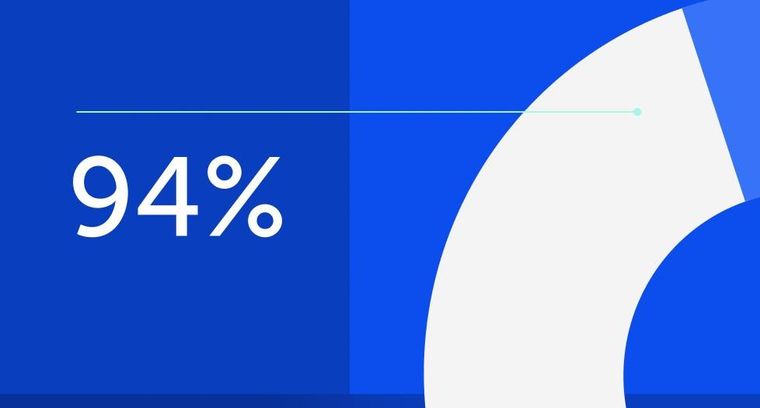
94% of researchers rate our articles as excellent or good
Learn more about the work of our research integrity team to safeguard the quality of each article we publish.
Find out more
ORIGINAL RESEARCH article
Front. Environ. Sci., 15 June 2023
Sec. Environmental Informatics and Remote Sensing
Volume 11 - 2023 | https://doi.org/10.3389/fenvs.2023.1214988
Kuttanad region in Kerala, India, is a place that predominantly consists of soft soil formations with low shear strength and low water resistance rendering them problematic for construction purposes. Pavements constructed on such soft deposits have been subjected to structural rutting and the high erodibility of the in-situ soil necessitates the need to use suitable ground improvement techniques. The present environmental scenario demands the implementation of sustainable techniques for ground rejuvenation and effective stabilizers for enhancing engineering properties. This study investigates the amelioration of Kuttanad soft soil using chitosan as a soil amendment to improve its durability and erodibility characteristics. The untreated and chitosan-treated samples were exposed to 5 h of wetting cycle followed by 43 h of drying cycles until their failure. The unconfined compressive strength (UCS) of samples prepared with different dosages (0.5, 2, 4%) and cured for 14, 28, 60, and 90 days was evaluated at the onset and after each drying cycle to measure their durability index. Kuttanad soil was amended with 2% and cured for 90 days withstood five cycles with a UCS of more than 1,000 kPa. The drip erosion tests were used to check the erodibility performance for the aforementioned different dosages and curing periods. The 2% and 4% chitosan amended samples resisted the entire test duration of 10 min indicating the highest water erosion resistance. The findings of the current study evaluated through durability and erosion tests reinforced the effectiveness of chitosan as an effective biopolymer for soft soils subjected to constant water attack and can be easily implemented in places with such vulnerability. A typical earthen canal lining amended with chitosan reduced the carbon emissions by 8.74 and 7.44 times compared to conventional amendments like lime and cement in Carbon Footprint Analysis.
The Kuttanad region in Kerala is a low-lying land on the west coast of India, separated from the Arabian Sea by a narrow strip of land. The area contains acid sulfate soils, hot summers with copious rainfall, and increased humidity, and remains submerged underwater for most of the year (Nair and Sreedharan, 1986). Besides having poor engineering properties, soft clay deposits in the region are subjected to frequent drying, even at ambient temperatures (Ayyar, 1966). These deposits are composed of silty clay with moderate amounts of organic content. Extensive soft clay deposits in this region expose the structures constructed on them to severe issues. Hence ground improvement techniques are imperative before taking on any construction work on such deposits to avoid structural failures. For pavement, a weak subgrade contributes to the failures such as structural rutting, especially for a low-volume flexible road. In the region of lower Kuttanad, pavements constructed on soft soils have suffered such failures (Moum et al., 1973). Another pertinent issue is the moisture attack in these soils, leading to the poor performance of the pavements within the designed period itself. Since Kuttanad is the rice bowl of Kerala, the ground improvement techniques adopted for improving the strength and durability of soils must be sustainable.
Soil stabilization can be classified into physical, mechanical, chemical, and biological approaches. Among these techniques, chemical stabilization is the most widely employed for enhancing particle interfacial interactions in soil by adding chemicals like Portland cement (Moh, 1962; Suksun et al., 2010; Jurong and Siau, 2021), lime (Moghal et al., 2016; Moghal et al., 2020a; Moghal et al., 2020b; Al-Mahbashi et al., 2021; Moghal et al., 2021; Shaker et al., 2021; Syed et al., 2021), fly ash (Moghal and Sivapullaiah, 2011; Moghal and Moghal, 2012; Mohammed et al., 2018), nano calcium silicate (Mohammed and Moghal, 2016; Moghal et al., 2023), coal gauge (Ashfaq et al., 2021; Ashfaq et al., 2022a; Ashfaq et al., 2022b), blast furnace slag (Sruthi et al., 2022), bitumen (Shubber et al., 2009), granite dust (Amulya et al., 2022), and geopolymer binder (Wang et al., 2021). Despite the simplicity of this procedure, chemically treated soil presents environmental risks, making them less desirable. Mechanical stabilization includes compaction, preloading, and soil replacement which are ineffective in this type of soil due to the soil’s high moisture content. Cement has served as one of the most preferred stabilizers owing to its excellent durability and strength characteristics. However, the heavy carbon footprint of chemical stabilizers has demanded a shift to alternative sustainable and eco-friendly approaches such as Microbial-induced calcite precipitation (MICP), and Biopolymer stabilization. However, MICP possesses certain limitations, such as requiring highly specialized environmental and growing conditions, applicable largely to coarse-grained soil, the production of ammonia as a by-product, and the challenge of achieving a uniform distribution of calcite (Dhami et al., 2016; Chittoori et al., 2019; Tang et al., 2020; Almajed et al., 2021; Sharma et al., 2021; Moghal et al., 2022a; Moghal et al., 2022b; Ramachandran et al., 2022). So, Biopolymer stabilization is an emerging soil stabilization technique with the benefits of reduction in environmental hazard risk is preferred (Vydehi and Moghal, 2022).
Biopolymers have exhibited tremendous results when amalgamated with different types of soils. Factors influencing the efficiency of a biopolymer inclusion include the biopolymer content, curing time, molding moisture content, and soil type (Fatehi et al., 2021). The implementation of biopolymers is still an emerging area of ground improvement in Kerala. Among biopolymers, cohesive soil treatment has been carried out using Xanthan Gum, Guar Gum, Gellan gum, Beta Glucan, and Lignin (Zhang et al., 2015; Fatehi et al., 2021; Vydehi and Moghal, 2022). It has been observed that the widely used Xanthan Gum, Gellan Gum, and Agar Gum have imparted only marginal improvement in the wet strength or water resistance characteristics (Chang et al., 2015). Chitosan has been predominantly employed in the area of geoenvironmental engineering works such as heavy metal remediation and erosion studies and has proven its efficiency in the respective fields (Orts et al., 2000; Adamczuk et al., 2021; Liu et al., 2022). The potential of chitosan in the amelioration of cohesive soils has been seldom explored by researchers. This study focuses on applying chitosan biopolymer to improve the durability and erodibility characteristics of Kuttanad clay. Chitosan is formed by the alkali deacetylation of chitin, which is formed from discarded crustacean exoskeleton shells. The preliminary procurement of the second most abundant cellulose, chitin was from the shells of mollusks in the year 1799. Ever since the production of chitin has risen to around 1,000 billion tons per year (Hahn et al., 2020). Around 1.5 million tons of the world’s waste crab, shrimp, and lobster shells are produced in southeast Asia each year, accounting for 6 to 8 million tons worldwide. Comparatively, the meat of a crab makes up just about 40% of its mass, whereas a tuna fish can be harvested as fillets for 75% of its weight (Knorr, 1991; Yan and Chen, 2015). Chitosan’s enormous potential for utilization in various industries has long been acknowledged due to its solubility, ability to build materials, biodegradability, and variety of bioactive qualities. Chitosan is a valuable substance in geotechnical and geo-environmental situations to enhance soil strength and lessen erosion during irrigation (Strand et al., 2003; Fang et al., 2004; Kamari et al., 2011; Aguilar et al., 2016; Li et al., 2020).
The resistance to moisture attack of polymer-stabilized soils is a matter that should be addressed, especially in the region of Kuttanad (Suganya and Sivapullaiah, 2015; Suganya and Sivapullaiah, 2017). Improving the durability against moisture of polymer–modified soil will significantly increase the stability of polymer-stabilized soils and eventually diminish the infrastructure’s long-term maintenance cost. The escalating global warming crisis has pushed engineers to undertake sustainability assessments for all works. With a vision to reduce carbon emissions associated with each geotechnical work, the research on implementing sustainable materials and evaluating their contribution to global warming becomes imperative. This study explores the suitability of chitosan as an effective stabilizer for soil amendment by evaluating its durability and erodibility characteristics. Additionally, the carbon emission analysis has been carried out for the chitosan mixed canal lining system and a comparison has been made to traditional chemical stabilizers.
The chitosan biopolymer used in this study was purchased from Swakit Biotech Private Limited, Basavanagudi, Bangalore. The material has a high molecular weight with the molecular formula C6H11NO4. Chitosan’s molecular weight is correlated with its viscosity. Increasing chitosan’s molecular weight and concentration could significantly enhance its characteristics. About 12% of non-free amino groups are present in its structure which provokes medium solubility and high viscosity levels. The process involved in the manufacturing of chitosan is depicted in Figure 1. The physical and chemical properties of chitosan biopolymer are mentioned in Table 1. Chitosan powder was taken at varying proportions of 0.5%, 2%, and 4% relative to the dry weight of the soil. The samples were prepared at the optimum moisture content (OMC) and maximum dry density.
For the current study, the samples were procured from the Kuttamangalam district of Kerala from a depth of approximately 2 m [Kuttamangalam, Kuttanad, India (9°29′14.9″ N 76° 23′25.1″ E)]. The index and engineering properties of the soil are summarized in Table 2. The soil has minerals such as quartz, microcline, muscovite, amphibole, gibbsite, magnetite, and pyrite (Suganya and Sivapullaiah, 2020). According to ASTM D4318-17 (2017), the base soil’s Atterberg plastic and liquid limits were assessed and discovered to be 42.57% and 55.73%, respectively. Based on gradation curves and Atterberg limits, the soil is classified as CH as per the ASTM D2487-06 (2017) (Unified Soil Classification 122 System) (Table 2). The maximum dry density (MDD) and optimum moisture content (OMC) of as 1.48 g/cm3 and 23.6% respectively, by the standard proctor test.
Figure 2 depicts the procedure adopted for the chitosan-stabilized samples’ alternate wetting and drying cycles. The durability tests have been conducted in accordance with ASTM-D559-03 (2003). As mentioned in Section 2.1, the samples have been prepared at various dosages of 0.5, 2, and 4% and will be hereafter designated as C1, C2, and C3. After the preparation of the samples, they were naturally cured for 14, 28, 60, and 90 days. Following the curing process, the samples were subjected to compression tests after every cycle until failure. The samples were immersed in deionized water at room temperature for 5 h. They were then dried in an oven at 70°C for 43 h. This process is referred to as one cycle of wetting and drying. The sample’s water content, mass, and dimensions were measured in each tested specimen to determine any change.
Where Qun1 represents the treated sample’s UCS value at its particular cycle (1,2,3,4,5) and Qun2 is the UCS value of the sample at the start of the test (initial cycle).
Compressive strength is one of the most widely used techniques for assessing the effectiveness of soil stabilization. The unconfined compressive strength was chosen to assess the durability of clay soil stabilized with chitosan in accordance with ASTM D 2166–06 (2010). Axial loading was applied on the top of each cylindrical specimen at a strain rate of 1 mm/min up to an axial strain of 5%. The samples were tested for their compressive strength at the onset and after each wetting and drying cycle.
The erosion test served as the basis for assessing the plaster’s resistance to water erosion by dripping action. Tests for drip erosion were conducted by the recommendations suggested in AENOR (2008) and (Walker, 2022). The test requisite is setting up a sample on a surface at an inclination of 27° with the horizontal. The soil specimen is subsequently subjected to water drizzle discharged from a location precisely 1 m above the specimen’s center. A 50 mL per minute release rate is used for the drops. While performing this test, the test specimen was exposed for 10 min or until it suffered severe erosional damage, whichever came first.
To eliminate any gravel-sized particles, the base soil described earlier was first sieved using sieve No. 4 (opening = 4.75 mm). The preparation of the base soil was then made with either water or chitosan solutions at a ratio of 4:1 (soil to water). The selected ratio is established on regional custom to provide adequate practicability. Preparing the sample involves hand-combining the soil, water, or base soil. The specimens prepared with either water or chitosan were thoroughly mixed by hand with a metal trowel for about 5 min to create a uniform mixture for the untreated soil sample (Aguilar et al., 2016). In a controlled atmosphere with a relative humidity of 60% and a temperature of 20°C, the earthy mixture was then poured into a cylindrical mold and air-dried for around 28 days. The cylindrical earthen specimens’ 55 mm diameter and 10 mm height were utilized for erosion testing.
The Scanning Electron Microscope provides direct observation of the clay particle arrangement or fracture surfaces through the soil sample. The samples after appropriate treatment were mounted onto the Aluminium mounting disc with the help of carbon tape. Then the samples were sputter coated with gold (10 nm) in an argon atmosphere in order to avoid charging problems during imaging. For the test, the samples were air-dried for analysis utilizing TESCAN VEGA 3 LMU high-performance, Variable Pressure Analytical SEM with LAB6 having a high resolution of 2 nm.
Sustainable development has become an integral element of all civil engineering works and demands environmental impact assessment at every stage of a product or work. In this regard, the carbon emissions produced from the construction of chitosan-treated canal lining have been estimated to understand the environmental contribution of chitosan as a stabilizer. Life Cycle Analysis addresses the environmental implications of any product or service from cradle to grave. The approach used for the estimation of carbon emissions follows the work of Shillaber et al. (2016) and Amulya et al. (2023). The phases identified for the calculation of carbon emissions include the materials, procurement and haulage of materials, and site operations. Similar case studies on amended soils have been carried out to bring out the efficiency of these novel materials over traditional stabilizers (Amulya et al., 2023; Varsha et al., 2023).
The results of untreated soil specimens indicated that no significant decrement or increment occurred in UCS for the different curing periods. As depicted in Figure 3, a significant increase in the strength from 14 days to 90 days for C1, C2, and C3, indicated that the biopolymer induces strength with time. It is also noted that the strength increases with an increase in dosage from C1 to C3 due to three-dimensional bridges formed by chitosan biopolymer with the clay minerals (Hataf et al., 2018). An integrated network of chitosan fiber distributed through the void spaces and across the soil particles leads to enhanced strength in the chitosan-amended soils. The C3 samples performed well compared to C1 and C2. By extending the curing period, chitosan consumes more time penetrating the diffuse double layer, which is filled with positive cations and surrounds the soil particles. The extension of the curing period is recommended for the bridging of soil particles via chitosan fibers. Chitosan has shown similar improvement in strength when amalgamated with CH soil with an increase in the curing period (Reddy et al., 2018). The two factors which mainly affect the strength development are biopolymer content, moisture content, and curing period. The degree of acetylation of chitosan is also an influencing factor on the strength performance. Chitosan with a higher degree of acetylation has successfully enhanced the strength of soils with comparatively higher clay content and acidic pH (Adamczuk and Jozefaciuk, 2022). Studies on cohesive soils have proved that chitosan is capable of imparting considerable strength at shorter and longer curing periods (Hataf et al., 2018) and a similar trend is depicted in Figure 3. A lower dosage of chitosan (0.16%) has also been successful in enhancing UCS strength up to 2.9 MPa in clay with low plasticity (Hataf et al., 2018). In the earlier stages, the chitosan merely acts as a filler material and leads to a uniformly stabilized soil fabric that resists compression. With an increase in the curing period, the fiber formations occur and cause the bridging of soil aggregations as shown in Figure 4. The strength imparted by chitosan (4.3 MPa) to the studied soil at 90 days of curing is higher than Xanthan Gum, and Beta-glucan stabilized fine-grained soils (Chang and Cho, 2012; Latifi et al., 2016). These sustainable materials can impart higher strength at lower dosages than traditional cement stabilizers in fine-grained soils (Chang et al., 2020).
FIGURE 4. SEM images of the 90 days cured (A) C2 before durability test (B) C2 after fifth cycle (C) C4 after fifth cycle.
In general, the friction and cohesion in a soil determine its mechanical strength. When soil and chitosan biopolymer are combined in the presence of water, the cohesiveness between soil particles is increased. At the initial curing periods of 14 and 28 days, the predominant mechanism which brings stability to the chitosan-soil mix is the ability of chitosan to act as a filler material (Figure 4). This leads to a uniformly distributed fabric of soil aggregations. As the curing period is increased to 60 days, fiber formations cause bridging of soil aggregations for C2 and C3. However, the wetting cycle results in the disintegration of bonds and the formation of interaggregate voids within the soil fabric leading to reduced UCS values (Hataf et al., 2018; Badakhshan et al., 2023). Another factor influencing the behavior of chitosan-amended soil is the charged nature of chitosan which facilitates an electrostatic interaction between the biopolymer and the diffuse double layer of clay minerals (Figure 5). Chitosan’s positive charge and the surface clay minerals’ negative charges combine to form an ionic bond, which improves the cohesiveness of the soil particles. The ionic interaction between the chitosan and soil particles via this mechanism increases the mechanical characteristics. When chitosan is added to soil, the chitosan fills the void spaces causing soil aggregations. The diffuse double layer decreases and leads to a stiffer matrix. The higher curing periods facilitate the formation of fibers which enhances the networking of these soil aggregations. This is the predominant factor that contributes to increased strength in chitosan-amended soils. Such mechanisms have been observed in both cohesionless and cohesive soils when amended with polysaccharide biopolymers (Hataf et al., 2018; Kannan and Sujatha, 2023).
The durability test results revealed that the UCS of the biopolymer-treated sample loses its strength over the wetting and drying cycles as shown in Figure 6. The loss in strength is very high in the initial testing cycles when compared to the successive cycles. It is also noted that even though C3 chitosan samples provided better UCS strength initially, the alternative wetting and drying cycles initiated the failure of the samples and could not maintain their strength. C2 chitosan-treated samples showed better durability against the alternative wetting and drying cycles. At higher dosages, fibers tend to cluster together, hindering the cohesion between particles which leads to poor performance of C3. However, the performance of C3 was better when compared to that of C1 owing to the insufficient amount of chitosan in C1 to impart substantial stability. The UCS of C1 and C3 for 14 and 28-day curing periods did not sustain even 2 cycles of wetting and drying cycles.
FIGURE 6. Variation in the Unconfined Compression Strength of Chitosan amended soil samples for different wetting and drying cycles.
The C2 dosage of 90 days curing period performed well among all samples for 5 successive wetting and drying cycles and retained a considerably good UCS strength of 1,020 kPa as shown in Figure 7. C2 performance over time was improved as the 14 and 28 days cured samples lasted for only 3 cycles whereas the 60 and 90 days cured samples lasted for 5 cycles of wetting and drying. The durability index of the stabilized samples was evaluated to better understand the effect of wetting and drying cycles and compressive strength. The durability index for the specimens was calculated utilizing the peak compressive strength computed after any number of cycles to the peak compressive strength computed at the initial cycle. The durability index of C2- 90 days is higher compared to all other samples as the reduction in UCS value was lesser over the successive cycles and lasted for 5 wetting and drying cycles as depicted in Figure 8. The increase in durability index can be directly linked to the higher stiffness of the chitosan-soil matrix and consequently higher compressive strength. As seen in Figure 8, the best performance is exhibited by C2 at higher curing periods of 60 and 90 days. The insufficient stability of the fabric and higher mass loss led to a drastic reduction in strength and durability index at 14 and 28 days of curing especially after the third cycle. Dosages higher than the optimum (2%) reduced the adhesion between soil particles and chitosan resulting in reduced strength (Kannan and Sujatha, 2023). C1 and C3 of 14 and 28 days failed after passing through 1 wetting and drying cycle. C3 samples caused fibers to agglomerate together and affected the adhesion between soil particles and chitosan. C1 exhibited poor performance due to the minimal bonding of soil particles with biopolymer and did not maintain its bond integrity over cycles causing failure. So, C2 can be considered an optimum dosage that ensures sufficient bonding and prevents the cluster formation of fibers.
FIGURE 7. Images of 90 days cured - 2% chitosan amended soft clay following alternative wetting and drying (w–d) cycles.
The weight loss for all the dosages and curing period was initially the same till the first cycle. Failure was initiated in C1 and C3 over the successive wetting cycle which led to a reduction of weight to 100% eventually. C2 dosage performed considerably well and weight loss was around 25% at the end of 5 cycles of wetting and drying. The weight loss Vs. No of cycles is plotted in Figure 9. The moisture content of C3 samples increases initially after the wetting cycle for the first cycle showing that the biopolymer chitosan is hydrophilic material as it absorbs water. The water content for other dosages was nearly the same without any significant change (Figure 10). After the successive cycles, the moisture content of the soil had reduced in the second and third cycles, and an increase in the trend was observed after the third cycle. The moisture content reduced considerably after the first cycle for C1 and the third cycle for C2 and C3 during the initial curing periods. Whereas, C3 showed an initial gain and later maintained a constant moisture change at higher curing periods. Though hydrophilic in nature, the hydrophilicity of chitosan is lesser compared to other biopolymers such as Xanthan Gum and Guar Gum (Vydehi and Moghal, 2022).
FIGURE 9. Percentage change of mass over different cycles of chitosan biopolymer-treated UCS samples.
FIGURE 10. Percentage change of water content over different cycles of chitosan-treated soil samples.
Up to the first cycle of wetting and drying, mass loss for C1 is reduced by 10%. Whereas, the effect of the wetting and drying on the mass loss for C2 and C3 was marginal for the initial cycle for all curing periods. The mass loss exhibited a steep decrease for C1 and C3 after the second and third cycles respectively. This decrease in mass loss led to reduced cross-sectional area and resulted in lower stiffness for the amended materials (Fatehi et al., 2023). This led to a drastic reduction in the compressive strength of C1 and C3 after the third cycle. Whereas, the 60 and 90-day cured C2 sample retained considerable strength in the range of 0.8–1 MPa. This can be attributed to the lower mass loss endured by C2 samples for the higher curing periods. Thus, C2 exhibited better water resistance and will survive the field conditions effectively.
The regression analysis of UCS for C1, C2, and C3 dosages is plotted in Figure 11. It shows that the strength increase in these three dosages is very similar and follows a similar trend over the increase in the curing period. The regression analysis equation has an R-value very close to one which shows that there is a strong positive correlation between the two variables of compressive strength and curing period.
The main advantages of replacing traditional stabilizers such as cement and lime with biopolymers include their higher strength achievement at much smaller dosages and lesser environmental impacts. Additionally, the loss in strength observed for the biopolymer-stabilized soil is progressive and this is evident from the higher number of cycles sustained by chitosan-stabilized samples. This can be attributed to the detachment and reattachment of fibers during the transition from the wetting cycle to the drying cycle as observed in other biopolymer-treated soils (Fatehi et al., 2023). Even though only a partial bonding is reaffirmed during the drying process for the soil-biopolymer fabric, the UCS strength retained after the successive cycles is higher in comparison to other biopolymers and chemical stabilizers (Chang et al., 2015). Though hydrophilic in nature, chitosan has exhibited comparatively higher wet strength and sustained a larger number of wetting and drying cycles compared to other biopolymers. Additionally, it can be observed from Figure 10 that the moisture variation is minimal beyond 3 cycles at 60 and 90 days of the curing period. The selection of chitosan for the present study was also based on its less hydrophilicity and its efficiency in resisting erosion-related attacks (Orts et al., 2000; Adamczuk et al., 2021).
The drip erosion test findings on untreated soft soil samples reveal that the soil has weak water resistance; it is not even able to resist the drip flow for more than a 5-min duration period, and after a 10-min exposure to water dripping, it has virtually totally disintegrated as in Figure 12. The drip erosion test when carried on soft soil treated with chitosan biopolymer performed well when compared to untreated soft soils. When compared to C1, the C2 and C3 dosages produced better results as in Figure 13. The C1 soil sample completed the 10-min testing period with only a minor modification to its regular form. The C3 and C2 performed admirably, maintaining their original shape throughout the testing, and they successfully resisted drip erosion. This is mostly attributable to the chitosan biopolymer, which increases the surfaces’ hydrophobicity and erosion resistance. The creation of a water-resistant barrier that prevents the soil particles from dispersing accounts for chitosan’s ability to efficiently block water. This barrier is created by the biopolymer’s strong hydrogen bonding and ionic interactions with the polymer chains.
FIGURE 12. Images of raw clay specimen(s) following drip erosion test (A) before the start of test (B) after 5 min of commencement of test (C) after 10 min of commencement of test.
FIGURE 13. Images of chitosan amended clay specimens following 10 min of drip erosion tests for (A) C1 (B) C2 and (C) C3.
A typical earth-lined canal of the Friant-Kern canal in California, United States as per (Byers, 1984), was considered in this study. The canal is 245 km long and is a source of livelihood for the farmland of Southern California. Due to several lining failures of the canal in 1970, they refurbished the canal using an amendment of soil-lime in an attempt to stabilize the slopes. The same section is used here to check the carbon emission of chitosan lining over the entire canal section and the operation and maintenance (O and M) roads on both sides of the canal. As seen in Figure 14, the canal depth was 6.7 m from the ground surface with a width of 17.7 m and slope of 1:2. Based on the experimental studies of durability and erodibility of soft soil using various dosages of chitosan, 2% has been used for the stabilization of clay in the canal lining and was considered for CFA analysis. The soil mixture was compacted with a water content of 23.4% to reach a uniform density of 14.51 kN/m3.
FIGURE 14. Schematic cross-sectional diagram of chitosan-treated clay canal lining considered for performing carbon footprint analysis (CFA).
The Embodied Carbon Equivalent Factors (ECFs) provided by (Hammond and Jones, 2008; Hammond et al., 2011; Ashfaq et al., 2020; Riofrio et al., 2021; Varsha et al., 2023) were utilized to calculate the embodied carbon emissions from the clay, chitosan, and water. Table 3 presents the calculated values from Phase 1. The calculations are provided in Supplementary Appendix A.
The quantification of carbon emissions while procuring and transferring the materials to the site was done. The machines used for the procurement and haulage were a pickup excavator and a heavy-duty dumper with capacities of 10-ton/lit capacity and 25-ton/lit capacity respectively. The to and fro haulage distance was considered to be 1 km for simplicity in the quantification. The ECF values for the fuel required for these vehicles were sourced from (Prusinski and Bhattacharja, 1999; Kecojevic and Komljenovic, 2011; Ashfaq et al., 2020). Table 4 lists the values of embodied carbon emissions produced by the procurement and haulage phase of the material. The factors which facilitated the emissions from this phase were the vehicle capacities, haulage distance, and type of fuel used by the vehicles.
The carbon emissions contributed by the site operations were estimated in Phase 3. A bulldozer with a 10-ton/lit capacity for spreading soil, a distributor truck with a 7,000 L capacity for spraying chitosan, a smooth wheel roller with a 12-ton/lit capacity for compacting soil in the canal base and side roads, and a slope compactor roller with a 9-ton/lit capacity for compacting soil in canal slopes were considered in the analysis. Table 5 presents the carbon emissions from Phase 3. The vehicle capacities and the number of trips influenced the overall carbon emissions in the current phase similar to Phase 2. The calculations and total carbon emissions from all three phases are reported in Supplementary Appendix A and Table 6, respectively.
To understand the sustainability of the chitosan stabilized canal lining, a comparison was made to conventional chemical stabilizers such as cement and lime. Hence the dosages adopted for the carbon emission analysis were 4% and 6% for cement and lime in Table 7 (Prusinski and Bhattacharja, 1999; Garzón et al., 2016). Phase 1 has been examined for comparison to understand the contribution of the different materials clearly. The carbon emissions with 4% cement and 6% lime contributed 45.4% and 54.4% of the total emissions, whereas chitosan contribute 0.2%, as shown in Figure 15. The ECF values for lime and cement were considered to be 0.76 and 0.95, respectively (Hammond and Jones, 2008; Hammond et al., 2011; Ashfaq et al., 2020). The excessive reliance on cement and lime has brought deteriorating impacts on the environment such as increased pH, affecting the quality of flora and fauna, and acceleration of global warming (Chang et al., 2015). A single ton of cement produces about 1 ton of CO2 (Worrell et al., 2001) and thereby needs to be replaced by materials with a lower carbon footprint (Chang et al., 2020). The present study indicated that the chitosan amendment has resulted in a reduction of carbon emissions by 8.74 and 7.44 times compared to lime and cement.
The current study focused on improving the water resistance and erodibility characteristics of chitosan-amended soft soil. The alternate wetting and drying and the erodibility performance of untreated and chitosan-treated soils led to the following conclusions.
• The unconfined compressive strength of the treated soil displayed a significant improvement of 4 MPa with extended curing periods (90 days).
• The variation in moisture content of soil samples exhibited a linear trend with chitosan dosage which is indicative of the non-polar nature of chitosan biopolymer. This affirms chitosan’s effectiveness in holding and retaining water, which further increases at higher dosages.
• Compared to C1 and C3 samples, C2 samples successfully performed well by resisting five wetting and drying cycles and successfully retaining about 22.6% of their initial dry strength. The C2 samples maintained a durability index of 20% at 60 days and 90 days curing periods after 5 wetting and drying cycles whereas C3 samples achieved a lesser durability index for the same curing periods and cycles.
• C2 and C3 samples sustained through 10 min of drip erosion test without any notable erosion and exhibited higher erosion resistance compared to C1 samples. Among the selected dosages, C2 appeared to enhance the performance of chitosan-treated soft soil in the most effective manner.
• The CFA conducted on chitosan-amended soft soil for the construction of a canal lining revealed that significant savings in terms of carbon emissions can be achieved with chitosan compared to traditional and conventional stabilizers like cement and lime.
The current study presents a practical understanding concerning the application of chitosan in erosion prevention and protection against a probable water attack for soft soils. The use of chitosan can significantly reduce the global warming potential by bringing down carbon emissions compared to the high carbon footprint contributed by cement and lime. The use of chitosan can offer an environment-friendly and sustainable approach in the field of construction and geotechnical engineering. It has exhibited considerably higher wet strength in comparison to conventional chemical stabilizers. After implementation in the field, they will be subjected to natural degradation after adequate service life. The research knowledge shared in the current study will facilitate practicing engineers to implement such novel materials by understanding the technical aspects and applicability of these technologies. The combination of chitosan with other novel materials may further enhance the survivability of the stabilized soil against harsh climatic conditions. The higher cost incurred for chitosan can be overcome by promoting its wide applicability and thereby enhancing the supply market.
The original contributions presented in the study are included in the article/Supplementary Material, further inquiries can be directed to the corresponding author.
Conceptualization, methodology, RR, and AM; formal analysis, investigation, RR; resources, AM, AA; data curation, RR, SR, and AM; writing—original draft preparation, RR, SR, AM, and AA; writing—review and editing, RR, SR, AM, AA; visualization, RR; supervision, AM; project administration, AM; funding acquisition, AA. All authors contributed to the article and approved the submitted version.
This study was supported by King Saud University, Riyadh, Saudi Arabia, Researchers Supporting Project Number 2023R279.
The authors would like to thank King Saud University, Riyadh, Saudi Arabia, Researchers Supporting Project Number 2023R279 for funding this research.
The authors declare that the research was conducted in the absence of any commercial or financial relationships that could be construed as a potential conflict of interest.
All claims expressed in this article are solely those of the authors and do not necessarily represent those of their affiliated organizations, or those of the publisher, the editors and the reviewers. Any product that may be evaluated in this article, or claim that may be made by its manufacturer, is not guaranteed or endorsed by the publisher.
The Supplementary Material for this article can be found online at: https://www.frontiersin.org/articles/10.3389/fenvs.2023.1214988/full#supplementary-material
Adamczuk, A., and Jozefaciuk, G. (2022). Impact of chitosan on the mechanical stability of soils. Molecules 27 (7), 2273. doi:10.3390/molecules27072273
Adamczuk, A., Kercheva, M., Hristova, M., and Jozefaciuk, G. (2021). Impact of chitosan on water stability and wettability of soils. Materials 14 (24), 7724. doi:10.3390/ma14247724
Aenor, A. E. (2008). UNE 41410 Bloques de tierra comprimida para muros y tabiques. Definiciones, especificaciones y métodos de ensayo, 26.
Aguilar, R., Nakamatsu, J., Ramírez, E., Elgegren, M., Ayarza, J., Kim, S., et al. (2016). The potential use of chitosan as a biopolymer additive for enhanced mechanical properties and water resistance of earthen construction. Constr. Build. Mater. 114, 625–637. doi:10.1016/j.conbuildmat.2016.03.218
Al-Mahbashi, A., Al-Shamrani, M. A., Moghal, A. A. B., and Vydehi, K. V. (2021). Correlation-based studies on resilient modulus values for fiber reinforced lime-blended clay. Int. J. Geosynth. Ground. Eng. 7 (3), 59. doi:10.1007/s40891-021-00305-7
Almajed, A., Lateef, M. A., Moghal, A. A. B., and Lemboye, K. (2021). State-of-the-art review of the applicability and challenges of microbial-induced calcite precipitation (MICP) and enzyme-induced calcite precipitation (EICP) techniques for geotechnical and geo-environmental applications. Crystals 11 (4), 370. doi:10.3390/cryst11040370
Amulya, G., Moghal, A. A. B., and Almajed, A. (2023). Sustainable binary blending for low-volume roads—reliability-based design approach and carbon footprint analysis. Materials 16, 2065. doi:10.3390/ma16052065
Amulya, G., Moghal, A. A. B., Basha, B. M., and Almajed, A. (2022). Coupled effect of granite sand and calcium lignosulphonate on the strength behavior of cohesive soil. Buildings 12 (10), 1687. doi:10.3390/buildings12101687
Ashfaq, M., Lal, M. H., Moghal, A. A. B., and Murthy, V. R. (2020). Carbon footprint analysis of coal gangue in geotechnical engineering applications. Indian Geotech. J. 50, 646–654. doi:10.1007/s40098-019-00389-z
Ashfaq, M., Moghal, A. A. B., and Almajed, A. (2022a). “Sustainability benefits of utilizing coal gangue as fill material in earthworks,” in Geo-congress, 453–462. doi:10.1061/9780784484050.047
Ashfaq, M., Moghal, A. A. B., and Basha, B. M. (2022b). The sustainable utilization of coal gangue in geotechnical and geoenvironmental applications. J. Hazard. Toxic. Radioact. Waste. 26 (3), 03122003. doi:10.1061/(ASCE)HZ.2153-5515.0000705
Ashfaq, M., Moghal, A. A. B., and Munwar Basha, B. (2021). Reliability-based design optimization of chemically stabilized coal gangue. J. Test. Eval. 51 (1), 20210176. doi:10.1520/JTE20210176
ASTM D422-63e1 (2007). Standard test method for particle-size analysis of soils. West Conshohocken, PA, USA: ASTM, 623. Available at: https://www.astm.org/d0422-63r98.html (Accessed on August 1, 2022).
ASTM D4972-19 (2019). Standard test methods for pH of soils. West Conshohocken, PA, USA: ASTM. Available at: https://www.astm.org/d2166-00.html (Accessed on August 1, 2022).
ASTM-D2166-06 (2010). Standard test method for unconfined compressive strength of cohesive soil. West Conshohocken, PA 19428-2959, USA: ASTM. Available at: https://www.astm.org/d2166-00.html (Accessed on August 1, 2022).
ASTM-D2487-06 (2017). Standard practice for classification of soils for engineering purposes (unified soil classification system). West Conshohocken, PA, USA: ASTM. Available at: https://www.astm.org/d2487-11.html (Accessed on August 1, 2022).
ASTM-D4318-17e1 (2017). Standard test methods for liquid limit, plastic limit, and plasticity index of soils. West Conshohocken, PA, USA: ASTM. Available at: https://www.astm.org/d4318-17e01.html (Accessed on August 1, 2022).
ASTM-D559-03 (2003). Standard test methods for wetting and drying compacted soil-cement mixtures. West Conshohocken, PA, USA: ASTM. Available at: https://www.astm.org/d0559-03.html (Accessed on August 1, 2022).
ASTM-D698-12 (2021). Standard test method for laboratory compaction characteristics of soil using standard effort (12,400 ft-lbf/ft3 625 (600 kN-m/m3)). West Conshohocken, PA, USA: ASTM. Available at: https://www.astm.org/d0698-12r21.html (Accessed on August 1, 2022).
ASTM-D854-14 (2016). Standard test method for specific gravity of soil solids by water pycnometer. West Conshohocken, PA, 621 USA: ASTM. Available at: https://www.astm.org/d0854-14.html (Accessed on August 1, 2022).
Badakhshan, E., Noorzad, A., and Vaunat, J. (2023). Stabilization of soft clays exposed to freeze–thaw cycles using chitosan. J. Cold. Reg. Eng. 37 (2), 04023004. doi:10.1061/jcrgei.creng-690
Byers, J. G. (1984). Treatment of expansive clay canal lining. Geotechnical Branch, Division of Research and Laboratory Services, Engineering and Research Center, US Department of the Interior, Bureau of Reclamation. Available at: https://www.usbr.gov/tsc/techreferences/research/GR8406.pdf (Accessed on April 1, 2023).
Chang, I., and Cho, G-C. (2012). Strengthening of Korean residual soil with β-1,3/1,6-glucan biopolymer. Constr. Build. Mater 30, 30–35. doi:10.1016/j.conbuildmat.2011.11.030
Chang, I., Lee, M., Tran, A. T. P., Lee, S., Kwon, Y. M., Im, J., et al. (2020). Review on biopolymer-based soil treatment (BPST) technology in geotechnical engineering practices. Transp. Geotech. 24, 100385. doi:10.1016/j.trgeo.2020.100385
Chang, I., Prasidhi, A. K., Im, J., and Cho, G. C. (2015). Soil strengthening using thermo-gelation biopolymers. Constr. Build. Mater 77, 430–438. doi:10.1016/j.conbuildmat.2014.12.116
Chittoori, B. C. S., Rahman, T., Burbank, M., and Moghal, A. A. B. (2019). “Evaluating shallow mixing protocols as application methods for microbial induced calcite precipitation targeting expansive soil treatment,” in Geo-congress 2019: Soil improvement, 250–259. doi:10.1061/9780784482117.025
Dhami, N. K., Reddy, M. S., and Mukherjee, A. (2016). Significant indicators for biomineralisation in sand of varying grain sizes. Constr. Build. Mater. 104, 198–207. doi:10.1016/j.conbuildmat.2015.12.023
Fang, H., Wenrong, H., and Yuezhong, L. (2004). Investigation of isolation and immobilization of a microbial consortium for decoloring of azo dye 4BS. Water. Res. 38 (16), 3596–3604. doi:10.1016/j.watres.2004.05.014
Fatehi, H., Ong, D. E., Yu, J., and Chang, I. (2021). Biopolymers as green binders for soil improvement in geotechnical applications: A review. Geosciences 11 (7), 291. doi:10.3390/geosciences11070291
Fatehi, H., Ong, D. E., Yu, J., and Chang, I. (2023). The effects of particle size distribution and moisture variation on mechanical strength of biopolymer-treated soil. Polymers 15 (6), 1549. doi:10.3390/polym15061549
Garzón, E., Cano, M., Okelly, B. C., and Sánchez-Soto, P. J. (2016). Effect of lime on stabilization of phyllite clays. Appl. Clay Sci. 123, 329–334. doi:10.1016/j.clay.2016.01.042
Hahn, T., Tafi, E., Paul, A., Salvia, R., Falabella, P., and Zibek, S. (2020). Current state of chitin purification and chitosan production from insects. J. Chem. Technol. Biotechnol. 95 (11), 2775–2795. doi:10.1002/jctb.6533
Hammond, G., and Jones, C. (2008). Inventory of carbon and energy: ICE; sustainable energy research team. UK: Department of Mechanical Engineering, University of Bath. Available at: https://perigordvacance.typepad.com/files/inventoryofcarbonandenergy.pdf (Accessed on April 1, 2023).
Hammond, G., Jones, C., Lowrie, E. F., and Tse, P. (2011). Embodied carbon. The inventory of carbon and energy (ICE). BSRIA BG10/2011. Available at: https://greenbuildingencyclopaedia.uk/wp-content/uploads/2014/07/Full-BSRIA-ICE-guide.pdf (Accessed on April 1, 2023).
Hataf, N., Ghadir, P., and Ranjbar, N. (2018). Investigation of soil stabilization using chitosan biopolymer. J. Clean. Prod. 170, 1493–1500. doi:10.1016/j.jclepro.2017.09.256
Jurong, B., and Siau, C. C. (2021). Estimation of strength development of cement-stabilized clayey soils with activity number, liquid limit, and apparent void ratio. Int. J. Geomech. 21 (8). doi:10.1061/(ASCE)GM.1943-5622.0002080
Kamari, A., Pulford, I. D., and Hargreaves, J. S. J. (2011). Chitosan as a potential amendment to remediate metal contaminated soil — a characterisation study. Colloids Surfaces B Biointerfaces. 82 (1), 71–80. doi:10.1016/j.colsurfb.2010.08.019
Kannan, G., and Sujatha, E. R. (2023). Crustacean polysaccharides for the geotechnical enhancement of organic silt: A clean and green alternative. Carbohydr. Polym. 299, 120227. doi:10.1016/j.carbpol.2022.120227
Kecojevic, V., and Komljenovic, D. (2011). Impact of bulldozer's engine load factor on fuel consumption, CO<sub>2</sub> emission and cost. Am. J. Environ. Sci. 7, 125–131. doi:10.3844/ajessp.2011.125.131
Knorr, D. (1991). Recovery and utilization of chitin and chitosan in food processing waste management. Food. Tech. 45, 114–122. ISSN: 0015-6639.
Latifi, N., Horpibulsuk, S., Meehan, C. L., AbdMajid, M. Z., Tahir, M. M., and Mohamad, E. T. (2016). Improvement of problematic soils with biopolymer—An environmentally friendly soil stabilizer. J. Mater Civ. Eng. 29, 04016204. doi:10.1061/(ASCE)MT.1943-5533.0001706
Li, B., Elango, J., and Wu, W. (2020). Recent advancement of molecular structure and biomaterial function of chitosan from marine organisms for pharmaceutical and nutraceutical application. Appl. Sci. 10 (14), 4719. doi:10.3390/app10144719
Liu, Y., Jia, J., Zhang, H., and Sun, S. (2022). Enhanced Cr (VI) stabilization in soil by chitosan/bentonite composites. Ecotoxicol. Environ. Saf. 238, 113573. doi:10.1016/j.ecoenv.2022.113573
Moghal, A. A. B., Ashfaq, M., Al-Obaid, A. A. K., Abbas, M. F, A., and Shaker, A. A. (2021). Compaction delay and its effect on the geotechnical properties of lime-treated semi-arid soils. Road Mater. Pavement Des. 22 (11), 2626–2640. doi:10.1080/14680629.2020.1784256
Moghal, A. A. B., Lateef, M. A., Abu Sayeed Mohammed, S., Ahmad, M., Usman, A. R., and Almajed, A. (2022a). Heavy metal immobilization studies and enhancement in geotechnical properties of cohesive soils by EICP technique. Appl. Sci. 10 (21), 7568. doi:10.3390/app10217568
Moghal, A. A. B., and Moghal, A. A. B. (2012). “Sustainable use of low lime fly ashes in geotechnical and geoenvironmental applications,” in GeoCongress 2012: State of the art and practice in geotechnical engineering, 3681–3690. doi:10.1061/9780784412121.377
Moghal, A. A. B., Mohammed, S. A. S., Almajed, A., and Al-Shamrani, M. A. (2020a). Desorption of heavy metals from lime-stabilized arid-soils using different extractants. Int. J. Civ. Eng. 18 (4), 449–461. doi:10.1007/s40999-019-00453-y
Moghal, A. A. B., Rasheed, R. M., and Mohammed, S. A. S. (2022b). Sorptive and desorptive response of divalent heavy metal ions from EICP-treated plastic fines. Ind. Geotech. J. 1-19, 315–333. doi:10.1007/s40098-022-00638-8
Moghal, A. A. B., Reddy, K. R., Mohammed, S. A. S., Shamrani, M. A. A., and Zahid, W. M. (2016). “Efficacy of lime treatment on the mercury retention characteristics of semi-arid soils,” in Geo-China. 2016, 41–48. doi:10.1061/9780784480045.006
Moghal, A. A. B., Sanaulla, P. F., Mohammed, S. A. S., and Rasheed, R. M. (2023). Leaching test protocols to evaluate contaminant response of nano-calcium silicate–treated tropical soils. J. Hazard. Toxic. Radioact. Waste. 27 (2), 04023002. doi:10.1061/JHTRBP.HZENG-1200
Moghal, A. A. B., and Sivapullaiah, P. V. (2011). “Characterization of lime and gypsum amended class F fly ashes as liner materials,” in Geo-frontiers 2011: Adv. Geotech. Eng., 1162–1171. doi:10.1061/41165(397)119
Moghal, A. A. B., Vydehi, K. V., Moghal, M. B., Almatrudi, R., Al-majed, A., and Al-Shamrani, M. A. (2020b). Effect of calcium-based derivatives on consolidation, strength, and lime-leachability behavior of expansive soil. J. Mater. Civ. Eng. 32 (4). doi:10.1061/(ASCE)MT.1943-5533.0003088
Moh, Z. C. (1962). Soil stabilization with cement and sodium additives. J. Soil. Mech. Found. Div. 88 (6), 81–105. doi:10.1061/JSFEAQ.0000478
Mohammed, S. A. S., and Moghal, A. A. B. (2016). Efficacy of nano calcium silicate (NCS) treatment on tropical soils in encapsulating heavy metal ions: Leaching studies validation. Innov. Infrastruct. Sol. 1, 21–12. doi:10.1007/s41062-016-0024-9
Mohammed, S. A. S., Moghal, A. A. B., and Lateef, M. A. (2018). “Strength characteristics of nano calcium silicate, fly ash, and lime blended tropical soils,” in Ifcee. 2018, 105–114. doi:10.1061/9780784481592.011
Moum, J., Rao, C. N., and Ayyar, T. S. R. (1973). A natural 17Å montmorillonite-organic complex from alleppey, Kerala state—India. Clays Clay Min. 21, 89–95. doi:10.1346/CCMN.1973.0210204
Nair, M. A., and Sreedharan, C. (1986). Agroforestry farming systems in the homesteads of Kerala, southern India. Agrofor. Syst. 4, 339–363. doi:10.1007/BF00048107
Orts, W. J., Sojka, R. E., and Glenn, G. M. (2000). Biopolymer additives to reduce erosion-induced soil losses during irrigation. Industrial Crops Prod. 11 (1), 19–29. doi:10.1016/S0926-6690(99)00030-8
Prusinski, J. R., and Bhattacharja, S. (1999). Effectiveness of Portland cement and lime in stabilizing clay soils. Transp. Res. Rec. 1652, 215–227. doi:10.3141/1652-28
Ramachandran, A. L., Mukherjee, A., and Dhami, N. K. (2022). Nanoscale to macroscale characterization of in—situ bacterial biopolymers for applications in soil stabilization. Front. Mater. 8, 546. doi:10.3389/fmats.2021.681850
Reddy, D. S., Kowshik, K., Kishor, M. J., Chittaranajan, M., and Sravani, E. (2018). “Investigation of chitosan bio-polymer effect on the geotechnical properties of an expansive soil,” in Proceedings of international conference on recent trends in engineering, materials, management and sciences ICRTEMMS-2018 (Accessed on June 1, 2023).
Riofrio, A., Alcivar, T., and Baykara, H. (2021). Environmental and economic viability of chitosan production in guayas-Ecuador: A robust investment and life cycle analysis. ACS omega 6 (36), 23038–23051. doi:10.1021/acsomega.1c01672
Shaker, A. A., Al-Shamrani, M. A., Moghal, A. A. B., and Vydehi, K. V. (2021). Effect of confining conditions on the hydraulic conductivity behavior of fiber-reinforced lime blended semiarid soil. Materials 14 (11), 3120. doi:10.3390/ma14113120
Sharma, M., Satyam, N., and Reddy, K. R. (2021). Effect of freeze-thaw cycles on engineering properties of bio-cemented sand under different treatment conditions. Eng. Geol. 284, 106022. doi:10.1016/j.enggeo.2021.106022
Shillaber, C. M., Mitchell, J. K., and Dove, J. E. (2016). Energy and carbon assessment of ground improvement works. II: Working model and example. J. Geotech. Geoenviron Eng. 142 (3), 04015084. doi:10.1061/(ASCE)GT.1943-5606.0001411
Shubber, A. A. M., Diogo, J. F. R., and Liu, X. Y. (2009). “Low-cost roads construction by soil stabilization using bituminous materials in Al-anbar gypseous sandy soil,” in Logistics: The emerging Frontiers of transportation and development in China, 2459–2466. doi:10.1061/40996(330)362
Sruthi, P. L., Reddy, P. H. P., and Moghal, A. A. B. (2022). Swelling behavior of alkali transformed kaolinitic clays treated with flyash and ground granulated blast furnace slag. Ind. Geotech. J. 52 (1), 145–160. doi:10.1007/s40098-020-00489-1
Strand, S. P., Vårum, K. M., and Østgaard, K. (2003). Interactions between chitosans and bacterial suspensions: Adsorption and flocculation. Colloids Surfaces B Biointerfaces. 27 (1), 71–81. doi:10.1016/S0927-7765(02)00043-7
Suganya, K., and Sivapullaiah, P. V. (2020). Compressibility of remoulded and cement-treated Kuttanad soil. Soil. Found. 60 (3), 697–704. doi:10.1016/j.sandf.2019.07.006
Suganya, K., and Sivapullaiah, P. V. (2015). Effect of changing water content on the properties of Kuttanad soil. J. Geotech. Geoenviron. Eng. 33, 913–921. doi:10.1007/s10706-015-9873-9
Suganya, K., and Sivapullaiah, P. V. (2017). Role of composition and fabric of Kuttanad clay: A geotechnical perspective. Bull. Eng. Geol. Environ. 76, 371–381. doi:10.1007/s10064-016-0911-5
Suksun, H., Runglawan, R., Avirut, C., Yuttana, R., and Apichat, S. (2010). Analysis of strength development in cement-stabilized silty clay from microstructural considerations. Constr. Build. Mater. 24 (10), 2011–2021. doi:10.1016/j.conbuildmat.2010.03.011
Syed, M., Moghal, A. A. B., and Chittoori, B. C. S. (2021). Reliability analysis of polyvinyl alcohol fiber-reinforced soft subgrade soil treated withLime and alkali activated stabilizer: A comparative study. J. Test. Eval. 51 (1). doi:10.1520/JTE20210054
Tang, C. S., Yin, L. Y., Jiang, N. J., Zhu, C., Zeng, H., Li, H., et al. (2020). Factors affecting the performance of microbial-induced carbonate precipitation (MICP) treated soil: A review. Environ. Earth. Sci. 79, 94–23. doi:10.1007/s12665-020-8840-9
Varsha, B., Moghal, A. A. B., Rehman, A. U., and Chittoori, B. C. S. (2023). Shear, consolidation characteristics and carbon footprint analysis of clayey soil blended with calcium lignosulphonate and granite sand for earthen dam application. Sustainability 15 (7), 6117. doi:10.3390/su15076117
Vydehi, K. V., and Moghal, A. A. B. (2022). Effect of biopolymeric stabilization on the strength and compressibility characteristics of cohesive soil. J. Mater. Civ. Eng. 34 (2). doi:10.1061/(ASCE)MT.1943-5533.0004068
Walker, P. (2022). “The Australian earth building handbook,” in The Australian earth building handbook SAI global limited (Australia: Standards Australia International Ltd).
Wang, S., Su, J., Wu, Z., Ma, W., Li, Y., and Hui, H. (2021). Silty clay stabilization using metakaolin-based geopolymer binder. Front. Phys. 657. doi:10.3389/fphy.2021.769786
Worrell, E., Price, L., Martin, N., Hendriks, C., and Meida, L. O. (2001). Carbon dioxide emissions from the global cement industry. Annu. Rev. Energy Env. 26, 303–329. doi:10.1146/annurev.energy.26.1.303
Yan, N., and Chen, X. (2015). Sustainability: Don't waste seafood waste. Nature 524 (7564), 155–157. doi:10.1038/524155a
Keywords: chitosan, drip erosion, durability index, unconfined compression strength, wetting and drying
Citation: Rasheed RM, Moghal AAB, Rambabu S and Almajed A (2023) Sustainable assessment and carbon footprint analysis of polysaccharide biopolymer-amended soft soil as an alternate material to canal lining. Front. Environ. Sci. 11:1214988. doi: 10.3389/fenvs.2023.1214988
Received: 09 May 2023; Accepted: 05 June 2023;
Published: 15 June 2023.
Edited by:
Yong Q. Tian, Central Michigan University, United StatesReviewed by:
Dominic E. L. Ong, Griffith University, AustraliaCopyright © 2023 Rasheed, Moghal, Rambabu and Almajed. This is an open-access article distributed under the terms of the Creative Commons Attribution License (CC BY). The use, distribution or reproduction in other forums is permitted, provided the original author(s) and the copyright owner(s) are credited and that the original publication in this journal is cited, in accordance with accepted academic practice. No use, distribution or reproduction is permitted which does not comply with these terms.
*Correspondence: Arif Ali Baig Moghal, YmFpZ0BuaXR3LmFjLmlu
Disclaimer: All claims expressed in this article are solely those of the authors and do not necessarily represent those of their affiliated organizations, or those of the publisher, the editors and the reviewers. Any product that may be evaluated in this article or claim that may be made by its manufacturer is not guaranteed or endorsed by the publisher.
Research integrity at Frontiers
Learn more about the work of our research integrity team to safeguard the quality of each article we publish.