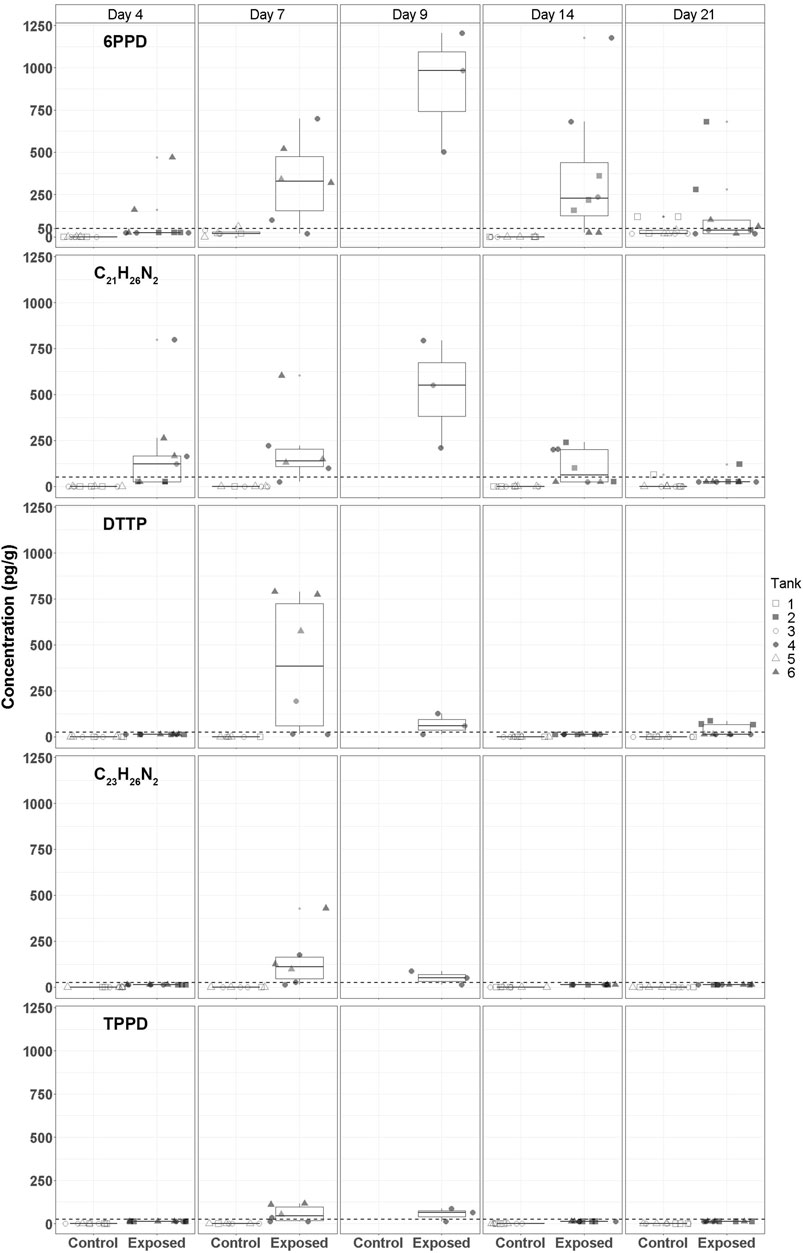
FIGURE 7. Concentrations of CR-related chemicals in lumpfish blood. Tanks 1, 3, and 5 were non-exposed controls, tanks 2, 4 and 6 were exposed. The limit of detection (LOD), indicated by the dashed line, was 50 pg for 6PPD and C21H26N2 and 25 pg for DTPD, TPPD and C23H26N2, respectively.
4 Discussion
4.1 Ingestion of CR particles
The ingestion of CR particles is not restricted to caged fish, but has also been shown experimentally for the marine mummichog (Fundulus heteroclitus; LaPlaca and Hurk, 2020), the freshwater fathead minnow (Pimephales promelas; LaPlaca et al., 2022) and goldfish (Carassius auratus; Chiba et al., 2023). Furthermore, five estuarine fish species collected from the wild were found to contain ‘suspected tire wear particles’ (Parker et al., 2020), indicating that ingestion of car tire rubber particles (TWP or CR) also occurs in natural habitats. The current study contributes to the increasing evidence that marine fish species have the potential to ingest CR particles, as evidenced herein for laboratory-reared juvenile lumpfish Cyclopterus lumpus. When the CR was offered to the lumpfish together with feed, three-quarters of the exposed fish ingested the CR particles, with >80 particles recorded in two individuals (Figure 2C). It should be noted that prior conditioning of the fish to regular feeding of food pellets supplied at the surface of the tanks may have increased their motivation to ingest floating particles compared to wild lumpfish feeding within their natural habitat. As a result, the absolute numbers of ingested CR particles may be higher than expected under natural conditions, especially when environmentally relevant exposure concentrations are considered. Although it cannot be ruled out that any natural ability to select against non-food items may have been reduced in the laboratory-reared lumpfish compared to their wild counterparts, the non-selective feeding observed in this study is consistent with the generally opportunistic feeding behavior previously ascribed to this species (Vandendriessche et al., 2007; Imsland et al., 2015). It is therefore suggested that wild lumpfish have the potential to ingest CR particles in the natural environment. This is supported by the observations of Parker et al. (2020), where ingestion of TWP and other microplastics was higher in species with a non-selective feeding strategy than in selective feeders.
As with many particle ingestion studies, the reported CR particle abundances in lumpfish GI-tracts represent snapshots at the time of sampling, as the fish were sacrificed after sampling and their feeding rates not examined over time. The resulting data therefore provide an insight into the general temporal trend for the whole group of exposed fish during the 1-week exposure and subsequent 2-week depuration phase rather than absolute values of ingestion. It is also important to note that CR particles ingested early during the exposure may have been egested already at the time of sampling, leading to an underestimation in ingestion. In contrast, CR particles found to be present in the GI-tract during the depuration phase may be (I) re-ingested particles that have previously been ingested and egested by other lumpfish, or (II) ingested CR particles that remained in the exposure tank that were not previously ingested or egested. Both scenarios could lead to overestimation of CR ingestion during the depuration phase. In contrast to the previous study on goldfish (Chiba et al., 2023) where the TWPs were egested within 48 h, CR particles were found to remain in the GI-tracts of some lumpfish for many days after the end of exposure (up to 14 days post exposure). If these CR particles were ingested sometime during the actual exposure period (days 1–7), it would indicate gut passage/retention times of several days or even weeks. In this case, the period available for car tire rubber additives to leach within the GI-tract and be transported to other organs and tissues would have been much longer than 48 h.
The high individual variability in the amount of CR ingested (range from 0 to 84 particles), may also be explained by inherent variations in the ‘personality’ of individual fish, where ‘curious’ and ‘brave’ fish ingest particles earlier and in larger quantities than more ‘shy’ or ‘timid’ fish (Chen et al., 2022). Furthermore, many studies assessing the presence of microplastic particles in populations of wild caught fish have also reported such large variations between individuals collected from the same location, shoal and time point, suggesting this is not specific to lumpfish (Wootton et al., 2021). It should also be noted that about a quarter of all fish had no CR in their GI-tract at the time of sampling. Although it cannot be determined for each individual lumpfish if this reflects selection against non-nutritious particles, fish personality, or egestion before sampling, some of the lumpfish examined for organic chemicals were found to have high blood concentrations of 6PPD and C21H26N2 on day 14, indicating that the additives were still present in the blood post-egestion (Supplementary Figures S10A, B). This type of temporal mismatch explains the relatively low R2 values for the linear regressions of CR particle presence in the GI-tract versus PD concentration in blood for these two compounds (Supplementary Figure S11).
4.2 Bioavailability and uptake of metals from car tire rubber
The CR granulate used for this study has previously been shown to contain ten different metals, where Zn was the main contributor (22.6 g/kg), followed by Fe and Cu (Halsband et al., 2020). Similar metal profiles and relative concentrations have been reported in other studies on car tire rubber chemical composition (Wagner et al., 2018, and references therein). The concentrations and relative proportions of metals can vary among tire materials, being influenced by factors such as different manufacturer formulations, size, age, and degree of weathering (Kreider et al., 2010; Wagner et al., 2018; Halsband et al., 2020). Zn and other metals have a high capacity to leach from CR materials into aqueous surroundings (Bocca et al., 2009; Halsband et al., 2020), suggesting that they may also become bioavailable when leaching into the GI-tract occurs upon ingestion of car tire particles. The most prominent metal in our blood samples was Fe, which is most likely to reflect its physiological function in oxygen transport by hemoglobin rather than uptake from CR particles. Although Zn was the second most abundant metal in lumpfish blood after Fe, there was no statistical difference in Zn levels between exposed and non-exposed fish, indicating that it was either not bioavailable or that uptake was regulated by the fish. This is not surprising, as Zn has many biological functions and plays a role in intercellular signaling, where Zn sequestration is controlled by Zn transporter proteins (Kambe et al., 2015). The same is true for the trace metals Cu and Mn, which have essential biological roles in energy metabolism and immune response (Chandrapalan and Knwong, 2021). The remaining metals measured in the current study exhibited low concentrations in lumpfish blood and no differences between exposed and non-exposed fish. Again, this indicates that they were not taken up from the CR, or that they were quickly detoxified and/or transferred from blood to other tissues, e.g., the liver.
4.3 Bioavailability and uptake of organic chemicals from car tire rubber
Organic additives typically represent between 5%–10% of the weight of vehicle tires (Wagner et al., 2018). The most abundant of organic chemicals and groups of organic chemicals reported in car tire rubber are benzothiazole, polycyclic aromatic hydrocarbons (PAHs), phthalates and several bisphenols (Rogge et al., 1993; Reddy and Quinn, 1997; Kreider et al., 2010; Halsband et al., 2020). Of these, benzothiazole was present in the highest amounts, with 110 ± 28 mg/kg in the CR material used here (Halsband et al., 2020, their Supplementary Table S2). Although benzothiazole was detected in the lumpfish blood, it was not found to be at significantly elevated concentrations in the exposed group (not shown) compared to the control group. It is therefore suggested that any potential uptake of benzothiazole from the CR was masked by other environmental sources of benzothiazole that the control fish were also subjected to, and that the concentrations measured represent background contamination rather than exposure to CR. Total PAH content of the rubber was around 50 mg/kg, but only small amounts leached into seawater (Halsband et al., 2020), and we thus did not consider PAHs further in this study. Owing to their hydrophobic properties and proven accumulation potential, target analysis of PAHs in organs such as the liver may have provided more information. The same applies to phthalates and bisphenols, which had a cumulative concentration of 36 mg/kg and 2.3 mg/kg in the CR material, respectively (Halsband et al., 2020).
The chemical mixtures 1,4-Benzenediamine, N,N′-mixed phenyl and tolyl derivatives (BENPAT, CAS: 68953-84–4) also known as Vulkanox 3100, and 1,4-Benzenediamine, N,N′-mixed tolyl and xylyl derivatives (BENTAX, CAS: 68478-45–5) are used as antiozonants in the manufacture of tires (Environment Canada and Health Canada, 2011). The antiozonant 6PPD has been reported to represent 1%–2% to the typical organic additive load of tires (DTSC and CalEPA, 2022). Concentrations of 1.5 and 1.9 ng 6PPD per mg dry weight were reported for two samples of road dust, suggesting it is already widespread in the natural environment (Klöckner et al., 2021). In line with our results, both 6PPD and DTPD have been found in TWP and CR, together with the oxidative transformation product of 6PPD, 6PPD-quinone (Zhao et al., 2023). The oxidation product 6PPD-quinone was recently identified as the cause of mass mortality events in the salmon species Oncorhynchus kisutch (Coho salmon) in the United States of America (Tian et al., 2020). Antiozonants, especially 6PPD and 6PPD-quinone transformation product, were therefore of particular interest in this study and were quantified with the help of additional GC-MS screening of the CR material together with commercially available standards for 6PPD-quinone. While 6PPD and 6PPD-quinone were both detected in the CR material, ranking first and fifth in concentration among the additives considered (Figure 7), the lumpfish blood samples did not exhibit any detectable presence of 6PPD-quinone. This suggests that 6PPD-quinone uptake into lumpfish blood is limited.
Although analysis of the Vulkanox 3100 mixture confirmed the presence of DPPD, DTPD and TPPD in the CR material exposed to the lumpfish, only TPPD and DTPD were found in the blood of exposed fish (Figure 7). This suggests that there are differences in the molecular structure of these three related PD chemicals that have strong impact on their uptake into lumpfish blood. Although further studies would be needed to verify the uptake and impacts of the three different chemicals, these preliminary findings indicate that using DPPD as a lone antiozonant additive in car tires could reduce exposure and uptake of PDs in marine fish, thus reducing the risks associated with car tires and their particulate emissions. We also report for the first time two structurally unidentified diamines in tire rubber particles, C21H26N2 and C23H26N2, which appear to be other PD analogues. As these two chemicals were not present in the Vulkanox 3100 standard mixture used in the study, it was not possible to confirm their exact identity. Importantly, both chemicals were also found in the lumpfish blood samples, indicating they are both able to leach and be taken up by the fish. Further work is recommended to accurately identify these two chemicals.
4.4 PDs as indicators of car tire rubber contamination and exposure
Results from this study show that tire related chemicals, especially some of the commonly used antiozonants, are readily taken up into blood of fish exposed to car tire rubber in the form of CR. The compound 6PPD reached the highest blood concentrations in our study, proportionate to its content in CR granulate relative to the other PDs. In addition, it is suggested that DTPD, TPPD, C21H26N2 and C23H26N2 have the potential to be utilized as new markers of tire rubber contamination and exposure. Interestingly, Zhao et al. (2023) found DTPD to be the most abundant PD antiozonant in CR, followed by 6PPD and DPPD, which were present in similar amounts. In contrast, they found that 6PPD dominated in TWP, followed by DTPD and DPPD. Although the PDs are taken up and eliminated from the lumpfish blood over time at different rates, some remained detectable for 2 weeks after the exposure had ended. It should be noted that the presence of PDs in samples collected towards the end of the depuration period could have been caused, at least partially, by re-ingestion of egested CR particles. The PDs C23H26N2 and DTPD peaked and decreased more rapidly than the other additives, indicating a faster uptake and turnover in blood. The decrease in the concentrations of all PDs towards the end of the 14-day depuration period indicates excretion or transformation into other chemicals, as well as potential transport into other tissues and organs (e.g., the liver). Given the observed concentration levels, together with variations in the timing of peak concentrations for the different PDs, it is proposed that a combination of the three most concentrated PDs, 6PPD, C21H26N2, and DTPD, could be used for a comprehensive characterization of tire rubber exposure in vertebrates, including humans. This is supported by a recent screening of PDs in rubber and elastomer products, which highlighted the specificity of 6PPD, DTPD and DPPD as tire-related PD antioxidants (Zhao et al., 2023). Considering that concentrations of 6PPD, DTPD and DPPD were lower in CR compared to TWP, likely due to extended weathering and leaching (Zhao et al., 2023), 6PPD and DTPD are likely suitable biomarkers for recent exposure to tire rubber or tire leachate along with the unidentified C21H26N2.
4.5 Toxicity of car tire rubber to aquatic organisms
The CR exposure presented here did not induce mortality, and sub-lethal toxicity endpoints were not studied. However, toxic responses from exposure to tire rubber particles, including increased mortality, have previously been observed in other fish species, including rainbow trout (Oncorhynchus mykiss; Kellough, 1991), and the freshwater fathead minnow (Pimephales promelas) and the estuarine mummichog (Fundulus heteroclitus; LaPlaca and Hurk, 2020; LaPlaca et al., 2022). Reported sublethal effects following tire rubber exposure include altered swimming behavior and reduced growth in estuarine fish (Siddiqui et al., 2022), and sub-lethal toxicity in fathead minnow embryos (Chibwe et al., 2021) and the zebrafish Danio rerio (McIntyre et al., 2014). Importantly, the available data strongly indicates that toxicity responses to car tire rubber and its associated chemicals can be species-specific. For example, low concentrations of 6PPD-quinone were found to be lethal for juvenile Coho salmon (24 h LC50 of 0.79 μg/L) (Tian et al., 2020), but not for the closely related chum salmon (Oncorhynchus keta; McIntyre et al., 2021), or other fish and invertebrate species (Hiki et al., 2021). Specific toxicity of 6PPD and 6PPD-quinone has also been shown for zebrafish (Varshney et al., 2022), the anadromous Coho salmon (Blair et al., 2021; McIntyre et al., 2021) and the white-spotted charr (Salvelinus leucomaenis pluvius; Hiki et al., 2021).
Several of the heavy metals present in the CR are known to be highly toxic to fish (reviewed in Garai et al., 2021). For example, Cd, Cr, and Pb are non-essential elements for fish (in contrast to Zn and Fe), and able to cause toxicity even at very low concentrations in some species. These metals are redox active components and drive the redox reaction to form reactive oxygen species (ROS). A large body of literature describes mechanisms on how heavy metals can affect multiple organs in sensitive fish due to oxidative stress, and finally accumulate in tissues (Shahjahan et al., 2022, and references therein). However, no uptake of metals was detected in the present study focusing on blood, suggesting either the absence of uptake, efficient detoxification, or bioaccumulation in other organs of the fish. To our knowledge, no literature describing the toxic effects of heavy metals in Cyclopterus lumpus currently exists, hampering the evaluation of the sensitivity or resilience of lumpfish for the metal concentrations found in our study. Given the observed differences in metal concentrations and proportions between the CR and the lumpfish blood samples, combined with a lack of statistical difference in the metal concentrations between control and exposure groups, there is no indication that CR ingestion leads to take up of metals or causes negative effects on the health of lumpfish.
Other studies have shown toxic effects from exposure to car tire rubber leachates, mostly for aquatic invertebrates, such as daphnids, copepods, mussels and amphipods (Wik and Dave, 2009; Khan et al., 2019; Capolupo et al., 2021; 2020; Halsband et al., 2020). These studies have proposed several suspect metals and compounds as candidates for inducing the observed negative effects on physiological and fitness endpoints, including benzothiazole, PAHs, phthalates, and Zn. It can be expected that some toxicological responses are different between invertebrates and vertebrates, resulting from differences in detoxification potential, exposure mode (ingestion of particles versus exposure to leachate), and uptake kinetics for toxic compounds. An increased mechanistic understanding is needed to inform appropriate risk assessments of CR and TWP emissions (Tamis et al., 2021). Accessible ingredient lists used in tire rubber production would increase transparency and facilitate such assessments. A first attempt in that direction, the global automotive declarable substance list (GADSL), is an initiative by the Global Automotive Stakeholders Group (GASG). However, ingredient derivatives and constituents that react and transform quickly, and therefore become depleted in biological tissues over short time scales, are not included in these lists (Trudsø et al., 2022). Although a body of data and knowledge is developing with regards to the chemical composition of car tires and which ingredients could be driving the observed toxicological responses in different organisms, further research is needed to fully elucidate the most bioavailable and hazardous metals and chemicals.
5 Conclusion
This study demonstrates the ingestion, GI-tract retention, and egestion of CR particles in the marine fish species, Cyclopterus lumpus, in an experimental feeding study. The results indicate that the tissue concentrations of individual tire additives correlate well with the number of ingested CR particles and indicate short lifetimes for these additives in blood. The uptake of organic PD antiozonants, including 6PPD, into lumpfish blood shows the potential for this group of chemicals to serve as specific biomarkers of recent exposure to CR, TWPs, or leachates from those. The unidentified additive C21H26N2 reported here as constituent of CR material was also detectable in blood and may be a suitable additional tire chemical marker upon future identification of its molecular structure. A combination of the most abundant PDs found in lumpfish blood, 6PPD, C21H26N2, and DTPD, is recommended for assessment for use as biomarkers. Further exposure studies incorporating a higher temporal resolution with additional sampling time points would enable the application of toxicokinetic models to compare the uptake and elimination rates of the different compounds. Importantly, we demonstrate that analysis of low volume blood samples can be a non-lethal method for the detection of recent tire chemical exposure. In sufficiently large vertebrates, including humans, repeated measurements of the same individual(s) are possible and would afford a more accurate assessment of temporal levels that could be linked to specific exposure scenarios or histories. Although negative effects on lumpfish exposed to CR particles were not observed in this study, the potential toxicity of PD antiozonants other than 6PPD should be assessed using acute and long-term exposures to CR or TWP particles and their associated leachates to inform risk assessments and improved regulatory work for this group of substances.
Data availability statement
The original contributions presented in the study are included in the article/Supplementary Material, further inquiries can be directed to the corresponding author.
Ethics statement
Ethical approval was not required for the study involving animals in accordance with the local legislation and institutional requirements because Tire rubber is currently not classified as a contaminant and thus not subject to approval.
Author contributions
CH, DH, AB, LS, and MC contributed to conception and design of the study. FH and KS conducted the experiments. DH and VN conducted the chemical analyses. FH performed the statistical analysis. FH wrote the first draft of the manuscript. CH, DH, and AB wrote sections of the manuscript. All authors contributed to the article and approved the submitted version.
Funding
The research was conducted as part of the Fram Centre flagship Contaminants (project RubberTox, 581/2021). The work was also supported through the Research Council of Norway project ANDROMEDA (Grants 312262, 311313), funded through the JPI Oceans framework. For additional financial support we thank the Norwegian Research Council (PlastPoll 2021; 311313/E40). AB and LS received additional funding by the European Union’s Horizon Europe programme under grant agreement No. 101060213.
Acknowledgments
We would like to thank Carl Ballantine and the technical staff at the Akvaplan-niva Forsknings-og Innovasjonsstasjon Kraknes (FISK) for assistance with the feeding experiment, Unni Mette Nordang at NILU, Tromsø, for laboratory support, Peter Andersson for assistance with graphical design, and Prof. Sophie Bourgeon at UiT–The Arctic University of Tromsø for valuable feedback on this study. We thank Martin Amund Langaas Johansen for the extraction and quantification of crumb rubber particles.
Conflict of interest
The authors declare that the research was conducted in the absence of any commercial or financial relationships that could be construed as a potential conflict of interest.
Publisher’s note
All claims expressed in this article are solely those of the authors and do not necessarily represent those of their affiliated organizations, or those of the publisher, the editors and the reviewers. Any product that may be evaluated in this article, or claim that may be made by its manufacturer, is not guaranteed or endorsed by the publisher.
Author disclaimer
The views and opinions expressed are however those of the author(s) only and do not necessarily reflect those of the European Union, and the European Union cannot be held responsible for them.
Supplementary material
The Supplementary Material for this article can be found online at: https://www.frontiersin.org/articles/10.3389/fenvs.2023.1219248/full#supplementary-material
References
Adams, J. K., Bethany, Y. D., Samantha, N. A., Liisa, M. J., Sarah, B., Stern, G., et al. (2021). Anthropogenic Particles (Including Microfibers and Microplastics) in Marine Sediments of the Canadian Arctic. Sci. Total Environ. 784, 147155. doi:10.1016/j.scitotenv.2021.147155
Blair, S. I., Barlow, C. H., and McIntyre, J. K. (2021). Acute Cerebrovascular Effects in Juvenile Coho Salmon Exposed to Roadway Runoff. Can. J. Fish. Aquatic Sci. 78 (2), 103–109. doi:10.1139/cjfas-2020-0240
Bø, S. M., Bohne, R. A., Aas, B., and Hansen, L. M. (2020). Material Flow Analysis for Norway’s Artificial Turfs. IOP Conf. Ser. Earth Environ. Sci. 588 (4), 042068. doi:10.1088/1755-1315/588/4/042068
Bocca, B., Forte, G., Petrucci, F., Costantini, S., and Izzo, P. (2009). Metals Contained and Leached from Rubber Granulates Used in Synthetic Turf Areas. Sci. Total Environ. 407 (7), 2183–2190. doi:10.1016/j.scitotenv.2008.12.026
Boucher, J., and Friot, D. (2017). Primary Microplastics in the Oceans: A Global Evaluation of Sources. IUCN Int. Union Conservation Nat., doi:10.2305/IUCN.CH.2017.01
Capolupo, M., Gunaalan, K., Booth, A. M., Lisbet, S., Paola, V., and Elena, F. (2021). The Sub-Lethal Impact of Plastic and Tire Rubber Leachates on the Mediterranean Mussel Mytilus Galloprovincialis. Environ. Pollut. (Barking, Essex 1987) 283, 117081. doi:10.1016/j.envpol.2021.117081
Capolupo, M., Sørensen, L., Jayasena, K. D. R., Booth, A. M., and Fabbri, E. (2020). Chemical Composition and Ecotoxicity of Plastic and Car Tire Rubber Leachates to Aquatic Organisms. Water Res. 169, 115270. doi:10.1016/j.watres.2019.115270
Chandrapalan, T., and Kwong, R. W. M. (2021). Functional Significance and Physiological Regulation of Essential Trace Metals in Fish. J. Exp. Biol. 224 (24), jeb238790. doi:10.1242/jeb.238790
Chen, Y., Li, W., Xiang, L., Mi, X., Duan, M., and Wu, C. (2022). Fish Personality Affects Their Exposure to Microplastics. Ecotoxicol. Environ. Saf. 233, 113301. doi:10.1016/j.ecoenv.2022.113301
Chiba, R., Fujinuma, R., Yoshitomi, T., Shimizu, Y., and Kobayashi, M. (2023). Ingestion of Rubber Tips of Artificial Turf Fields by Goldfish. Sci. Rep. 13 (1), 1344. doi:10.1038/s41598-023-28672-3
Chibwe, L., Joanne, P., Shires, K., Khan, H., Clarence, S., Lavalle, C., et al. (2021). A Deep Dive into the Complex Chemical Mixture and Toxicity of Tire Wear Particle Leachate in Fathead Minnow. Environ. Toxicol. Chem. 41, 1144–1153. doi:10.1002/etc.5140
Dtsc and CalEPA, (2022). Product-chemical profile for motor vehicle tires containing 6PPD - final version. Sacramento, California, United States: Department of Toxic Substances Control (DTSC), California Environmental Protection Agency (CalEPA) and Safer Consumer Products.
Environment Canada and Health Canada, (2011). Screening assessment for the challenge 1,4-benzenediamine, N,N’-Mixed phenyl and tolyl derivatives and 1,4-benzenediamine, N,N`-Mixed tolyl and xylyl derivatives.” screening assessment CAS RN 68953-84-4 and 68478-45-5. Health Canada: Environment Canada.
Erman, B., Mark, J. E., and Roland, C. M. (2013). The science and technology of rubber. Fourth edition (Amsterdam ; Boston, USA: Elsevier Academic Press).
Evangeliou, N., Grythe, H., Klimont, Z., Heyes, C., Eckhardt, S., Lopez-Aparicio, S., et al. (2020). Atmospheric Transport Is a Major Pathway of Microplastics to Remote Regions. Nat. Commun. 11 (1), 3381. doi:10.1038/s41467-020-17201-9
Furuseth Ingvild, S., and Rødland, E. S. (2020). Reducing the release of microplastic from tire wear: nordic efforts. Nordic Council of Ministers. doi:10.6027/NA2020-909
Galtung, K. (2023). Exposure of marine invertebrates to car tire rubber: uptake of particles and related organic chemicals. MSc, Department of Arctic and Marine Biology. Tromsø, Norway: UiT - the Arctic University of Norway.
Garai, P., Banerjee, P., Mondal, P., Nimai, Ch, and Saha, Ra (2021). Effect of Heavy Metals on Fishes: Toxicity and Bioaccumulation. J. Clin. Toxicol., 1–10. doi:10.35248/2161-0495.21.s18.001
Goßmann, I., Halbach, M., and BarbaraScholz-Böttcher, M. (2021). Car and Truck Tire Wear Particles in Complex Environmental Samples – A Quantitative Comparison with ‘Traditional’ Microplastic Polymer Mass Loads. Sci. Total Environ. 773, 145667. doi:10.1016/j.scitotenv.2021.145667
Halsband, C., Sørensen, L., Booth, A. M., and Herzke, D. (2020). Car Tire Crumb Rubber: Does Leaching Produce a Toxic Chemical Cocktail in Coastal Marine Systems? Front. Environ. Sci. 8. doi:10.3389/fenvs.2020.00125
Hedges and Company, (2021). How many cars are there in the world? Statistics by country. Hudson, Ohio, USA: Hedges and Company. https://hedgescompany.com/blog/2021/06/how-many-cars-are-there-in-the-world/.
Hiki, K., Asahina, K., Kato, K., Yamagishi, T., Omagari, R., Iwasaki, Y., et al. (2021). Acute Toxicity of a Tire Rubber-Derived Chemical, 6PPD Quinone, to Freshwater Fish and Crustacean Species. Environ. Sci. Technol. Lett. 8 (9), 779–784. doi:10.1021/acs.estlett.1c00453
Hiki, K., and Yamamoto, H. (2022). The Tire-Derived Chemical 6PPD-Quinone Is Lethally Toxic to the White-Spotted Char Salvelinus Leucomaenis Pluvius but Not to Two Other Salmonid Species. Environ. Sci. Technol. Lett. 9 (12), 1050–1055. doi:10.1021/acs.estlett.2c00683
Huang, W., Shi, Y., Huang, J., Deng, C., Tang, S., Liu, X., et al. (2021). Occurrence of Substituted P-Phenylenediamine Antioxidants in Dusts. Environ. Sci. Technol. Lett. 8 (5), 381–385. doi:10.1021/acs.estlett.1c00148
Imsland, A. K., Reynolds, P., Eliassen, G., Hangstad, T. A., Nytrø, A. V., Foss, A., et al. (2015). Feeding Preferences of Lumpfish (Cyclopterus Lumpus L.) Maintained in Open Net-Pens with Atlantic Salmon (Salmo Salar L.). Aquaculture 436, 47–51. doi:10.1016/j.aquaculture.2014.10.048
Jarlskog, I., Jaramillo-Vogel, D., Rausch, J., Gustafsson, M., Strömvall, A.-M., and Andersson-Sköld, Y. (2022). Concentrations of Tire Wear Microplastics and Other Traffic-Derived Non-Exhaust Particles in the Road Environment. Environ. Int. 170, 107618. doi:10.1016/j.envint.2022.107618
Kambe, T., Tsuji, T., Hashimoto, A., and Itsumura, N. (2015). The Physiological, Biochemical, and Molecular Roles of Zinc Transporters in Zinc Homeostasis and Metabolism. Physiol. Rev. 95 (3), 749–784. doi:10.1152/physrev.00035.2014
Kellough, R. M. (1991). The effects of scrap automobile tires in water. Ontario: Queen’s Printer for Ontario.
Khan, F. R., Halle, L. L., and Palmqvist, A. (2019). Acute and Long-Term Toxicity of Micronized Car Tire Wear Particles to Hyalella Azteca. Aquat. Toxicol. 213, 105216. doi:10.1016/j.aquatox.2019.05.018
Klöckner, P., Seiwert, B., Weyrauch, S., Escher, B. I., Reemtsma, T., and Wagner, S. (2021). Comprehensive Characterization of Tire and Road Wear Particles in Highway Tunnel Road Dust by Use of Size and Density Fractionation. Chemosphere 279, 130530. doi:10.1016/j.chemosphere.2021.130530
Knight, L. J., Parker-Jurd, F. N. F., Al-Sid-Cheikh, M., and Thompson, R. C. (2020). Tyre Wear Particles: An Abundant yet Widely Unreported Microplastic? Environ. Sci. Pollut. Res. 27 (15), 18345–18354. doi:10.1007/s11356-020-08187-4
Kole, P. J., Löhr, A. J., Van Belleghem, F. G. A. J., and Ragas, Ad M. J. (2017). Wear and Tear of Tyres: A Stealthy Source of Microplastics in the Environment. Int. J. Environ. Res. Public Health 14 (10), 1265. doi:10.3390/ijerph14101265
Krang, A.-S., Olshammar, M., Edlund, D., Hållén, J., Stenfors, E., and Friesen, L. W. von (2019). Sammanställning av kunskap och åtgärdsförslag för att minska spridning av mikroplast från konstgräsplaner och andra utomhusanläggningar för idrott och lek. Göteborg, Sweden: IVL Svenska Miljöinstitutet.
Kreider, M. L., Julie, M. P., Britt, L. M., Leonard, I. S., and Finley, B. L. (2010). Physical and Chemical Characterization of Tire-Related Particles: Comparison of Particles Generated Using Different Methodologies. Sci. Total Environ. 408 (3), 652–659. doi:10.1016/j.scitotenv.2009.10.016
LaPlaca, S. B., and Hurk, P. van den (2020). Toxicological Effects of Micronized Tire Crumb Rubber on Mummichog (Fundulus Heteroclitus) and Fathead Minnow (Pimephales Promelas). Ecotoxicology 29 (5), 524–534. doi:10.1007/s10646-020-02210-7
LaPlaca, S. B., Rice, C. D., and Hurk, P. van den (2022). Chronic Toxicity of Tire Crumb Rubber Particles to Mummichog (Fundulus Heteroclitus) in Episodic Exposures. Sci. Total Environ. 846, 157447. doi:10.1016/j.scitotenv.2022.157447
Leads, R. R., and Weinstein, J. E. (2019). Occurrence of Tire Wear Particles and Other Microplastics within the Tributaries of the Charleston Harbor Estuary, South Carolina, USA. Mar. Pollut. Bull. 145, 569–582. doi:10.1016/j.marpolbul.2019.06.061
Li, X., Berger, W., Craig, M., and Mattina, M. J. I. (2010). Characterization of Substances Released from Crumb Rubber Material Used on Artificial Turf Fields. Chemosphere 80 (3), 279–285. doi:10.1016/j.chemosphere.2010.04.021
Magnusson, K., Eliasson, K., Anna, F., Haikonen, K., Hultén, J., Olshammar, M., et al. (2016). Swedish Sources and Pathways for Microplastics to the Marine Environment. https://www.ivl.se/download/18.694ca0617a1de98f473b16/1628417679619/FULLTEXT01.pdf.
McIntyre, J. K., Prat, J., Cameron, J., Wetzel, J., Mudrock, E., Peter, K. T., et al. (2021). Treading Water: Tire Wear Particle Leachate Recreates an Urban Runoff Mortality Syndrome in Coho but Not Chum Salmon. Environ. Sci. Technol. 55 (17), 11767–11774. doi:10.1021/acs.est.1c03569
McIntyre, J. K., Davis, J. W., Incardona, J. P., Stark, J. D., Anulacion, B. F., and Scholz, N. L. (2014). Zebrafish and Clean Water Technology: Assessing Soil Bioretention as a Protective Treatment for Toxic Urban Runoff. Sci. Total Environ. 500-501, 173–180. doi:10.1016/j.scitotenv.2014.08.066
Parker, B. W., Beckingham, B. A., Ingram, B. C., Ballenger, J. C., Weinstein, J. E., and Sancho, G. (2020). Microplastic and Tire Wear Particle Occurrence in Fishes from an Urban Estuary: Influence of Feeding Characteristics on Exposure Risk. Mar. Pollut. Bull. 160, 111539. doi:10.1016/j.marpolbul.2020.111539
Parker-Jurd, , Florence, N. F., Napper, I. E., Geoffrey, D., Abbott, S. H., and Thompson, R. C. (2021). Quantifying the Release of Tyre Wear Particles to the Marine Environment via Multiple Pathways. Mar. Pollut. Bull. 172, 112897. doi:10.1016/j.marpolbul.2021.112897
Reddy, C. M., and Quinn, J. G. (1997). Environmental Chemistry of Benzothiazoles Derived from Rubber. Environ. Sci. Technol. 31 (10), 2847–2853. doi:10.1021/es970078o
Rogge, W. F., Hildemann, M. A., Mazurek, G. R. C., Simoneit, B. R. T., and Simoneit, B. R. T. (1993). Sources of Fine Organic Aerosol. 3. Road Dust, Tire Debris, and Organometallic Brake Lining Dust: Roads as Sources and Sinks. Environ. Sci. Technol. 27 (9), 1892–1904. doi:10.1021/es00046a019
Seiwert, B., Nihemaiti, M., Troussier, M., Weyrauch, S., and Reemtsma, T. (2022). Abiotic Oxidative Transformation of 6-PPD and 6-PPD Quinone from Tires and Occurrence of Their Products in Snow from Urban Roads and in Municipal Wastewater. Water Res. 212, 118122. doi:10.1016/j.watres.2022.118122
Shahjahan, Md, Taslima, K., Rahman, M. S., Al-Emran, Md, Shanon, I. A., and Faggio, C. (2022). Effects of Heavy Metals on Fish Physiology – A Review. Chemosphere 300, 134519. doi:10.1016/j.chemosphere.2022.134519
Siddiqui, S., Dickens, J. M., Cunningham, B. E., Hutton, S. J., Pedersen, E. I., Harper, B., et al. (2022). Internalization, Reduced Growth, and Behavioral Effects Following Exposure to Micro and Nano Tire Particles in Two Estuarine Indicator Species. Chemosphere 296, 133934. doi:10.1016/j.chemosphere.2022.133934
Smithers, (2019). MARKET REPORT The Future of Global Tires to 2024. https://www.smithersapex.com/services/market-reports/transportation/global-tire-markets-to-2024.
Sundt, P., Haugedal, S., Rem, T., and Schulze, P.-E. (2020). Norske landbaserte kilder til mikroplast - Miljødirektoratet 1648 Miljødirektoratet. https://www.miljodirektoratet.no/publikasjoner/2021/april-2021/norske-landbaserte-kilder-til-mikroplast/.
Tamis, J. E., Koelmans, A. A., Dröge, R., Nicolaas, H. B. M., Kaag, M. C., Keur, P. C., et al. (2021). Environmental Risks of Car Tire Microplastic Particles and Other Road Runoff Pollutants. Microplastics Nanoplastics 1 (1), 10. doi:10.1186/s43591-021-00008-w
Tian, Z., Zhao, H., Peter, K. T., Gonzalez, M., Wetzel, J., Wu, C., et al. (2020). A Ubiquitous Tire Rubber–Derived Chemical Induces Acute Mortality in Coho Salmon. Science 371, 185–189. doi:10.1126/science.abd6951
Trudsø, L. L., Nielsen, M. B., Hansen, S. F., Syberg, K., Kampmann, K., Khan, F. R., et al. (2022). The Need for Environmental Regulation of Tires: Challenges and Recommendations. Environ. Pollut. 311, 119974. doi:10.1016/j.envpol.2022.119974
UNEP (2018). Mapping of Global Plastics Value Chain and Plastics Losses to the Environment: With a Particular Focus on Marine Environment. http://www.unep.org/pt-br/node/27212.
Vandendriessche, S., Messiaen, M., O’Flynn, S., Vincx, M., and Degraer, S. (2007). Hiding and Feeding in Floating Seaweed: Floating Seaweed Clumps as Possible Refuges or Feeding Grounds for Fishes. Estuar. Coast. Shelf Sci. 71 (3), 691–703. doi:10.1016/j.ecss.2006.09.017
Varshney, S., Gora, A. H., Siriyappagouder, P., Kiron, V., and PålOlsvik, A. (2022). Toxicological Effects of 6PPD and 6PPD Quinone in Zebrafish Larvae. J. Hazard. Mater. 424, 127623. doi:10.1016/j.jhazmat.2021.127623
Wagner, S., Hüffer, T., Klöckner, P., Wehrhahn, M., Hofmann, T., and Reemtsma, T. (2018). Tire Wear Particles in the Aquatic Environment - A Review on Generation, Analysis, Occurrence, Fate and Effects. Water Res. 139, 83–100. doi:10.1016/j.watres.2018.03.051
Wang, W., Cao, G., Zhang, J., Wu, P., Chen, Y., Chen, Z., et al. (2022). Beyond Substituted P-Phenylenediamine Antioxidants: Prevalence of Their Quinone Derivatives in PM2.5. Environ. Sci. Technol. 56 (15), 10629–10637. doi:10.1021/acs.est.2c02463
Wbcsd and Tire Industry Project, (2019). Global ELT Management - A Global State of Knowledge on Regulation, Management Systems, Impacts of Recovery and Technologies. https://www.wbcsd.org/Sector-Projects/Tire-Industry-Project/End-of-Life-Tires-ELTs.
Wik, A., and Dave, G. (2009). Occurrence and Effects of Tire Wear Particles in the Environment – A Critical Review and an Initial Risk Assessment. Environ. Pollut. 157 (1), 1–11. doi:10.1016/j.envpol.2008.09.028
Wootton, N., Reis-Santos, P., and BronwynGillanders, M. (2021). Microplastic in Fish – A Global Synthesis. Rev. Fish Biol. Fish. 31 (4), 753–771. doi:10.1007/s11160-021-09684-6
Zhao, H. N., Hu, X., Gonzalez, M., Rideout, C. A., Hobby, G. C., Fisher, M. F., et al. (2023). Screening P-Phenylenediamine Antioxidants, Their Transformation Products, and Industrial Chemical Additives in Crumb Rubber and Elastomeric Consumer Products. Environ. Sci. Technol. 57 (7), 2779–2791. doi:10.1021/acs.est.2c07014
Keywords: anti-ozonants, biomarker, blood, car tire additives, 6PPD and 6PPD-quinone, GC-MS, heavy metals, phenylenediamines
Citation: Hägg F, Herzke D, Nikiforov VA, Booth AM, Sperre KH, Sørensen L, Creese ME and Halsband C (2023) Ingestion of car tire crumb rubber and uptake of associated chemicals by lumpfish (Cyclopterus lumpus). Front. Environ. Sci. 11:1219248. doi: 10.3389/fenvs.2023.1219248
Received: 08 May 2023; Accepted: 29 September 2023;
Published: 12 October 2023.
Edited by:
Jane A. Entwistle, Northumbria University, United KingdomReviewed by:
Mohamed Ahmed Ibrahim Ahmed, Assiut University, EgyptPaula S. Tourinho, Masaryk University, Czechia
Copyright © 2023 Hägg, Herzke, Nikiforov, Booth, Sperre, Sørensen, Creese and Halsband. This is an open-access article distributed under the terms of the Creative Commons Attribution License (CC BY). The use, distribution or reproduction in other forums is permitted, provided the original author(s) and the copyright owner(s) are credited and that the original publication in this journal is cited, in accordance with accepted academic practice. No use, distribution or reproduction is permitted which does not comply with these terms.
*Correspondence: Claudia Halsband, clh@akvaplan.niva.no