- 1Shandong Provincial Key Laboratory of Water and Soil Conservation and Environmental Protection, College of Agriculture and Forestry Science, Linyi University, Linyi, China
- 2Philippine Christian University Center for International Education, Manila, Philippines
- 3School of Pharmacy, Linyi University, Linyi, China
- 4Linyi Academy of Agricultural Sciences, Linyi, China
The objective of this study was to investigate the effects of different rates of straw returning on soil aggregate stability, phosphatase activities, and the available nitrogen (N) and phosphorus (P) within different soil aggregate sizes. The experiment included five treatments: 1) no straw returning and no chemical fertilizer, 2) chemical fertilizer only (150 kg N ha-1, 75 kg P ha-1, and 75 kg K ha-1), 3) 20% straw returning with chemical fertilizer, 4) 60% straw returning with chemical fertilizer, and 5) 100% straw returning with chemical fertilizer. Soil samples were collected 3.5 years after the start of the experiment and separated into four aggregate sizes (<0.25 mm, 0.25–1 mm, 1–2 mm, and 2–7 mm) using the dry sieving method. Soil acid phosphomonoesterase (AcP) and alkaline phosphomonoesterase (AlP); phosphodiesterase (PD); pyrophosphatase (PrA) activities; and soil NO3−−N, NH4+−N, and resin-P were determined within soil aggregates. The results showed that straw returning rates did not significantly impact soil aggregate distribution. However, straw returning increased soil AcP, AlP, and PD in <2 mm aggregates, and high rates of straw returning led to high enzyme activities. Soil phosphatase activities were also higher in 1–2 mm aggregates. All straw returning and chemical fertilization treatments increased soil NO3−−N and resin-P concentrations but had much less effect on soil NH4+−N concentrations. Additionally, the study revealed that soil pH, the concentrations of NH4+−N, NO3−−N, resin-P, and CaCO3 significantly influenced soil phosphatase activities, but their impact varied across different sizes of aggregates.
1 Introduction
The intensive winter wheat–summer maize cropping system in the central North China Plain is known for high inputs of chemical fertilizers, inadequate organic matter, high crop yields, and large amounts of crop residues (Zhang et al., 2016; Lu et al., 2020). Traditionally, both wheat and maize straws were used as fuel for cooking or burned on the farm to clean the field for planting the next crop. Since the on-farm straw burning practice was banned in 2008, a large quantity of straw must be incorporated into the soil. Straw returning has been shown to increase soil organic matter (SOM) and available soil nutrients (Tan et al., 2015), improve soil porosity and structure (Yao et al., 2015), reduce soil bulk density, and enhance soil enzyme activities (Wang et al., 2018). However, the straw return was challenging due to the lack of appropriate field equipment in some hilly areas, where straws are currently removed from fields. As a consequence, intensive farming has led to soil quality degradation (i.e., lack of SOM, low soil fertility, poor nutrient use, subsequently low yield, and degraded soil structure) (Liu and Diamond, 2005), which greatly influences the long-term productive capacity of the soil (Vitousek et al., 2019).
Soil aggregates are vital for soil structure and fertility (Mrquez et al., 2019), and the associated soil characteristics within and between aggregates are essential for soil quality (Lehmann et al., 2017). Straw returning has been shown to increase the proportion of soil macroaggregates larger than 0.25 mm (Alidad et al., 2012; Zhang et al., 2018; Zhao et al., 2018; Cao et al., 2021). Additionally, the distribution of soil aggregates and the mean weight diameter (MWD) also differed from the straw returning mode (Ma et al., 2020). However, the effects of straw returning on phosphorus (P) distribution among soil aggregates remain unclear. For instance, soil P could be enriched in soil macroaggregates or microaggregates due to different soil types (Ahmed et al., 2017). Studies have shown that 80% of soil total P was contained in soil macroaggregates (>2 mm) (Zhang et al., 2021), while soil total P or available P concentrations were highest in <0.053 mm soil aggregates (Cheng et al., 2019; Deng et al., 2021). Additionally, different sizes of soil aggregates also affect P loss from soil (Garland et al., 2018; Li et al., 2020; Cao et al., 2021). Therefore, the input of exogenous organic materials can potentially impact the structure of soil aggregates and P distribution among aggregates.
Phosphatase enzymes are responsible for mineralization of organic matter to release phosphate ions (H2PO4− and HPO42–) in soil (Criquet and Braud, 2008). Soil acid phosphomonoesterase (AcP), alkaline phosphomonoesterase (AlP), phosphodiesterase (PD), and pyrophosphatase (PrA) are specific enzymes that facilitate this process. Studies have shown that straw returning significantly increased soil alkaline phosphatase activity (Wang et al., 2018), and phosphatase activity decreased with decreasing aggregate size (Tian et al., 2022), but the effect of straw returning on soil phosphatase activities may not be long term (Stegarescu et al., 2021). Furthermore, the turnover of nutrients is closely related to soil aggregates and soil structure (Six et al., 2000; Galantini et al., 2004) because soil aggregates can provide physical protection for nutrients from microbial decomposition (Six et al., 2004; Barthès et al., 2008), and the availability of nutrients would conversely influence phosphatase activities. Thus, straw returning may cause a difference in soil phosphatase activities and P availability among soil aggregates.
The existing research on soil aggregates following straw returning to the field has mainly focused on water-stable aggregates (Meng et al., 2014; Garbuz et al., 2016). Although studies have primarily centered on soil carbon status among aggregates (Wang et al., 2015; He et al., 2018; Wang et al., 2018), few have investigated the concentration of available P and phosphatase activities after straw returning to the field. The study was conducted in a typical Yimeng mountainous area in Northern China, where cinnamon soil (Calcaric Cambisol, FAO) accounts for up to 50% of the land area. Straw returning is not common practice in this area, and the soils often suffer from erosion and low P nutrients. The objective of this study was to determine the effects of straw returning on the structure and internal available P and other nutrients and phosphatase activities of soil aggregates in a calcareous soil under a maize–wheat cropping system.
2 Materials and methods
2.1 Site description and experimental design
The field experiment was conducted on cinnamon soil (Calcaric Cambisols in FAO classification) at Fei County (117°54′ E and 35°13′ N), Shandong Province, Northern China. The region has a temperate continental monsoon climate; the annual mean temperature and precipitation are 14.1°C and 849 mm, respectively; and 60%–80% of the annual precipitation occurs during the maize-growing season in summer. The initial soil properties in the study area were 8.05 pH, 1.12% TC, 0.16% TN, and 548 mg kg-1 TP. Winter wheat was planted in early or mid-October with a seeder and harvested in early June of the following year. Then, summer maize was planted after the wheat harvest and harvested in early October. Five treatments were as follows: 1) CK (control - no fertilizer and no straw), 2) C (chemical fertilizer only), 3) SR20 (20% straw returning with chemical fertilizer), 4) SR60 (60% straw returning with chemical fertilizer), and 5) SR100 (100% amount of straw returning with chemical fertilizer). The chemical fertilizer treatment consisted of 150 kg N ha-1 urea and with about 5.48 kg N from NH4H2PO4, 45 kg P ha-1 as NH4H2PO4, and 45 kg K ha-1 as KCl. Each plot was 3 m × 4 m in size with a 1-m buffer zone between plots. All treatments were replicated four times, except CK (three times due to limited experimental area), and plots were arranged according to a single-factor, completely randomized experiment design. Prior to the initiation of this experiment, no straw returning was performed to the soil in this field previously. For this study, only maize straw was returned to the field, and the amount of the maize straw that was returned based on the average straw weight (8,700 kg/ha dry weight) in the field. The nutrient contents of straws returned to the fields were 687 g TC kg-1, 9.16 g TN kg-1, and 2.83 g TP kg-1. Maize straw was chopped into 1-cm-long pieces and disked into 0–20 cm soil layer.
2.2 Soil sampling
Intact soil core samples were collected from each plot by pushing a plastic frame (10 cm long, 10 cm wide, and 20 cm deep) into the soil after the wheat harvest, 3.5 years after the initiation of the experiment. After being transported to the laboratory, the soil cores were gently broken into small clods according to the natural texture and dried at 4 °C in a refrigerated cabinet until the soil water content was around 8%. After being sieved through a 7-mm sieve, soil aggregates were measured with the dry screening method (Zhang et al., 2013). The dry sieving method was chosen in our study as aggregates divided by the wet-sieving method can hardly be used for the determination of soil nutrient contents and soil phosphatase activities. A sub-sample was sieved (<2 mm) and stored at 4 °C for analysis of enzyme activities within a week. Another subsample was air-dried, sieved, and analyzed for basic soil properties.
2.3 Soil aggregate fractionation measurement
Cold air-dried soil samples (100 g) were agitated by a mechanical shaker (OA SS203, Ortoalresa, Spain) at 50 Hz frequency for 2 min on a stack of sieves (2–7 mm, 1–2 mm, 0.25–1 mm, and <0.25 mm) until soil aggregate samples were enough for further analysis. The aforementioned procedure was repeated several times. All soil aggregate samples were weighed and stored at 4 °C until further analysis. The MWD of soil aggregates was calculated using the following formula (Kemper and Rosenau, 1986):
where Xi is the mean diameter (mm) and Wi is the weight proportion of each size fraction.
2.4 Soil properties
Soil pH was measured using a glass electrode (soil/water ratio of 1.0:2.5). Soil carbonate content was measured with a sieved air-dried sample (<100 mesh) using the pressure calcimeter method: 1.0 g of soil and 2 mL of 6 M HCl containing 3% by weight of FeCl2·4H2O are placed at the bottom of a 100-mL sealing reaction vessel; the acid was mixed with soil by turning the vessel sideways; an 18-gauge hypodermic needle was inserted, which is attached to the pressure transducer and voltage meter, the results were recorded; and then CO2 concentration was calculated using a calibration curve to obtain carbonate content (Loeppert and Suarez, 1996). Soil organic carbon (SOC) content was measured with sieved air-dried soil (<100 mesh)) using the dichromate oxidation method, and SOM content was calculated based on OM = 1.724 × SOC (Nelson and Sommers, 1996).
Soil available nitrogen (N) and P concentrations were determined for all grades of soil aggregates. Ammonium N and nitrate N concentrations were determined by the colorimetric method after being extracted with 1: 5 2 M KCl solutions (Mulvaney, 1996). The content of available P in soil was determined by the anionic exchange resin strips combined with molybdenum blue method, and the blue color was detected at 700 nm using the Agilent Cary 100 UV–Vis spectrophotometer (Sharpley, 2000).
2.5 Soil phosphatase assay
Soil acid phosphomonoesterase activities (EC 3.1.3.2, AcP), alkaline phosphomonoesterase activities (EC 3.1.3.1, AlP), and the phosphodiesterase activities (PD) were determined by the method of Tabatabai et al. (1994). Briefly, soil acid phosphomonoesterase activities were determined as follows: a fresh soil sample (1.0 g) was mixed with 1 mL of 50 mM disodium phenyl phosphate in 4 mL of modified universal buffer at pH 6.5 and 0.2 mL of toluene before incubating for 1 h at 37 °C. The alkaline phosphomonoesterase activities were determined using the same procedure, except the universal buffer pH was adjusted to 11. Universal buffer was prepared by mixing 12.1 g of Tris (hydroxymethyl) aminomethane, 11.6 g of succinic acid, 14.0 g of citric acid, and 6.3 g of boric acid in 488 mL of sodium hydroxide solution [C (NaOH) = 1 mol L-1], then diluting to 1 L, and storing at low temperature for future use. Modified universal buffer can be obtained by diluting the universal buffer from 200 mL to 1 L and modifying it to the needed pH of 6.5 for AcP and 11.0 for AlP. After incubation, 1 mL 0.5 mol L-1 CaCl2 and 4 mL mol L-1 NaOH were added to terminate the reaction. Phosphodiesterase activities (PD) were determined by incubating 1.0 g fresh soil with 1 mL of 50 mM sodium bis (p-nitrophenyl) phosphate and 4 mL of pH 8.0 buffer prepared by dissolving 6.1 g of Tris-base, adjusting to pH 8.0 with sulfuric acid solution, and then fixing the volume to 1 L. To stop phosphodiesterase activities, 1 mL 0.5 mol L-1 CaCl2 and 4 mL of CaCl2-Tris (hydroxymethyl aminomethane) were used. Fluorescence produced by the aforementioned enzymatic reactions was measured colorimetrically at 410 nm using the Agilent Cary 100 UV–Vis spectrophotometer. For the aforementioned enzyme assays, controls were included for each soil sample. Pyrophosphatase activities (EC 3.6.1.1, PrA) were also assayed following the method of Tabatabai et al. (1994). Fresh soil (1.0 g) was mixed with 3 mL of 50 mM sodium pyrophosphate, and after incubating for 1 h at 37°C, 25 mL of 0.5 M H2SO4 and 3 mL of pH 8.0 modified universal buffer (the same universal buffer as phosphomonoester activities modified with pH 8.0) were added and shook using a horizontal oscillator for 3 min, and then centrifuged to obtain the supernatant. About 2 mL of the supernatants was taken in 50-mL volumetric flasks, the developers were added, the volume fixed, and the color compared at 700 nm using the UV–Vis spectrophotometer (Agilent, Santa Clara, CA) after 15 min of color development.
2.6 Statistical analyses
The differences among treatments or grades were tested by one-way ANOVA using SPSS 16.0 (SPSS Inc., Chicago, Ill., United States). Mean separation was determined using Tukey’s or Dunnett’s T3 test based on the results of homogeneity of variances (p-value < 0.05). A detrended correspondence analysis (DCA) was applied, and the lengths of the gradients were less than 3. Then, a redundancy analysis (RDA) was used to identify the effects of soil properties on soil phosphatase activities among treatments and soil aggregates using Canoco Software 4.5 (Microcomputer Power, Ithaca, NY, United States). The soil factors were standardized by error variance when RDA was performed. All soil properties included in the RDA analysis were D standardized as Z-scores to remove the unit’s influence. Soil phosphatase activity scores were “divided by standard deviation,” soil phosphatase activity data chose the “do not transform” item, soil samples were “standardized by norm,” and the phosphatase activities were “standardized by error variance” when RDA was carried out. The Monte Carlo permutation test (999 permutations) was used to select factors that significantly influenced soil phosphatase activities. Figures were prepared using SigmaPlot 10 (SYSTAT, Point Richmond, CA, United States).
3 Results
3.1 Effect of different rates of maize straw returning on soil pH, SOM, carbonate, and soil aggregate distribution
Compared to the use of chemical fertilizer alone, the maximum straw returning treatment (SR100) significantly increased SOM by 19% and reduced carbonate concentration by 51% (Table 1). The second highest straw returning treatment (SR60) significantly reduced carbonate concentration but did not significantly increase SOM. The low rate of straw returning (SR20) neither significantly affected the contents of organic matter nor those of carbonate. In addition, no significant changes in soil pH were found among treatments.
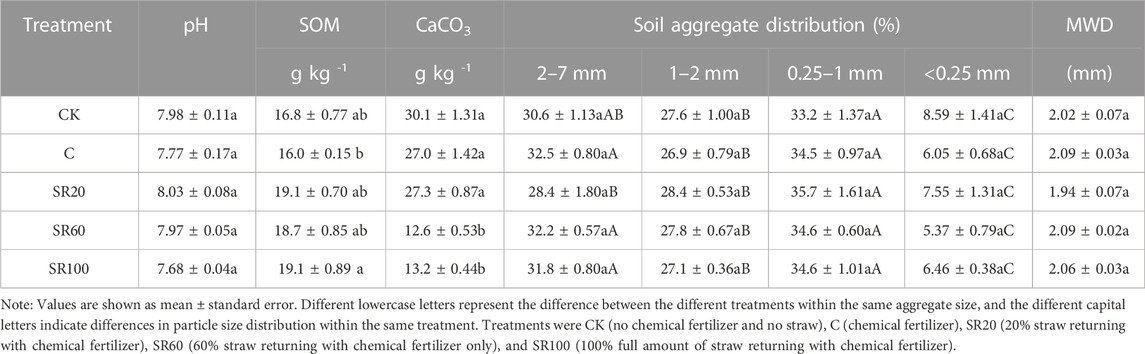
TABLE 1. Effects of different rates of maize straw returning on soil chemical properties, aggregate distribution, and MWD.
The soil aggregates in the CK sample were almost equally distributed among small macroaggregates (33.2%, 0.25–1 mm), medium macroaggregates (27.6%, 1–2 mm), and large macroaggregates (30.6%, 2–7 mm); the microaggregates (<0.25 mm) were relatively small (8.6%). As for 2–7 mm aggregates, results showed that SR20 treatment significantly decreased the proportion of 2–7 mm aggregates compared to other treatments. Except for that, no significant differences were observed among treatments within the same size of aggregate. In addition, different treatments did not affect the soil MWD.
3.2 Effects of straw returning rate on the available N, P concentration among soil aggregates
All sizes of soil aggregates under CK treatment had significantly lower NO3−−N concentrations than those of other treatments, while medium macroaggregates (1–2 mm) showed significantly higher NO3−−N concentrations than those of other sizes of aggregates under the same treatments (Figure 1A). The high concentrations of NH4+−N were found in microaggregates (<0.25 mm), while the low concentrations were from medium macroaggregates (1–2 mm) for all treatments (Figure 1B). The NH4+−N concentration in <0.25 mm soil aggregates showed that SR100 treatment had the highest and SR20 treatment had the lowest NH4+−N contents, significantly different with C and CK treatments. For 0.25–1 mm aggregates, only the SR100 treatment had significantly higher NH4+−N contents than the other treatments. No significant difference was observed for NH4+−N contents associated with 1–2 mm aggregates among treatments. For 2–7 mm aggregates, the CK sample had the highest NH4+−N contents, which were significantly higher than those of the SR20 and SR100 treatments. Under the same treatment, NH4+−N contents ordinarily followed the sequence from high to low as < 0.25 mm > 2–7 mm > 0.25–1 mm > 1–2 mm. Only the SR20 treatment showed that NH4+−N was significantly high in 2–7 mm, followed by < 0.25 mm and 1–2 mm, and at last, 1–2 mm.
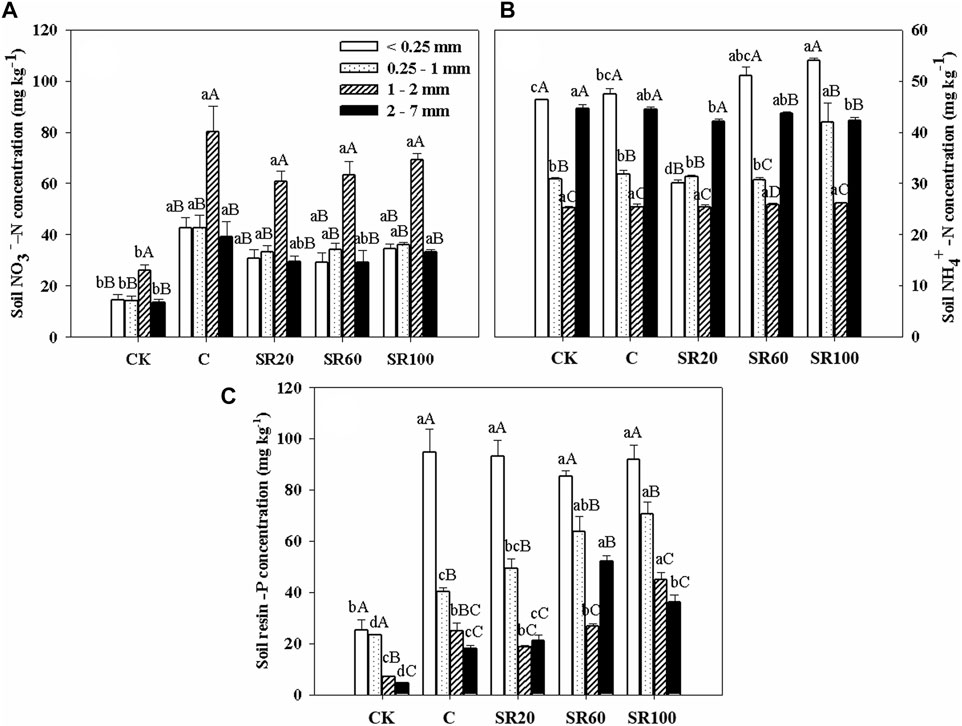
FIGURE 1. Soil aggregate-associated available NO3−−N (A), NH4+−N (B), and phosphorus (C) under different treatments [CK (no chemical fertilizer and no straw), C (chemical fertilizer only), SR20 (20% straw returning with chemical fertilizer), SR60 (60% straw returning with chemical fertilizer), and SR100 (100% full amount of straw returning with chemical fertilizer)]. Values are shown as the mean ± standard error. Different lowercase letters represent the difference among different treatments within the same aggregate size, and different capital letters indicate the difference among the grain levels of the same processing different aggregates.
The order of resin-P concentration was <0.25 mm aggregates >0.25–1 mm aggregates > 1–2 mm aggregates = 2–7 mm aggregates under CK, C, SR20, and SR100 treatments (Figure 1C). Only SR60 treatment showed that resin-P in 2–7 mm aggregates was significantly higher than that in 1–2 mm aggregates. Within the same aggregate, no clear trend was shown among treatments, but resin-P followed the order of SR100 > SR60 > SR20 > C > CK from high to low in 0.25–1 mm and 1–2 mm aggregates. Furthermore, the higher returning rate of straw had positive effects on soil resin-P concentration.
3.3 Effects of maize straw returning rate on soil aggregate-associated AcP, AlP, PD, and PrA
As shown in Figure 2A, AcP in <0.25 mm microaggregates under CK and SR20 treatments was significantly lower than those under SR100 and SR60 treatments. Within 0.25–1 mm aggregates, only SR100 treatment significantly increased AcP. As for 1–2 mm aggregates, SR20 treatment had the lowest AcP, which was significantly lower than that in SR100. For AcP in 2–7 mm aggregates, there was no significant difference among treatments. As seen from CK treatment, 1–2 mm aggregates showed the highest AcP, whereas SR60 treatment showed the highest AcP in both 1–2 mm and <0.25 mm aggregates. The rest of the treatments showed that <0.25 mm aggregates had the highest AcP. All treatments showed that aggregates of 0.25–1 mm had the lowest AcP.
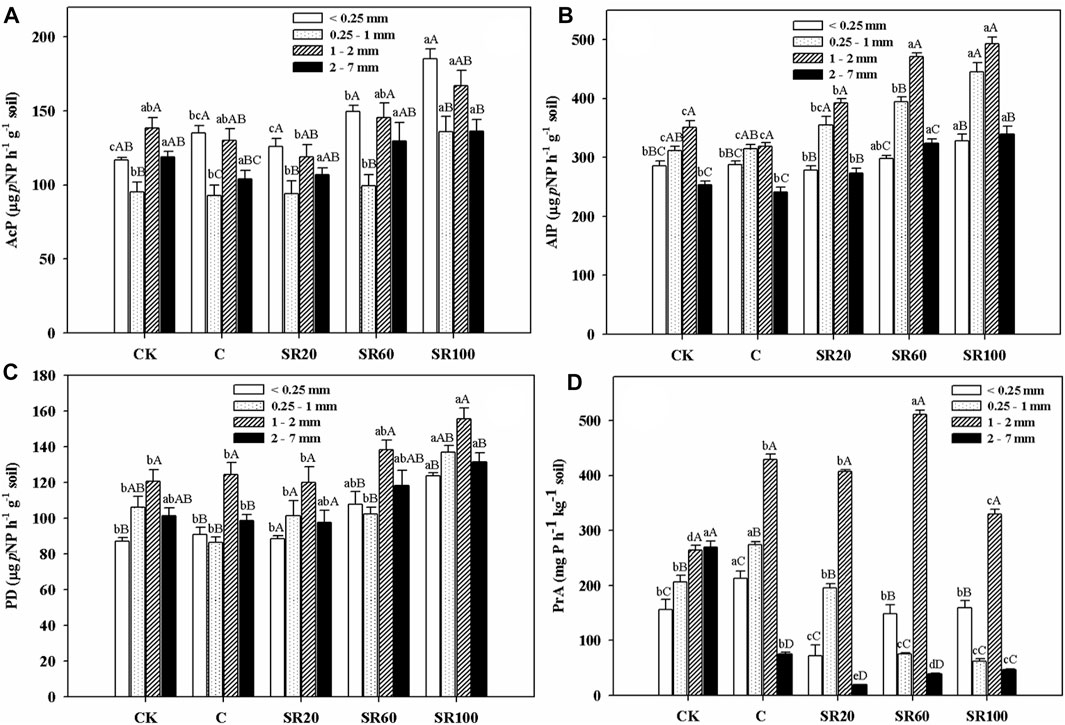
FIGURE 2. Soil aggregate-associated AcP (A), AlP (B), PD (C) and PrA (D) under different treatments [CK (no chemical fertilizer and no straw), C (chemical fertilizer only), SR20 (20% straw returning with chemical fertilizer), SR60 (60% straw returning with chemical fertilizer), and SR100 (100% full amount of straw returning with chemical fertilizer)]. Values are shown as mean ± standard error. Different lowercase letters represent the difference among the different treatments within the same aggregate size, and the different capital letters indicate the difference among the grain levels of the same processing different aggregates.
Soil AlP was higher in 1–2 mm aggregates than in 2–7 mm aggregates under C and CK treatments (Figure 2B). Straw returning increased soil AlP associated with 1–2 mm aggregates, while only SR100 and SR60 treatments increased AlP associated with 0.25–1 mm and 2–7 mm aggregates. As for AlP within <0.25 mm aggregates, only SR100 had higher AlP than CK and C treatments. The soil AlP associated with 1–2 mm and 0.25–1 mm aggregates were higher than that associated with <0.25 mm and 2–7 mm aggregates under SR100, SR60, and SR20 treatments, while soil AlP within 1–2 mm aggregates were even more higher than within 0.25–1 mm aggregates under SR60 treatment. Furthermore, soil AlP in 1–2 mm aggregates was only higher than that in 2–7 mm aggregates under CK and C treatments.
As shown in Figure 2C, PD of <0.25 mm, 0.25–1 mm, and 1–2 mm aggregates under SR100 treatment was significantly higher than that under C, CK, and SR20 treatments. Soil PD in 2–7 mm aggregates under SR100 treatment was significantly higher than that under C treatment, and there was no significant difference compared with other treatments. Considering the same treatment, PD under SR20 treatment had no significant difference among aggregates. Under other four treatments, 1–2 mm aggregates had higher PD than other aggregates, and <0.25 mm or 0.25–1 mm showed the lowest value.
Unlike other soil phosphatase activities, soil PrA was significantly affected by treatments and among aggregates (Figure 2D). Soil PrA associated with <0.25 mm and 0.25–1 mm aggregates was significantly higher under C treatment than under other treatments. Soil PrA in 0.25–1 mm aggregates was highest under SR60 treatment, followed by C and SR20 treatments, and then SR100, and CK treatments. It is worth noting that CK treatment had the highest PrA in 2–7 mm aggregates, followed by C, SR100, SR60, and SR20 treatments. Under the same treatment, soil macroaggregates had higher PrA than microaggregates.
3.4 Multivariate analysis of soil phosphatase activities and soil properties
Redundancy analysis was conducted to investigate the relationships between soil phosphatase activities in different soil aggregates. In aggregates <0.25 mm, soil phosphatase activities were significantly related to soil available nitrogen (N) concentration, soil organic matter (SOM), and carbonate contents (Figure 3A). The soil properties explained 77.5% of the variation in soil phosphatase activities (F = 12.3, P = 0.001), with the first (RDA1) and second (RDA2) axes explaining 57.0% and 66.3% of the accumulated variation in soil phosphatase activities, respectively. The RDA1 explained 73.6% of the variation in the relationship between the soil phosphatase activities and soil properties (F = 18.1, P = 0.001), while the RDA2 explained 98.4% of the accumulated variation (F = 20.6, P = 0.001).
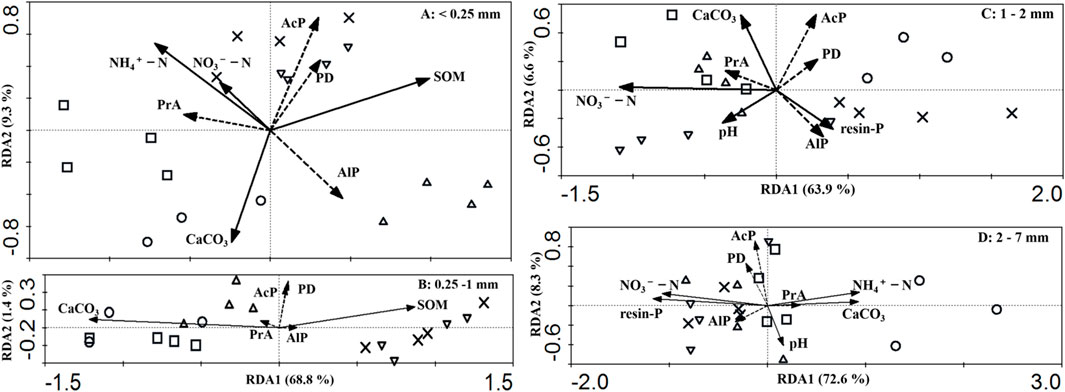
FIGURE 3. Redundancy analysis of ordination triplot of soil phosphatase activities in different soil aggregates. Samples are displayed as points, and soil variables are shown as arrows. The direction of the arrow shows the increase in environmental factors: CK (○)—no chemical fertilizer and no straw; C (□)—chemical fertilizer only; SR20 (△) - 20% straw returning with chemical fertilizer; SR60 (▽)—60% straw returning with chemical fertilizer; and SR100 (×)—100% full amount of straw returning with chemical fertilizer.
In aggregates 0.25–1 mm, soil phosphatase activities were significantly related to SOM and carbonate contents (Figure 3B). The soil properties explained 70.2% of the variation in soil phosphatase activities (F = 21.5, P = 0.001), with the first (RDA1) and second axes (RDA2) explaining 68.8% and 70.2% of the accumulated variation in soil phosphatase activities, respectively. The RDA1 explained 98% of the variation in the relationship between the soil phosphatase activities and soil properties (F = 40.6, P = 0.001), while the RDA2 explained 100% of the accumulated variation (F = 2.09, P = 0.312).
In 1–2 mm aggregates, soil phosphatase activities were significantly related to NO3−−N, resin-P, CaCO3, and pH (Figure 3C). The soil properties explained 73.4% of the variation in soil phosphatase activities (F = 9.68, P = 0.001), with the RDA1 and RDA2 axes explaining 63.9% and 70.5% of the accumulated variation in soil phosphatase activities, respectively. The RDA1 explained 87.1% of the variation in the relationship between the soil phosphatase activities and soil properties (F = 24.9, P = 0.001), while the RDA2 explained 96% of the accumulated variation (F = 6.28, P = 0.111).
In 2–7 mm aggregates, soil phosphatase activities were significantly related to NO3−−N, resin-P, CaCO3 contents, and pH (Figure 3D). The soil properties explained 81.3% of the variation in soil phosphatase activities (F = 10.5, P = 0.001), with the RDA1 and RDA2 explaining 72.6% and 80.9% of the accumulated variation in soil phosphatase activities, respectively. The RDA1 explained 89.3% of the variation in the relationship between the soil phosphatase activities and soil properties (F = 29.9, P = 0.001), while the RDA2 explained 99.4% of the accumulated variation in the aforementioned relationship (F = 11.5, P = 0.001).
4 Discussion
4.1 Soil pH, SOM, and carbonate concentration
Unlike previous studies that found returning straw could decrease (Sahrawat, 2005; Bai et al., 2013; Cheng et al., 2023) or increase (Wang et al., 2013) soil pH, this study showed no significant change in soil pH during 3.5 years of the experiment. This could be attributed to the high soil-buffering capacity due to the high concentration of CaCO3 in the soil (Wang et al., 2013; Zamanian and Kuzyakov, 2019). Straw returning treatments with high percentages (60% and 100%) led to a reduction of approximately 50% in carbonate concentration within 3.5 years. Continuation of the straw returning practice might eventually neutralize all or most carbonate, leading to a reduction in soil pH. The increase in soil organic matter (SOM) resulting from straw returning may take longer and require larger amounts of straw. Although many studies reported an increase in SOM (0–20 cm soil depth) due to straw returning (Ma et al., 2020), significant results may take several years to emerge, as reported by Zhao et al. (2018) who observed a positive influence on SOC after 7 years. Chemical fertilization, mainly with N inputs, might cause reduction in SOM (Zhao et al., 2018), likely the same situation as observed under the C treatment. Additionally, straw returning can lead to priming effects, increasing microbial activity and causing SOM depletion (Fang et al., 2018). The decrease in CaCO3 content in SR60 and SR100 can be attributed to two reasons. First, organic acids released during the decomposition of the high rate of straw residue (Sahrawat, 2005; Cao et al., 2021) through root exudates and microbial respiration (Sahrawat, 2005; Wang et al., 2013) can contribute to the decrease. Second, the high rate of straw returning treatments can improve soil water permeability (Alidad et al., 2012; Gorokhova and Chursin, 2021), and the presence of H2O and CO2 could accelerate CaCO3 dissolution (Gorokhova and Chursin, 2021).
4.2 Soil aggregate distribution and associated available N and P concentrations
The mean weight diameter (MWD), an important index for assessing soil aggregate size distribution, can reveal the physical structure of soil and reflect soil quality (Ma et al., 2020). Previous studies have shown that straw returning increased MWD (Huang et al., 2017; Ma et al., 2020; Cao et al., 2021), but we found no significant change in MWD in our study, likely due to the use of the dry sieving method, which differs from the wet sieving method used in previous studies. As seen in Table 1, 2–7 mm soil aggregates were increased by the C, SR60, and SR100 treatments, but not the SR20 treatment. High rates of straw returning increased the proportion of >2 mm soil aggregates, consistent with previous reports (Wang et al., 2015; Ma et al., 2020), showing that fresh SOC can stimulate microbial activity to produce soil binders (Six et al., 2000), so it is easy for soil aggregate agglomeration. The C treatment also increased the proportion of >2 mm soil aggregates, which may be caused by the same reason, but the carbon source for microbial activity came from the depletion of soil original SOM. The low proportion of >2 mm soil aggregates under SR20 treatment showed high microbial activity but inadequate soil fresh carbon input.
The concentration of NO3−−N within all sizes of aggregates in C and straw returning treatments was higher than that in the CK treatment, likely due to the chemical fertilization of N (Nagatake et al., 2018). Furthermore, straw returning could increase soil cation exchange capacity (Cheng et al., 2023) and decrease N leaching in soil (Yang et al., 2016), which could also increase NO3− adsorption to soil aggregates and NO3−−N concentration. Our study also found that within the same treatment, NO3− was strongly associated with 1–2 mm soil aggregates.
Previous studies have suggested that straw returning could increase soil N availability due to N input from straw (Wang et al., 2018), immobilizing soil mineral N, and releasing N from straw decomposition (Takahashi et al., 2003; Thuy et al., 2008). However, few studies have investigated NO3−−N and NH4+−N distribution among different soil aggregates. We found that the SR100 treatment significantly increased NH4+−N concentration in <0.25 mm and 0.25–1 mm aggregates. The reasons might be that the SR100 treatment increased soil organic carbon content, which decreased NH4+ adsorption to the soil caused by reduced exchange sites for NH4+ (Zhang et al., 2022). All treatments showed that NH4+−N concentrations were higher in <0.25 mm and 2–7 mm aggregates but lower in 0.25–1 mm and 1–2 mm aggregates. Soil aggregates of <0.25 mm showed the highest NH4+−N concentration, likely due to their large surface area, which has been reported in previous studies to accumulate nutrients (Adesodun et al., 2007; Wang W. et al., 2011; Mitran et al., 2018). NH4+−N concentration was also high in 2–7 mm aggregates, possibly due to CaCO3 content shown by RDA analysis (Figure 3D). CaCO3 content has been reported to have positive effects on soil macroaggregates (Ge et al., 2019), which provide physical protection for soil organic carbon content from microbial decomposition (Wei et al., 2013), which was proved to be important for NH4+ retention in soil (Baldock and Nelson, 2000).
The application of chemical fertilizer alone or combined with straw returning was shown to increase soil available P due to P release from the fertilizer (Wang W. et al., 2011; Ahmed et al., 2017) or organic matter mineralization (Chen et al., 2018; Cheng et al., 2019; Cao et al., 2021). Previous studies reported that a high rate of straw returning has positive effects on soil available P in aggregates, indicating that more nutrients are contained in straw (Ma et al., 2020). This study found that resin-P concentrations generally increased as aggregate size decreased, which is consistent with previous findings and might be caused by the increasing surface area with decreasing size of aggregates (Mitran et al., 2018; Cheng et al., 2019).
4.3 Soil aggregate-associated phosphatase activities
Phosphatase activities play a crucial role in soil P availability. This study found that straw returning had positive treatment effects on soil acid phosphomonoesterase activities (AcP) in aggregates <2 mm and alkaline phosphomonoesterase activities (AlP) and phosphodiesterase activities (PD) in all aggregates. These findings were consistent with those of previous studies, which suggested that the increasing substrate availability caused by straw returning (Li et al., 2022), and cumulative effects on soil enzyme activities from annual straw incorporation, might contribute to the significant effects of a high rate of straw returning (Wang et al., 2018; Li et al., 2022). The high AcP, AlP, and PD in 1–2 mm aggregates indicate those aggregates provide a more suitable environment for microbial population, and soil enzymes due to the suitable environment probably had higher phosphomonoesterase and phosphodiesterase activities.
Pyrophosphatase in soil plays a role in catalyzing pyrophosphate to orthophosphate (Tabatabai et al., 1994). Pyrophosphatase activities (PrA) are negatively related to soil pH (Tabatabai and Dick, 1979), but no significance of soil pH was found in this study. The study also found that the activities of pyrophosphatase are closely related to substrate (pyrophosphate) content, which correlated positively with microbial P (Reitzel and Turner, 2014), especially with soil fungal communities (Bünemann et al., 2004; Makarov et al., 2005; Koukol et al., 2008). The previous studies showed that straw returning affects fungal communities, resulting in more fungal communities under medium and high levels of straw returning (Wang et al., 2021). The high activities of PrA in 1–2 mm aggregates under C, SR20, SR60, and SR100 treatments might show more fungal microbial biomass, which provided more pyrophosphate as substrates.
4.4 Relationships among straw returning, soil properties, soil aggregates, and soil phosphatase activities
Only four significant factors could influence <0.25 mm soil aggregates associated with phosphatase activities. Soil AcP, AlP, and PD positively related to soil organic matter, and PrA positively correlated with NH4+−N and NO3−−N concentration. Soil AcP, AlP, and PD positively related to soil organic carbon, which had been found previously (Saha et al., 2008; Wang J. B. et al., 2011; Wei et al., 2015) due to substrate effect and more favorable environmental factors for enzymes (Zhang et al., 2012; Zhang et al., 2016).
For 0.25–1 mm soil aggregates associated with phosphatase activities, only SOM and CaCO3 contents significantly influenced enzyme activities. SOM positively influences AlP, PrA, and soil CaCO3. Soil organic carbon increasing phosphatase activities were extensively reported (Tabatabai and Dick, 1979; Saha et al., 2008; Wang J. B. et al., 2011; Wei et al., 2015). Unlike the report of soil CaCO3 negatively related to soil PrA (Tabatabai and Dick, 1979), this study found a positive relationship between CaCO3 and PrA (also in 1–2 and 2–7 mm aggregates, Figures 3C, D). This was possible because the soil pH in this study still ranged in the optimum pH for soil PrA (Dick and Tabatabai, 1978), which covered the effect of CaCO3.
Soil pH, NO3−−N, resin-P, and CaCO3 were considered significant factors influencing soil phosphatase activities in 1–2 mm aggregates. Soil pH negatively correlated with soil AcP and PD, while soil AlP was attributed to soil resin-P concentration. Through the data analysis, the study found that alkaline phosphomonoesterase was a determining factor for P availability in 1–2 mm aggregates in calcareous soils and a high rate of straw returning had higher alkaline phosphatase activities.
The study found that soil pH, NH4+−N, NO3−−N, resin-P, and CaCO3 are significant factors that influence soil phosphatase activities in 2–7 mm aggregates. Similar to those in 1–2 mm aggregates, soil pH negatively correlated with soil AcP and PD, while soil AlP positively related to soil resin-P and NO3−−N concentration. NH4+−N and CaCO3 had a positive effect on soil PrA. Soil available nitrogen concentration positively correlated with soil PrA or soil AlP, except for 0.25–1 mm aggregates. This can be explained by soil biota secreting more enzymes to enhance the supply of N and P when soil nutrition is limited (Zhang et al., 2012). Soil pH was considered to have a negative effect on soil PrA, but the effect would be weak in neutral and alkaline soil (Tabatabai and Dick, 1979); thus, we found a slight influence between soil pH and PrA (Figures 3C, D). In addition, the soil pH in our study had no difference among treatments.
5 Conclusion
Straw returning with chemical fertilizer could increase soil phosphatase activities, and a high rate of straw returning treatment could be more desirable. The phosphatase activities within <0.25 mm, 0.25–1 mm, 1–2 mm, and 2–7 mm aggregates were influenced differently by soil properties under different treatments, with significant factors including soil pH, NH4+−N, NO3−−N, resin-P, SOM, and CaCO3. These factors can be classified into 1) SOM, which influences the substrate of phosphatase, and 2) soil pH, available N and P, and CaCO3, which influence the environment and soil biota. More research should be conducted on straw returning to calcareous soils, especially on soil aggregates and their associated enzyme activities, as they have not been extensively studied.
In conclusion, the short term (3.5 years) of maize straw returning to the field had no significant influence on the calcareous soil aggregate distribution. However, a high rate of straw returning significantly increased SOM and activities of soil enzymes (acid phosphomonoesterase, alkaline phosphomonoesterase, and phosphodiesterase). The soil pyrophosphatase activities showed a different trend from the other three enzymes and were significantly influenced by soil available N and CaCO3 concentration. Therefore, a high rate of straw returning is recommended to improve soil nutrition and phosphatase activities in calcareous soils.
Data availability statement
The original contributions presented in the study are included in the article/Supplementary Material; further inquiries can be directed to the corresponding authors.
Author contributions
X-JL: conceptualization, data curation, and original draft preparation. G-NZ: design of methodology and supervision. ZW: writing—review and editing. Q-DH: validation. PL: resources and supervision. All authors contributed to the article and approved the submitted version.
Conflict of interest
The authors declare that the research was conducted in the absence of any commercial or financial relationships that could be construed as a potential conflict of interest.
Publisher’s note
All claims expressed in this article are solely those of the authors and do not necessarily represent those of their affiliated organizations, or those of the publisher, the editors, and the reviewers. Any product that may be evaluated in this article, or claim that may be made by its manufacturer, is not guaranteed or endorsed by the publisher.
References
Adesodun, J. K., Adeyemi, E. F., and Oyegoke, C. O. (2007). Distribution of nutrient elements within water stable aggregates of two tropical agro-ecological soils under different land uses. Soil Tillage Res. 92, 190–197. doi:10.1016/j.still.2006.03.003
Ahmed, E. H., Anjum, S. I., and Zhang, M. K. (2017). Effects of fertilization on phosphorus distribution in water-stable aggregates of soils with different properties. Toxicol. Environ. Chem. 99 (1), 32–47. doi:10.1080/02772248.2016.1172584
Alidad, K., Mehdi, H., Sadegh, A., Hassan, R., and Sanaz, B. (2012). Organic resource management: Impacts on soil aggregate stability and other soil physico-chemical properties. Agric. Ecosyst. Environ. 148, 22–28. doi:10.1016/j.agee.2011.10.021
Bai, Y., Gu, C., Tao, T., Chen, G., and Shan, Y. (2013). Straw incorporation increases solubility and uptake of cadmium by rice plants. Acta Agric. Scand. Sect. B Soil Plant Sci. 63, 193–199. doi:10.1080/09064710.2012.743582
Baldock, J. A., and Nelson, P. N. (2000). “Soil organic matter,” in Handbook of soil science. Editor M. E. Sumner (Boca Raton, USA: CRC Press).
Barthès, B. G., Kouakoua, E., Larré-Larrouy, M. C., Razafimbelo, T. M., de Luca, E. F., Azontonde, A., et al. (2008). Texture and sesquioxide effects on water-stable aggregates and organic matter in some tropical soils. Geoderma 143 (1), 14–25. doi:10.1016/j.geoderma.2007.10.003
Bünemann, E. K., Smernik, R. J., Marschner, P., and McNeill, A. M. (2004). Microbial synthesis of organic and condensed forms of phosphorus in acid and calcareous soils. Soil Biol. biochem. 40, 932–946. doi:10.1016/j.soilbio.2007.11.012
Cao, D. Y., Lan, Y., Sun, Q., Yang, X., Chen, W. F., Meng, J., et al. (2021). Maize straw and its biochar affect phosphorus distribution in soil aggregates and are beneficial for improving phosphorus availability along the soil profile. Eur. J. Soil Sci. 72 (5), 2165–2179. doi:10.1111/ejss.13095
Chen, Y., Camps-Arbestain, M., Shen, Q., Singh, B., and Cayuela, M. L. (2018). The long-term role of organic amendments in building soil nutrient fertility: A meta-analysis and review. Nutr. Cycl. Agroecosyst. 111, 103–125. doi:10.1007/s10705-017-9903-5
Cheng, R., Da, P. G., Tian, Q. B., Yan, Q. G., Xi, W. S., and Li, Y. G. (2023). Straw return alleviates the negative effects of saline sodic stress on rice by improving soil chemistry and reducing the accumulation of sodium ions in rice leaves. Agri. Ecosyst. Environ. 342, 108253. doi:10.1016/j.agee.2022.108253
Cheng, Z. B., Chen, Y., Gale, W. J., and Zhang, F. H. (2019). Inorganic phosphorus distribution in soil aggregates under different cropping patterns in northwest China. J. Soil Sci. 19, 157–165. doi:10.1007/s42729-019-00022-1
Criquet, S., and Braud, A. (2008). Effects of organic and mineral amendments on available P and phosphatase activities in a degraded Mediterranean soil under short-term incubation experiment. Soil Tillage Res. 98, 164–174. doi:10.1016/j.still.2007.11.001
Deng, X., Xu, T. L., Dong, W. W., Zhang, Q., and Liang, Y. J. (2021). Distribution of organic phosphorus in soil aggregates from apple-pear Orchard of China. Eurasian Soil Sci. 54 (1), 72–79. doi:10.1134/s1064229321010038
Dick, W. A., and Tabatabai, M. A. (1978). Inorganic pyrophosphatase activity of soils. Soil Biol. biochem. 10, 58–65. doi:10.1016/0038-0717(78)90011-1
Fang, Y., Nazaries, L., Singh, B. K., and Singh, B. P. (2018). Microbial mechanisms of carbon priming effects revealed during the interaction of crop residue and nutrient inputs in contrasting soils. Glob. Chang. Biol. 24, 2775–2790. doi:10.1111/gcb.14154
Galantini, J. A., Senesi, N., Brunetti, G., and Rosell, R. (2004). Influence of texture on organic matter distribution and quality and nitrogen and sulphur status in semiarid Pampean grassland soils of Argentina. Geoderma 123 (1), 143–152. doi:10.1016/j.geoderma.2004.02.008
Garbuz, S. A., Yaroslavtseva, N. V., and Kholodov, V. A. (2016). Enzymatic activity inside and outside of water-stable aggregates in soils under different land use. Eurasian Soil Sci. 49 (3), 367–375. doi:10.1134/s1064229316030030
Garland, G., Bünemann, E. K., Oberson, A., Frossard, E., Snapp, S., Chikowo, R., et al. (2018). Phosphorus cycling within soil aggregate fractions of a highly weathered tropical soil: A conceptual model. Soil Biol. biochem. 116, 91–98. doi:10.1016/j.soilbio.2017.10.007
Ge, N., Wei, X. R., Xiang, W. C., Liu, X. T., Shao, M. G., Jia, X. X., et al. (2019). Soil texture determines the distribution of aggregate-associated carbon, nitrogen and phosphorous under two contrasting land use types in the Loess Plateau. Catena 172, 148–157. doi:10.1016/j.catena.2018.08.021
Gorokhova, N., and Chursin, I. N. (2021). Carbonates in irrigated soils of the Caspian depression. Arid. Ecosyst. 11, 193–199. doi:10.1134/s2079096121020062
He, Y. T., He, X. H., Xu, M. G., Zhang, W. J., Yang, X. Y., and Huang, S. M. (2018). Long-term fertilization increases soil organic carbon and alters its chemical composition in three wheat-maize cropping sites across central and south China. Soil Tillage Res. 177, 79–87. doi:10.1016/j.still.2017.11.018
Huang, R., Lan, M. L., Liu, J., and Gao, M. (2017). Soil aggregate and organic carbon distribution at dry land soil and paddy soil: The role of different straws returning. Environ. Sci. Pollut. Res. 24, 27942–27952. doi:10.1007/s11356-017-0372-9
Kemper, W. D., and Rosenau, R. C. (1986). “Aggregate stability and size distribution,” in Methods of soil analysis. Part 1. Physical and mineralogical methods. Agronomy monograph. Editor A. Klute 2nd ed. (Madison, USA: American Society of Agronomy and Soil Science Society of America).
Koukol, O., Novák, F., and Hrabal, R. (2008). Composition of the organic phosphorus fraction in basidiocarps of saprotrophic and mycorrhizal fungi. Soil Biol. biochem. 40, 2464–2467. doi:10.1016/j.soilbio.2008.04.021
Lehmann, A., Leifheit, E. F., and Rillig, M. C. (2017). “Mycorrhizas and soil aggregation,” in Mycorrhizal mediation of soil. Editors N. C. Johnson, C. Gehring, and J. Jansa (Amsterdam, Netherlands: Elsevier).
Li, F. Y., Liang, X. Q., Li, H., Jin, Y. B., Jin, J. W., He, M. M., et al. (2020). Enhanced soil aggregate stability limits colloidal phosphorus loss potentials in agricultural systems. Environ. Sci. Eur. 32, 17. doi:10.1186/s12302-020-0299-5
Li, X. D., Mao, N., Jiang, H., Jiang, X. Y., Li, C., Gong, X., et al. (2022). Synergistic effects of straw and earthworm addition on microbial diversity and microbial nutrient limitation in a subtropical conservation farming system. Soil Tillage Res. 224, 105500. doi:10.1016/j.still.2022.105500
Liu, J., and Diamond, J. (2005). China's environment in a globalizing world. Nature 435 (7046), 1179–1186. doi:10.1038/4351179a
Loeppert, R. H., and Suarez, G. L. (1996). “Carbonates and gypsum,” in Methods of soil analysis part 3 chemical methods. Editors D. L. Sparks, A. L. Page, and P. A. Helmke (Madison, USA: American Society of Agronomy and Soil Science Society of America).
Lu, H., Hu, L., Zheng, W., Yao, S., and Qian, L. (2020). Impact of household land endowment and environmental cognition on the willingness to implement straw incorporation in China. J. Clean. Prod. 262, 121479. doi:10.1016/j.jclepro.2020.121479
Ma, S. T., Kan, Z. R., Qi, J. Y., and Zhang, H. (2020). Effects of straw return mode on soil aggregates and associated carbon in the North China Plain. Agronomy 10, 61. doi:10.3390/agronomy10010061
Makarov, M. I., Haumaier, L., Zech, W., Marfenina, O. E., and Lysak, L. V. (2005). Can 31P NMR spectroscopy be used to indicate the origins of soil organic phosphates? Soil Biol. biochem. 37, 15–25. doi:10.1016/j.soilbio.2004.07.022
Meng, Q. F., Sun, Y. T., Zhao, J., Zhou, L. R., Ma, X. F., Zhou, M., et al. (2014). Distribution of carbon and nitrogen in water-stable aggregates and soil stability under long-term manure application in solonetzic soils of the Songnen plain, northeast China. J. Soils Sediments 14 (6), 1041–1049. doi:10.1007/s11368-014-0859-7
Mitran, T., Mani, P. K., Bandyopadhyay, P. K., and Basak, N. (2018). Effects of organic amendments on soil physical attributes and aggregate-associated phosphorus under long-term rice-wheat cropping. Pedosphere 28, 823–832. doi:10.1016/s1002-0160(17)60423-5
Mrquez, C. O., García, V. J., Schultz, R. C., and Isenhart, T. M. (2019). A conceptual framework to study soil aggregate dynamics. Eur. J. Soil Sci. 70 (3), 466–479. doi:10.1111/ejss.12775
Mulvaney, R. L. (1996). “Nitrogen-inorganic forms,” in Methods of soil analysis part 3 chemical methods. Editors D. L. Sparks, A. L. Page, and P. A. Helmke (Madison, USA: American Society of Agronomy and Soil Science Society of America).
Nagatake, A., Mukumbuta, I., Yasuda, K., Shimizu, M., Kawai, M., and Hatano, R. (2018). Temporal dynamics of nitrous oxide emission and nitrate leaching in renovated grassland with repeated application of manure and/or chemical fertilizer. Atmosphere 9, 485. doi:10.3390/atmos9120485
Nelson, D. W., and Sommers, L. E. (1996). “Total carbon, organic carbon, and organic matter,” in Methods of soil analysis part 3 chemical methods. Editors D. L. Sparks, A. L. Page, and P. A. Helmke (Madison, USA: American Society of Agronomy and Soil Science Society of America).
Reitzel, K., and Turner, B. (2014). Quantification of pyrophosphate in soil solution by pyrophosphatase hydrolysis. Soil Biol. biochem. 74, 95–97. doi:10.1016/j.soilbio.2014.03.001
Saha, S. V., Prakash, S., Kundu, N., Kumar, B. L., Eur, J., and Mina, B. L. (2008). Soil enzymatic activity as affected by long term application of farm yard manure and mineral fertilizer under a rainfed soybean–wheat system in N-W Himalaya. Eur. J. Soil Biol. 44, 309–315. doi:10.1016/j.ejsobi.2008.02.004
Sahrawat, K. L. (2005). Fertility and organic matter in submerged rice soils. Curr. Sci. 88, 735–739.
Sharpley, A. (2000). “Bioavailable phosphorus in soil,” in Methods of phosphorus analysis for soils, sediments, residuals and waters. Editor G. M. Pierzynski (North Carolina, USA: North Carolina State University).
Six, J., Bossuyt, H., Degryze, S., and Denef, K. (2004). A history of research on the link between (micro) aggregates, soil biota, and soil organic matter dynamics. Soil Tillage Res. 79 (1), 7–31. doi:10.1016/j.still.2004.03.008
Six, J., Elliott, E. T., and Paustian, K. (2000). Soil macroaggregate turnover and microaggregate formation: A mechanism for C sequestration under no-tillage agriculture. Soil Biol. biochem. 32, 2099–2103. doi:10.1016/s0038-0717(00)00179-6
Stegarescu, G., Reintam, E., and Tõnutare, T. (2021). Cover crop residues effect on soil structural stability and phosphatase activity. Acta Agr. Scand. B-S p. 71 (9), 992–1005. doi:10.1080/09064710.2021.1973083
Tabatabai, M. A., Angel, S., Bottomley, P., Bezdicek, D., Smith, S., Tabatabai, A., et al. (1994). “Soil enzymes,” in Method of soil analysis. Editors S. H. Mickelson, and J. M. Bigham (Madison, USA: American Society of Agronomy and Soil Science Society of America).
Tabatabai, M. A., and Dick, W. A. (1979). Distribution and stability of pyrophosphatase in soils. Soil Biol. biochem. 11, 655–659. doi:10.1016/0038-0717(79)90035-x
Takahashi, S., Uenosono, S., and Ono, S. (2003). Short- and long-term effects of rice straw application on nitrogen uptake by crops and nitrogen mineralization under flooded and upland conditions. Plant Soil 251, 291–301. doi:10.1023/a:1023006304935
Tan, C. J., Cao, X., Yuan, S., Wang, W. Y., Feng, Y. Z., and Qiao, B. (2015). Effects of long-term conservation tillage on soil nutrients in sloping fields in regions characterized by water and wind erosion. Sci. Rep. 5, 17592. doi:10.1038/srep17592
Thuy, N. H., Shan, Y. H., Bija, S., Wang, K. R., Cai, Z. C., Yadvinder, S., et al. (2008). Nitrogen supply in rice-based cropping systems as affected by crop residue management. Soil Sci. Soc. Am. J. 72, 514–523. doi:10.2136/sssaj2006.0403
Tian, S. Y., Wang, M. W., Jiang, Y. J., Zhang, C. Z., Li, D. M., Chen, X. Y., et al. (2022). Organic fertilization promotes crop productivity through changes in soil aggregation. Soil Biol. biochem. 165, 108533. doi:10.1016/j.soilbio.2021.108533
Vitousek, P. M., Naylor, R., Crews, T., David, M. B., Drinkwater, L. E., Holland, E., et al. (2019). Nutrient imbalances in agricultural development. Science 324 (5934), 1519–1520. doi:10.1126/science.1170261
Wang, E. Z., Lin, X. L., Tian, L., Wang, X. G., Li, J., Jin, F., et al. (2021). Effects of short-term rice straw return on the soil microbial community. Agriculture 11, 561. doi:10.3390/agriculture11060561
Wang, J. B., Chen, Z. H., Chen, L. J., Zhu, A. N., and Wu, Z. J. (2011a). Surface soil phosphorus and phosphatase activities affected by tillage and crop residue input amounts. Plant Soil Environ. 57, 251–257. doi:10.17221/437/2010-pse
Wang, W., Chen, W. C., Wang, K. R., Xie, X. L., Yin, C. M., and Chen, A. L. (2011b). Effects of long-term fertilization on the distribution of carbon, nitrogen and phosphorus in water-stable aggregates in paddy soil. Agril. Sci. China 10, 1932–1940. doi:10.1016/s1671-2927(11)60194-6
Wang, X. J., Jia, Z. K., Liang, L. Y., Yang, B. P., Ding, R. X., Nie, J. F., et al. (2015). Maize straw effects on soil aggregation and other properties in arid land. Soil Tillage Res. 153, 131–136. doi:10.1016/j.still.2015.05.001
Wang, X. J., Jia, Z. K., Liang, L. Y., Zhao, Y. F., Yang, B. P., Ding, R. X., et al. (2018). Changes in soil characteristics and maize yield under straw returning system in dryland farming. Field Crop Res. 218, 11–17. doi:10.1016/j.fcr.2017.12.003
Wang, Y., Tang, C., Wu, J., Liu, X., and Xu, J. (2013). Impact of organic matter addition on pH change of paddy soils. J. Soils Sediments 13, 12–23. doi:10.1007/s11368-012-0578-x
Wei, T., Zhang, P., Wang, K., Ding, R., Yang, B., Nie, J., et al. (2015). Effects of wheat straw incorporation on the availability of soil nutrients and enzyme activities in semiarid areas. PLoS One 10, e0120994. doi:10.1371/journal.pone.0120994
Wei, X., Li, X., Jia, X., and Shao, M. (2013). Accumulation of soil organic carbon in aggregates after afforestation on abandoned farmland. Biol. Fertil. Soils 49, 637–646. doi:10.1007/s00374-012-0754-6
Yang, H. S., Xu, M. M., Koide, R. T., Liu, Q., Dai, Y. J., Liu, L., et al. (2016). Effects of ditch-buried straw return on water percolation, nitrogen leaching and crop yields in a rice-wheat rotation system. J. Sci. Food Agr. 96, 1141–1149. doi:10.1002/jsfa.7196
Yao, S., Teng, X., and Zhang, B. (2015). Effects of rice straw incorporation and tillage depth on soil puddlability and mechanical properties during rice growth period. Soil Tillage Res. 146, 125–132. doi:10.1016/j.still.2014.10.007
Zamanian, K., and Kuzyakov, Y. (2019). Contribution of soil inorganic carbon to atmospheric CO2: More important than previously thought. Glob. Change Biol. 25, e1–e3. doi:10.1111/gcb.14463
Zhang, A. M., Chen, Z. H., Zhang, G. N., Chen, L. J., and Wu, Z. J. (2012). Soil phosphorus composition determined by 31P NMR spectroscopy and relative phosphatase activities influenced by land use. Eur. J. Soil Biol. 52, 73–77. doi:10.1016/j.ejsobi.2012.07.001
Zhang, P., Chen, X., Wei, T., Yang, Z., Jia, Z., Yang, B., et al. (2016). Effects of straw incorporation on the soil nutrient contents, enzyme activities, and crop yield in a semiarid region of China. Soil Tillage Res. 160, 65–72. doi:10.1016/j.still.2016.02.006
Zhang, S. X., Li, Q., Lü, Y., Zhang, X. P., and Liang, W. J. (2013). Contributions of soil biota to C sequestration varied with aggregate fractions under different tillage systems. Soil Biol. biochem. 62, 147–156. doi:10.1016/j.soilbio.2013.03.023
Zhang, W. Z., Chen, X. Q., Wang, H. Y., Wei, W. X., and Zhou, J. M. (2022). Long-term straw return influenced ammonium ion retention at the soil aggregate scale in an Anthrosol with rice-wheat rotations in China. J. Integr. Agr. 21, 521–531. doi:10.1016/s2095-3119(20)63592-4
Zhang, X. F., Xin, X. L., Zhu, A. N., Yang, W. L., Zhang, J. B., Ding, S. J., et al. (2018). Linking macroaggregation to soil microbial community and organic carbon accumulation under different tillage and residue managements. Soil Tillage Res. 178, 99–107. doi:10.1016/j.still.2017.12.020
Zhang, Y. Q., Dalal, R. C., Bhattacharyya, R., Meyer, G., Wang, P., Menzies, N. W., et al. (2021). Effect of long-term no-tillage and nitrogen fertilization on phosphorus distribution in bulk soil and aggregates of a Vertisol. Soil Tillage Res. 205, 104760. doi:10.1016/j.still.2020.104760
Keywords: straw returning, soil aggregate, phosphomonoesterase, phosphodiesterase, pyrophosphatase
Citation: Lin X-J, Zhang G-N, Wang Z, Han Q-D and Leng P (2023) Phosphatase activities and available nutrients in soil aggregates affected by straw returning to a calcareous soil under the maize–wheat cropping system. Front. Environ. Sci. 11:1208323. doi: 10.3389/fenvs.2023.1208323
Received: 19 April 2023; Accepted: 29 June 2023;
Published: 27 July 2023.
Edited by:
Kaibo Wang, Chinese Academy of Sciences (CAS), ChinaReviewed by:
Aliza Pradhan, National Institute of Abiotic Stress Management (ICAR), IndiaThioro Fall, University of Florida, United States
Copyright © 2023 Lin, Zhang, Wang, Han and Leng. This is an open-access article distributed under the terms of the Creative Commons Attribution License (CC BY). The use, distribution or reproduction in other forums is permitted, provided the original author(s) and the copyright owner(s) are credited and that the original publication in this journal is cited, in accordance with accepted academic practice. No use, distribution or reproduction is permitted which does not comply with these terms.
*Correspondence: Guang-Na Zhang, gnzhang@lyu.edu.cn; Zhen Wang, wangzhen@lyu.edu.cn