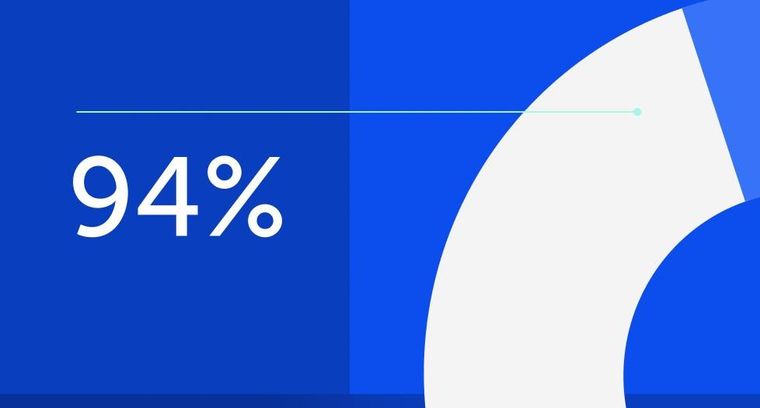
94% of researchers rate our articles as excellent or good
Learn more about the work of our research integrity team to safeguard the quality of each article we publish.
Find out more
REVIEW article
Front. Environ. Sci., 12 June 2023
Sec. Environmental Economics and Management
Volume 11 - 2023 | https://doi.org/10.3389/fenvs.2023.1188643
This article is part of the Research TopicNexus Between Innovations, Environmental Challenges and Labor MobilityView all 13 articles
Technological advances have played a critical role in the production of flower crops, enabling farmers to maximize yields and reduce losses while also improving the quality of flowers. These advances have included the development of new breeding techniques, such as molecular marker-assisted breeding, and the use of modern technologies like high-throughput phenotyping to identify and select superior cultivars. In addition, precision farming techniques, such as the use of sensors and remote monitoring systems, have made it possible to closely monitor crop growth and optimize inputs like water and fertilizer, leading to higher yields and improved resource efficiency. Advancements in biotechnology have also resulted in the development of transgenic plants that are resistant to pests and diseases, reducing the need for chemical pesticides and improving plant health. Modern molecular genetic tools, particularly genome editing with CRISPR/Cas9 nucleases, are emerging in addition to conventional approaches of investigating these plants. Furthermore, the use of novel growing systems, such as hydroponics and vertical farming, has allowed for year-round flower production in controlled environments, mitigating the challenges associated with seasonal changes and climate variability. These innovations have also made it possible to produce high-quality flowers in urban areas, bringing fresh blooms closer to consumers. Overall, technological advances in flower crops have revolutionized the floriculture industry, enabling growers to produce high-quality flowers in a more sustainable and efficient manner. These advancements have not only improved the productivity and profitability of flower farming but have also contributed to the conservation of natural resources and the protection of the environment.
The cultivation of flowers has been intertwined with human civilization for centuries. Ancient cultures like the Egyptians, Greeks, and Romans were known to cultivate and use flowers for various purposes, including as offerings to their gods, for medicinal purposes, and to make fragrances and perfumes (Dafni et al., 2006). Flower production saw a seminal shift when colonial powers used Naval power along with railways to transport planting material across the continents thus bringing hitherto newer ornamental species and cultivars into gardens. As the demand for flowers increased over time, growers began to adopt new technologies to improve the quality and yield of their crops (Wani et al., 2018). The 20th century saw the advent of air and refrigerated transport and hence the ability to move flowers over long distances, particularly in the post-fifties which was the single most contributor to development of floriculture into a trans-continental and global enterprise. Flower production moved to areas around the equator that required less investment in energy in comparison to traditional production areas in northern latitudes. Newer flower types and cultivars were bred that withstood the rigours of long-distance transport, including improved post-harvest technologies and marketing that completed the value chain. Technologies have been rapidly developed and deployed to reduce the collective carbon footprint of the floriculture industry. In the post-2000s, flower industry has undergone the most rapid and paradigm shift in harnessing technologies that have emerged as the world ushered in the 4.0 industrial revolution (Hossen et al., 2020). Today, growers have access to cutting-edge technologies that have revolutionized flower production (Maraveas, 2023). As a result, the ability to handle big data in recent years has allowed the floriculture industry to make use of the most significant technological advances like genetic engineering (Cardoso and Vendrame, 2022; Khan et al., 2022) to create new flower varieties with improved characteristics, such as longer shelf-life, unique colors, and fragrance. By manipulating the plant’s genetic code, breeders can develop flowers that are more resistant to pests and diseases, which reduces the need for harmful chemicals and makes the industry more sustainable (Huylenbroeck, and Bhattarai, 2022). AI and IoT have enabled the flower industry to reap the benefits of precision agriculture. This technology involves the use of sensors, drones, and GPS mapping to optimize crop inputs, reduce waste, and increase yields. By monitoring plant health and growth, growers can adjust irrigation, fertilization, and pest management practices more precisely, which reduces the overall environmental impact of flower production (Belal et al., 2021; Ferroukhi et al., 2023). Automation, robotics, and artificial intelligence (AI) have also played a significant role in reducing labor costs and increasing efficiency in flower cultivation. For example, automated planting and harvesting systems can perform tasks much faster and more accurately than manual labor, reducing the need for human workers (Jha et al., 2019). While these technological advances have greatly improved the quality and yield of flower crops and made the industry more sustainable, there are also potential drawbacks to consider. Genetic engineering raises questions about the safety of modified plants and their potential impact on the environment. Automation and use of artificial intelligence may lead to job losses, particularly in the global south where most of the production areas are currently located. Therefore, it is essential to explore the potential impact of these technological innovations on the environment, economy, and society and use them responsibly to promote sustainable and equitable flower production.
According to a report by the International Trade Centre, the global flower trade was valued at over USD 104 billion in 2019, with exports from developing countries accounting for a significant share of this value. However, the flower industry also faces challenges, including increasing competition, climate change, environmental degradation and gender equity. Technological innovations can help address some of these challenges, but they should be used responsibly and in conjunction with other sustainable practices such as organic farming, biodiversity conservation, and fair labor standards and health issues. In addition, technological advances should be accessible to all stakeholders, including small-scale farmers, and should not contribute to widening the existing economic and social disparities in the flower industry. By promoting sustainable and equitable flower production, we can ensure that this beautiful and culturally significant part of crop husbandry continues to thrive for generations to come. Technology has significantly impacted the floriculture industry, leading to significant advances in production, management, and sustainability. However, the looming challenge is to sustain and promote equity, inclusion and reduction of economic disparity by harnessing fourth industrial revolution in channelling its effects on advances in genetic engineering, climate control, carbon neutrality and making technologies available to all. Previous reviews on ornamental crops have primarily focused on a few technologies as evidenced by several available reviews (Rihn et al., 2022; Lea-Cox et al., 2013; Mahmud et al., 2023) whereas Rihn et al. (2022), on the other hand, examined factors linked to the inclination of the nursery industry to utilize automation and mechanization, and discussed the barriers to adoption for currently available technologies. This review article stands out as a comprehensive overview of available technologies for ornamental crop production. It discusses the potential benefits of using advanced technologies that are already employed in other crop industries and introduces the concept of virtual flowers, a new idea presented in this article. To the best of our knowledge, this is the first review article to delve so deeply into the significant technological advances for the ornamental industry, which have the potential to transform the floriculture industry.
The ornamental industry is continuously enhancing its production, distribution, and marketing processes by leveraging existing agricultural technologies, while also exploring new and emerging ones. Below are several technologies that are either currently being utilized or hold promising potential for adoption within the ornamental industry.
Biotechnology has made significant advances in ornamental crops, such as flowers and decorative plants (Darqui et al., 2017). Tissue culture has been employed to produce a large number of identical plants within short period and efficiently, which can be useful for breeding and propagating new varieties of ornamental crops (Din et al., 2022). This technique can also be used to produce new varieties of plants with desirable traits. Using genetic engineering technology and transgenics, new varieties of ornamental crops with desirable traits, such as brighter colors, longer vase life, resistant to insect pests have been developed (Table 1) (Azadi et al., 2016; Boutigny et al., 2020). Marker-assisted breeding has been used to identify desirable traits in ornamental crops, such as improved color or longer vase life (Ahn et al., 2020; Chandler and Tribe, 2022; Suprasanna and Jain, 2022). This technique can be used to speed up the breeding process and create new varieties of ornamental crops more quickly. RNA interference has been used to silence specific genes in ornamental crops, leading to improved traits such as longer vase life or improved resistance to pests and diseases. Genome editing has been used to make precise edits to the DNA of ornamental crops, leading to improved traits (Table 1) (Sirohi et al., 2022; Ahmad et al., 2023; Jin et al., 2023). Overall, biotechnology has made significant advances in ornamental crops, leading to new varieties of plants with improved traits, increased resistance to pests and diseases, and more sustainable production practices (Din et al., 2021). The spread of the commercialization of GM ornamentals has been impeded by economic and regulatory constraints despite the positive scientific outlook for transgenic flowers. It is now time to deal with the legal challenges to the commercial distribution of GM plants, including flowers. Due to the costs and skills required for commercial development, the release of ornamental items will remain exceedingly challenging in the absence of an internationally suitable and authorised framework for regulation of GM crops. Reduced regulatory requirements for decorative plants and other non-food plants are needed to reduce this nuisance (Noman et al., 2017). By leveraging these advances, horticulturists can produce new varieties of ornamental crops that are more resilient, beautiful, and sustainable. In this review, we have made an effort to highlight recent advances and the need for attention in order to maximise the long-term sustainability of technology and society (Table 1).
TABLE 1. Some specific examples of various biotechnological interventions in improving targeted ornamental traits.
Nanotechnology is a field of science that involves manipulating matter at the nanoscale level, which is typically between 1 and 100 nm in size. In the flower industry, nanotechnology is being explored for various applications, including improving flower quality, disease resistance, and shelf life. One application of nanotechnology in flowers is the use of nanoscale particles to improve the efficiency of fertilizers and pesticides. By attaching fertilizer or pesticide molecules to nanoparticles, growers can increase the efficiency of these products, reducing the amount needed and minimizing environmental impact. Nanoparticles can also be used to deliver nutrients and other compounds directly to plant cells, improving uptake and reducing waste. Another application of nanotechnology in flowers is the development of nanosensors for monitoring plant growth and health. Nanosensors can be designed to detect changes in temperature, humidity, nutrient levels, and other factors that affect plant growth. Nano biosensors have the potential to revolutionize agriculture by not only monitoring soil and plant health but also by predicting outbreaks of pests or diseases. By detecting changes in the biochemical makeup of plants or soil at a molecular level, nano biosensors can provide farmers with early warning signs of potential problems (Rai et al., 2022). This allows them to take preemptive measures to mitigate the impact of these issues, such as applying targeted treatments or adjusting their growing practices. This information can be used to optimize growing conditions and improve flower quality. In addition, nanotechnology can be used to develop materials with unique properties that are useful in the flower industry. For example, researchers are developing nanomaterials that can absorb and release water slowly, helping to maintain proper moisture levels in flower arrangements. Nanomaterials can also be used to develop coatings that improve the durability and longevity of flowers, reducing waste and improving the sustainability of the industry. Cut flowers have ornamental value and are commercially very important, but the flowers’ shelf-life is very short (Solgi et al., 2009), due to higher microbial contamination (Lu et al., 2010a). The early wilting of the flowers is due to microbial and stem barrier infection that causes stem blockage which limits the uptake and transport of water, leading to water imbalances (Lü et al., 2010; Witte et al., 2014). Hence, it is important to overcome stem blockage by controlling microbial infections. Several reports have suggested that nano-silver has the potential to broaden the vase life of cut flowers (Li et al., 2012; Alekasir et al., 2017; Amingad et al., 2017). The most important nanoparticle, graphene oxide (GO) is a graphene-imitating carbon-based NPs containing enormous quantities of oxygenated groups with an extensive surface area that contributes a first-rate capability to transfer nourishment for sluggish-discharge fertilizers (Zhang et al., 2014; Rana et al., 2021). Overall, nanotechnology has the potential to revolutionize the flower industry by improving efficiency, reducing waste, and improving flower quality and sustainability. While many of these applications are still in the research phase, they offer exciting possibilities for the future of flower production and distribution.
Greenhouse technology is widely used in horticulture to create an optimal growing environment for plants. Greenhouses allow growers to control the temperature, humidity, and other environmental factors to create an optimal growing environment for plants (Koukounaras, 2021). This can be particularly important for growing plants in regions with extreme climates or for producing crops out of season. Greenhouses can help to protect plants from pests and diseases by creating a barrier between the plants and the outside environment. This can help to reduce the need for pesticides and other chemical interventions (Messelink et al., 2021). Greenhouses can extend the growing season for plants by providing a sheltered environment that protects them from the elements. This can be particularly important for producing crops out of season or in regions with short growing seasons (Nassar and Ribeiro, 2020; Pereira et al., 2021). Greenhouses can help to conserve water by collecting and recycling irrigation water. This can be particularly important in regions with limited water resources or in areas with high water usage. Greenhouses can help to increase the productivity of horticultural crops by providing a controlled environment that promotes plant growth and reduces the risk of crop loss due to weather or pests. Greenhouses can help to maximize space utilization by allowing plants to be grown vertically or in tightly spaced rows (Stanghellini, 2013). This can help to increase the yield per unit area of land. Overall, greenhouse technology is a key tool in modern horticulture, allowing growers to produce high-quality crops year-round in a controlled environment. Keeping in view the sustainability there is an urgent need to upgrade or device smart greenhouses (Figure 1). Smart greenhouses use sensors and automation technology to monitor plant growth and create an optimal environment for flowers to flourish. This technology can increase crop yields, reduce waste, and improve the overall quality of the flowers. By leveraging the benefits of greenhouse technology, horticulturists can increase productivity, conserve resources, and produce crops that are healthier and more sustainable.
Post-harvest technology in flowers has advanced significantly in recent years, with new technologies and techniques being developed to extend the vase life of cut flowers and improve their quality. Here are some of the key advances in post-harvest technology in flowers. Modified atmosphere packaging (MAP) involves storing cut flowers in an environment with controlled levels of oxygen, carbon dioxide, and humidity. This can help to extend the vase life of cut flowers by reducing respiration rates and slowing the growth of bacteria and fungi. Ethylene is a gas produced by plants that can cause flowers to wilt and decay. New technologies, such as ethylene-absorbing sachets and controlled atmosphere storage, can help to reduce the levels of ethylene around cut flowers, extending their vase life (Yang et al., 2021). Temperature is a critical factor in post-harvest flower care. New techniques, such as hydrocooling and forced-air cooling, can help to reduce the temperature of cut flowers quickly after harvest, reducing respiration rates and extending vase life (Rabiza-Świder et al., 2021). The quality of water used to hydrate cut flowers can affect their vase life. New techniques, such as reverse osmosis and ultraviolet sterilization, can help to improve the quality of water used in post-harvest flower care. New treatments, such as pulsing with sugar or plant hormones, can help to extend the vase life of cut flowers by promoting water uptake and reducing the growth of bacteria and fungi (Ichimura et al., 2007; Kazaz et al., 2019). Research and development in the floriculture industry are ongoing, and new chemicals are continually being developed to improve the vase life of cut flowers. Here are some examples of new chemicals that have shown promising results in recent studies: Nitric oxide (NO): NO is a natural signaling molecule that plays a role in regulating plant growth and development. Studies have shown that treating cut flowers with NO can delay senescence (the aging process) and increase vase life (Hussain et al., 2022). NO can also improve the quality of flowers by enhancing color, scent, and overall appearance. Polyamines are organic compounds found in all living cells, including plants. They are involved in various physiological processes, such as cell division, differentiation, and stress response. Recent studies have shown that treating cut flowers with polyamines, such as putrescine and spermidine, can improve their vase life by reducing senescence and preserving flower quality (Qu et al., 2020; Mazrou et al., 2022). Chitosan is a biodegradable polymer derived from chitin, which is found in the shells of crustaceans. It has antimicrobial and antifungal properties and has been shown to improve the vase life of cut flowers by reducing microbial growth and maintaining water balance (Ali et al., 2022). Essential oils: Essential oils are volatile compounds extracted from plants, and they have been used for centuries in various applications, including aromatherapy and medicine. Recent studies have shown that treating cut flowers with essential oils, such as lavender and rosemary, can improve their vase life by reducing microbial growth and enhancing flower quality (Teerarak et al., 2019; El-Sayed et al., 2021). Overall, advances in post-harvest technology in flowers are helping to improve the quality and longevity of cut flowers, reducing waste and improving the sustainability of the floral industry. By leveraging the latest technologies and techniques, horticulturists can produce high-quality flowers that retain their beauty and freshness for longer periods of time.
Irrigation technology has been playing an essential role in flower crop production by providing the right amount of water to the plants, increasing crop yield, and optimizing the use of water resources. In general, irrigation technology in flower crops involves the application of water in the right amount and at the right time, using advanced systems and methods. One of the main advances in irrigation technology for flower crops is the use of drip irrigation systems. These systems provide water directly to the root zone of plants, minimizing water loss due to evaporation and runoff. They also allow for precise control of water application, reducing the risk of over- or under-watering. Another irrigation technology that has gained popularity in recent years is the use of soil moisture sensors. These sensors measure the moisture content in the soil and provide real-time data to growers, allowing them to adjust irrigation schedules and avoid water stress or over-watering. In addition, advanced irrigation controllers are now available that use weather data to adjust irrigation schedules automatically based on the local climate conditions, further optimizing water use. Finally, fertigation technology is also being used in flower crops, where fertilizers are injected into the irrigation system to provide nutrients directly to the roots. This approach ensures that the plants receive the necessary nutrients for healthy growth while minimizing fertilizer waste and runoff. Overall, irrigation technology has revolutionized flower crop production by providing precise control over water and nutrient application, resulting in increased yields, improved quality, and reduced water usage. Automation through an IoT system could be an effective approach to improve a conventional surface irrigation system operation. An automated surface irrigation system refers to its operation with timers, sensors or computers or mechanical appliances with minimal manual involvement (Figure 2). Many researchers have reported that automation in irrigation projects using an intelligent irrigation controller and wireless sensor network could save water up to 38% (Al-Ghobari, et al., 2013; Bowlekar et al., 2019). Automation is a smart technique to deal with the problem of high labor requirements and low water application efficiency of surface irrigation systems (Table 2). Many soil moisture sensors such as tensiometer, gypsum block, granular matrix sensor, time-domain reflectometer, dielectric probe are commercially available for soil moisture measurement and they could generally be used for manual or integrated with automatic irrigation control systems through an IoT system (Bowlekar et al., 2019; Hardie, 2020; Vera et al., 2021; Pramanik et al., 2022). The sensor senses the water advance front and gives a signal to cut-off the flow.
Smart irrigation systems using Internet of Things (IoT) technology have been successfully implemented for ornamental crop production. For example, Banda-Chávez et al. (2018) developed an IoT-based sensor network using an IoT platform and soil moisture sensors (YL-69) to automate the irrigation of ornamental plants (Figure 2). Beeson and Brooks (2006) also used an evapotranspiration (ETo) model-based smart irrigation system for wax-leaf privet, reducing water usage by 22.22% annually compared to traditional overhead irrigation methods. While there are limited studies on IoT-based automatic irrigation systems for the ornamental industry, the promising potential of this technology in other crop industries suggests it could benefit ornamental crop production. However, sensor-to-sensor variability and accurate sensor positioning are important factors that can affect efficacy. Determining the optimal number of sensors for a particular nursery environment depends on various factors such as the accuracy and repeatability of the sensors, variation among sensors, spatial variability of the nursery environment, and cost.
Limited resources, such as fertile soil and clean water, are already a reality in many parts of the world. Climate change further exacerbates the challenges of conventional farming practices, as the availability and quality of arable land become increasingly limited. Soilless cultivation, also known as hydroponics, is a method of growing plants without soil, using a nutrient-rich solution instead. By eliminating the need for soil, soilless cultivation systems can help conserve water and open up urban areas, such as residential rooftops, for food production in close proximity to consumers (Fussy and Papenbrock, 2022). The development of automation and computer technology, coupled with the greenhouse feature, has accelerated the adoption of soilless cultivation in many developed countries in recent years. Conventional farming practices typically rely on soil-bound methods, which can have a range of negative impacts on the environment, including high and inefficient water demand, large land requirements, fertilizer use, soil degradation, and loss of biodiversity. It is imperative to explore alternative approaches to food production, such as soilless cultivation, to address these challenges and ensure sustainable agricultural practices for the future (Killebrew and Wolff 2010; Walls 2006). In the flower industry, soilless cultivation is gaining popularity due to its ability to improve crop yields, reduce water consumption, and minimize the use of pesticides and fertilizers (AlShrouf, 2017). One of the benefits of soilless cultivation in flowers is the ability to control growing conditions more precisely. By controlling the temperature, humidity, and nutrient levels of the growing solution, growers can optimize plant growth and flower quality (Zimmermann and Fischer, 2020). Soilless cultivation also eliminates the need for soil, which can reduce the risk of soil-borne diseases and pests (Savvas, 2002; Hussain et al., 2014). There are several methods of soilless cultivation that can be used in the flower industry (Kahraman and Akçal, 2018; Khalaj and Noroozharaf, 2020; Kromwijk and van Os, 2020). For example, one common method is to grow flowers in a nutrient-rich solution that is recirculated through a series of pipes or channels. This method is known as a recirculating hydroponic system. Another method is to grow flowers in a substrate, such as rockwool or coco coir, which is soaked in a nutrient-rich solution (Leiva et al., 2019; Kharrazi et al., 2020). This method is known as a substrate-based hydroponic system. Soilless cultivation can also be combined with other technologies to further improve flower production. For example, some growers use vertical farming techniques to maximize space utilization and improve growing conditions. Vertical farming involves growing plants in stacked layers, using artificial lighting and environmental controls to optimize plant growth. Overall, soilless cultivation has the potential to revolutionize the flower industry by improving crop yields, reducing water and fertilizer consumption, and minimizing the risk of pests and diseases. By using nutrient-rich solutions and advanced environmental controls, growers can produce high-quality flowers with less waste and environmental impact (Figure 3).
Aeroponics is a modern agricultural technique that involves growing plants in an air or mist environment without soil. Instead of soil, plants are grown in a nutrient-rich solution that is misted onto the roots. Aeroponics is becoming increasingly popular in flower crop production because of its many advantages (Nir, 1982). One advantage of aeroponics is that it allows for more efficient use of resources like water and nutrients. Because the nutrient solution is misted onto the roots, plants can absorb more of the nutrients and water than they would if they were grown in soil. This can lead to faster growth and higher yields. Another advantage of aeroponics is that it reduces the risk of disease and pests. Because plants are grown in a sterile environment, there is less chance of soil-borne diseases or pests affecting the crop. This can reduce the need for pesticides and other chemicals, making aeroponics a more sustainable and environmentally-friendly option. Aeroponics can also be used to grow flowers year-round, regardless of weather conditions. This makes it possible to produce flowers in areas where traditional outdoor growing methods may not be feasible. The performance of the system has been tested successfully (growing and rooting) with several plants, such as ornamental plants like carnation, croton, chrysanthemum, Eustoma geranium, euonymus, Ficus, philodendron, dracaena, dieffenbachia, Zantedeschia, etc (de Kreij and van der Hoeven, 1997; Christie and Nichols, 2004; Hayden, 2006; Fascella and Zizzo, 2007). In order to analyse plant root systems for a variety of studies, researchers need a practical growing system. An aeroponic system that was created by a French engineer and modified by members of the legume community, was detailed by Cai et al. (2023) and was initially intended for nodulating legume root systems. For researchers looking to better understand root growth and development, this approach offers various benefits. With this aeroponic device, researchers can grow hundreds of plants at once. Even though it is not a sterile system, it may be kept axenic in the lab with appropriate procedures. During plant growth, the nutritional medium can be changed based on the demands of the experiment. Overall, aeroponics is a promising technology for flower crop production, offering many benefits over traditional growing methods. However, it requires careful monitoring and management to ensure optimal plant growth and health.
Vertical farming is a growing trend in the agriculture industry, and it is also being explored for flower production. Vertical farming in flowers involves growing plants in vertical layers, often in controlled environments, such as indoor facilities or greenhouses (Touliatos et al., 2016). Numerous countries including Korea, Japan, China, Germany, the United Arab Emirates, China, France, India, Sweden, Singapore, and the United States, have convened to discuss vertical farming. They have repeatedly endorsed the concept as integral to the long-term sustainability of their cities (Despommier, 2014). Vertical farming can provide several benefits to flower growers (Figure 4). Firstly, it allows for the efficient use of space, as plants can be stacked vertically, increasing the amount of production per unit area of land. Furthermore, indoor farming provides a low-impact system that can significantly reduce travel costs, as well as reduce GHG emissions, by cutting down on travel distances between distant farms and local markets (Astee, and Kishnani, 2010; Mukherji and Morales, 2010). Also, vertical farming could ignite local economies by providing much-needed “green collar” jobs to urban areas (Healy and Rosenberg, 2013; Mukherji and Morales, 2010). This is particularly advantageous in urban areas where land is limited and expensive. Secondly, vertical farming can offer precise control over growing conditions, such as temperature, humidity, light, and nutrient levels, ensuring consistent plant growth and reducing the risk of disease and pest infestations (Al-Kodmany, 2018). By using artificial lighting and hydroponic or aeroponic systems, vertical farms can provide optimal growing conditions for flowers, resulting in higher quality and more consistent blooms (Despommier, 2010; Eve, 2015; Levenston, 2017; Meinhold, 2017). Thirdly, vertical farming can reduce the need for pesticides and herbicides, as plants are grown in a controlled environment with fewer pests and diseases. This reduces the environmental impact of flower production and provides safer working conditions for growers. Finally, vertical farming can also reduce water usage, as hydroponic and aeroponic systems recycle water, reducing waste and conserving resources. While vertical farming in flowers is still in its early stages, it has the potential to transform flower production by providing a more sustainable, efficient, and cost-effective way to grow flowers. With further research and development, vertical farming could become a key method of flower production in the future.
Green roofs and walls are becoming more popular in urban areas as a way to add green space and reduce the urban heat island effect. Advances in materials and installation techniques have made it easier and more cost-effective to install and maintain these features. Green roofs and walls are innovative and sustainable solutions to improve urban environments by incorporating vegetation into buildings. Green roofs are roofs that are covered with plants and vegetation, often including a layer of soil and a drainage system, to support the growth of vegetation. They can provide several benefits, including reducing urban heat island effects, improving stormwater management by retaining rainwater, and providing additional insulation to buildings to reduce energy consumption (Mayrand and Clergeau, 2018). They can also provide habitat for wildlife and improve air quality by filtering pollutants (Haaland and van den Bosch, 2015). Green walls, also known as living walls or vertical gardens, are similar to green roofs, but they are installed vertically on the side of buildings. They can be made up of a variety of plant species and can be used for both aesthetic and functional purposes. Green walls can also provide similar benefits to green roofs, such as improving air quality and reducing urban heat island effects. Both green roofs and walls require proper installation and maintenance to ensure their long-term viability. They can also provide unique opportunities for urban agriculture and rooftop gardens, creating additional benefits for local communities. Overall, green roofs and walls are sustainable solutions to improve urban environments and promote green infrastructure (Elmqvist et al., 2015).
Green roofs and walls can support a variety of vegetation, including flower crops. In fact, adding flower crops to green roofs and walls can increase their aesthetic value and provide additional benefits to the environment. Flower crops such as sedum, lavender, and wildflowers are often used in green roofs due to their hardiness and ability to withstand harsh weather conditions. They can also attract pollinators, such as bees and butterflies, which play an important role in the pollination of plants. When it comes to green walls, flower crops can be arranged in different patterns and designs to create beautiful living walls (Pétremand et al., 2017). For instance, a mix of flowering plants such as petunias, pansies, and geraniums can be used to create a colorful and vibrant wall. In addition to their aesthetic benefits, green roofs and walls with flower crops can also provide environmental benefits such as reducing urban heat island effects and improving air quality (Ode et al., 2023). They can also provide opportunities for urban agriculture, such as growing edible flowers and herbs (Li et al., 2016). However, it is important to note that green roofs and walls with flower crops require regular maintenance to ensure their health and viability. This includes proper irrigation, fertilization, and pest management. Overall, incorporating flower crops into green roofs and walls can be a sustainable and beautiful solution for urban environments.
Robotics is a rapidly developing field that is also making strides in the floriculture industry. Despite the clear benefits of existing approaches to robotizing harvesting operations in gardens, the relationship between a robot’s degree of autonomy and its ability to perform multiple agricultural tasks on a single platform has not been thoroughly investigated. Developing effective calculation methods will be crucial to unlocking the full potential of mobile robots by increasing their autonomy and expanding their functionality. At the same time, the issues of increasing the technical efficiency of solutions aimed at achieving a high level of robot autonomy require development. This can be achieved by applying intelligent approaches to the complex processing of data coming from a complex of information devices (Bechar et al., 2016; Grimstad, 2017) Robotics in flower crops involves the use of automated and autonomous systems to perform various tasks related to the cultivation, care, and harvesting of flower crops (Abarna and Selvakumar 2015). Robotic mowers, trimmers, and other automated maintenance equipment can now be used to maintain lawns and other outdoor spaces (Oliveira et al., 2021). In addition, the availability of a skilled workforce that accepts repetitive tasks in uncomfortable greenhouse conditions is decreasing rapidly, causing a reduced availability of workforce (Adegbola et al., 2019; Arad et al., 2020). Furthermore, the issue of labor shortage has become even more relevant during the current COVID-19 pandemic caused by the SARS-CoV-2 virus, which has limited international travel for migrant workers (Woo et al., 2020). These machines can work continuously and efficiently, reducing the need for manual labor and improving overall maintenance quality. One significant benefit of using robotics in flower crops is the potential for increased efficiency and productivity. Robots can work 24/7, perform tasks with precision and consistency, and work in harsh environments without risking the health and safety of human workers. Robots can be used to perform tasks such as planting, pruning, weeding, and harvesting (Sori et al., 2018; Uchida et al., 2019; Verbiest et al., 2020; Tarannum et al., 2021). For example, robots equipped with sensors, cameras, and other technology can navigate fields, identify flowers, and accurately harvest them with minimal damage to the plant. Some examples of flower harvest robots currently on the market include the Flower Robotics’ “Ryden” and the “Harvest Croo” system, which is designed specifically for harvesting strawberries but could potentially be adapted for use with other types of flowers. QuickFlora is testing another robot ‘FloraBot’ to automate the flower arrangement process. FloraBot designs advanced technologies which enable customers to automate the handling and assembly of fresh flower arrangements something that was impossible in the past (www.flora.bot). Everything starts with a vision of how to increase efficiency, improve product quality and provide scalability when needed, using flower-friendly robotics. In simple terms, robots do things that have not been possible before in mass-production floral environments. We are able to automate the assembly of floral products and processes that were previously off-limits to automation. These robots can also monitor plant growth and detect any diseases or pests that could harm the crops, allowing for quicker response times to prevent the spread of disease and reduce the need for chemical treatments (Figure 5). Similar robots can be designed using a combination of computer vision and machine learning algorithms to recognize flower types and colors and arrange them into a desired pattern or design. The robots can be equipped with a specialized gripper that can pick up and handle delicate flowers without damaging them. Similarly, “Starship robots” are designed to transport food packages over short distances and have been successfully used to deliver flowers to customers (www.starship.xyz). They are operated by Starship Technologies, a company founded by two Skype co-founders, Ahti Heinla and Janus Friis.
FIGURE 5. Conceptual design of robotic systems for performing various tasks in ornamental nurseries, (A) plant health monitoring, and (B) plant carrier platform with loading and unloading system.
An automated system for harvesting Gerbera jamesonii cut flowers grown in greenhouses has been developed using image processing (Kawollek, and Rath, 2008). The harvesting process uses an industrial robot with six axes and an additional linear axis, equipped with an end-effector using razor blades for cutting the flower stems. The whole harvesting system, including the robots, end-effectors, transport unit, and cameras, is calibrated online using special calibration algorithms. The system analyzes image data from eight different viewpoints to identify all flower stems in at least one of the positions, enabling stepwise harvesting, if necessary, in different positions of the plant. To ensure a collision-free process, a path-planning module is integrated, and an algorithm for flower stem tracking predicts the emerging of the stem in the following non-visible area. In harvesting experiments, 80% of all flower stems were harvested, with a decreasing rate of harvest with an increasing number of flower stems per plant. For plants with one or two flower stems, 98% of the flower stems were harvested, while for plants with five or more flower stems, 51% of all flower stems were harvested. Al-beeshi et al. (2015) designed a self-propelled robot, which can analyze soil moisture and monitor and adjust the water pump’s condition in order to activate the soil watering function. A multi-task robotic work cell for greenhouse transplanting and seedlings has been developed by Han et al. (2018). The work cell mainly consists of two conveyors, a filling unit, a control system, and a transplanting system, which is made up of multi-grippers designed to automatically pick up and plant whole rows of seedlings. Several studies have been conducted on the development of robots that can perform various agricultural tasks with precision and accuracy. Chang et al. (2016) developed a low-cost planting robot that can navigate straight lines with great accuracy. The robot is equipped with a drilling mechanism that can dig up to 30 cm depth, and an ultrasonic sensor is used to detect the drilling depth. Gao et al. (2017) designed a prototype of a robot sprayer that can adjust the spray angle according to crop height, canopy shape, thickness, and plant density. The system uses magnetic sensors installed on the tracks to detect the canopy ridge. Wang et al. (2022) designed an intelligent and modular greenhouse seedling inspection robot that can acquire images of seedlings and environmental data during cultivation. The robot’s environmental data collection module can accurately obtain data on the light intensity, temperature, humidity, and CO2 concentration in the greenhouse. Masuzawa et al. (2017) developed a mobile robot capable of supporting flower harvesting by utilizing a simultaneous localization and mapping (SLAM) algorithm to map the environment. The robot has an RGB-D camera installed not only to measure the distance to objects but also to allow for person-following capability. The robot uses image-based person detection and tracking and trajectory generation for following movements to trace the person’s trajectory as a guide to safe path planning. Ohi et al. (2018) utilized the same technology to develop a robot that can perform fully autonomous precision pollination of bramble plants in a greenhouse environment. The robot uses vision techniques to detect the position of flowers for pollination, and an RGB-D camera installed on the robotic arm enables precise short-range positioning. These advancements in robot technology show great potential for improving agricultural practices and increasing efficiency in various tasks, from planting and spraying to harvesting and pollination. While the use of robotics in flower crops is still in its early stages, it has the potential to significantly improve the efficiency, quality, and sustainability of flower production. However, the development and adoption of flower harvest robots face challenges such as cost, technical complexity, and the need for further refinement to ensure they can operate in diverse field environments. By leveraging robotics, floriculturists can maximize their yields, reduce labor costs, and minimize their environmental impact, leading to a more sustainable and profitable industry.
Imaging technology has become an important tool for the floriculture industry. Imaging technology in flower crops involves the use of advanced cameras and sensors to capture detailed images of plants, flowers, and their surrounding environments. These imaging techniques include visible imaging (machine vision), imaging spectroscopy (multispectral and hyperspectral remote sensing), thermal infrared imaging, fluorescence imaging, 3D imaging and tomographic imaging (MRT, PET and CT) (Li et al., 2014). These images are then analyzed using advanced algorithms and computer programs to provide insights into plant growth, development, and health. One of the primary applications of imaging technology in flower crops is for the detection and diagnosis of plant diseases and pests (Saleem et al., 2020). By capturing high-resolution images of plants and analyzing them using artificial intelligence and machine learning algorithms, floriculturists can quickly and accurately detect early signs of disease or pest infestations, allowing for timely intervention and treatment (Sasaki et al., 1999; Chaerle et al., 2007). Imaging technology can also be used to monitor plant growth and development, including factors such as leaf area, stem diameter, and flower size. This information can be used to optimize growth conditions, such as lighting, temperature, and irrigation, to maximize plant health and productivity (Nakarmi and Tang, 2012; Tilly et al., 2012; Longchamps et al., 2023).
Plant phenotyping robots are cutting-edge technology that allows for the high-throughput measurement of morphological, chemical, and physiological properties of a large number of plants. Multiple robotic systems have been developed for different phenotyping missions. Robotic phenotyping has the potential to efficiently monitor changes in plant traits over time, both in controlled environments and in the field (Atefi et al., 2021). Various studies have discussed the use of image-based techniques, including 2D and 3D reconstruction, to extract architectural traits such as inflorescence length and width, inflorescence volume (weight), grain shape and size, grain angle, number of grains, and number of flowers (Faroq et al., 2013; Crowell et al., 2014; Gage et al., 2017; Rudolph et al., 2019). To measure morphological traits in plant phenotyping, a robot equipped with LIDAR or a camera can automatically capture images or point cloud data from various angles of the inflorescence. Physiological traits are important indicators of stress or disease in plants. For example, the temperature of the spikes has been used to detect water stress in plants (Panozzo et al., 1999). A robotic arm equipped with a temperature sensor can potentially grasp the spike and insert the sensor into spikelets to record their temperature. In fruit quality assessment, various properties such as water content, sugar content, chlorophyll, carotenoid, soluble solids, acidity, and firmness need to be measured. Spectroscopy and spectral imagery are non-destructive and high-throughput methods that can be used to estimate these qualitative parameters (Atefi et al., 2021; Shao and He, 2008; Wu et al., 2008; Nishizawa et al., 2009; Penchaiya et al., 2009; Ecarnot et al., 2013; Guo et al., 2013; Dykes et al., 2014; Wang et al., 2015; Mancini et al., 2020). A robotic system can be utilized to monitor the dynamics of fruit attributes for hundreds of growing fruits per day. For instance, a portable spectrometer can be attached to the robot’s end-effector. The robot can detect the fruit on the plant, grasp it, and gather its spectral data to infer its quality parameters. This approach offers a high-throughput and non-destructive method for fruit quality assessment, enabling efficient monitoring of fruit development over time. In addition, imaging technology can also be used to assess the quality of flowers, providing insights into factors such as color, shape, and size, which are important for marketability and consumer appeal (Prunet and Duncan, 2020). Overall, imaging technology is proving to be a valuable tool in the floriculture industry, providing floriculturists with the ability to detect and treat plant diseases, optimize growth conditions, and improve the quality of their products.
Computerized monitoring systems in agriculture are technology-driven systems that use sensors and automated data collection to track and monitor various aspects of crop production. These systems can provide farmers with real-time data on soil moisture, temperature, weather patterns, and other environmental factors, allowing them to make more informed decisions about irrigation, fertilization, and other inputs. Computerized monitoring systems can also be used to detect and respond to potential problems, such as pest infestations or disease outbreaks before they have a chance to cause significant damage. Overall, computerized monitoring systems are helping to improve the efficiency and productivity of agricultural operations, while also reducing waste and environmental impact.
Data analytics is playing an increasingly important role in agriculture, helping farmers to make more informed decisions and improve crop yields. Here are some of the key areas of data analytics in agriculture: Predictive analytics: Predictive analytics uses data analysis techniques to make predictions about future outcomes, such as crop yields or weather patterns. This information can help farmers to make more informed decisions about planting, harvesting, and other aspects of agricultural production (Lassoued et al., 2021). Precision agriculture: Precision agriculture involves using data analytics to optimize crop production by tailoring inputs such as water, fertilizer, and pesticides to specific areas of a field (Griffin et al., 2018; Akhter et al., 2021). This approach can improve crop yields while reducing waste and environmental impact. Remote sensing: Remote sensing involves using satellites, drones, and other technologies to collect data on crop health, soil moisture, and other environmental factors. This data can be used to identify areas of a field that require additional inputs or interventions. Data visualization tools can be used to display data in an easy-to-understand format, helping farmers to identify trends and make informed decisions (Kumar et al., 2019a; 2019b). For example, farmers can use data visualization tools to track weather patterns, crop yields, and other key metrics. Supply chain analytics: Supply chain analytics can be used to optimize the distribution and delivery of agricultural products, reducing waste and improving efficiency. Overall, data analytics is transforming agriculture by providing farmers with real-time data on crop health, weather patterns, and other environmental factors. By leveraging the power of data, farmers can make more informed decisions about planting, harvesting, and other aspects of agricultural production, contributing to a more sustainable and secure food supply.
The Internet of Things (IoT) is a new technology that allows devices to connect remotely to achieve smart farming (Patil and Kale, 2016). The IoT has begun to influence a vast range of industries, from health, trade, communications, energy and agriculture, to enhance efficiency and performance across all markets (Elijah and Rahman, 2018; Sisinni et al., 2018; Shi et al., 2019). The Internet of Things (IoT) is a network of connected devices and sensors that communicate and exchange data with each other. In the flower industry, IoT is being used to improve the efficiency of flower production and supply chain management. IoT sensors can be used to monitor environmental conditions such as temperature, humidity, and light levels, which are critical factors in flower growth and development. By collecting data on these factors, growers can optimize growing conditions to ensure the best quality and yield of flowers. For example, sensors can be used to adjust the temperature and humidity levels in a greenhouse to create the ideal environment for flower growth. IoT can also be used to monitor the health and growth of flowers. For instance, sensors can be placed on individual plants to measure their growth rate, nutrient uptake, and water consumption. This can help growers to identify any issues early on and take corrective measures before they become serious. The Internet of Things (IoT) technology enables the remote monitoring of plants and animals, retrieving information from mobile phones and other devices. Through the use of sensors and instruments, farmers can assess weather patterns and anticipate production levels. The IoT also plays a crucial role in water management, allowing for the monitoring and control of water flow, assessment of crops’ water requirements, and optimization of water usage, like never before (Yong et al., 2018). With sensors and cloud connectivity through the gateway, the status of the water supply can be remotely monitored based on soil and plant needs (Mekala et al., 2017). The benefits of IoT technology extend to correcting nutrient deficiencies, pests, and diseases, as farmers cannot manually monitor every plant. This technology has ushered farmers into a new era of modern agriculture (Mittal and Singh, 2007). In addition, IoT can be used to improve supply chain management by providing real-time data on the location and condition of flowers during transportation. IoT sensors can track the temperature, humidity, and other conditions during shipping and alert growers and distributors if there are any deviations from the ideal conditions. Overall, IoT has the potential to improve the efficiency and quality of flower production and supply chain management. By using IoT sensors and devices, growers and distributors can optimize growing conditions, reduce waste, and ensure the best quality and freshness of flowers for consumers.
Artificial intelligence (AI) is a broad term that refers to the ability of machines to perform tasks that typically require human intelligence, such as learning, problem-solving, and decision-making. In the flower industry, AI is being used to improve flower production and quality, as well as to optimize supply chain management. One application of AI in flowers is to analyze and interpret data from sensors and cameras to optimize growing conditions. AI algorithms can be used to analyze large datasets of environmental and plant growth data to identify patterns and make predictions about the optimal conditions for growing different types of flowers (Figure 6). For example, AI algorithms can help growers optimize the amount of water, fertilizer, and light that flowers receive, which can improve yield and quality (Song and He, 2007; Eli-Chukwu, 2019; Vincent et al., 2019). Another application of AI in flowers is to improve disease detection and prevention (Adedoja et al., 2019). AI algorithms can be trained to recognize the symptoms of different plant diseases and to detect them early on. This can help growers take corrective measures before the disease spreads and affects the entire crop. AI can also be used to develop predictive models for disease outbreaks based on environmental and weather data. In addition, AI can be used to optimize supply chain management by analyzing data from sensors and cameras to monitor the condition and location of flowers during transportation (Figures 5, 6). AI algorithms can predict the best routes and transportation methods for delivering flowers while minimizing waste and reducing costs (Wang et al., 2017). Overall, AI has the potential to improve the efficiency, quality, and sustainability of flower production and supply chain management. By analyzing data and making predictions (Talaviya et al., 2020), AI can help growers optimize growing conditions, detect and prevent diseases, and improve supply chain logistics for delivering high-quality flowers to consumers.
Machine learning is a type of artificial intelligence that enables computers to learn from data and improve their performance over time without being explicitly programmed. In the flower industry, machine learning is being used to improve flower production and quality, as well as to optimize supply chain management. One application of machine learning in flowers is to analyze and interpret data from sensors and cameras to optimize growing conditions (Benos et al., 2021). Machine learning algorithms can be used to analyze large datasets of environmental and plant growth data to identify patterns and make predictions about the optimal conditions for growing different types of flowers. For example, machine learning algorithms can help growers optimize the amount of water, fertilizer, and light that flowers receive, which can improve yield and quality (Sun et al., 2019; Virnodkar et al., 2020). Another application of machine learning in flowers is to improve disease detection and prevention. Machine learning algorithms can be trained to recognize the symptoms of different plant diseases and to detect them early on. This can help growers take corrective measures before the disease spreads and affects the entire crop. Machine learning can also be used to develop predictive models for disease outbreaks based on environmental and weather data. In addition, machine learning can be used to optimize supply chain management by analyzing data from sensors and cameras to monitor the condition and location of flowers during transportation (Liakos et al., 2018; Zhang J. et al, 2021). Machine learning algorithms can predict the best routes and transportation methods for delivering flowers while minimizing waste and reducing costs.
The integration of IoT, AI, and machine learning has significant potential to revolutionize flower production and supply chain management (Figure 7). IoT sensors and devices can help growers and distributors optimize growing conditions and reduce waste, while AI and machine learning can help them make data-driven decisions to improve efficiency, quality, and sustainability. For instance, the use of IoT sensors can monitor factors such as soil moisture, temperature, and light levels, helping growers create the ideal growing conditions for each flower variety. This can lead to increased yields, reduced water usage, and lower energy costs. Additionally, IoT devices can monitor the flowers’ post-harvest conditions, ensuring they are stored and transported at optimal temperatures to maintain their quality and freshness. The use of AI and machine learning can further enhance flower production and supply chain management. By analyzing vast amounts of data, AI can help growers make informed decisions on when to harvest their crops, reducing waste and increasing efficiency. AI can also predict and detect diseases early, allowing growers to take preventive measures and reduce the need for harmful pesticides. Furthermore, machine learning can help growers optimize their logistics and supply chain management, ensuring that flowers reach consumers quickly and efficiently. By analyzing historical data on demand and supply, machine learning can help growers forecast demand accurately, reducing overproduction and minimizing waste. In conclusion, the integration of IoT, AI, and machine learning can help flower growers and distributors to create a more efficient, sustainable, and high-quality supply chain. By leveraging these technologies, they can reduce waste, improve growing conditions, and deliver fresher, higher-quality flowers to consumers. This not only benefits the flower industry but also helps promote sustainability and environmental protection.
FIGURE 7. The Future of Ornamental industry: Unleashing the Potential of integrated IoT, AI, and Robotics.
RFID (Radio Frequency Identification) technology is an automated identification system that uses radio waves to transfer data between a tag attached to an object and a reader. A Radio Frequency Identification (RFID) tag functions as a barcode that can hold information (Kumar and Srivastava, 2014). There are two main types of RFID tags: active and passive, with a variety of shapes and forms available for different applications (Fernandez, 2014). Passive tags can be made from paper, plastic, or vinyl (Want, 2006) and are designed to withstand continuous exposure to the elements, including water, heat, dirt, and chemicals, making them suitable for outdoor nursery production environments. Each RFID label has a unique identification code and can be encoded with important production information such as genus, species, cultivar, and planting dates. Passive RFID labels are relatively inexpensive, and as adoption increases, costs are expected to decrease due to economies of scale. These labels are read using a specific UHF radio frequency with an RFID interrogator. One of the advantages of RFID in agricultural operations is that it does not require a clean label, unlike barcodes which can be obscured by dirt or damage (Ruiz-Garcia and Lunadei, 2011). RFID tags have been used for tagging and tracking animals and plants, health monitoring (Wang et al., 2006), identifying and tracking livestock, and monitoring irrigation system management (Floyd 2015; Deng et al. 2020).
In the flower industry, RFID technology is used for tracking and tracing flowers from the field to the store, providing greater transparency and efficiency in the supply chain (Ampatzidis et al., 2009; Jones et al., 2005; Verdouw et al., 2013). One application of RFID in flowers is the tracking of individual flower stems from the time they are harvested to the time they are sold. Each stem can be tagged with an RFID tag, which contains information about the grower, variety, and other key details. As the flowers move through the supply chain, RFID readers can be used to track their location and monitor temperature and humidity levels, ensuring that the flowers are properly handled and stored (Luvisi et al., 2010). RFID tags are also used to monitor temperature and humidity during transport, ensuring that the flowers are kept in optimal conditions (Dose et al., 2021). RFID technology can also be used to improve inventory management and reduce waste in the flower industry. By tracking the movement of flowers in real-time, retailers can better manage their inventory levels, reducing the risk of overstocking or stockouts. This can help to reduce waste by ensuring that flowers are sold before they spoil or lose their freshness.
Another application of RFID in flowers is the ability to provide consumers with information about the flowers they are purchasing. By scanning the RFID tag with a smartphone or other device, consumers can access information about the flower’s origin, growing conditions, and other details. This can help to build trust between consumers and growers and promote greater sustainability and transparency in the industry. Overall, RFID technology offers numerous benefits for the flower industry, including improved supply chain efficiency, better inventory management, and greater transparency for consumers. As RFID technology continues to evolve, its use in the flower industry will likely become even more widespread and impactful.
LED (Light Emitting Diode) technology is revolutionizing the way flowers are grown by providing a more efficient and customizable light source for plants (Al Murad et al., 2021). LEDs are more energy-efficient than traditional light sources, and they can be tuned to specific wavelengths that optimize plant growth and flowering. One application of LEDs in flower cultivation is in the production of cut flowers. By using LEDs to provide the optimal light spectrum for flower growth, growers can achieve faster growth, increased flower size, and improved color and fragrance. This can help to increase yield and quality while reducing production costs, as LEDs use less energy than traditional lighting methods (Table 3). Another application of LEDs in flowers is in the production of potted plants and indoor flowers (Mills-Ibibofori, et al., 2019; Kang et al., 2019). LEDs can be used to provide a specific light spectrum that promotes healthy growth, even in low-light environments. This can be particularly useful in urban areas or areas with limited access to natural light, where plants may struggle to thrive without artificial lighting. In addition, LEDs can be used to control the timing and quality of flower blooms (Paradiso and Proietti, 2022). By adjusting the light spectrum and duration, growers can induce flowers to bloom at specific times of the year or in response to certain environmental cues. This can be particularly useful for the production of holiday flowers, such as Cyclamen, chrysanthemums, poinsettias, gerberas, petunias, Easter lilies (Heo et al., 2003; Higuchi et al., 2012; Park et al., 2016; Mills-Ibibofori, et al., 2019). However, in general, studies investigating the effects of LED light on flower crops typically vary in terms of the specific flower species (Mills-Ibibofori et al., 2019) being studied, the type and intensity of the LED light used, the duration and timing of the light treatments, and the specific growth or developmental parameters being measured (Craig and Runkle, 2013; Park and Runkle, 2016; Matysiak, 2021; Vendrame et al., 2022). These studies often aim to optimize plant growth and development, increase yield, improve flower quality and color, or reduce disease incidence (Cioc et al., 2022; Rashidi et al., 2023). Results can vary widely depending on these factors, and further research is needed to better understand the effects of LED lighting on flower crops under different growing conditions. Overall, LEDs offer numerous benefits for the flower industry, including improved growth and flowering, reduced energy costs, and greater control over bloom timing and quality. As LED technology continues to evolve, it is likely that its use in the flower industry will become even more widespread and impactful.
Virtual flowers refer to computer-generated images or animations of flowers that are created using software programs and displayed on electronic devices such as computers, smartphones, or virtual reality headsets. Virtual flowers can be used in a variety of ways, including as digital decorations for websites or social media posts, as 3D models for gaming or animation, and as virtual bouquets or gifts. Virtual flower gardens can also be created for educational or entertainment purposes, allowing users to explore and learn about different types of flowers in a virtual environment. One advantage of virtual flowers is that they can be created and shared easily and quickly, without the need for physical flowers or transportation. This can be particularly useful for events or occasions where flowers may be difficult to obtain or transport, such as in remote or urban areas. Virtual flowers also offer the opportunity for greater customization and personalization than traditional flowers. Users can choose from a wide range of flower types, colors, and arrangements to create a unique and personalized gift or message. Virtual flowers can also be easily modified or updated, allowing for changes in color or arrangement to suit different preferences or occasions. Overall, virtual flowers offer a fun and creative way to enjoy the beauty of flowers in a digital environment. While they cannot replace the physical experience of receiving or giving real flowers, they offer a unique and convenient alternative for those who may not have access to physical flowers or who want to explore the creative possibilities of digital technology.
Advances in landscaping technology have led to the development of new tools and techniques that make it easier and more efficient to design, install, and maintain outdoor spaces. 3D modeling software is widely used in the landscaping industry for designing and planning outdoor spaces. Here are some of the top 3D modeling software used for landscaping:
SketchUp: SketchUp is a user-friendly 3D modeling software that allows designers to create detailed 3D models of landscapes, buildings, and other outdoor spaces. It features a large library of 3D models, allowing designers to add plants, trees, furniture, and other elements to their design.
AutoCAD: AutoCAD is a powerful software that is widely used in the architecture, engineering, and construction industry. It allows designers to create 2D and 3D models of landscapes, buildings, and other outdoor spaces. AutoCAD also features advanced tools for creating accurate measurements, annotations, and other design elements.
Lumion: Lumion is a 3D visualization software that is widely used in the landscaping industry. It allows designers to create stunning 3D renderings of landscapes, buildings, and other outdoor spaces. Lumion features a large library of 3D models, textures, and materials, allowing designers to create realistic and visually appealing designs (Figure 8).
Vectorworks: Vectorworks is a powerful 3D modeling software that is widely used in the landscaping industry. It features advanced tools for creating detailed 2D and 3D models of landscapes, buildings, and other outdoor spaces. Vectorworks also features advanced tools for creating accurate measurements, annotations, and other design elements.
Blender: Blender is a free and open-source 3D modeling software that is widely used in the landscaping industry. It features a large community of users and developers, allowing designers to access a large library of 3D models, textures, and materials. Blender also features advanced tools for creating animations and visual effects, making it an ideal tool for creating animated walkthroughs of outdoor spaces. Overall, 3D modeling software is an essential tool for landscape designers, architects, and other professionals in the landscaping industry. It allows them to create detailed and accurate models of outdoor spaces, reducing the risk of errors and misunderstandings.
Drones equipped with cameras and sensors can be used to create detailed maps of landscapes, Drone mapping is becoming an increasingly popular technology in the floriculture and landscaping industries. It involves using drones equipped with cameras to capture high-resolution aerial images of a landscape, allowing designers to analyze topography, vegetation, and other factors that can affect the design and maintenance of outdoor spaces which can then be used to create detailed maps, 3D models, and other visualizations (Kleinschroth et al., 2022). Here are some of the ways that drone mapping is being used in floriculture and landscaping: Site surveys: Drones can be used to quickly and accurately survey a landscape, allowing designers and landscapers to gather detailed information about the topography, vegetation, and other features of the site. Plant health monitoring: Drones equipped with specialized cameras can be used to monitor the health of plants in a greenhouse or outdoor growing environment (Pantos et al., 2023). This can help growers detect issues such as nutrient deficiencies, water stress, and pest infestations before they become more serious problems (Attada and Katta, 2019). Irrigation management: Drones can be used to monitor irrigation systems and detect issues such as leaks, clogs, and other problems that can waste water and damage plants. Design visualization: Drones can be used to capture high-resolution aerial images of a landscape, which can then be used to create detailed 3D models and other visualizations (Figure 9). This can help designers and clients better understand the layout, features, and potential of a site. Precision application of inputs: Drones equipped with spraying equipment can be used to apply fertilizers, pesticides, and other inputs to crops and landscaping areas with greater precision than traditional ground-based methods (Hassler and Baysal-Gurel, 2019). This can reduce waste, improve efficiency, and reduce the risk of damage to non-target areas. Overall, drone mapping is a powerful tool for floriculture and landscaping professionals, providing a detailed and accurate view of a landscape from above. It can help improve efficiency, reduce waste, and improve the health and productivity of plants and other vegetation.
There is a growing trend towards using sustainable and environmentally-friendly materials in landscaping, such as recycled materials, native plants, pot making, floral arrangement making, and permeable paving (Darras, 2020). These materials can reduce environmental impact and provide long-term cost savings. Overall, advances in landscaping technology are making it easier and more efficient to design, install, and maintain outdoor spaces. These technologies are helping to create more sustainable and enjoyable landscapes that can benefit both people and the environment.
Blockchain technology is a secure and decentralized database system that has been adopted in various industries, including agriculture. In the flower industry, blockchain technology is being explored as a means to improve supply chain management, traceability, and transparency. Blockchain technology can provide a secure and transparent record of every transaction along the flower supply chain, from the grower to the retailer. This can help to increase consumer trust by ensuring that the flowers are grown and distributed sustainably and ethically. By using blockchain technology, consumers can verify the origin, quality, and freshness of flowers, and can be confident that they are purchasing a genuine product. In addition, blockchain technology can help to reduce the risk of fraud, counterfeiting, and mislabeling in the flower industry. It can provide a secure and tamper-proof record of every step in the supply chain, which can help to identify any irregularities or inconsistencies. This can help to reduce waste, increase efficiency, and save costs. Furthermore, blockchain technology can also provide benefits to flower growers by improving payment systems and reducing transaction costs. By using blockchain-based payment systems, growers can receive payment faster, without the need for intermediaries such as banks or payment processors. Overall, blockchain technology has the potential to transform the flower industry by improving transparency, traceability, and efficiency throughout the supply chain. With further adoption and development, blockchain technology could become a key tool in ensuring the sustainability, quality, and authenticity of flowers for consumers around the world.
E-commerce platforms allow for online sales of flowers and plants, making it easier for customers to purchase and receive products. Here are some global e-commerce platforms for flowers:
Interflora: A global flower delivery service that operates in over 140 countries, providing same-day and next-day flower delivery options.
Florist.com: A global online flower delivery service that offers a wide range of floral arrangements and gift options for various occasions.
Flower Chimp: An online florist that operates in several Southeast Asian countries, including Malaysia, Singapore, and the Philippines.
FloraQueen: A global flower delivery service that operates in over 100 countries, providing fresh flower arrangements for various occasions.
Bloom & Wild: An online flower delivery service that operates in several European countries, including the United Kingdom, Germany, and France.
Euroflorist: An online flower delivery service that operates in several European countries, including Sweden, the Netherlands, and Germany.
Avas Flowers: An online flower delivery service that operates in the United States and provides same-day delivery options for many areas.
JustFlowers.com: An online flower delivery service that operates in the United States of and provides same-day delivery options for many areas.
FlowerAdvisor: An online florist that operates in several countries across Asia, including Indonesia, Singapore, and Malaysia.
daFlores: A global flower delivery service that operates in over 180 countries, providing fresh flower arrangements for various occasions.
To address existing challenges and promote sustainable large-scale production of ornamental plants, innovative solutions are being developed (Cardoso and Vendrame, 2022). One such project, FloraGuard (Lavorgna et al., 2020), aims to combat the illegal online market of endangered plants by tracking, providing information, strengthening legislation, and exploring strategies. This initiative seeks to reduce the over-collection and commercialization of threatened or endangered plants that are unlawfully extracted from their natural habitats.
The flower industry has experienced noteworthy benefits due to the ongoing advancement of technology, including enhanced efficacy in production procedures, superior flower quality, and increased profitability. As the floral sector experiences further development and expansion, the imperative for sustainable and ethical methodologies becomes increasingly pressing. The overuse of pesticides and fertilisers in flower cultivation is a significant ecological issue that can result in detrimental consequences for the environment and the wellbeing of labourers engaged in the production process. The implementation of precision agriculture methodologies, which entail the utilisation of sensors and data analytics, can potentially mitigate the excessive application of chemicals by furnishing precise insights regarding soil quality, pest invasions, and crop nourishment necessities. In addition, the adoption of biodegradable packaging materials and the integration of energy-efficient systems within the flower production sector can substantially mitigate the industry’s carbon emissions. The attainment of this objective can be realised by embracing sustainable energy alternatives, such as solar or wind energy, and integrating circular economy tenets, which involve the recycling or repurposing of waste materials. One additional ethical issue prevalent in the flower industry pertains to the potential exploitation of labour in developing nations, which serve as the primary location for flower cultivation. In order to tackle this matter, it is possible to implement fair trade practises and certifications, which can guarantee that labourers are remunerated equitably, provided with secure working environments, and granted access to educational and healthcare resources. Moreover, the utilisation of technology can facilitate the advancement of social and economic growth within regional communities by creating novel employment prospects and fostering entrepreneurial activities. The utilisation of e-commerce platforms has the potential to establish a direct connection between consumers and small-scale flower growers, thereby obviating the requirement for intermediaries and augmenting the profit margins of the growers. To conclude, the flower industry must prioritise sustainability, ethical practices, and social responsibility in the development and implementation of novel technologies. By endorsing these principles, the floral industry can sustain its growth and satisfy the increasing need for aesthetically pleasing and significant flowers, all while making a positive impact on both the environment and society.
Author MAW and AD wrote the intial draft, conceived the idea, prepared figures, ITN, TR, RAL, and ZAB helped in collecting data and literature, JMA-K, SMJ, and MM helped in the language editing and funding acciquisition. All authors contributed to the article and approved the submitted version.
This work was supported by The Deanship of Scientific Research, Vice Presidency for Graduate Studies and Scientific Research, King Faisal University, Saudi Arabia (Project No. GRANT 3507). This work was also supported by Department of Biosystems Engineering, Auburn University. USA.
We acknowledge the support of The Deanship of Scientific Research, Vice Presidency for Graduate Studies and Scientific Research, King Faisal University, Saudi Arabia. We also acknowledge the support of Department of Biosystems Engineering, Auburn University. USA
The authors declare that the research was conducted in the absence of any commercial or financial relationships that could be construed as a potential conflict of interest.
All claims expressed in this article are solely those of the authors and do not necessarily represent those of their affiliated organizations, or those of the publisher, the editors and the reviewers. Any product that may be evaluated in this article, or claim that may be made by its manufacturer, is not guaranteed or endorsed by the publisher.
Abarna, J., and Selvakumar, A. (2015). Rose flower harvesting robot. Int. J. Appl. Eng. Res., 4216–4222.
Adedoja, A., Owolawi, P. A., and Mapayi, T. “Deep learning based on nasnet for plant disease recognition using leave images,” in Proceedings of the International Conference on Advances in Big Data, Computing and Data Communication Systems, Winterton, South Africa, August, 2019. doi:10.1109/ICABCD.2019.8851029
Adegbola, Y. U., Fisher, P. R., and Hodges, A. W. (2019). Economic evaluation of transplant robots for plant cuttings. Sci. Hortic. 246, 237–243. doi:10.1016/j.scienta.2018.10.070
Ahmad, H. M. (2023). “Recent trends in genome editing technologies for agricultural crop improvement,” in Sustainable agriculture in the era of the OMICs revolution. Editors C. S. Prakash, S. Fiaz, M. A. Nadeem, F. S. Baloch, and A. Qayyum (Berlin, Germany: Springer). doi:10.1007/978-3-031-15568-0_17
Ahn, C. H., Ramya, M., An, H. R., Park, P. M., Kim, Y.-J., Lee, S. Y., et al. (2020). Progress and challenges in the improvement of ornamental plants by genome editing. Plants 9, 687. doi:10.3390/plants9060687
Akhter, R., and Sofi, S. A. (2021). Precision agriculture using IoT data analytics and machine learning. J. King Saud. Univ. Comput. Inf. Sci. 34, 5602–5618. doi:10.1016/j.jksuci.2021.05.013
Al Murad, M., Razi, K., Jeong, B. R., Samy, P. M. A., and Muneer, S. (2021). Light emitting diodes (LEDs) as agricultural lighting: Impact and its potential on improving physiology, flowering, and secondary metabolites of crops. Sustainability 13, 1985. doi:10.3390/su13041985
Al-Beeshi, B., Al-Mesbah, B., Al-Dosari, S., and El-Abd, M. “The greenhouse robot,” in Proceedings of the 2015 IEEE 28th Canadian Conference on Electrical and Computer Engineering (CCECE), Halifax, NS, Canada, May 2015, 1489–1494.
Al-Ghobari, H. M., Mohammad, F. S., El Marazky, M. S. A., and Dewidar, A. Z. (2017). Automated irrigation systems for wheat and tomato crops in arid regions. Water sa. 43, 354–364.
Al-Kodmany, K. (2018). The vertical farm: a review of developments and implications for the vertical city. Buildings 8 (2), 24. doi:10.3390/buildings8020024
Alekasir, K., Hassani, R. N., and Azar, A. M. (2017). Effects of silver nanoparticles (SNPs) pulsing treatment and sucrose holding on flower and leaf senescence of cut rose. J. Ornamen Plants. 7, 103–113.
Ali, E. F., Issa, A. A., Al-Yasi, H. M., Hessini, K., and Hassan, F. A. S. (2022). The efficacies of 1-methylcyclopropene and chitosan nanoparticles in preserving the postharvest quality of Damask rose and their underlying biochemical and physiological mechanisms. Biology 11, 242.
Alsanius, B. W., Bergstrand, K.-J., Hartmann, R., Gharaie, S., Wohanka, W., Dorais, M., et al. (2017). Ornamental flowers in new light: Artificial lighting shapes the microbial phyllosphere community structure of greenhouse grown sunflowers (Helianthus annuus L). Sci. Hortic. 216, 234–247. doi:10.1016/j.scienta.2017.01.022
AlShrouf, A. (2017). Hydroponics, aeroponic and aquaponic as compared with conventional farming. Int. Sch. Res. Netw. Agron. (ISRN) 27, 247–255.
Amingad, V., Sreenivas, K. N., Fakrudin, B., Seetharamu, G. K., Shankarappa, T. H., et al. (2017). Comparison of silver nanoparticles and other metal nanoparticles on postharvest attributes and bacterial load in cut roses var. Taj Mahal. Int. J. Pure Appl. Biosci. 5, 579–584. doi:10.18782/2320-7051.2610
Ampatzidis, Y. Z., Vougioukas, S. G., Bochtis, D. D., and Tsatsarelis, C. A. (2009). A yield mapping system for hand-harvested fruits based on RFID and GPS location technologies: Field testing. Precis. Agr. 10, 63–72. doi:10.1007/s11119-008-9095-8
Arad, B., Balendonck, J., Barth, R., Ben-Shahar, O., Edan, Y., Hellström, T., et al. (2020). Development of a sweet pepper harvesting robot. J. Field Robot. 37, 1027–1039. doi:10.1002/rob.21937
Astee, L. Y., and Kishnani, N. T. (2010). Building integrated agriculture: Utilising rooftops for sustainable food crop cultivation in Singapore. J. Green Build. 5, 105–113. doi:10.3992/jgb.5.2.105
Atefi, A., Ge, Y., Pitla, S., and Schnable, J. (2021). Robotic technologies for high-throughput plant phenotyping: Contemporary reviews and future perspectives. Front. Plant Sci. 12, 611940. doi:10.3389/fpls.2021.611940
Attada, V., and Katta, S. (2019). A methodology for automatic detection and classification of pests using optimized SVM in greenhouse crops. Int. J. Eng. Adv. Technol. 8, 1485–1491. doi:10.35940/ijeat.f8133.088619
Azadi, P., Bagheri, H., Nalousi, A. M., Nazari, F., and Chandler, S. F. (2016). Current status and biotechnological advances in genetic engineering of ornamental plants. Biotechnol. Adv. 34, 1073–1090. doi:10.1016/j.biotechadv.2016.06.006
Banda-Chávez, M. J., Pablo Serrano-Rubio, J., Osvaldo Manjarrez-Carrillo, A., Maria Rodriguez-Vidal, L., and Herrera-Guzman, R. “Intelligent wireless sensor network for ornamental plant care,” in Proceedings of the IECON 2018—44th Annual Conference of the IEEE Industrial Electronics Society, Washington, DC, USA, October 2018.
Bechar, A., and Vigneault, C. (2016). Agricultural robots for field operations: Concepts and components. Biosyst. Eng. 149, 94–111. doi:10.1016/j.biosystemseng.2016.06.014
Beeson, R., and Brooks, J. (2006). Evaluation of a model based on reference crop evapotranspiration (ETo) for precision irrigation using overhead sprinklers during nursery production of ligustrum japonica. Proc. V. Int. Symp. Irrig. Hortic. Crops 792, 85–90.
Belal, A. A., El-Ramady, H., Jalhoum, M., Gad, A., and Mohamed, E. S. (2021). “Precision farming technologies to increase soil and crop productivity,” in Agro-environmental sustainability in MENA regions. SW. Editors M. Abu-hashim, F. Khebour Allouche, and A. Negm (Berlin, Germany: Springer), 117–154. doi:10.1007/978-3-030-78574-1_6
Belayneh, B. E., Lea-Cox, J. D., and Lichtenberg, E. (2013). Costs and benefits of implementing sensor-controlled irrigation in a commercial pot-in-pot container nursery. HortTechnology 23, 760–769. doi:10.21273/horttech.23.6.760
Benos, L., Tagarakis, A. C., Dolias, G., Berruto, R., Kateris, D., and Bochtis, D. (2021). Machine learning in agriculture: A comprehensive updated review. Sensors 21, 3758. doi:10.3390/s21113758
Boutigny, A. L., Dohin, N., Pornin, D., and Rolland, M. (2020). Overview and detectability of the genetic modifications in ornamental plants. Hortic. Res. 7, 11. doi:10.1038/s41438-019-0232-5
Bowlekar, A. P., Patil, S. T., Kadam, U. S., Mane, M. S., Nandgude, S. B., and Palte, N. K. (2019). Performance evaluation of real time automatic irrigation system on the yield of cabbage (Brassica oleracea L). Int. J. Pure Appl. Biosci. 7, 160–165. doi:10.18782/2320-7051.7433
Cioc, M., Dziurka, M., and Pawłowska, B. (2022). Changes in endogenous phytohormones of gerbera jamesonii axillary shoots multiplied under different light emitting diodes light quality. Molecules 27, 1804. doi:10.3390/molecules27061804
Cai, J., Veerappan, V., Arildsen, K., Sullivan, C., Piechowicz, M., Frugoli, J., et al. (2023). A modified aeroponic system for growing small-seeded legumes and other plants to study root systems. Plant Methods 19, 21. doi:10.1186/s13007-023-01000-6
Cardoso, J. C., and Vendrame, W. A. (2022). Innovation in propagation and cultivation of ornamental plants. Horticulturae 8, 229. doi:10.3390/horticulturae8030229
Chaerle, L., Hagenbeek, D., De Bruyne, E., and Van Der Straeten, D. (2007). Chlorophyll fluorescence imaging for disease-resistance screening of sugar beet. Plant Cell Tissue Organ Cult. 91. doi:10.1007/s11240-007-9282-8
Chandler, S. F., and Tribe, D. (2022). “Modern techniques for plant breeding in ornamentals,” in Floriculture and ornamental plants. Handbooks of crop diversity: Conservation and use of plant genetic resources. Editors S. K. Datta, and Y. C. Gupta (Singapore: Springer). doi:10.1007/978-981-15-3518-5_19
Chang, C. L., Song, G. B., and Lin, K. M. (2016). Two-Stage guidance control scheme for high-precision straight-line navigation of a four-wheeled planting robot in a greenhouse. Trans. ASABE 59, 1193–1204.
Chappell, M., Dove, S. K., van Iersel, M. W., Thomas, P. A., and Ruter, J. (2013). Implementation of wireless sensor networks for irrigation control in three container nurseries. HortTechnology 23, 747–753. doi:10.21273/horttech.23.6.747
Christie, C. B., and Nichols, M. A. (2004). Aeroponics-A production system and research tool. Acta Hortic. 648, 185–190. doi:10.17660/actahortic.2004.648.22
Coates, R. W., Delwiche, M. J., Broad, A., Holler, M., Evans, R., Oki, L., et al. (2012). Wireless sensor network for precision irrigation control in horticultural crops. St. Joseph, MI, USA: American Society of Agricultural and Biological Engineers.
Craig, D. S., and Runkle, E. S. (2013). A moderate to high red to far-red light ratio from light-emitting diodes controls flowering of short-day plants. J. Am. Soc. Hortic. Sci. 138, 167–172. doi:10.21273/jashs.138.3.167
Craig, D. S., and Runkle, E. S. (2016). An intermediate phytochrome photoequilibria from night-interruption lighting optimally promotes flowering of several long-day plants. Environ. Exp. Bot. 121, 132–138. doi:10.1016/j.envexpbot.2015.04.004
Crowell, S., Falcão, A. X., Shah, A., Wilson, Z., Greenberg, A. J., and McCouch, S. R. (2014). High-resolution inflorescence phenotyping using a novel image-analysis pipeline, PANorama. Plant Physiol. 165, 479–495. doi:10.1104/pp.114.238626
de Kreij, C., and van der Hoeven, B. (1997). Effect of humic substances, pH and its control on growth of chrysanthemum in aeroponics. Proc. 9th Int. Congr. Soil. Cult., 207–230.
Dafni, A., Lev, E., Beckmann, S., and Eichberger, C. (2006). Ritual plants of Muslim graveyards in northern Israel. J. Ethnobiol. Ethnomed 2, 38. doi:10.1186/1746-4269-2-38
Darqui, F. S., Radonic, L. M., Hopp&Bilbao, H. E. M. L., and Lopez Bilbao, M. (2017). Biotechnologicalimprovement of ornamental plants. Ornam. Hortic. 23, 279–288. doi:10.14295/oh.v23i3.1105
Darras, A. (2020). Implementation of sustainable practices to ornamental plant cultivation worldwide: A critical review. Agronomy 10, 1570. doi:10.3390/agronomy10101570
De Cáceres González, F. F. N., Davey, M. R., Sanchez, E. C., and Wilson, Z. A. (2015). Conferred resistance to Botrytis cinerea in Lilium by overexpression of the RCH10 chitinase gene. Plant Cell Rep. 34, 1201–1209. doi:10.1007/s00299-015-1778-9
Deng, F., Zuo, P., Wen, K., and Wu, X. (2020). Novel soil environment monitoring system based on RFID sensor and LoRa. Computers and Electronics in Agriculture 169, 105169. doi:10.1016/j.compag.2019.105169
Despommier, D. (2014). Encyclopedia of food and agricultural ethics (vertical farms in horticulture). Dordrecht, Netherlands: Springer.
Despommier, D. (2010). The vertical farm: Feeding the world in the 21st century. New York, NY, USA: Thomas Dunne Books.
Din, A., Qadri, Z. A., Wani, M. A., Iqbal, S., Malik, S. A., Bhat, Z. A., et al. (2022). Developing an efficient in vitro callusing and regeneration protocol in Dendranthema × grandiflorum Kitam. J. Crop Sci. Biotechnol. 25, 393–405. doi:10.1007/s12892-022-00140-w
Din, A., Qadri, Z. A., Wani, M. A., Rather, Z. A., Iqbal, S., Malik, S. A., et al. (2021). Congenial in vitro γ-ray-Induced mutagenesis underlying the diverse array of petal colours in Chrysanthemum (Dendranthema grandiflorum kitam) cv“Candid”. Biol. Life Sci. Forum 4, 21. doi:10.3390/IECPS2020-08780
Dose, V., Wallenhorst, A., Tijan, E., and Jovic, M. “Implementation of RFID technology in perishable goods transport,” in Proceedings of the 2021 44th International Convention on Information, Communication and Electronic Technology (MIPRO), Opatija, Croatia, September 2021. doi:10.23919/mipro52101.2021.9597038
Dykes, L., Hoffmann, L., Portillo-Rodriguez, O., Rooney, W. L., and Rooney, L. W. (2014). Prediction of total phenols, condensed tannins, and 3-deoxyanthocyanidins in sorghum grain using near-infrared (NIR) spectroscopy. J. Cereal Sci. 60, 138–142.
Ecarnot, M., Bączyk, P., Tessarotto, L., and Chervin, C. (2013). Rapid phenotyping of the tomato fruit model, Micro-Tom, with a portable VIS–NIR spectrometer. Plant Physiol. Biochem. 70, 159–163. doi:10.1016/j.plaphy.2013.05.019
El-Sayed, I. M., and El-Ziat, R. A. (2021). Utilization of environmentally friendly essential oils on enhancing the postharvest characteristics ofChrysanthemum morifolium Ramat cut flowers. Heliyon 7 (1), e05909. doi:10.1016/j.heliyon.2021.e05909
Eli-Chukwu, N. C. (2019). Applications of artificial intelligence in agriculture: A review. Eng. Technol. Appl. Sci. Res. 9, 4377–4383. doi:10.48084/etasr.2756
Elijah, O., Rahman, T. A., Orikumhi, I., Leow, C. Y., and Hindia, M. N. (2018). An overview of internet of things (IoT) and data analytics in agriculture: Benefits and challenges. IEEE Internet Things J. 5, 3758–3773. doi:10.1109/jiot.2018.2844296
Elmqvist, T., Setälä, H., Handel, S., van der Ploeg, S., Aronson, J., Blignaut, J., et al. (2015). Benefits of restoring ecosystem services in urban areas. Curr. Opin. Environ. Sustain. 14, 101–108. doi:10.1016/j.cosust.2015.05.001
Eve, L. (2015). PlantLab could grow fruit and vegetables for the entire world in a space smaller than holland. Available online:http://inhabitat.com/dutch-company-plantlabs-agricultural-revolution-could-grow-the-worlds-fruit-and-veg-in-a-space-smaller-than-holland/ (accessed July 15, 2017).
Faroq, A.-T., Adam, H., Dos Anjos, A., Lorieux, M., Larmande, P., Ghesquière, A., et al. (2013). P-TRAP: A panicle trait phenotyping tool. BMC Plant Biol. 13, 122. doi:10.1186/1471-2229-13-122
Fascella, G., and Zizzo, G. (2007). Preliminary results of aeroponic cultivation of anthurium andreanum for cut flower production. Acta Hortic. 747, 233–240. doi:10.17660/actahortic.2007.747.27
Fernandez, R. T. (2014). Rfid: How it works and what it can do for the green industry. Am. Newsl. 9, 6–11.
Floyd, R. E. (2015). “RFID in Animal-Tracking Applications,” in IEEE Potentials 34 (5), 32–33. doi:10.1109/MPOT.2015.2410308
Ferroukhi, M., Saadi, H., Bendib, R., Berracheddi, L., and Cherifi, A. (2023). “Connected sensors for a smart green farm,” in Advanced computational techniques for renewable energy systems. IC-AIRES 2022. Editor M. Hatti (Berlin, Germnay: Springer). doi:10.1007/978-3-031-21216-1_23
Fussy, A., and Papenbrock, J. (2022). An overview of soil and soilless cultivation techniques—chances, challenges and the neglected question of sustainability. Plants 11, 1153. doi:10.3390/plants11091153
Gage, J. L., Miller, N. D., Spalding, E. P., Kaeppler, S. M., and de Leon, N. (2017). Tips: A system for automated image-based phenotyping of maize tassels. Plant Methods 13, 21. doi:10.1186/s13007-017-0172-8
Gao, C., Qi, L., Wu, Y., Feng, J., and Yang, Z. “Design and testing of a self-propelled air-blowing greenhouse sprayer,” in Proceedings of the 2017 ASABE Annual International Meeting, Spokane, WA, USA, July 2017.
Griffin, T. W., Shockley, J. M., and Mark, T. B. (2018). “Economics of precision farming,” in Precision agriculture basics. Editors D. K. Shannon, D. E. Clay, and N. R. Kitchen (Madison, WI, USA: American Society of Agronomy), 221–230.
Grift, T., Zhang, Q., Kondo, N., and Ting, K. C. (2008). A review of automation and robotics for the bio-industry. J. Biomechatron. Eng. 1, 37–54.
Grimstad, L., and From, P. (2017). The thorvald II agricultural robotic system. Robotics 6, 24. doi:10.3390/robotics6040024
Guo, Z., Huang, W., Chen, L., Wang, X., and Peng, Y. (2013). “Nondestructive evaluation of soluble solid content in strawberry by near infrared spectroscopy,” in Piageng 2013: Image Processing and Photonics for Agricultural Engineering. (Bellingham: International Society for Optics and Photonics), 87610O.
Haaland, C., and van den Bosch, C. K. (2015). Challenges and strategies for urban green-space planning in cities undergoing densification: A review. Urban For. Urban Green. 14, 760–771. doi:10.1016/j.ufug.2015.07.009
Han, L., Mao, H., Kumi, F., and Hu, J. (2018). Development of a multi-Task robotic transplanting workcell for greenhouse seedlings. Applied Engineering in Agriculture 34 (2), 335–342. doi:10.13031/aea.12462
Hardie, M. (2020). Review of novel and emerging proximal soil moisture sensors for use in agriculture. Sensors 20, 6934. doi:10.3390/s20236934
Hassler, S. C., and Baysal-Gurel, F. (2019). Unmanned aircraft system (UAS) technology and applications in agriculture. Agronomy 9, 618. doi:10.3390/agronomy9100618
Hayden, A. L. (2006). Aeroponic and hydroponic systems for medicinal herb, rhizome, and root crops. HortScience 41 (3), 536–538. doi:10.21273/hortsci.41.3.536
Heo, J. W., Lee, C. W., Murthy, H. N., and Paek, K. Y. (2003). Influence of light quality and photoperiod on flowering ofCyclamen persicum Mill. cv. ‘Dixie White. Plant Growth Regul. 40, 7–10. doi:10.1023/a:1023096909497
Higuchi, Y., Sumitomo, K., Oda, A., Shimizu, H., and Hisamatsu, T. (2012). Day light quality affects the night-break response in the short-day plant chrysanthemum, suggesting differential phytochrome-mediated regulation of flowering. J. Plant Physiol. 169, 1789–1796. doi:10.1016/j.jplph.2012.07.003
Hossen, M. A., Talukder, M. R. A., Al Mamun, M. R., Rahaman, H., Paul, S., Rahman, M. M., et al. (2020). Mechanization status, promotional activities and government strategies of Thailand and Vietnam in comparison to Bangladesh. AgriEngineering 2, 489–510. doi:10.3390/agriengineering2040033
Hussain, A., Shah, F., Ali, F., and Yun, B. W. (2022). Role of nitric oxide in plant senescence. Front. Plant Sci. 13, 851631. doi:10.3389/fpls.2022.851631
Hussain, A., Iqbal, K., Aziem, S., Mahato, P., and Negi, A. K. (2014). A review on the science of growing crops without soil (soilless culture)—a novel alternative for growing crops. Int. J. Agric. Crop. Sci. 7, 833–842.
Huylenbroeck, J. V., and Bhattarai, K. (2022). Ornamental plant breeding: Entering a new era? Ornam. Hortic. 28 (3), 297–305. doi:10.1590/2447-536X.v28i3.2516
Ichimura, K., and Shimizu-Yumoto, H. (2007). Extension of the vase life of cut roses by treatment with sucrose before and during simulated transport. Bull. Natl. Inst. Flor. Sci. 7, 17–27.
Jha, K., Doshi, A., Patel, P., and Shah, M. (2019). A comprehensive review on automation in agri-culture using artificial intelligence. Artif. Intell. Agric. 2, 1–12. doi:10.1016/j.aiia.2019.05.004
Jin, C., Dong, L., Wei, C., Wani, M. A., Yang, C., Li, S., et al. (2023). Creating novel ornamentals via new strategies in the era of genome editing. Front. Plant Sci. 14, 1142866. doi:10.3389/fpls.2023.1142866
Jones, A., Ali, U., and Egerstedt, M. “Optimal pesticide scheduling in precision agriculture,” in Proceedings of the ACM/IEEE 7th International Conference on Cyber-Physical Systems (ICCPS), Vienna, Austria, April 2016, 1–8.
Jones, P., Clarke-Hill, C., Comfort, D., Hillier, D., and Shears, P. (2005). Radio frequency identification and food retailing in the UK. Brit. Food J. 107, 356–360. doi:10.1108/00070700510602156
Kahraman, Ö., and Akçal, A. (2018). Yhe effects of diffirent nutrient solutions on summer snowflake’s development in soilless culture. COMU J. Agric. Fac. 6, 169–176.
Kang, D., Jeong, H. K., Park, Y. G., and Jeong, B. R. (2019). Flowering and morphogenesis of kalanchoe in response to quality and intensity of night interruption light. Plants 8, 90. doi:10.3390/plants8040090
Kawollek, M., and Rath, T. (2008). Robotic harvest of cut flowers based on image processing by usingGerbera jamesonii as model plant. Acta Hortic. 801, 557–564. doi:10.17660/ActaHortic.2008.801.62
Kazaz, S., Doğan, E., Kılıç, T., Şahin, E. G. E., and Seyhan, S. (2019). Influence of holding solutions on vase life of cut hydrangea flowers (Hydrangea macrophylla Thunb). Fresenius Environ. Bull. 28, 3554–3559.
Khalaj, M. A., and Noroozisharaf, A. (2020). Efficiency of ammonium and nitrate ratios on macronutrient content and morphological properties of Gerbera jamesonii cut flower. Agric. Conspec. Sci. 85 (3), 281–289.
Khan, N., Ray, R. L., Zhang, S., Osabuohien, E., and Ihtisham, M. (2022). Influence of mobile phone and internet technology on income of rural farmers: Evidence from Khyber Pakhtunkhwa Province, Pakistan. Pak Techno Soc. 68, 101866. doi:10.1016/j.techsoc.2022.101866
Kharrazi, M., Sharifi, A., Nejatizadeh, S., Khadem, A., and Moradian, M. (2020). Selection of optimal cultivation media for gerbera (Gerbera Jamesonii) growth in the hydroponic culture system. J. Hortic. Sci. 34 (2), 261–271. doi:10.22067/jhorts4.v34i2.79193
Killebrew, K., and Wolff, H. (2010). Environmental impacts of agricultural technologies. Evans Sch. Policy Anal. Res. Group 65, 1–18.
Kim, J., Chappell, M., Van Iersel, M. W., and Lea-Cox, J. D. (2014). Wireless sensors networks for optimization of irrigation, production, and profit in ornamental production. Acta Hortic. 1037, 643–649. doi:10.17660/actahortic.2014.1037.82
Kleinschroth, F., Banda, K., Zimba, H., Dondeyne, S., Nyambe, I., Spratley, S., et al. (2022). Drone imagery to create a common understanding of landscapes. Landsc. Urban Plan. 228, 104571. doi:10.1016/j.landurbplan.2022.104571
Koukounaras, A. (2021). Advanced greenhouse horticulture: New technologies and cultivation practices. Horticulturae 7, 1. doi:10.3390/horticulturae7010001
Kromwijk, J. A. M., and Vanos, E. A. (2020). “Advances in soilless culture of ornamentals,” in Advances in horticultural soilless culture. Editor N. S. GRUDA (Cambridge, England: Burleigh Dodds Science Publishing Limited), 442p.
Kui, L., Chen, H., Zhang, W., He, S., Xiong, Z., Zhang, Y., et al. (2017). Building a genetic manipulation tool box for orchid biology: Identification of constitutive promoters and application of CRISPR/Cas9 in the orchid, dendrobium officinale. Front. Plant Sci. 7, 2036. doi:10.3389/fpls.2016.02036
Kumar, L. S., Salim, F., Aani, A., Kahtan, H., Darr, M. J., and Al-bashiri, H. (2019b). Data visualisation for smart farming using mobile application. Int. J. Comput. Sci. Netw. Secur. 19, 1–7.
Kumar, L. S., Salim, F., Aani, A., Kahtan, H., Darr, M. J., and Al-bashiri, H. (2019a). Data visualisation for smart farming using mobile application. Int. J. Comput. Sci. Netw. Secur., 1–7.
Kumar, V., and Srivastava, A. (2018). The role of RFID in agro-food sector. Open Access J. 14. doi:10.19080/artoaj.2018.14.555924
Lassoued, R., Macall, D. M., Smyth, S. J., Phillips, P. W. B., and Hesseln, H. (2021). Expert insights on the impacts of, and potential for, agricultural big data. Sustainability 13, 2521. doi:10.3390/su13052521
Lavorgna, A., Middleton, S. E., Pickering, B., and Neumann, G. (2020). FloraGuard: Tackling the online illegal trade in endangered PlantsThrough a cross-disciplinary ICT-enabled methodology. J. Contemp. Crim. Justice 36, 428–450. doi:10.1177/1043986220910297
Lea-Cox, J. D., and Belayneh, B. E. (2013). Implementation of sensor-controlled decision irrigation scheduling in pot-in-pot nursery production. Acta Hortic. 1034, 93–100.
Lea-Cox, J. D., Ristvey, A. G., and Kantor, G. F. (2008). Using wireless sensor technology to schedule irrigations and minimize water use in nursery and greenhouse production systems. Comb. Proc. Int. Plant Propagators Soc. 58, 512–518.
Leiva, J. A., Wilson, P. C., Albano, J. P., Nkedikizza, P., and O’connor, G. A. (2019). Pesticide sorption to soilless media components used for ornamental plant production and aluminum water treatment residuals. ACS omega 4 (18), 17782–17790. doi:10.1021/acsomega.9b02296
Levenston, M. (2011). Philips lighting promotes city Farming.City farmer news. Available online:http://www.cityfarmer.info/2011/12/10/ (accessed on July 15, 2017).
Li, H., Huang, X., Li, J., Liu, J., Joyce, D., and He, S. (2012). Efficacy of nano-silver in alleviating bacteria-related blockage in cut rose cv. Movie Star stems. Postharvest Biol. Technol. 74, 36–41. doi:10.1016/j.postharvbio.2012.06.013
Li, L., Zhang, Q., and Huang, D. (2014). A review of imaging techniques for plant phenotyping. Sensors 14, 20078–20111. doi:10.3390/s141120078
Li, X., Yin, X., and Wang, Y. (2016). Diversity and ecology of vascular plants established on the extant world-longest ancient city wall of nanjing, China. Urban For. Urban Green 18, 41–52. doi:10.1016/j.ufug.2016.05.007
Liakos, K., Busato, P., Moshou, D., Pearson, S., and Bochtis, D. (2018). Machine learning in agriculture: A review. Sensors 18, 2674. doi:10.3390/s18082674
Longchamps, L., Tisseyre, B., Taylor, J., Sagoo, L., Momin, A., Fountas, S., et al. (2022). Yield sensing technologies for perennial and annual horticultural crops: A review. Precis. Agric. 23, 2407–2448. doi:10.1007/s11119-022-09906-2
Lü, P., Cao, J., He, S., Liu, J., Li, H., Cheng, G., et al. (2010a). Nano-silver pulse treatments improve water relations of cut rose cv. Movie Star flowers. Postharvest Biol. Technol. 57, 196–202. doi:10.1016/j.postharvbio.2010.04.003
Lü, P., He, S., Li, H., Cao, J., and Xu, H. (2010b). Effects of nano-silver treatment on vase life of cut rose cv. Movie Star flowers. J. Sci. Food Agric. Environ. 8, 1118–1122.
Luvisi, A., Panattoni, A., Bandinelli, R., Rinaldelli, E., Pagano, M., Gini, B., et al. (2010). Radiofrequency identification tagging in ornamental shrubs: An application in rose. HortTechnology hortte 20 (6), 1037–1042. doi:10.21273/hortsci.20.6.1037
Mahmud, M. S., Zahid, A., and Das, A. K. (2023). Sensing and automation technologies for ornamental nursery crop production: current status and future prospects. Sensors 23, 1818. doi:10.3390/s23041818
Mancini, M., Mazzoni, L., Gagliardi, F., Balducci, F., Duca, D., Toscano, G., et al. (2020). Application of the non-destructive NIR technique for the evaluation of strawberry fruits quality parameters. Foods 9, 441. doi:10.3390/foods9040441
Manuel Banda-Chávez, J., Pablo Serrano-Rubio, J., Osvaldo Manjarrez-Carrillo, A., Maria Rodriguez-Vidal, L., and Herrera-Guzman, R. “Intelligent wireless sensor network for ornamental plant care,” in Proceedings of the IECON 2018—44th Annual Conference of the IEEE Industrial Electronics Society, Washington, DC, USA, October 2018.
Maraveas, C. (2023). Incorporating artificial intelligence technology in smart greenhouses: Current state of the art. Appl. Sci. 13, 14. doi:10.3390/app13010014
Masuzawa, H., Miura, J., and Oishi, S. “Development of a mobile robot for harvest support in greenhouse horticulture—person following and mapping,” in Proceedings of the 2017 IEEE/SICE International Symposium on System Integration (SII), Taipei, Taiwan, December 2017, 541–546.
Matysiak, B. (2021). The effect of supplementary LED lighting on the morphological and physiological taits of miniature rosa _ hybrida ‘aga’ and the development of powdery mildew (Podosphaera pannosa) under greenhouse conditions. Plants 10, 417. doi:10.3390/plants10020417
Mayrand, F., and Clergeau, P. (2018). Green roofs and green walls for biodiversity conservation: A contribution to urban connectivity? Sustainability 10, 985. doi:10.3390/su10040985
Mazrou, R. M., Hassan, S., Yang, M., and Hassan, F. A. S. (2022). Melatonin preserves the postharvest quality of cut roses through enhancing the antioxidant system. Plants 11, 2713. doi:10.3390/plants11202713
Meinhold, B. (2013). Aeroponic vertical farm: High-yield terraced rice paddies for the Philippines. El Segundo, California, United States: Inhabitat.
Mekala, M. S., and Viswanathan, P. A. “Survey: Smart agriculture IoT with cloud computing,” in Proceedings of the 2017 International Conference on Microelectronic Devices, Circuits and Systems (ICMDCS), Vellore, India, August 2017, 1–7.
Messelink, G. J., Lambion, J., Janssen, A., and van Rijn, P. C. J. (2021). Biodiversity in and around greenhouses: Benefits and potential risks for pest management. Insects 12 (10), 933. doi:10.3390/insects12100933
Mills-Ibibofori, T., Dunn, B. L., Maness, N., and Payton, M. (2019). Effect of LED lighting and gibberellic acid supplementation on potted ornamentals. Horticulturae 5 (3), 51. doi:10.3390/horticulturae5030051
Mittal, A., and Singh, A. (2007). “Microcontroller based pest management system,” in Proceedings of the Second International Conference on Systems (ICONS’07), Martinique, France, April 2007, 43.
Mukherji, N., and Morales, A. (2010). Zoning for urban agriculture. Zoning practice 3. Chicago, IL, USA: American Planning Association.
Nakarmi, A., and Tang, L. (2012). Automatic inter-plant spacing sensing at early growth stages using a 3d vision sensor. Comput. Electr. Agric. 82, 23–31. doi:10.1016/j.compag.2011.12.011
Nassar, P. P. M., and Ribeiro, M. G. (2020). Considerations for cholinesterase biomonitoring in flower and ornamental plant greenhouse workers. Sci. Total Environ. 711, 135228. doi:10.1016/j.scitotenv.2019.135228
Nir, I. (1982). Growing plants in aeroponics growth system. Acta Hortic. 126, 435–448. doi:10.17660/ActaHortic.1982.126.49
Nishihara, M., Nakatsuka, T., Hosokawa, K., Yokoi, T., Abe, Y., Mishiba, K. I., et al. (2006). Dominant inheritance of white-flowered and herbicide-resistant traits in transgenic gentian plants. Plant Biotechnol. 23 (1), 25–31. doi:10.5511/plantbiotechnology.23.25
Nishizawa, T., Mori, Y., Fukushima, S., Natsuga, M., and Maruyama, Y. (2009). Non-destructive analysis of soluble sugar components in strawberry fruits using near-infrared spectroscopy. Nippon Shokuhin Kagaku Kogaku KaishiJ. Japanese Soc. Food Sci. Technol. 56, 229–235.
Nitarska, D., Boehm, R., Debener, T., Lucaciu, R. C., and Halbwirth, H. (2021). First genome edited poinsettias: Targeted mutagenesis of flavonoid 3′-hydroxylase using CRISPR/Cas9 results in a colour shift. Plant Cell Tissue Organ Cult. (PCTOC) 147, 49–60. doi:10.1007/s11240-021-02103-5
Noman, A., Aqeel, M., Deng, J., Khalid, N., Sanaullah, T., and Shuilin, H. (2017). Biotechnological Advancements for Improving Floral Attributes in Ornamental Plants. Front. Plant Sci. 8, 530. doi:10.3389/fpls.2017.00530
Ode Sang, A., Thorpert, P., and Fransson, A-M. (2022). Planning, designing, and managing green roofs and green walls for public health – an ecosystem services approach. Front. Ecol. Evol. 10, 804500. doi:10.3389/fevo.2022.804500
Ohi, N., Lassak, K., Watson, R., Strader, J., Du, Y., Yang, C., et al. (2018). “Design of an autonomous precision pollination robot,” in Proceedings of the 2018 IEEE/RSJ International Conference on Intelligent Robots and Systems (IROS), Madrid, Spain, October 2018, 7711–7718.
Oliveira, L. F. P., Moreira, A. P., and Silva, M. F. (2021). Advances in agriculture robotics: A state-of-the-art review and challenges ahead. Robotics 10, 52. doi:10.3390/robotics10020052
Panozzo, J. F., Eagles, H. A., Cawood, R. J., and Wootton, M. (1999). Wheat spike temperatures in relation to varying environmental conditions. Aust. J. Agric. Res. 50, 997–1006. doi:10.1071/ar98142
Pantos, C., Hildmann, H., and Valente, J. (2023). Experimental connectivity analysis for drones in greenhouses. Drones 7, 24. doi:10.3390/drones7010024
Paradiso, R., and Proietti, S. (2022). Light-quality manipulation to control plant growth and photomorphogenesis in greenhouse horticulture: The state of the art and the opportunities of modern LED systems. J. Plant Growth Regul. 41, 742–780. doi:10.1007/s00344-021-10337-y
Park, Y., and Runkle, E. S. (2016). Investigating the merit of including far-red radiation in the production of ornamental seedlings grown under sole-source lighting. Acta Hortic 1134, 259–266. doi:10.17660/ActaHortic.2016.1134.35
Park, I. S., Cho, K. J., Kim, J., Cho, J. Y., Lim, T. J., and Oh, W. (2016). Growth and flowering responses of petunia to various artificial light sources with different light qualities. Korean J. Hortic. Sci. Technol. 34, 55–66.
Park, Y. G., Muneer, S., Soundararajan, P., Manivnnan, A., and Jeong, B. R. (2017). Light quality during night interruption affects morphogenesis and flowering in geranium. Hortic. Environ. Biotechnol. 58, 212–217. doi:10.1007/s13580-017-0246-6
Patil, K. A., and Kale, N. R. (2016). “A model for smart agriculture using IoT,” in Proceedings of the 2016 International Conference on Global Trends in Signal Processing, Information Computing and Communication, Jalgaon, India, December 2016, 543–545.
Penchaiya, P., Bobelyn, E., Verlinden, B. E., Nicolaï, B. M., and Saeys, W. (2009). Non-destructive measurement of firmness and soluble solids content in bell pepper using NIR spectroscopy. J. Food Eng. 94, 267–273.
Pereira, P. C. G., Parente, C. E., Carvalho, G. O., Torres, J. P., Meire, R. O., Dorneles, P. R., et al. (2021). A review on pesticides in flower production: A push to reduce human exposure and environmental contamination. Environ. Pollut. 289, 117817. doi:10.1016/j.envpol.2021.117817
Pétremand, G., Chittaro, Y., Braaker, S., Brenneisen, S., Gerner, M., Obrist, M. K., et al. (2017). Ground beetle (Coleoptera: Carabidae) communities on green roofs in Switzerland: Synthesis and perspectives. Urban Ecosyst. 21, 119–132. doi:10.1007/s11252-017-0697-7
Pourhosseini, L., Kermani, M. J., Habashi, A. A., and Khalighi, A. (2013). Efficiency of direct and indirect shoot organogenesis in different genotypes of Rosa hybrida. Plant Cell Tissue Organ Cult. 112, 101–108. doi:10.1007/s11240-012-0210-1
Pramanik, M., Khanna, M., Singh, M., Singh, D., Sudhishri, S., Bhatia, A., et al. (2022). Automation of soil moisture sensor-based basin irrigation system. Smart Agric. Technol. 2, 100032. doi:10.1016/j.atech.2021.100032
Prunet, N., and Duncan, K. (2020). Imaging flowers: A guide to current microscopy and tomography techniques to study flower development. J. Exp. Bot. 71, 2898–2909. doi:10.1093/jxb/eraa094
Qu, Y., Jiang, L., Wuyun, T., Mu, S., Xie, F., Chen, Y., et al. (2020). Effects of exogenous putrescine on delaying senescence of cut foliage of Nephrolepis cordifolia. Front. Plant Sci. 11, 566824. doi:10.3389/fpls.2020.566824
Rabiza-Świder, J., Skutnik, E., Jędrzejuk, A., and Łukaszewska, A. (2020). Postharvest treatments improve quality of cut peony flowers. Agronomy 10, 1583. doi:10.3390/agronomy10101583
Rai, R., Nalini, P., and Singh, Y. P. (2022). “Nanotechnology for sustainable horticulture development: Opportunities and challenges,” in Innovative approaches for sustainable development. Editors S. S. Mahdi, and R. Singh (Berlin, Germany: Springer). doi:10.1007/978-3-030-90549-1_12
Rana, R. A., Siddiqui, M. N., Skalicky, M., Brestic, M., Hossain, A., and Kayesh, E. (2021). Prospects of nanotechnology in improving the productivity and quality of horticultural crops. Horticulturae 7, 332. doi:10.3390/horticulturae7100332
Rashidi, A., and TehranifarA Samiei, L. (2023). Modifying spectral distributions during the seedling stage influences the flowering and branching of Petunia × hybrida. Sci. Hortic. 309, 111664. doi:10.1016/j.scienta.2022.111664
Rihn, A. L., Velandia, M., Warner, L. A., Fulcher, A., Schexnayder, S., and LeBude, A. (2022). Factors correlated with the propensity to use automation and mechanization by the US nursery industry. Agribusiness 39, 110–130.
Rudolph, R., Herzog, K., Töpfer, R., and Steinhage, V. (2019). Efficient identification, localization and quantification of grapevine inflorescences and flowers in unprepared field images using Fully Convolutional Networks. Vitis 58, 95–104.
Ruiz-Garcia, L., and Lunadei, L. (2011). The role of RFID in agriculture: Applications, limitations and challenges. Comput. Electron. Agric. 79, 42–50. doi:10.1016/j.compag.2011.08.010
Saleem, M. H., Khanchi, S., Potgieter, J., and Arif, K. M. (2020). Image-based plant disease identification by deep learning meta-architectures. Plants 9, 1451. doi:10.3390/plants9111451
Sanches, G. M., and Otto, R. (2022). A novel approach for determining nitrogen requirement based on a new agronomic principle—Sugarcane as a crop model. Plant Soil 472, 29–43. doi:10.1007/s11104-021-05263-7
Sarmast, M. K. (2019). Transient expression-based CRISPR/Cas9 system for manipulation of tall fescue SGR gene. J. Plant Prod. Res. 56, 35–43.
Sasaki, Y., Okamoto, T., Imou, K., and Torii, T. (1999). Automatic diagnosis of plant disease: Recognition between healthy and diseased leaf. J. Jpn. Soc. Agric. Mach. Jpn. 61, 119–126.
Savvas, D. (2002). “Nutrient solution recycling,” in Hydroponic production of vegetables and ornamentals (Athens, Greece: Embryo Publications), 299–343.
Sen, S., Kumar, S., Ghani, M., and Thakur, M. (2013). Agrobacterium mediated genetic transformation of chrysanthemum (Dendranthema grandiflora Tzvelev) with rice chitinase gene for improved resistance against Septoria obesa. Plant Pathol. J. 12, 1–10. doi:10.3923/ppj.2013.1.10
Shao, Y., and He, Y. (2008). Nondestructive measurement of acidity of strawberry using Vis/NIR spectroscopy. Int. J. Food Prop 11, 102–111.
Shi, X., An, X., Zhao, Q., Liu, H., Xia, L., Sun, X., et al. (2019). State-of- the- art internet of things in protected agriculture. Sensors 19, 1833. doi:10.3390/s19081833
Sirohi, U., Kumar, M., Sharma, V. R., Teotia, S., Singh, D., Chaudhary, V., et al. (2022). CRISPR/Cas9 system: A potential tool for genetic improvement in floricultural crops. Mol. Biotechnol. 64, 1303–1318. doi:10.1007/s12033-022-00523-y
Sisinni, E., Saifullah, A., Han, S., Jennehag, U., and Gidlund, M. (2018). Industrial internet of things: Challenges, opportunities, and directions. IEEE Trans. Ind. Inf. 14, 4724–4734. doi:10.1109/tii.2018.2852491
Solgi, M., Kafi, M., Taghavi, T. S., and Naderi, R. (2009). Essential oils and silver nanoparticles (SNP) as novel agents to extend vase-life of gerbera (Gerbera jamesonii cv. “Dune”) flowers. Post. Biol. Technol. 53, 155–158. doi:10.1016/j.postharvbio.2009.04.003
Song, H., and He, Y. “Crop nutrition diagnosis expert system based on artificial neural networks,” in Proceedings of the Third International Conference on Information Technology and Applications (ICITA’05), Sydney, NSW, Australia, July, 2005. doi:10.1109/ICITA.2005.108
Sori, H., Inoue, H., Hatta, H., and Ando, Y. (2018). Effect for a paddy weeding robot in wet rice culture. J. Robot. Mechatron. 30, 198–205. doi:10.20965/jrm.2018.p0198
Stanghellini, C. “Horticultural production in greenhouses: Efficient use ofwater,” in Proceedings of the International Symposium on Growing Media andSoilless Cultivation, Leuven, Belgium, June 2013, 1034.
Sun, A. Y., and Scanlon, B. R. (2019). How can big data and machine learning benefit environment and water management: A survey of methods, applications, and future directions. Environ. Res. Lett. 14, 073001. doi:10.1088/1748-9326/ab1b7d
Suprasanna, P., and Jain, S. M. (2022). Biotechnology and induced mutations in ornamental plant improvement. Acta Hortic. 1334, 1–12. doi:10.17660/ActaHortic.2022.1334.1
Talaviya, T., Shah, D., Patel, N., Yagnik, H., and Shah, M. (2020). Implementation of artificial intelligence in agriculture for optimisation of irrigation and application of pesticides and herbicides. Artif. Intell. Agric. 4, 58–73. doi:10.1016/j.aiia.2020.04.002
Tarannum, N., Rhaman, M. K., Khan, S. A., and Shakil, S. R. (2015). A brief overview and systematic approch for using agricultural robot in developing countries. J. Mod. Sci. Technol. 3, 88–101.
Tasaki, K., Yoshida, M., Nakajima, M., Higuchi, A., Watanabe, A., and Nishihara, M. (2020). Molecular characterization of an anthocyanin-related glutathione S-transferase gene in Japanese gentian with the CRISPR/Cas9 system. BMC Plant Biol. 20, 370–414. doi:10.1186/s12870-020-02565-3
Teerarak, M., and Laosinwattana, C. (2019). Essential oil from ginger as a novel agent in delaying senescence of cut fronds of the fern (Davallia solida (G Forst) Sw). Postharvest Biol. Technol. 156, 110927. doi:10.1016/j.postharvbio.2019.06.001
Tilly, N., Hoffmeister, D., Liang, H., Cao, Q., Liu, Y., Lenz-Wiedemann, V., et al. (2012). Evaluation of terrestrial laser scanning for rice growth monitoring. Int. Arch. Photogramm. Remote Sens. Spat. Inf. Sci. 39, 351–356. doi:10.5194/isprsarchives-xxxix-b7-351-2012
Touliatos, D., Dodd, I. C., and McAinsh, M. (2016). Vertical farming increases lettuce yield per unit area compared to conventional horizontal hydroponics. Food Energy Secur 5, 184–191. doi:10.1002/fes3.83
Trevisan, R. G., Bullock, D. S., and Martin, N. F. (2021). Spatial variability of crop responses to agronomic inputs in on-farm precision experimentation. Precis. Agric. 22, 342–363. doi:10.1007/s11119-020-09720-8
Uchida, T. F., and Yamano, T. “Development of a remoto control type weeding machine with stirring chains for a paddy field,” in Proceedings of the 22nd International Conference on Climbing and Walking Robots and Support Technologies for Mobile Machines (CLAWAR 2019), Kuala Lumpur, Malaysia, August 2019, 61–68.
Vendrame, W. A., Xu, J., and Beleski, D. (2022). Evaluation of the effects of culture media and light sources on in vitro growth of brassavola nodosa (L) lindl. Hybrid. Horticulturae 8, 450. doi:10.3390/horticulturae8050450
Vera, J., Conejero, W., Mira-García, A. B., Conesa, M. R., and Ruiz-Sánchez, M. C. (2021). Towards irrigation automation based on dielectric soil sensors. J. Hortic. Sci. Biotechnol. 96, 696–707. doi:10.1080/14620316.2021.1906761
Verbiest, R., Ruysen, K., Vanwalleghem, T., Demeester, E., and Kellens, K. (2020). Automation and robotics in the cultivation of pome fruit: Where do we stand today? J. Field Robot. 38, 513–531. doi:10.1002/rob.22000
Verdouw, C., Beulens, A., and van der Vorst, J. (2013). Virtualisation of floricultural supply chains: A review from an internet of things perspective. Comput. Electron. Agric. 99, 160–175. doi:10.1016/j.compag.2013.09.006
Vincent, D. R., Deepa, N., Elavarasan, D., Srinivasan, K., Chauhdary, S. H., and Iwendi, C. (2019). Sensors driven AI-based agriculture recommendation model for assessing land suitability. Sensors19 19, 3667. doi:10.3390/s19173667
Virnodkar, S. S., Pachghare, V. K., Patil, V. C., and Jha, S. K. (2020). Remote sensing and machine learning for crop water stress determination in various crops: A critical review. Precis. Agric. 21, 1121–1155. doi:10.1007/s11119-020-09711-9
Walls, M. (2006). Agriculture and environment. MTT Agrifood Res. Finl. https://www.oecd.org/agriculture/topics/agriculture-and-the-environment/.
Wang, H., Peng, J., Xie, C., Bao, Y., and He, Y. (2015). Fruit quality evaluation using spectroscopy technology: a review. Sensors 15, 11889–11927.
Wang, B., Ding, Y., Wang, C., Li, D., Wang, H., Bie, Z., et al. G-ROBOT: An intelligent greenhouse seedling height inspection robot. J. Robotics 2022, 1–14. doi:10.1155/2022/9355234
Wang, G., Sun, Y., and Wang, J. (2017). Automatic image-based plant disease severity estimation using deep learning. Comput. Intell. Neurosci. 2017, 1–8. doi:10.1155/2017/2917536
Wang, N., Zhang, N., and Wang, M. (2006). Wireless sensors in agriculture and food industry—recent development and future perspective. Comput. Electron. Agric. 50, 1–14. doi:10.1016/j.compag.2005.09.003
Wani, M. A., et al. (2018). “Floriculture sustainability initiative: The dawn of new era,” in Sustainable agriculture reviews. Editor E. Lichtfouse (Berlin, Germany: Springer). doi:10.1007/978-3-319-75190-0_4
Want, R. (2006). An introduction to RFID technology. IEEE Pervasive Comput. 5, 25–33. doi:10.1109/mprv.2006.2
Wheeler, W. D., Chappell, M., van Iersel, M., and Thomas, P. (2020). Implementation of soil moisture sensor based automated irrigation in woody ornamental production. J. Environ. Hortic. 38, 1–7. doi:10.24266/0738-2898-38.1.1
Witte, Y. D., Harkema, H., and Doorn, W. G. (2014). Effect of antimicrobial compounds on cutGerbera flowers: Poor relation between stem bending and numbers of bacteria in the vase water. Post. Biol. Technol. 91, 78–83. doi:10.1016/j.postharvbio.2013.12.018
Woo, S., Uyeh, D. D., Kim, J., Kim, Y., Kang, S., Kim, K. C., et al. (2020). Analyses of work efficiency of a strawberry-harvesting robot in an automated greenhouse. Agronomy 10, 1751. doi:10.3390/agronomy10111751
Wu, G. F., Huang, L. X., and He, Y. (2008). Research on the sugar content measurement of grape and berries by using Vis/NIR spectroscopy technique. Guang pu xue yu Guang pu fen xiGuang pu 28, 2090–2093.
Xiang, D., Nguyen, C. D., Felter, L., Clark, D., and Huo, H. (2020). The effects of preharvest LED light, melatonin and AVG treatments on the quality of postharvest snapdragon and vase life. J. Floric. Landscaping 6, 14–19. doi:10.25081/jfcls.2020.v6.6236
Yang, H., Lim, S., Lee, J.-H., Choi, J.-W., and Shin, I.-S. (2021). Influence of solution combination for postharvest treatment stage on vase life of cut hydrangea flowers (Hydrangea macrophylla cv. ‘verena’). Horticulturae 7, 406. doi:10.3390/horticulturae7100406
Yong, W., Shuaishuai, L., Li, L., Minzan, L., Ming, L., Arvanitis, K. G., et al. (2018). Smart sensors from ground to cloud and web intelligence. IFAC Pap. OnLine 51, 31–38. doi:10.1016/j.ifacol.2018.08.057
Zhang, B., Xu, X., Huang, R., Yang, S., Li, M., and Guo, Y. (2021a). CRISPR/Cas9-mediated targeted mutation reveals a role for AN4 rather than DPL in regulating venation formation in the corolla tube of Petunia hybrida. Hortic. Res. 8, 116. doi:10.1038/s41438-021-00555-6
Zhang, J., Rao, Y., Man, C., Jiang, Z., and Li, S. (2021b). Identification of cucumber leaf diseases using deep learning and small sample size for agricultural Internet of Things. Int. J. Distrib. Sens. Netw. 17, 155014772110074. doi:10.1177/15501477211007407
Zhang, M., Gao, B., Chen, J., Li, Y., Creamer, A. E., and Chen, H. (2014). Slow-release fertilizer encapsulated by graphene oxide films. Chem. Eng. J. 255, 107–113.
Keywords: artificial intelligence, internet of things, robotics, soilless culture, vertical farming, flower industry, data analytics, image technology
Citation: Wani MA, Din A, Nazki IT, Rehman TU, Al-Khayri JM, Jain SM, Lone RA, Bhat ZA and Mushtaq M (2023) Navigating the future: exploring technological advancements and emerging trends in the sustainable ornamental industry. Front. Environ. Sci. 11:1188643. doi: 10.3389/fenvs.2023.1188643
Received: 17 March 2023; Accepted: 22 May 2023;
Published: 12 June 2023.
Edited by:
Irfan Ullah, Nanjing University of Information Science and Technology, ChinaReviewed by:
Muhammad Zeeshan, Liaoning Technical University, ChinaCopyright © 2023 Wani, Din, Nazki, Rehman, Al-Khayri, Jain, Lone, Bhat and Mushtaq. This is an open-access article distributed under the terms of the Creative Commons Attribution License (CC BY). The use, distribution or reproduction in other forums is permitted, provided the original author(s) and the copyright owner(s) are credited and that the original publication in this journal is cited, in accordance with accepted academic practice. No use, distribution or reproduction is permitted which does not comply with these terms.
*Correspondence: Muneeb Ahmad Wani, d2FuaW11bmVlYjA1QGdtYWlsLmNvbQ==
Disclaimer: All claims expressed in this article are solely those of the authors and do not necessarily represent those of their affiliated organizations, or those of the publisher, the editors and the reviewers. Any product that may be evaluated in this article or claim that may be made by its manufacturer is not guaranteed or endorsed by the publisher.
Research integrity at Frontiers
Learn more about the work of our research integrity team to safeguard the quality of each article we publish.