- 1State Key Laboratory of Desert and Oasis Ecology, Xinjiang Institute of Ecology and Geography, Chinese Academy of Sciences (CAS), Ürümqi, China
- 2University of Chinese Academy of Sciences, Beijing, China
- 3College of Agronomy, Heilongjiang Bayi Agricultural University, Daqing, China
Soil salinization threatening natural and agricultural production challenges global food security. Halophytes are of great interest in soil desalinization in recent years; yet, there is a lack of a comprehensive quantitative overview of biotic and abiotic factors for halophytes’ desalinization performance across global scales. Here, a meta-analysis was conducted using 400 observations from 53 peer-reviewed studies to assess desalinization by halophytes in relation to 27 variables. Results showed that soil salinity was significantly decreased in halophytes field on average by 37.7% compared to control on a global scale (p < 0.05). Desalinization performance was better in cold and hot regions than in temperate regions, in dry regions than in wet regions, in alkaline saline soils than in neutral saline soils, and in conditions with low sand content than high sand content. Under aboveground harvest treatment, desalinization increased with the years of cultivation, while no trends were detected under no harvest treatment, indicating the importance of aboveground accumulation. Desalinization was not related to soil CaCO3 content but was accompanied by soil structure improvement, nutrition enrichment, and microbe propagation, implying other root-microbe-soil interactions rather than CaCO3 dissolution play important roles. Shoot biomass could be used as an indicator of the desalinization performance, and the performance would not be decreased due to the high uptake selectivity for K+ over Na+. Notably, desalinization was similar in the pot experiments and field experiments, but pot experiments would magnify the contribution of aboveground salt accumulation to desalinization. Our findings can help to expand the applicability and efficiency of halophytes for sustainable agricultural development in saline soils.
1 Introduction
The global population is expected to reach 9.7 billion by 2050, and it is estimated that agricultural production should increase by 70%–110% to meet global food demand (Tilman et al., 2011; FAO, 2021). Soil salinization, exacerbated by climate change and intensive land usage, impacts both natural and agricultural production and challenges global food security (Panta et al., 2014; Jesus et al., 2015; Eswar et al., 2021). It affects an area of more than 1 billion hectares in over 100 countries all over the world. Worse still, the amount of salt-affected land expanded continuously each year at a rate of approximately 1–2 million ha of land or even more (Qadir et al., 2014; Ivushkin et al., 2019; Hopmans et al., 2021), which means 50% of arable lands will be lost by the mid-21st century (Mahajan and Tuteja, 2005). Obviously, there is a contradiction between food security and accelerated soil salinization worldwide which puts an urgent of saline land utilization and remediation (Khanom, 2016; Litalien and Zeeb, 2020; Hopmans et al., 2021).
Owing to the limitation of fresh water resources, the improvement and utilization of saline soil gradually changed from conventional physical and chemical measures to comprehensive utilization of halophytes (Rozema and Flowers, 2008; Nouri et al., 2017; Litalien and Zeeb, 2020). Halophytes are naturally evolved salt-tolerant plants that are widely distributed in a variety of saline habitats, from coastal areas and salt marshes to drylands, providing a good perspective of saline agriculture (Rabhi et al., 2010a; Rozema and Schat, 2013; Panta et al., 2014; Nikalje et al., 2018; Barcia-Piedras et al., 2019). There has recently been an increased interest for halophytes in soil phyto-desalinization, particularly in developing countries, producing economic benefits while limiting soil erosion and environmental disturbance (Ravindran et al., 2007; Rabhi et al., 2010a; Barcia-Piedras et al., 2019). Halophytes are also considered to be an ideal alternative to glycophytes hyperaccumulators for phytoremediation of heavy metals pollution in contaminated saline soils, where most glycophytes cannot survive (Manousaki and Kalogerakis, 2011; Anjum et al., 2014; Wang et al., 2014).
However, there are still many concerns about this technology, including its universality, the influence of cultivated practice, and the possible mechanisms of desalinization (Shabala, 2013; Jesus et al., 2015). The performance can be influenced by environmental conditions, which directly or indirectly result in different patterns of water-salt migration (Rajkumar et al., 2013; Jesus et al., 2015; Eswar et al., 2021), and the differences may consequently affect the performance of halophytes desalinization. For example, in arid areas with scarce precipitation but strong evaporation, soil salinization is mainly due to the upward force driving the salt accumulation on the soil surface, while in humid and coastal areas the driving force of salinization may be related to seawater movements (Ketabchi et al., 2016; Khanom, 2016; Pauw et al., 2017; Eswar et al., 2021). Cultivated practices are important for subsequent crop cultivation, of which the desalinization duration is of great concern because the progress may take longer than traditional desalinization techniques, for it depends on the rate of ion extraction of halophytes and the improvement in physical-water properties due to the presence of root systems (Rabhi et al., 2010a; Shabala, 2013; Jesus et al., 2018). Halophytes selection will affect the desalinized soil layer because of the root growth and distribution within soil layers, which may also affect subsequent crop root development and yields (Barrett-Lennard, 2002; Rabhi et al., 2010a; Liang and Shi, 2021). In addition to the factors affecting the performance of halophytes mentioned above, the possible mechanisms of halophytes desalinization need clarifying (Shabala, 2013; Jesus et al., 2015; Jesus et al., 2018; Litalien and Zeeb, 2020). Debate on the main mechanism contributing to soil remediation has yet to be settled, including aboveground salt accumulation and root-microbe-soil interactions which improve soil properties leading to salt leaching (Qadir et al., 2000; Rabhi et al., 2009; Rasouli et al., 2013). The mechanisms are crucial to improve the phytoremediation-beneficial traits to enlarge the applicability and effectiveness of halophytes desalinization.
So far, there are several narrative reviews on the tolerance of halophytes and the theoretical frameworks of possible mechanisms of their soil desalinization (Kronzucker and Britto, 2011; Rabhi et al., 2015; Nikalje et al., 2018; Hopmans et al., 2021), while all of the above concerns about halophytes desalinization performance need further clarification. Meta-analysis is a statistical method for quantitatively synthesizing the findings of previous studies, compared with traditional narrative reviews, providing more objective conclusions (Hedges et al., 1999; Liao et al., 2012; Leifheit et al., 2014). Since it was introduced into ecology in the 1990s, it has become an important means for summarizing evidence across studies to reach general conclusions (Liao et al., 2012; Leifheit et al., 2014; Alvarez et al., 2017; Song et al., 2019; Beyene et al., 2022), to find the main reasons for the inconsistent results between studies (Liao et al., 2012; Wang et al., 2021; Beyene et al., 2022; Hu et al., 2023), to uncover the underlying mechanisms (Wang et al., 2021; Beyene et al., 2022; Feng et al., 2022), and to find the existing problems and research prospects in the field (Leifheit et al., 2014; Alvarez et al., 2017; Song et al., 2019; Hu et al., 2023). It can be well revealed by meta-analysis for the general rule of the effect of plant growth such as cultivation (Alvarez et al., 2017; Hu et al., 2023), plantation (Liao et al., 2012; Wang et al., 2021), and non-native plant invasion (Beyene et al., 2022) on soil properties and its influencing factors and underlying ecological mechanisms. Here, we use a meta-analysis aimed to assess halophytes desalinization performance on a global scale and its influencing biotic and abiotic factors, and the desalinization mechanisms. The following aspects were analyzed in detail: 1) the relationships between desalinization by halophytes and their growing environment including climate and soil physical and chemical properties; 2) cultivated practices that would influence subsequent crops cultivation such as duration of halophytes planting and lifeforms selection influencing desalinization within different soil layers; 3) the potential mechanism of aboveground and underground parts leading to desalinization. We expect these results would help to better understand and expand the applicability and efficiency of halophytes in ongoing saline agriculture.
2 Materials and methods
2.1 Data compilation
Peer-reviewed publications were collected from January 1990 to September 2022 by searching Web of Science [included 1) WoS Core Collection, 2) BIOSIS Previews, 3) Inspec, 4) Chinese Science Citation Database, 5) Derwent Innovations Index, 6) KCI-Korean Journal Database, 7) MEDLINE and 8) SciELO Citation Index] with a search of (*halophyt*) AND (*desalin* OR *restorat* OR *remediat* OR *recla$m* OR *ameliorat* OR salt remov* OR “soil propert*” OR “electrical conductivity” OR soil salt content OR “soil salinity” OR “total dissolved solids”) (topic). A total of 2,885 publications were collected and we excluded those titles that showed reviews or meta-analysis or conducted in aquatic ecosystems. Then articles were screened by the following criteria: 1) studies needed to have a control, including bare land with no halophytes or pre-soil salinity condition, but excluding that used bulk soil as a control for rhizosphere soil; 2) studies needed to report indicators that can reflect soil salinity. With the criteria mentioned above, we found that studies on soil salinity measurements are relatively rare compared with aboveground measurements. Plus 4 pieces of literature obtained from the review, this analysis finally included 53 studies. A total of 400 trials were obtained by the following criteria: 1) different species studied within each publication were treated as parallel experiments that generated different trials; 2) multiple trials within each publication obtained from different chosen factors were treated as independent (Lajeunesse, 2011; Leifheit et al., 2014); 3) additional treatments such as straw mulching, chemical amendments addition during the experiment share a same control with halophytes field were not independent and thus excluded; 4) multiple trials were not independent when they were measured in time sequence and shared a same before planted control, and thus the measures of the last time sequence were selected (Lajeunesse, 2011; Chen et al., 2013; Leifheit et al., 2014).
Apart from soil salinity data (salt content, electrical conductivity, total dissolved solids), soluble Na+, K+, Ca2+, Mg2+, Cl−, SO42- and HCO3− content, concentrations of Zn, Cu, Cd, and Pb, climatic data (mean annual air temperature, MAT, aridity index, AI, mean annual precipitation, MAP, potential evapotranspiration, PET), soil properties (bulk density, BD, sand content, CaCO3 content, pH value, soil organic carbon, SOC, total nitrogen, TN, available phosphorus, AP, and available potassium, AK, soil microbial biomass, MB), plant growth (biomass, shoot Na+, K+, and Cl− content and the amount of Na+ and Cl− removed by shoot), duration and setting of experiment, harvest or not, soil layers, and lifeforms (annual and perennial) were collected. If the author did not record climate data, then they were obtained from the studies at the same site, otherwise, MAT, MAP, and PET were obtained from the CRU TS database produced by the National Centre for Atmospheric Science (NCAS) (version 4.06, https://crudata.uea.ac.uk/cru/data/hrg/), and AI was calculated by the formula
2.2 Data analysis
Meta-analysis was conducted in the statistical software MetaWin v. 2.1. The effects of halophytes cultivation on desalinization and other soil properties were evaluated through natural logarithm response ratio (LnRR), which allows combining different units of a variable and is commonly employed in ecology (Hedges et al., 1999; Alvarez et al., 2017; Beyene et al., 2022). For each observation, the LnRR was calculated as (Hedges et al., 1999):
with a variation (v) of:
where Xt and Xc are the mean values of soil properties in the halophytes planted group and in control, respectively. st and sc are the standard deviations (SD), and nt and nc represent the number of samples. Standard error (SE) of the variables was transformed to SD by the formula
We assumed that a random effect model would fit our data set better based on the hypothesis that effect sizes did not share the same true value across all studies (Gurevitch and Hedges, 1999; Hedges et al., 1999). Then between-experiment variance (τ2) was calculated (Hedges et al., 1999):
then the weighting factor for each LnRR datapoint was re-calculated as:
and the overall weighted natural logarithm response ratio (LnRR++) of desalinization to halophytes plantation was computed by:
Their corresponding confidence intervals (CIs) were produced by bootstrapping function (3,999 iterations) (Leifheit et al., 2014; Alvarez et al., 2017; Hu et al., 2023). Positive values indicated an increase in soil salinity after halophytes plantation and negative values indicated a decrease in salinity. If 95% CIs were separated from zero-line, significant desalinization effects were considered by halophytes, otherwise, there is no difference between the halophytes-planted groups and control.
LnRR++ was transformed to effect size (%) according to the following equation:
The Q test was performed to evaluate between-group heterogeneity and the subcategories. Categorical factors were considered statistically different if the significance level of Q statistics between groups was <0.05. Linear meta-regression was performed to examine the relationships between LnRR and continuous predictor variables using restricted maximum likelihood estimator (RMLE) method (Song et al., 2019; Sang et al., 2022). Publication bias was determined by using egger’s regression and fail-safe numbers (Supplementary Table S1) using “metafor” package in R software and MetaWin v. 2.1, respectively (Chen et al., 2013; Sang et al., 2022).
3 Results
3.1 Desalinization performance of halophytes on a global scale
Positive values indicated an increase in soil salinity after halophytes cultivation and negative values indicated a decrease in salinity. If 95% CIs were separated from zero-line, significant desalinization effects were considered by halophytes, otherwise, there is no difference between the halophytes-planted groups and control (Leifheit et al., 2014; Alvarez et al., 2017). Soil salinity was significantly decreased in halophytes field on average by 37.7% compared to control on a global scale (p < 0.05). Soluble ions (Na+, K+, Ca2+, Mg2+, Cl−, SO42-, HCO3−) and heavy metal concentrations (Cu, Zn, Cd, and Pb) significantly decreased as well (p < 0.05) Figure 1.
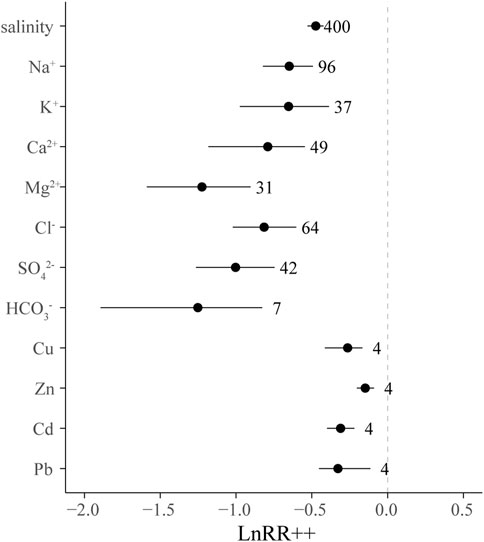
FIGURE 1. LnRR++ and bias-corrected 95% CIs of soil salinity, Na+, K+, Ca2+, Mg2+, Cl−, SO42-, HCO3−. LnRR++ was considered significant if the 95% CI did not overlap with 0. The number next to the 95% CI is the number of trials in the level.
3.2 The relationships between desalinization and environmental factors
Meta-regression takes the result of each independent experiment as an index of effect, and analyzes the correlations with environmental factors, which can reveal the desalination performance of halophytes in different environments (Beyene et al., 2022). A convex linear correlation was found between LnRR_salinity and MAT in the range of −1.3°C–29.1°C, and LnRR_salinity was relatively high at around 13 °C (R2 = 0.08, p < 0.01) (Figure 2). LnRR_salinity was negatively linear correlated with AI (R2 = 0.04, p < 0.01), accompanied by a positive linear correlation with MAP (R2 = 0.04, p < 0.01), but a negative linear correlation with PET (R2 = 0.03, p < 0.01).
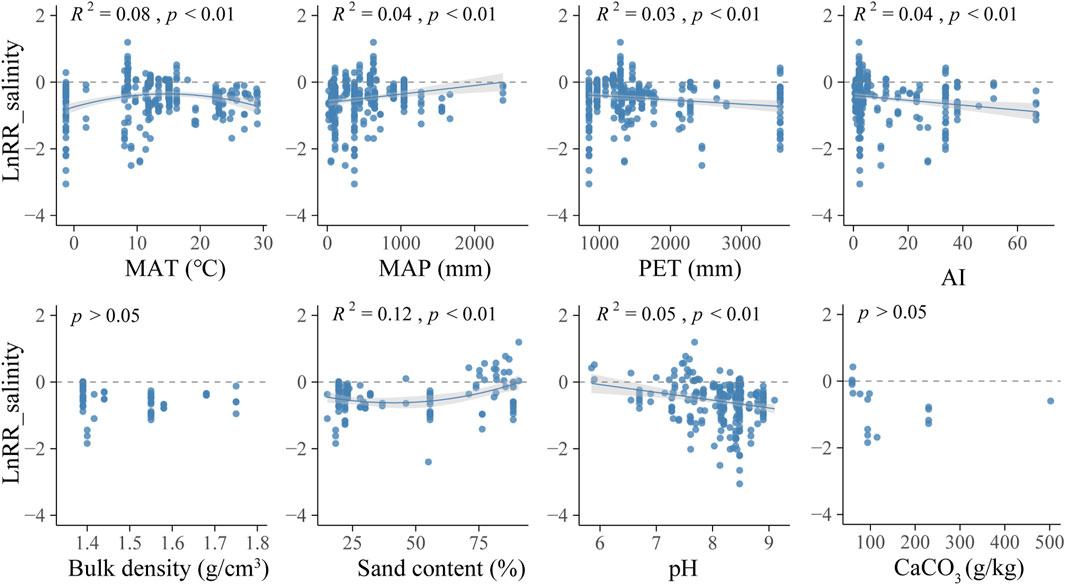
FIGURE 2. Relationships between LnRR_salinity and local climate conditions and soil properties. MAT, mean annual air temperature; AI, aridity index; MAP, mean annual precipitation; PET, potential evapotranspiration. Linear meta-regression is plotted with a solid line and the shaded area on either side represents the 95% CIs. Each point represents LnRR_salinity of each trial.
There is a concave linear relationship between sand content and LnRR, and LnRR_salinity increases significantly when it is greater than 75% (R2 = 0.12, p < 0.01) (Figure 2). LnRR_salinity showed a negative linear correlation with pH value (R2 = 0.05, p < 0 .01), but no relationships between LnRR_salinity and bulk density or CaCO3 content were found in the analysis (p > 0.05).
3.3 Cultivated practices regulating the desalinization performance
Experiment settings significantly influenced LnRR++_salinity, and LnRR++_salinity exhibited a significant difference between harvest practices in field experiments (p < 0.05). Effect sizes were −22.3% (p < 0.05) in field experiments under no-harvest conditions, −39.4% (p < 0.05) in field experiments under harvest conditions, −42.3% (p < 0.05) in pot experiments, and −43.3% (p < 0.05) in natural experiments (p < 0.05) (Figure 3).
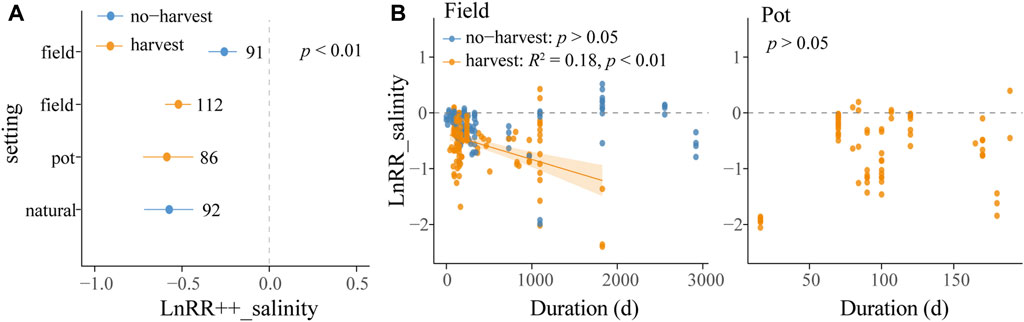
FIGURE 3. (A) LnRR++_salinity and bias-corrected 95% CIs for the levels of moderators “setting” and “harvest.” LnRR++_salinity was considered significant if the 95% CI did not overlap with 0. The number next to the 95% CI is the number of trials in the level. (B) Relationships between LnRR_salinity and planting duration under different “setting” and “harvest” conditions. Linear meta-regression is plotted with a solid line and the shaded area on either side represents the 95% CIs. Blue points represent LnRR_salinity of no-harvest groups and orange points represent LnRR_salinity of harvest groups.
In the range of 0 to about 3,000 days experiment duration, a negative linear correlation was found between LnRR_salinity and experiment duration in harvested field conditions (R2 = 0.18, p < 0.05) (Figure 3), and no correlation was detected in no-harvested conditions and pot experiments. No correlation was found between LnRR_salinity and experiment duration in the range of 0–180 days (p > 0.05).
Desalinization effects showed significant differences among different soil layers (p < 0.05) (Figure 4). Effect sizes of annual halophytes were −39.4% (p < 0.05), −19.9% (p < 0.05) and −2.66% (p > 0.05) in 0–30 cm, 30–60 cm, and >60 cm soil layers, respectively. Those of perennial halophytes were −39.1% (p < 0.05), −47.0% (p < 0.05) and −27.1% (p > 0.05) in 0–30 cm, 30–60 cm and >60 cm soil layers, respectively.
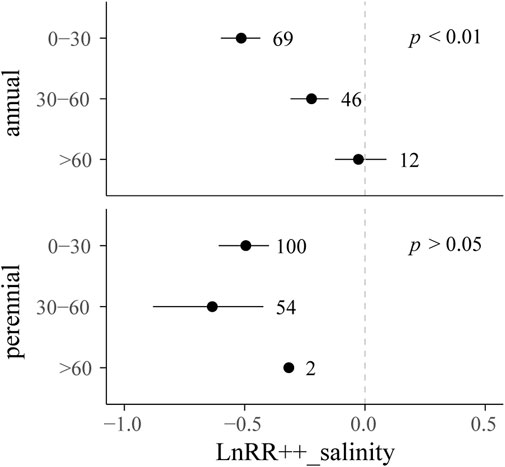
FIGURE 4. LnRR++_salinity and bias-corrected 95% CIs for the levels of moderators “lifeform” and “soil layer.” LnRR++_salinity was considered significant if the 95% CI did not overlap with 0. The number next to the 95% CI is the number of trials in the level.
3.4 Shoot salt accumulation in halophytes
LnRR_salinity was negatively correlated with shoot biomass (R2 = 0.08, p < 0.01) and Cl− removal by shoot biomass (R2 = 0.16, p = 0.03), and positively correlated with shoot Na+ content (R2 = 0.07, p = 0.04). In pot experiments, a negative linear correlation was found between LnRR_salinity and shoot biomass (R2 = 0.11, p < 0.01), shoot Cl− content (R2 = 0.42, p < 0.01) and Cl− removal by shoot biomass (R2 = 0.44, p = 0.03), and no relationship was found between LnRR_salinity and shoot Na+ content and Na+ removal by shoot (p > 0.05). In field experiments, no linear correlation was found between LnRR_salinity and shoot biomass, shoot Na+ content, shoot Cl− content, Na+ removal by shoot biomass or Cl− removal by shoot biomass (p > 0.05) (Figure 5).
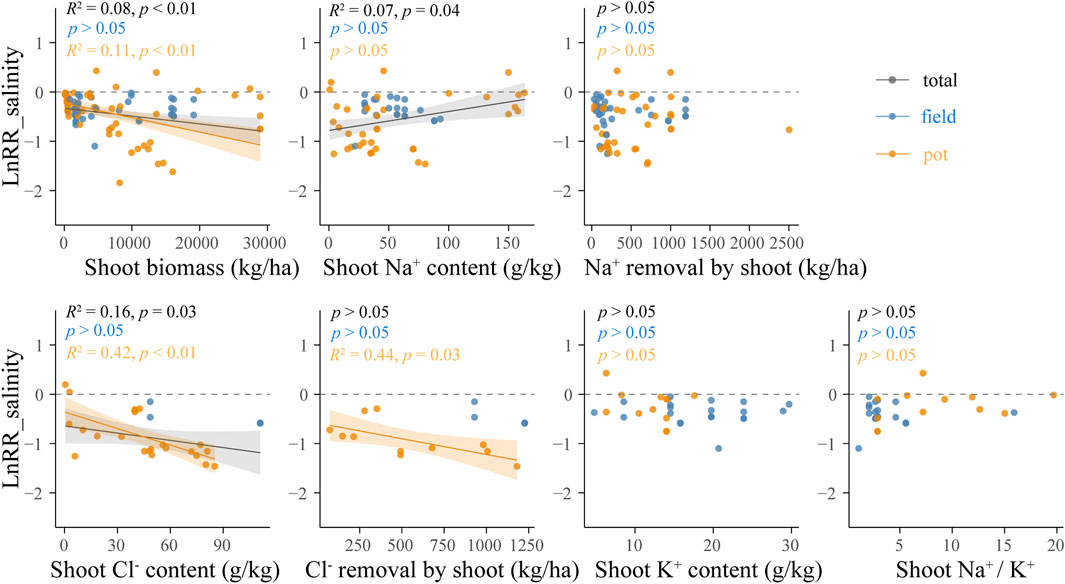
FIGURE 5. Relationships between LnRR_salinity and shoot biomass, shoot Na+, K+ and Cl− content, Na+ and Cl− removal by shoot, and shoot Na+/K+ under field and pot conditions. Linear meta-regression is plotted with a solid line and the shaded area on either side represents the 95% CIs. Black: all data; blue: field; orange: pot.
In both pot and field experiments, no relationship was found between LnRR_salinity and shoot K+ content or shoot Na+/K+ (p > 0.05).
3.5 The relationships between desalinization and response of soil properties
Correlations between desalinization performance and LnRR_salinity of soil properties can help to reveal the underground mechanisms during desalinization (Wang et al., 2021; Beyene et al., 2022). LnRR_salinity exhibited significantly positive linear correlated with LnRR_BD (R2 = 0.30, p < 0.01), LnRR_pH (R2 = 0.02, p = 0.04), and LnRR_AK (R2 = 0.19, p < 0.01), negative correlated with LnRR_SOC (R2 = 0.11, p = 0.04), LnRR_TN (R2 = 0.08, p = 0.01), and LnRR_MB (R2 = 0.82, p < 0.01), and not correlated with LnRR_AP (p > 0.05) (Figure 6).
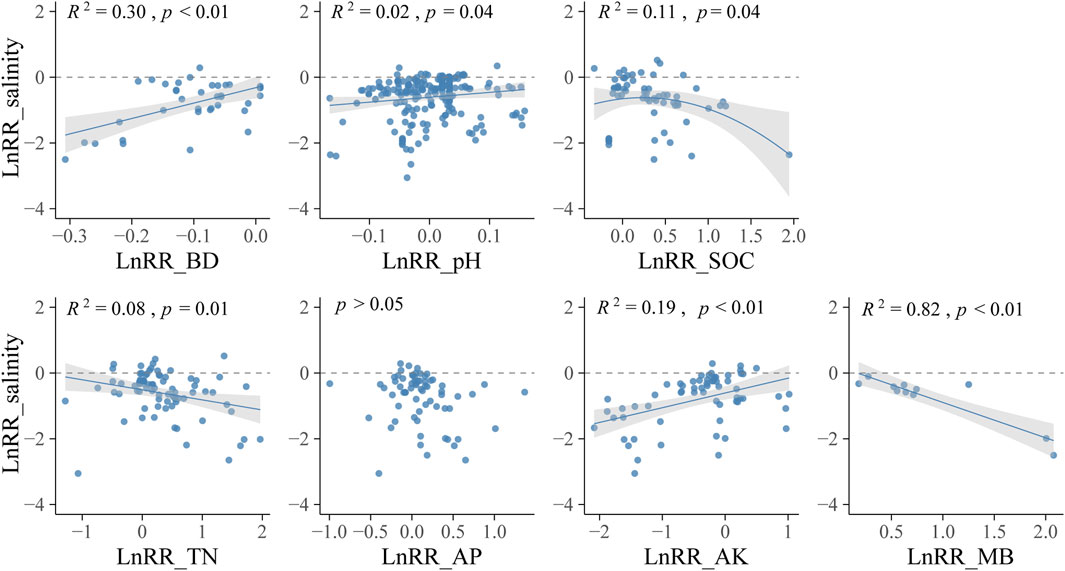
FIGURE 6. Relationships between LnRR_salinity and LnRR_salinity of soil properties. BD, bulk density; SOC, soil organic carbon; TN, total nitrogen; AP, available phosphorus; AK, available potassium; MB, microbial biomass. Linear meta-regression is plotted with a solid line and the shaded area on either side represents the 95% CIs. Each point represents LnRR_salinity of each trial.
4 Discussion
4.1 Climate and soil properties affecting desalinization by halophytes
It has been studied that MAT is one of the most important drivers affecting the salinization process, and plants could mitigate the sensitivity of soil surface temperature change with air temperature (Han et al., 2010; Ma et al., 2018; Eswar et al., 2021). The convex linear regression between LnRR_salinity and MAT in the range of −1.3°C–30 °C showed larger effects of halophytes desalinization in cold and hot regions. This was consistent with the results of previous studies indicating that in hot areas, soil desalinization by halophytes could be attributed to canopy shading resulting in the alleviation of the high-temperature driving salinization process, such as soil evaporation (Panta et al., 2014; Jian et al., 2015; Nouri et al., 2017; Eswar et al., 2021). The amplification of soil desalinization effects in cold areas might be related to the influence on soil freeze-thaw process. Salt in the soil profile would accumulate at the surface layer in the freezing period and initial evaporation period due to large evaporation, which are also the main causes of soil salinization in cold and arid areas (Han et al., 2010; Zong et al., 2022). The increase of vegetation or stubble or soil moisture may migrate the temperature change and reduce the evaporation, which might influence the accumulation of salt on the soil surface (Flerchinger et al., 2003; Wu et al., 2019; Qin et al., 2021).
There was a negative correlation between LnRR_salinity and AI, indicating that in arid and semiarid areas, most of which secondary salinized, soil desalinization by halophytes could be better than in wet areas with high rainfall and low evaporation. As Eswar et al. (2021) pointed out, the underlying mechanisms of salinization are different between arid areas and humid coastal areas. In arid areas, scarce precipitation and a large amount of evaporation occur, and vegetation could reduce soil evaporation by increasing coverage and improving soil texture (Puigdefábregas, 2005; Jian et al., 2015; Cao et al., 2020). While in coastal areas with high precipitation providing downward forces, salinization is often caused by seawater movements, i.e., intrusion and floods (Ketabchi et al., 2016; Khanom, 2016; Pauw et al., 2017). Therefore, halophytes applied in such areas should be better considered to gradually improve soil properties in the meantime provide economic benefits. Relationships between desalinization and environmental conditions indirectly corroborated the importance of land cover by halophytes in inhibiting salinity by reducing surface temperature and evaporation (Panta et al., 2014; Nouri et al., 2017).
Desalinization performance was better under low sand content conditions than that under high sand content conditions where salinity even increased. This was consistent with the results of previous studies in which soil with a high content of clay and silt particles has a strong ability to hold water and salt, and the reduction of soil salt content by halophytes in such conditions might be partially due to the penetration of soil by roots to increase soil pores and thus increase water infiltration and salt leaching (Jesus et al., 2015; Nunes et al., 2015). However, soil with high sand content has poor water and salt holding capacity and weakened upward-driven force, which might not only lead to relatively lower soil salt content before plantation but also increase soil water and salt holding capacity by the input of organic matter and their positive effects on soil aggregations (Zhang et al., 2016a; Cao et al., 2020; Li et al., 2020). This indicated that soil desalinization by halophytes may be more beneficial to reduce salt surface accumulation and increase salt leaching and infiltration in the areas with heavy topsoil and loose deep soil.
During the alkalization process, Na+ in the soil solution enters the soil colloid, so that the soil colloid contains more replaceable sodium (Litalien and Zeeb, 2020). When +1 charged cations dominate soils, clay particles are especially dispersed, resulting in a poor soil structure with few macropores and thus increasing water evaporation and reducing water infiltration (Litalien and Zeeb, 2020). In this study, it was found that desalinization was greater in the area of high pH, which may be owing to the efficient rhizosphere processes in such area. Soil pH value could be reduced by rhizosphere processes, such as root respiration or root exudates which further improve soil structure (Qadir et al., 1996; Qadir et al., 2005; Akhter et al., 2003).
4.2 Cultivated practices affecting desalinization by halophytes
The effect of experiment duration on LnRR_salinity was correlated only with field experiments under harvest conditions, showing that continuous planting of multiple growing seasons would decrease salinity to a greater degree. This was consistent with the results of previous studies indicating under non-harvest conditions, salt taken up by halophytes could be returned to the soil by leaf shedding on the surface, and this might even include salt taken up from groundwater (Feng et al., 2018; Cao et al., 2020). Whether in pot or field experiments planting for one growing season, no increase in desalinization was found with the extension of planting duration, indicating seasonal variations acting on phytodesalination capacity, because of different water movements and root respiration within seasons (Qadir et al., 2007; Rabhi et al., 2010b; Feng et al., 2018; Liang and Shi, 2021). The study of seasonal variations of halophytes desalinization could be helpful in the application of soil improvement performance and to arrange the later crops (Rabhi et al., 2010a; Rabhi et al., 2010b).
The desalinization of halophytes performed mainly within 0–60 cm soil layers, and the soil layer deeper than 60 cm was not the case. This was consistent with the results of previous reviews in which the desalinization effect was mainly limited to the rooting zone (Qadir et al., 2000). The roots of annual plants are mainly distributed in the range of 0–30 cm layer, while perennials have deeper root distribution and root biomass reserves underground (Barrett-Lennard, 2002; Monti and Zatta, 2009). Due to the differences in root growth between annual plants and perennial plants, the soil desalinization capacity of annual plants is greater in 0–30 cm layer than that of 30–60 cm layer, and perennial plants performed similarly in both layers. In addition, annual plants are fast-growing, and have a large amount of seed, while perennials are slow to establish but can be harvested continually after successful establishment. The species of different lifeforms can be selected according to different purposes (Nikalje et al., 2018).
4.3 Aboveground accumulation and root-soil interaction of desalinization by halophytes
Aboveground accumulation and root-soil interaction are two main parts of soil desalinization. Studies proved the potential of halophytes for salt uptake (Ravindran et al., 2007; Rabhi et al., 2009; Rabhi et al., 2010a), while Gharaibeh et al. (2011) suggested that salt uptake in aboveground biomass is a small percentage comparing with original salt content or salt input by irrigation in saline soils and, in consequence, root rhizosphere processes would be the main mechanism of desalinization. This analysis showed that harvest significantly increased desalinization, and desalinization was detected to be greater with larger shoot biomass, indicating the important contribution of aboveground salt accumulation to desalinization and that shoot biomass could be used as an indicator of the desalinization performance of halophytes. However, no correlation was detected between LnRR_salinity and Na+ or Cl− removal of shoot in field conditions, indicating root-soil interaction also played an important role in phytodesalination (Qadir et al., 1996; Qadir et al., 2005; Akhter et al., 2003; Liang and Shi, 2021). There have been some possible mechanisms of soil desalinization in plant root-microbe-soil processes (Jesus et al., 2015; Rabhi et al., 2015; Liang and Shi, 2021). Respiration of roots and microorganisms, root exudates, and decomposition of organic matter generating acid matter reduce the soil pH of alkaline soil and dissolve CaCO3, and then Ca2+ exchange Na+ from soil colloids (Qadir et al., 1996; Qadir et al., 2005); roots exudates and turnover and the propagation of microorganisms increase soil organic matter; roots gather and penetrate the soil to improve soil pore size and increase precipitation interception and runoff infiltration (Li et al., 2020; Rathore et al., 2022). In this study, desalinization performance is accompanied by the decrease of BD and pH and the increase of SOC, TN, and MB, which further corroborates the important roles of root-soil interactions and the improvement of soil structure and soil fertility (Figure 6). However, no relationship between LnRR_salinity of soil salinity and CaCO3 content. It should be noted that the precipitation and dissolution of CaCO3 co-exist in soil, affected by climatic conditions, land use types, and soil properties. CaCO3 content may show different trends during vegetation restoration (Liu et al., 2017; Gao et al., 2018; Xu et al., 2021). The change of CaCO3 content and the contribution of CaCO3 dissolution to phytodesalination needs further clarification.
Plant inputs stimulate the activity and size of the soil microbial community, which could enhance the nutrient cycle and reduce soil pH of saline-alkali soil (Li et al., 2022; Liu et al., 2022; Rathore et al., 2022). Microbial biomass and the resulting microbial necromass, part of which can be relatively recalcitrant, provides binders for aggregates that physically protect soil organic matters and improve soil structure (Bronick and Lal, 2005; Zhu et al., 2018; Zhu et al., 2020; Shao et al., 2022). In this study, MB increased in halophytes field, and the response showed a strong correlation with desalinization effect, which highlights the importance of microbes in the process of soil improvement during desalinization (Figure 6). In addition, microbes play a critical role in developing plant salt tolerance strategies by regulatory mechanisms including ions transportation and homeostasis, osmotic regulation, hormone balance, antioxidant mechanisms, and other stress signaling (Acuña et al., 2019; Salwan et al., 2019; Diao et al., 2022; Wang et al., 2022). The interaction between plants and microbes suggests that halophytes promote microbial colonization compared with bare land, and microbes could directly or indirectly affect soil desalination by improving soil properties and promoting plant growth (Figures 5, 6). However, many pieces of research focus on halophytes root-associated microbes improving salt tolerance of crops (Acuña et al., 2019; Salwan et al., 2019; Wang et al., 2022), and halophytes effects on soil pH, nutrients, structures, and microbes (Li et al., 2022; Liu et al., 2022; Rathore et al., 2022). There is a clear need for more studies on halophyte-microbe-soil interactions, especially the multiple roles of microbes in halophytes desalinization.
In some areas, high soil salinity is accompanied with high concentrations of bioavailable heavy metals. This particularly occurs in coastal areas where received industrial and urban wastes, and in arid and semi-arid regions where mining and agricultural practices contribute to the increase of both heavy metals and salinity (Duarte et al., 2010; Wang et al., 2014; Lutts and Lefèvre, 2015; Guan et al., 2018). In the face of the combination of salt and heavy metals pollution, it is impossible for glycophytes accumulators to remediate these soils, because glycophytes cannot survive in saline soils, contrary to halophytes. Halophytes are now receiving increasing attention as potential candidates for remediation of these heavy metal-polluted saline soils (Manousaki and Kalogerakis, 2011; Wang et al., 2014; Lutts and Lefèvre, 2015). Studies about phytoremediation by halophytes mainly focused on the effect of salinity on heavy metals accumulation and distribution characteristics (Manousaki and Kalogerakis, 2009; Zhang et al., 2016b; Kahli et al., 2021), and the mechanisms of salinity alleviating heavy metals toxicity and promoting growth (Ghnaya et al., 2007; Bankaji et al., 2016; Zhou et al., 2018; Patel and Parida, 2021), indicating the importance of salinity on heavy metals phytoremediation. These results could also suggest that the interactions of salt and heavy metals have effects on plant growth and, in turn, on desalinization (Bankaji et al., 2016; Zhang et al., 2016b; Zhang et al., 2020). However, few studies investigated the salinity and heavy metals interaction effects on soil desalinization. As the salinization process intensifies, soil desalinization could be taken into consideration in the assessment of halophytes for the phytoremediation of heavy metals contaminated saline soils.
Na+ and K+ compete for binding sites of roots in saline soil due to the similarity of physical and chemical properties. Jesus et al. (2015) implied that plants that absorb a lot of potassium might increase the potential requirements for nutrients or indicate that the plants are highly selective to K+ over Na+. As a consequence, sodium uptake would be less in this kind of plants. However, K+ plays a vital role in plants, and halophytes need to maintain a certain ratio of sodium to potassium (Matinzadeh et al., 2013; Percey et al., 2016). This analysis showed that desalinization capacity did not correlate with shoot K+ content, indicating that halophytes with high selectivity for K+ in their roots may not reduce their desalinization capacity, which further suggests that appropriate K application could be permitted during phytodesalination.
In this study, desalinization by halophytes was similar in the pot experiments and field experiments (Figure 3), and shoot Cl− accumulation contributed more to desalinization in pot experiments than in field experiments (Figure 5; Supplementary Figure S2). Pot experiments could control leaching (Qadir et al., 1996; Qadir et al., 2005; Rabhi et al., 2009; Gharaibeh et al., 2011), thereby soluble ions that should have been leached out of the rhizosphere would be absorbed by the plants. So, it should be noted that pot experiments would magnify the contribution of aboveground salt accumulation to desalinization.
5 Conclusion and further perspectives
Our results provide insights into how the desalinization performance depends on abiotic and biotic experimental conditions and its desalinized mechanisms. Results showed that desalinization performance was better in cold and hot regions than in temperate regions, in dry regions than in wet regions, in alkaline saline soils than in neutral saline soils, and in conditions with low sand content than high sand content. Under aboveground harvest treatment, desalinization increased with the years of cultivation, while no trends were detected under no harvest treatment, indicating the importance of aboveground accumulation. Desalinization was not related to soil CaCO3 content but was accompanied by soil properties improvement, implying other root-soil interactions rather than CaCO3 dissolution play important roles. Shoot biomass could be used as an indicator of the desalinization performance, and the performance would not be decreased due to the high uptake selectivity for K+ over Na+. Desalinization of annuals mainly happened in 0–30 cm soil layers, and that of perennials happened in 0–60 cm layers. Desalinization was similar in the pot experiments and field experiments, but it should be noted that pot experiments would magnify the contribution of aboveground salt accumulation to desalinization.
Our results corroborate some mechanisms of phytodesalination, including the decrease of evaporation and soil surface temperature, salt accumulation in shoot biomass, and the improvement of soil properties. Meanwhile, we found some mechanisms that need to be verified and explored (Figure 7). Future research should be focused on: to verify the change of soil CaCO3, the contribution of CaCO3 dissolution to desalinization, and its required environmental conditions; to uncover halophyte-microbe-soil interactions affecting desalinization, especially the multiple roles of microbes in halophytes desalinization; to explore how halophytes influence the process of salt accumulation caused by freeze-thaw cycles. Furthermore, the exploitation of the economic value of halophytes is still critical to popularize the technology of phytodesalination.
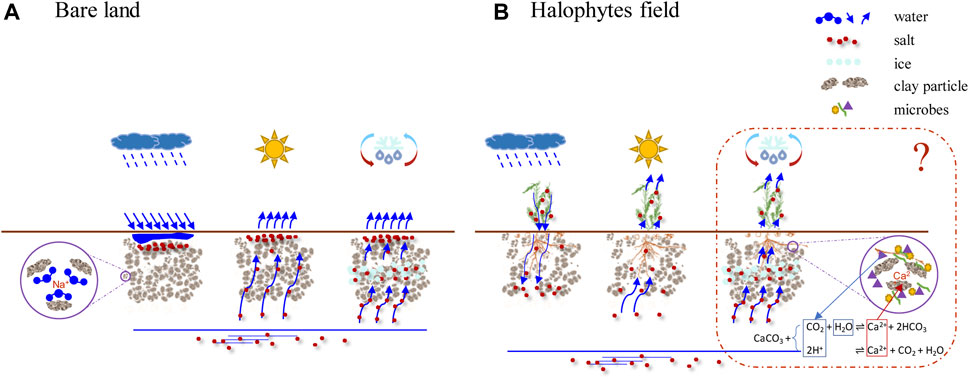
FIGURE 7. Role of halophytes in soil desalinization. (A) Bare land, (B) Halophytes field. Basically, we proposed that halophytes cultivation would decrease soil salt content, improve soil structure, and increase soil organic carbon, nutrients, and microbial biomass in the rooting zone. The relevant mechanisms were compiled from the results of previous publications (Panta et al., 2014; Nouri et al., 2017) and this study, including aboveground salt accumulation, an increase of salt leaching, land cover decreasing evaporation, and water table reduction. The red dotted line box shows the differences with previous studies and possible mechanisms that need further clarification, including the contribution of CaCO3 dissolution to desalination and the required environmental conditions, the multiple roles of microbes in halophytes desalinization, and the possible influence of salt accumulation caused by freeze-thaw cycles.
Data availability statement
The raw data supporting the conclusion of this article will be made available from the corresponding authors upon reasonable request, without undue reservation.
Author contributions
SW and JL: conceptualization, writing and analysis. YL: analysis and methodology. YW: visualization. CT: supervision and funding acquisition. All authors contributed to the article and approved the submitted version.
Funding
This work was supported by the Natural Science Foundation of Xinjiang Uygur Autonomous Region (2022D01D084), National Key Research and Development Program of China (2021YFD1900801-02), and Major Project of Chinese Agriculture Ministry (NK2022180402).
Conflict of interest
The authors declare that the research was conducted in the absence of any commercial or financial relationships that could be construed as a potential conflict of interest.
Publisher’s note
All claims expressed in this article are solely those of the authors and do not necessarily represent those of their affiliated organizations, or those of the publisher, the editors and the reviewers. Any product that may be evaluated in this article, or claim that may be made by its manufacturer, is not guaranteed or endorsed by the publisher.
Supplementary material
The Supplementary Material for this article can be found online at: https://www.frontiersin.org/articles/10.3389/fenvs.2023.1198540/full#supplementary-material
Abbreviations
MAT, mean annual air temperature; MAP, mean annual precipitation; PET, potential evapotranspiration; AI, aridity index; BD, bulk density; SOC, soil organic carbon; TN, total nitrogen; AP, available phosphorus; AK, available potassium; MB, microbial biomass; LnRR, natural logarithm response ratio; LnRR++, weighted natural logarithm response ratio.
References
Acuña, J. J., Campos, M., Mora, M. D., Jaisi, D. P., and Jorquera, M. A. (2019). ACCD-producing rhizobacteria from an Andean Altiplano native plant (Parastrephia quadrangularis) and their potential to alleviate salt stress in wheat seedlings. Appl. Soil Ecol. 136, 184–190. doi:10.1016/j.apsoil.2019.01.005
Akhter, J., Mahmood, K., Malik, K. A., Ahmed, S., and Murray, R. (2003). Amelioration of a saline sodic soil through cultivation of a salt-tolerant grass Leptochloa fusca. Environ. Conserv. 30, 168–174. doi:10.1017/S0376892903000158
Alvarez, R., Steinbach, H. S., and De Paepe, J. L. (2017). Cover crop effects on soils and subsequent crops in the pampas: a meta-analysis. Soil Tillage Res. 170, 53–65. doi:10.1016/j.still.2017.03.005
Anjum, N. A., Ahmad, I., Válega, M., Mohmood, I., Gill, S. S., Tuteja, N., et al. (2014). Salt marsh halophyte services to metal-metalloid remediation: assessment of the processes and underlying mechanisms. Crit. Rev. Environ. Sci. Technol. 44, 2038–2106. doi:10.1080/10643389.2013.828271
Bankaji, I., Caçador, I., and Sleimi, N. (2016). Assessing of tolerance to metallic and saline stresses in the halophyte Suaeda fruticosa: the indicator role of antioxidative enzyme. Ecol. Indic. 64, 297–308. doi:10.1016/j.ecolind.2016.01.020
Barcia-Piedras, J. M., Pérez-Romero, J. A., Mateos-Naranjo, E., Camacho, M., and Redondo-Gómez, S. (2019). Effect of prior salt experience on desalination capacity of the halophyte Arthrocnemum macrostachyum. Desalination 463, 50–54. doi:10.1016/j.desal.2019.03.006
Barrett-Lennard, E. G. (2002). Restoration of saline land through revegetation. Agric. Water Manag. 53, 213–226. doi:10.1016/S0378-3774(01)00166-4
Beyene, B. B., Li, J., Yuan, J., Dong, Y., Liu, D., Chen, Z., et al. (2022). Non-native plant invasion can accelerate global climate change by increasing wetland methane and terrestrial nitrous oxide emissions. Glob. Chang. Biol. 28, 5453–5468. doi:10.1111/gcb.16290
Bronick, C. J., and Lal, R. (2005). Soil structure and management: a review. Geoderma 124, 3–22. doi:10.1016/j.geoderma.2004.03.005
Cao, Q., Yang, B., Li, J., Wang, R., Liu, T., and Xiao, H. (2020). Characteristics of soil water and salt associated with Tamarix ramosissima communities during normal and dry periods in a semi-arid saline environment. Catena 193, 104661. doi:10.1016/j.catena.2020.104661
Chen, H., Li, X., Hu, F., and Shi, W. (2013). Soil nitrous oxide emissions following crop residue addition: a meta-analysis. Glob. Chang. Biol. 19, 2956–2964. doi:10.1111/gcb.12274
Diao, F., Jia, B., Wang, X., Luo, J., Hou, Y., Li, F. Y., et al. (2022). Proteomic analysis revealed modulations of carbon and nitrogen by arbuscular mycorrhizal fungi associated with the halophyte Suaeda salsa in a moderately saline environment. Land Degrad. Dev. 33, 1933–1943. doi:10.1002/ldr.4274
Duarte, B., Caetano, M., Almeida, P. R., Vale, C., and Cacador, I. (2010). Accumulation and biological cycling of heavy metal in four salt marsh species, from Tagus estuary (Portugal). Environ. Pollut. 158, 1661–1668. doi:10.1016/j.envpol.2009.12.004
Eswar, D., Karuppusamy, R., and Chellamuthu, S. (2021). Drivers of soil salinity and their correlation with climate change. Curr. Opin. Env. Sust. 50, 310–318. doi:10.1016/j.cosust.2020.10.015
FAO (2021). The state of the world’s land and water resources for food and agriculture - systems at breaking point. Synthesis report 2021. Rome: FAO. doi:10.4060/cb7654en
Feng, J., He, K., Zhang, Q., Han, M., and Zhu, B. (2022). Changes in plant inputs alter soil carbon and microbial communities in forest ecosystems. Glob. Chang. Biol. 28, 3426–3440. doi:10.1111/gcb.16107
Feng, X., An, P., Li, X., Guo, K., Yang, C., and Liu, X. (2018). Spatiotemporal heterogeneity of soil water and salinity after establishment of dense-foliage Tamarix chinensis on coastal saline land. Ecol. Eng. 121, 104–113. doi:10.1016/j.ecoleng.2017.06.031
Flerchinger, G. N., Sauer, T. J., and Aiken, R. A. (2003). Effects of crop residue cover and architecture on heat and water transfer at the soil surface. Geoderma 116, 217–233. doi:10.1016/S0016-7061(03)00102-2
Gao, Y., Dang, P., Zhao, Q., Liu, J., and Liu, J. (2018). Effects of vegetation rehabilitation on soil organic and inorganic carbon stocks in the Mu Us Desert, northwest China. Land Degrad. Dev. 29, 1031–1040. doi:10.1002/ldr.2832
Gharaibeh, M. A., Eltaif, N. I., and Albalasmeh, A. A. (2011). Reclamation of highly calcareous saline sodic soil using Atriplex halimus and by-product gypsum. Int. J. Phytoremediat. 13, 873–883. doi:10.1080/15226514.2011.573821
Ghnaya, T., Slama, I., Messedi, D., Grignon, C., Ghorbel, M. H., and Abdelly, C. (2007). Cd-induced growth reduction in the halophyte Sesuvium portulacastrum is significantly improved by NaCl. J. Plant Res. 120, 309–316. doi:10.1007/s10265-006-0042-3
Guan, Q., Song, N., Wang, F., Yang, L., and Liu, Z. (2018). Contamination levels and health risk assessments of heavy metals in an oasis-desert zone: a case study in northwest China. Environ. Sci. Pollut. Res. 25, 22606–22618. doi:10.1007/s11356-018-2015-1
Gurevitch, J., and Hedges, L. V. (1999). Statistical issues in ecological meta-analysis. Ecology 80, 1142–1149. doi:10.1890/0012-9658(1999)080[1142:SIIEMA]2.0.CO;2
Han, L., Tsunekawa, A., and Tsubo, M. (2010). Monitoring near-surface soil freeze-thaw cycles in northern China and Mongolia from 1998 to 2007. Int. J. Appl. Earth Obs. Geoinf. 12, 375–384. doi:10.1016/j.jag.2010.04.009
Hedges, L. V., Gurevitch, J., and Curtis, P. S. (1999). The meta-analysis of response ratios in experimental ecology. Ecology 80, 1150–1156. doi:10.1890/0012-9658(1999)080[1150:TMAORR]2.0.CO;2
Hopmans, J. W., Qureshi, A. S., Kisekka, I., Munns, R., Grattan, S. R., Rengasamy, P., et al. (2021). Critical knowledge gaps and research priorities in global soil salinity. Adv. Agron. 169, 1–191. doi:10.1016/bs.agron.2021.03.001
Hu, Q., Thomas, B. W., Powlson, D., Hu, Y., Zhang, Y., Jun, X., et al. (2023). Soil organic carbon fractions in response to soil, environmental and agronomic factors under cover cropping systems: a global meta-analysis. Agric. Ecosyst. Environ. 355, 108591. doi:10.1016/j.agee.2023.108591
Ivushkin, K., Bartholomeus, H., Bregt, A. K., Pulatov, A., Kempen, B., and de Sousa, L. (2019). Global mapping of soil salinity change. Remote Sens. Environ. 231, 111260. doi:10.1016/j.rse.2019.111260
Jesus, J. M., Danko, A. S., Fiúza, A., and Borges, M. T. (2015). Phytoremediation of salt-affected soils: a review of processes, applicability, and the impact of climate change. Environ. Sci. Pollut. Res. 22, 6511–6525. doi:10.1007/s11356-015-4205-4
Jesus, J. M., Danko, A. S., Fiúza, A., and Borges, M. T. (2018). Comparison of vegetative bioremediation and chemical amendments for non-calcareous highly saline-sodic soil remediation. Water Air Soil Pollut. 229, 274. doi:10.1007/s11270-018-3928-5
Jian, S., Zhao, C., Fang, S., and Yu, K. (2015). Effects of different vegetation restoration on soil water storage and water balance in the Chinese Loess Plateau. Agric. For. Meteorol. 206, 85–96. doi:10.1016/j.agrformet.2015.03.009
Kahli, H., Sbartai, H., Cohen-Bouhacina, T., and Bourguignon, J. (2021). Characterization of cadmium accumulation and phytoextraction in three species of the genus Atriplex (canescens, halimus and nummularia) in the presence or absence of salt. Plant Physiol. biochem. 166, 902–911. doi:10.1016/j.plaphy.2021.06.027
Ketabchi, H., Mahmoodzadeh, D., Ataie-Ashtiani, B., and Simmons, C. T. (2016). Sea-level rise impacts on seawater intrusion in coastal aquifers: review and integration. J. Hydrol. 535, 235–255. doi:10.1016/j.jhydrol.2016.01.083
Khanom, T. (2016). Effect of salinity on food security in the context of interior coast of Bangladesh. Ocean. Coast. Manag. 130, 205–212. doi:10.1016/j.ocecoaman.2016.06.013
Kronzucker, H. J., and Britto, D. T. (2011). Sodium transport in plants: a critical review. New Phytol. 189, 54–81. doi:10.1111/j.1469-8137.2010.03540.x
Lajeunesse, M. J. (2011). On the meta-analysis of response ratios for studies with correlated and multi-group designs. Ecology 92, 2049–2055. doi:10.1890/11-0423.1
Leifheit, E. F., Veresoglou, S. D., Lehmann, A., Morris, E. K., and Rillig, M. C. (2014). Multiple factors influence the role of arbuscular mycorrhizal fungi in soil aggregation - a meta-analysis. Plant Soil 374, 523–537. doi:10.1007/s11104-013-1899-2
Li, J., Cui, L., Delgado-Baquerizo, M., Wang, J., Zhu, Y., Wang, R., et al. (2022). Fungi drive soil multifunctionality in the coastal salt marsh ecosystem. Sci. Total Environ. 818, 151673. doi:10.1016/j.scitotenv.2021.151673
Li, J., Yuan, X., Ge, L., Li, Q., Li, Z., Wang, L., et al. (2020). Rhizosphere effects promote soil aggregate stability and associated organic carbon sequestration in rocky areas of desertification. Agric. Ecosyst. Environ. 304, 107126. doi:10.1016/j.agee.2020.107126
Liang, J., and Shi, W. (2021). Cotton/halophytes intercropping decreases salt accumulation and improves soil physicochemical properties and crop productivity in saline-alkali soils under mulched drip irrigation: a three-year field experiment. Field Crops Res. 262, 108027. doi:10.1016/j.fcr.2020.108027
Liao, C., Luo, Y., Fang, C., Chen, J., and Li, B. (2012). The effects of plantation practice on soil properties based on the comparison between natural and planted forests: a meta-analysis. Glob. Ecol. Biogeogr. 21, 318–327. doi:10.1111/j.1466-8238.2011.00690.x
Litalien, A., and Zeeb, B. (2020). Curing the earth: a review of anthropogenic soil salinization and plant-based strategies for sustainable mitigation. Sci. Total Environ. 698, 134235. doi:10.1016/j.scitotenv.2019.134235
Liu, X., Lu, X., Zhao, W., Yang, S., Wang, J., Xia, H., et al. (2022). The rhizosphere effect of native legume Albizzia julibrissin on coastal saline soil nutrient availability, microbial modulation, and aggregate formation. Sci. Total Environ. 806, 150705. doi:10.1016/j.scitotenv.2021.150705
Liu, Y., Dang, Z. Q., Tian, F. P., Wang, D., and Wu, G. L. (2017). Soil organic carbon and inorganic carbon accumulation along a 30-year grassland restoration chronosequence in semi-arid regions (China). Land Degrad. Dev. 28, 189–198. doi:10.1002/ldr.2632
Lutts, S., and Lefèvre, I. (2015). How can we take advantage of halophyte properties to cope with heavy metal toxicity in salt-affected areas? Ann. Bot. 115, 509–528. doi:10.1093/aob/mcu264
Ma, L., Yang, S., Simayi, Z., Gu, Q., Li, J., Yang, X., et al. (2018). Modeling variations in soil salinity in the oasis of Junggar Basin, China. Land Degrad. Dev. 29, 551–562. doi:10.1002/ldr.2890
Mahajan, S., and Tuteja, N. (2005). Cold, salinity and drought stresses: an overview. Arch. Biochem. Biophys. 444, 139–158. doi:10.1016/j.abb.2005.10.018
Manousaki, E., and Kalogerakis, N. (2009). Phytoextraction of Pb and Cd by the Mediterranean saltbush (Atriplex halimus L.): metal uptake in relation to salinity. Environ. Sci. Pollut. Res. 16, 844–854. doi:10.1007/s11356-009-0224-3
Manousaki, E., and Kalogerakis, N. (2011). Halophytes present new opportunities in phytoremediation of heavy metals and saline soils. Ind. Eng. Chem. Res. 50, 656–660. doi:10.1021/ie100270x
Matinzadeh, Z., Breckle, S. W., Mirmassoumi, M., and Akhani, H. (2013). Ionic relationships in some halophytic Iranian Chenopodiaceae and their rhizospheres. Plant Soil 372, 523–539. doi:10.1007/s11104-013-1744-7
Monti, A., and Zatta, A. (2009). Root distribution and soil moisture retrieval in perennial and annual energy crops in Northern Italy. Agric. Ecosyst. Environ. 132, 252–259. doi:10.1016/j.agee.2009.04.007
Nikalje, G. C., Srivastava, A. K., Pandey, G. K., and Suprasanna, P. (2018). Halophytes in biosaline agriculture: mechanism, utilization, and value addition. Land Degrad. Dev. 29, 1081–1095. doi:10.1002/ldr.2819
Nouri, H., Borujeni, S. C., Nirola, R., Hassanli, A., Beecham, S., Alaghmand, S., et al. (2017). Application of green remediation on soil salinity treatment: a review on halophytoremediation. Process Saf. Environ. Prot. 107, 94–107. doi:10.1016/j.psep.2017.01.021
Nunes, M. R., Denardin, J. E., Pauletto, E. A., Faganello, A., and Pinto, L. F. S. (2015). Effect of soil chiseling on soil structure and root growth for a clayey soil under no-tillage. Geoderma 259-260, 149–155. doi:10.1016/j.geoderma.2015.06.003
Panta, S., Flowers, T., Lane, P., Doyle, R., Haros, G., and Shabala, S. (2014). Halophyte agriculture: success stories. Environ. Exp. Bot. 107, 71–83. doi:10.1016/j.envexpbot.2014.05.006
Patel, M., and Parida, A. K. (2021). Salinity alleviates the arsenic toxicity in the facultative halophyte Salvadora persica L. by the modulations of physiological, biochemical, and ROS scavenging attributes. J. Hazard. Mater. 401, 123368. doi:10.1016/j.jhazmat.2020.123368
Pauw, P. S., Groen, J., Groen, M. M. A., van der Made, K. J., Stuyfzand, P. J., and Post, V. E. A. (2017). Groundwater salinity patterns along the coast of the Western Netherlands and the application of cone penetration tests. J. Hydrol. 551, 756–767. doi:10.1016/j.jhydrol.2017.04.021
Percey, W. J., Shabala, L., Wu, Q., Su, N., Breadmore, M. C., Guijt, R. M., et al. (2016). Potassium retention in leaf mesophyll as an element of salinity tissue tolerance in halophytes. Plant Physiol. Biochem. 109, 346–354. doi:10.1016/j.plaphy.2016.10.011
Puigdefábregas, J. (2005). The role of vegetation patterns in structuring runoff and sediment fluxes in drylands. Earth Surf. Process. Landforms 30, 133–147. doi:10.1002/esp.1181
Qadir, M., Ghafoor, A., and Murtaza, G. (2000). Amelioration strategies for saline soils: a review. Land Degrad. Dev. 11, 501–521. doi:10.1002/1099-145X(200011/12)11:6<501::AID-LDR405>3.0.CO;2-S
Qadir, M., Noble, A. D., Oster, J. D., Schubert, S., and Ghafoor, A. (2005). Driving forces for sodium removal during phytoremediation of calcareous sodic and saline-sodic soils: a review. Soil Use Manage 21, 173–180. doi:10.1079/SUM2005312
Qadir, M., Oster, J. D., Schubert, S., Nobel, A. D., and Sahrawat, K. L. (2007). Phytoremediation of sodic and saline-sodic soils. Adv. Agron. 96, 197–247. doi:10.1016/S0065-2113(07)96006-X
Qadir, M., Quillérou, E., Nangia, V., Murtaza, G., Singh, M., Thomas, R. J., et al. (2014). Economics of salt-induced land degradation and restoration. Nat. Resour. Forum 38, 282–295. doi:10.1111/1477-8947.12054
Qadir, M., Qureshi, R. H., and Ahmad, N. (1996). Reclamation of a saline-sodic soil by gypsum and Leptochloa fusca. Geoderma 74, 207–217. doi:10.1016/S0016-7061(96)00061-4
Qin, Y., Bai, Y., Chen, G., Liang, Y., Li, X., Wen, B., et al. (2021). The effects of soil freeze-thaw processes on water and salt migrations in the western Songnen Plain, China. Sci. Rep. 11, 3888. doi:10.1038/s41598-021-83294-x
Rabhi, M., Atia, A., Abdelly, C., and Smaoui, A. (2015). New parameters for a better evaluation of vegetative bioremediation, leaching, and phytodesalination. J. Theor. Biol. 383, 7–11. doi:10.1016/j.jtbi.2015.07.027
Rabhi, M., Ferchichi, S., Jouini, J., Hamrouni, M. H., Koyro, H. W., Ranieri, A., et al. (2010a). Phytodesalination of a salt-affected soil with the halophyte Sesuvium portulacastrum L. to arrange in advance the requirements for the successful growth of a glycophytic crop. Bioresour. Technol. 101, 6822–6828. doi:10.1016/j.biortech.2010.03.097
Rabhi, M., Hafsi, C., Lakhdar, A., Hajji, S., Barhoumi, Z., Hamrouni, M. H., et al. (2009). Evaluation of the capacity of three halophytes to desalinize their rhizosphere as grown on saline soils under nonleaching conditions. Afr. J. Ecol. 47, 463–468. doi:10.1111/j.1365-2028.2008.00989.x
Rabhi, M., Karray-Bouraoui, N., Medini, R., Attia, H., Athar, H. U. R., Abdelly, C., et al. (2010b). Seasonal variations in phytodesalination capacity of two perennial halophytes in their natural biotope. J. Biol. Res. (Thessalon.) 14, 181–189. Available at: http://www.jbr.gr/papers20102/05-Rabhi.pdf.
Rajkumar, M., Prasad, M. N. V., Swaminathan, S., and Freitas, H. (2013). Climate change driven plant-metal-microbe interactions. Environ. Int. 53, 74–86. doi:10.1016/j.envint.2012.12.009
Rasouli, F., Pouya, A. K., and Karimian, N. (2013). Wheat yield and physico-chemical properties of a sodic soil from semi-arid area of Iran as affected by applied gypsum. Geoderma 193, 246–255. doi:10.1016/j.geoderma.2012.10.001
Rathore, A. P., Chaudhary, D. R., and Jha, B. (2022). Assessing the effects of Salicornia brachiata Roxb. growth on coastal saline soil quality over temporal and spatial scales. Appl. Soil Ecol. 169, 104196. doi:10.1016/j.apsoil.2021.104196
Ravindran, K. C., Venkatesan, K., Balakrishnan, V., Chellappan, K. P., and Balasubramanian, T. (2007). Restoration of saline land by halophytes for Indian soils. Soil Biol. Biochem. 39, 2661–2664. doi:10.1016/j.soilbio.2007.02.005
Rozema, J., and Flowers, T. (2008). Crops for a salinized world. Science 322, 1478–1480. doi:10.1126/science.1168572
Rozema, J., and Schat, H. (2013). Salt tolerance of halophytes, research questions reviewed in the perspective of saline agriculture. Environ. Exp. Bot. 92, 83–95. doi:10.1016/j.envexpbot.2012.08.004
Salwan, R., Sharma, A., and Sharma, V. (2019). Microbes mediated plant stress tolerance in saline agricultural ecosystem. Plant Soil 442, 1–22. doi:10.1007/s11104-019-04202-x
Sang, J., Lakshani, M. M. T., Deepagoda, T. K. K. C., Shen, Y., and Li, Y. (2022). Drying and rewetting cycles increased soil carbon dioxide rather than nitrous oxide emissions: a meta-analysis. J. Environ. Manage. 324, 116391. doi:10.1016/j.jenvman.2022.116391
Shabala, S. (2013). Learning from halophytes: physiological basis and strategies to improve abiotic stress tolerance in crops. Ann. Bot. 112, 1209–1221. doi:10.1093/aob/mct205
Shao, P. S., Li, T., Dong, K. K., Yang, H. J., and Sun, J. K. (2022). Microbial residues as the nexus transforming inorganic carbon to organic carbon in coastal saline soils. Soil Ecol. Lett. 4, 328–336. doi:10.1007/s42832-021-0118-y
Song, J., Wan, S., Piao, S., Knapp, A. K., Classen, A. T., Vicca, S., et al. (2019). A meta-analysis of 1,119 manipulative experiments on terrestrial carbon-cycling responses to global change. Nat. Ecol. Evol. 3, 1309–1320. doi:10.1038/s41559-019-0958-3
Tilman, D., Balzer, C., Hill, J., and Beforta, B. L. (2011). Global food demand and the sustainable intensification of agriculture. Proc. Natl. Acad. Sci. U. S. A. 108, 20260–20264. doi:10.1073/pnas.1116437108
Wang, H. L., Tian, C. Y., Jiang, L., and Wang, L. (2014). Remediation of heavy metals contaminated saline soils: a halophyte choice? Environ. Sci. Technol. 48, 21–22. doi:10.1021/es405052j
Wang, Y., Chen, L., Xiang, W., Ouyang, S., Zhang, T., Zhang, X., et al. (2021). Forest conversion to plantations: a meta-analysis of consequences for soil and microbial properties and functions. Glob. Chang. Biol. 27, 5643–5656. doi:10.1111/gcb.15835
Wang, Y., Sun, Q., Liu, J., Wang, L., Wu, X., Zhao, Z., et al. (2022). Suaeda salsa root-associated microorganisms could effectively improve maize growth and resistance under salt stress. Microbiol. Spectr. 10, e0134922–22. doi:10.1128/spectrum.01349-22
Wu, M., Wu, J., Tan, X., Huang, J. S., Jansson, P. E., and Zhang, W. (2019). Simulation of dynamical interactions between soil freezing/thawing and salinization for improving water management in cold/arid agricultural region. Geoderma 338, 325–342. doi:10.1016/j.geoderma.2018.12.022
Xu, T., Zhang, M., Ding, S., Liu, B., Chang, Q., Zhao, X., et al. (2021). Grassland degradation with saline-alkaline reduces more soil inorganic carbon than soil organic carbon storage. Ecol. Indic. 131, 108194. doi:10.1016/j.ecolind.2021.108194
Zhang, C., Sale, P. W. G., and Tang, C. (2016b). Cadmium uptake by Carpobrotus rossii (Haw.) Schwantes under different saline conditions. Environ. Sci. Pollut. Res. 23, 13480–13488. doi:10.1007/s11356-016-6508-5
Zhang, J. G., Xu, X. W., Zhao, Y., Lei, J. Q., Li, S. Y., and Wang, Y. D. (2016a). Effect of shifting sand burial on soil evaporation and moisture–salt distribution in a hyper-arid desert. Environ. Earth Sci. 75, 1417. doi:10.1007/s12665-016-6184-2
Zhang, S., Ni, X., Arif, M., Yuan, Z., Li, L., and Li, C. (2020). Salinity influences Cd accumulation and distribution characteristics in two contrasting halophytes, Suaeda glauca and Limonium aureum. Ecotoxicol. Environ. Safe. 191, 110230. doi:10.1016/j.ecoenv.2020.110230
Zhou, M., Han, R., Ghnaya, T., and Lutts, S. (2018). Salinity influences the interactive effects of cadmium and zinc on ethylene and polyamine synthesis in the halophyte plant species Kosteletzkya pentacarpos. Chemosphere 209, 892–900. doi:10.1016/j.chemosphere.2018.06.143
Zhu, X., Liang, C., Masters, M. D., Kantola, I. B., and DeLucia, E. H. (2018). The impacts of four potential bioenergy crops on soil carbon dynamics as shown by biomarker analyses and DRIFT spectroscopy. GCB Bioenergy 10, 489–500. doi:10.1111/gcbb.12520
Zhu, X., Xie, H., Masters, M. D., Luo, Y., Zhang, X., and Liang, C. (2020). Microbial trade-off in soil organic carbon storage in a no-till continuous corn agroecosystem. Eur. J. Soil Biol. 96, 103146. doi:10.1016/j.ejsobi.2019.103146
Keywords: saline soil, soil salinity, halophytic, desalination, phytoremediation, phytodesalination
Citation: Wang S, Liu J, Wang Y, Liu Y and Tian C (2023) Performance of halophytes in soil desalinization and its influencing factors: a meta-analysis. Front. Environ. Sci. 11:1198540. doi: 10.3389/fenvs.2023.1198540
Received: 06 June 2023; Accepted: 22 November 2023;
Published: 05 December 2023.
Edited by:
Krishnaswamy Jayachandran, Florida International University, United StatesReviewed by:
Bassam Taha Al-Iessa, Qatar University, QatarÂngelo P. Matos, Federal University of Santa Catarina, Brazil
Copyright © 2023 Wang, Liu, Wang, Liu and Tian. This is an open-access article distributed under the terms of the Creative Commons Attribution License (CC BY). The use, distribution or reproduction in other forums is permitted, provided the original author(s) and the copyright owner(s) are credited and that the original publication in this journal is cited, in accordance with accepted academic practice. No use, distribution or reproduction is permitted which does not comply with these terms.
*Correspondence: Jinbiao Liu, bGl1amluNjVAMTYzLmNvbQ==; Changyan Tian, dGlhbmNoeUBtcy54amIuYWMuY24=