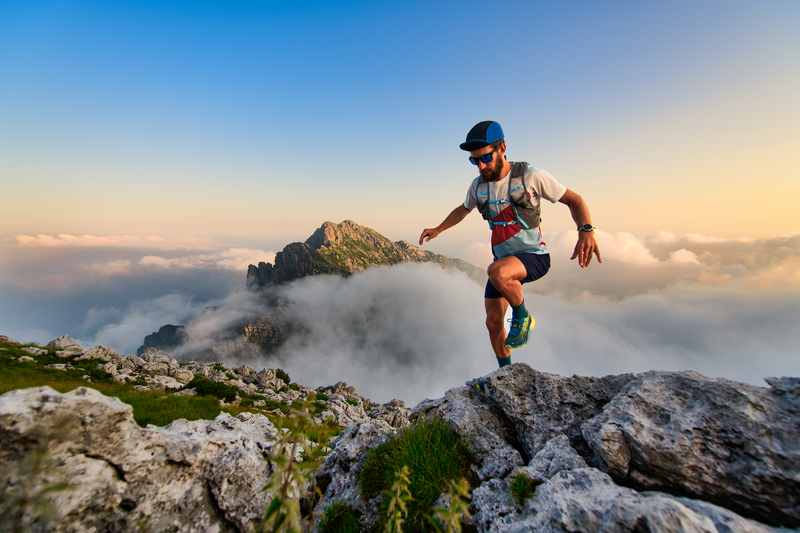
95% of researchers rate our articles as excellent or good
Learn more about the work of our research integrity team to safeguard the quality of each article we publish.
Find out more
ORIGINAL RESEARCH article
Front. Environ. Sci. , 28 August 2023
Sec. Freshwater Science
Volume 11 - 2023 | https://doi.org/10.3389/fenvs.2023.1188139
This article is part of the Research Topic Women at the Frontier of Freshwater Science View all 10 articles
Agricultural irrigation practices have changed through time as technology has enabled more efficient conveyance and application. In some agricultural regions, irrigation can contribute to incidental aquifer recharge important for groundwater return flows to streams. The Henrys Fork Snake River, Idaho (United States) overlies a portion of the Eastern Snake Plain Aquifer, where irrigated agriculture has occurred for over a century. Using irrigator interviews, aerial and satellite imagery, and statistical streamflow analysis, we document the impact of farm-scale decisions on basin-scale hydrology. Motivated to improve economic efficiency, irrigators began converting from surface to center-pivot sprinkler irrigation in the 1950s, with rapid adoption of center-pivot sprinklers through 2000. Between 1978–2000 and 2001–2022, annual surface-water diversion decreased by 311 Mm3 (23%) and annual return flow to the river decreased by 299 Mm3 over the same period. Some reaches that gained water during 1978–2000 lost water to the aquifer during the later period. We use an interdisciplinary approach to demonstrate how individual farm-scale improvements in irrigation efficiency can cumulatively affect hydrology at the landscape scale and alter groundwater-surface water relationships. Return flows are an important part of basin hydrology in irrigated landscapes and we discuss how managed and incidental aquifer recharge can be implemented to recover return flows to rivers.
Improving irrigation efficiency is typically framed as a way to minimize water not put to its intended beneficial use (Burt et al., 1997), water often colloquially characterized as “lost” or “wasted” during conveyance and application (Jensen, 2007; Lankford, 2012). Lining or piping canals and converting to more precise application—in contrast to more traditional techniques, like earthen canals and flood irrigation—are methods touted to increase irrigation efficiency (Richter et al., 2017). Increasing irrigation efficiency is often prescribed in water-limited systems as means of basin-scale water conservation (Contor and Taylor, 2013) and can be attractive to those seeking to reduce stream withdrawals to provide water for environmental objectives or junior water rights-holders (Richter et al., 2017; Owens et al., 2022). Indeed, state, federal, and international programs and policies incentivize increasing irrigation efficiency to conserve water for reallocation to other users (Huffaker, 2008; Levidow et al., 2014; Pérez-Blanco et al., 2021).
But irrigation water lost at the farm-scale to inefficient irrigation practices is retained within basin-scale hydrology. Water delivered in earthen canals or applied in excess of crop uptake infiltrates soils and can recharge aquifers or follow surface and subsurface pathways to return to the river (Venn et al., 2004; Ferencz and Tidwell, 2022). Streamflow diverted for irrigation and recovered in rivers is often referred to as “return flow” and allow water to be used more than once (Jensen, 2007). In fact, in long-irrigated agricultural watersheds, return flows may be a fundamental component of the modern hydrologic cycle (e.g., Kendy and Bredehoeft, 2006; Hu et al., 2017; Oyonarte et al., 2022) and important to junior water users and aquatic ecosystems. Return flows can contribute streamflow during critical low-flow periods (Fernald and Guldan, 2006; Walker et al., 2021; Ferencz and Tidwell, 2022) and provide cool streamflow input (Essaid and Caldwell, 2017; Alger et al., 2021), although return timing is dependent on irrigation application, soil conditions, and local geology (Ochoa et al., 2007; Linstead, 2018). Thus, return flows can bolster the ability to meet environmental flow and temperature objectives in water-limited systems (Lonsdale et al., 2020; Van Kirk et al., 2020) while also supplying water to other users (Owens et al., 2022). In short, return flows are an important part of basin hydrology, but are at risk of decline as policy- and climate-induced water scarcity nudges agricultural regions towards increasing irrigation efficiency (Scott et al., 2014; Pérez-Blanco et al., 2020; Walker et al., 2021).
This sets the stage for an irrigation efficiency trap—where market forces incentivize farmers toward irrigation efficiency improvements that often do not result in the intended basin-scale water conservation—and in fact, may increase water consumption (Grafton et al., 2018; Wheeler et al., 2020). Increased resource consumption due to increased efficiency is described by the Jevons paradox (York and McGee, 2016) and has been well documented in theoretical and modeling studies related to irrigation. Such a change in water consumption is partially due to a difference in scale, where improving irrigation efficiency is perceived differently at the farm scale than the basin scale (Qureshi et al., 2011; Lankford et al., 2020). Irrigators consider increasing irrigation efficiency as a component of improving their individual economic efficiency, i.e., maximizing the difference between production benefits and input costs (Cai et al., 2003; Qureshi et al., 2011). Thus, incentive is strong for irrigators to use their full water allocation by putting more land into production or harvesting an additional or more water-intensive crop (English, 1990; Ward and Pulido-Velazquez, 2008; Xu and Song, 2022)—particularly within water management structures that lack mechanisms for reducing water allocations to a given user to reallocate for other purposes (e.g., doctrine of prior appropriation). Social scientists have documented that some farmers perceive increased irrigation efficiency as a means to maximize revenue, rather than to reduce total on-farm water consumption (Knox et al., 2012; Wheeler et al., 2020; Hamidov et al., 2022). Physical scientists have clearly documented that high irrigation efficiency risks an increase in consumptive water use for a given water allocation (Ward and Pulido-Velazquez, 2008; Scott et al., 2014; Grafton et al., 2018), thus diminishing river return flow (Hu et al., 2017; Linstead, 2018). Yet, the idea to use farm-scale irrigation efficiency for basin-scale water conservation persists (Pérez-Blanco et al., 2021).
Combatting the irrigation efficiency trap requires understanding how humans interact with irrigated landscapes and water resources at multiple scales. Combining irrigator surveys with physical measurements of landscape characteristics, irrigation conversion, streamflow diversion, water availability, and return flows allow for cross-scale examination and integrate the socio-hydrological nature of the problem. Few studies document the irrigation efficiency trap from farm-scale decisions to basin-scale hydrologic outcomes with measured social and physical data (e.g., Wheeler et al., 2020; Anderson, 2022). But irrigation systems are complex social-ecological systems (Lam, 2004) and integrating the hydrologic and social components of irrigation efficiency are important for system understanding and resilience (Fernald et al., 2015; Dunham et al., 2018). To adapt and prepare accordingly, we must examine place-based farm-scale irrigation decisions and how these decisions collectively impact basin-scale hydrology. We can then identify strategies that maintain agricultural and environmental water uses, are robust to climate variability, and are actionable for decision makers (Welsh et al., 2013; Lankford et al., 2020).
We use the Henrys Fork watershed, Snake River, Idaho (United States)—an agricultural watershed that exemplifies those throughout the American West—for place-based research on the relationship between farm-scale decisions and watershed-scale hydrology. Irrigated agriculture has been in place since 1879 (Van Kirk and Griffin, 1997) and contributes to a $10 billion USD regional economy (Idaho Water Resources Board, 2009). The Henrys Fork overlies the headwater portion of the Eastern Snake Plain Aquifer (ESPA; Figure 1), a 28,000 km2 unconfined aquifer that provides baseflow to the Snake River system (Hipke et al., 2022). In addition to agriculture, the Henrys Fork hosts a recreational fishery worth $50 million USD (Van Kirk, 2021) and is an important component of local watershed management (Joint Committee, 2018). However, studies have modeled a decline in irrigation return flow and groundwater discharge to the river since 1980 (Contor et al., 2004; Sukow, 2021). The reduction of return flow in the Henrys Fork is part of a larger regional hydrologic change, where groundwater pumping, increased irrigation efficiency, and decreased surface-water diversion across southern Idaho has diminished ESPA storage (Stewart-Maddox et al., 2018) and contributions to Snake River streamflow (Olenichak, 1998). Thus, the irrigation efficiency trap is on display in the Henrys Fork and surrounding region.
FIGURE 1. The Henrys Fork watershed (A) and the watershed relative to the Eastern Snake Plain Aquifer (B). Data sourced from Airbus, U.S. Geological Survey, NGA, NASA, CGIAR, NCEAS, NLS, OS, NMA, Geodatastyrelsen, GSA, GSI, and the GIS User Community.
Therefore, we use a unique interdisciplinary dataset that includes 1) irrigator interviews to understand motivations for irrigation conversion through time, 2) landscape imagery analysis to quantify spatiotemporal irrigation conversion, and 3) hydrologic measurements with statistical analysis from 1978 to 2022 to quantify changes in surface-water diversion, reach gains, and return flows to the river and examine hydrologic change from the farm-to basin-scale. Our research questions are:
1) What motivated farmers to convert to more efficient irrigation application?
2) When and at what rate did farmers improve their irrigation efficiency?
3) How did these changes affect basin-scale hydrology?
Our first two questions consider on-farm irrigation efficiency, defined as evapotranspiration divided by the water applied to a field. Our third research question considers project-level irrigation efficiency, defined as water consumptively used by crops (i.e., evapotranspiration) divided by total water withdrawn (Thompson, 1988; Burt et al., 1997; Zalidis et al., 1997). Project-level efficiency accounts for two sources of inefficiency: 1) loss of water in the conveyance system between the point of diversion and the point of field application, and 2) water applied at the field scale that is not consumed by crops. Losses in both components of the irrigation system can be due to evaporation and to seepage into soils and aquifers below the crop root zone.
We use our results to outline the potential for aquifer recharge to maintain and recover return flows.
The Henrys Fork watershed is 8,300 km2 located in the headwaters of the Snake River Basin, Idaho, United States, ranging in elevation from 1,470 m to 3,800 m (Figure 1). Snowmelt and headwater springs provide an average annual unregulated streamflow of 3,140 Mm3. The surface-water system is managed to provide irrigation to 1,012 km2 of agricultural land in the low-elevation areas of the watershed, where producers primarily grow potato, alfalfa, and grain crops (U.S. Bureau of Reclamation, 2012b). Surface water is stored in three reservoirs in the watershed (Henrys Lake, 111 Mm3; Island Park Reservoir, 167 Mm3; Grassy Lake, 18.8 Mm3). Teton Dam, on the Teton River, was completed in 1975 to store 247 Mm3, but the dam failed in 1976 as the reservoir was filling for the first time and was not rebuilt (Reisner, 1993; U.S. Bureau of Reclamation, 2012a).
On average, 1,400 Mm3 of surface water (45% of average annual unregulated flow) is diverted for agricultural irrigation (U.S. Bureau of Reclamation, 2012b) and is largely delivered by unlined, earthen canals that divert water directly from the Henrys Fork and its tributaries. Irrigators also use groundwater, which accounts for ∼25% of the total water withdrawn for irrigation in the watershed. Proportional use of groundwater for irrigation is similar across the ESPA and the state of Idaho as a whole. In 2015, total annual groundwater pumped from the ESPA in the Henrys Fork watershed was ∼200 Mm3 (Lovelace et al., 2020). Although long-term watershed-specific data on groundwater withdrawal are not available, groundwater withdrawal for irrigation in Idaho has been increasing at a rate of ∼19 Mm3 per year, while withdrawal of surface water for irrigation has been decreasing at ∼61 Mm3 per year (see Supplementary Material).
Access to irrigation water is subject to water-rights priority based on the prior appropriation doctrine (Van Kirk et al., 2019) and largely organized under one irrigation district and ∼30 canal companies (Van Kirk and Griffin, 1997). Under the prior appropriation doctrine in the western United States, state governments allocate surface water based on the date water was first diverted and put to “beneficial use” as defined by the state (Van Kirk et al., 2019). Irrigation districts and canal companies are local entities responsible for managing conveyance systems for water delivery to individual irrigators who are shareholders within the organization (Armstrong and Jackson-Smith, 2017). In the Henrys Fork, surface water users have rights senior to those of groundwater users and water resources are conjunctively managed (Stewart-Maddox et al., 2018). The basin is fully adjudicated, and surface water rights include allowance for reasonable conveyance loss (Vonde, 2016).
Irrigated land in the Henrys Fork watershed is separated into four regions: North Fremont, Egin Bench, Lower Watershed, and Teton Valley (Table 1). These four primary irrigated regions account for >95% of surface-water diversion in the watershed and >95% of the current and historic canal conveyance system (Joint Committee, 2018); all other irrigated acreage is primarily groundwater-irrigated. Regarding water rights, North Fremont has predominantly junior water rights and experiences significant water shortages annually (U.S. Bureau of Reclamation and Idaho Water Resource Board, 2015). Egin Bench has predominantly senior water rights, surplus water in average water years, and meets its demand even in successive drought years. The Lower Watershed meets most of its irrigation demand in average water years, but experiences a deficit in drought years that follow a drought year (U.S. Bureau of Reclamation and Idaho Water Resource Board, 2015). Essentially all conveyance in the Lower Watershed and Egin Bench is delivered through the 19th-century earthen canal system. Most conveyance in North Fremont has been converted to pipelines, beginning with small canals in the 1970s. We exclude Teton Valley from our analysis because the irrigated region does not interact with the ESPA, but rather a smaller, hydraulicly distinct aquifer (Bayrd, 2006). For all irrigation regions studied, we can assume a constant value for total irrigable area as no new irrigation rights have been granted in decades, particularly since the groundwater moratorium in the 1990s (Van Kirk et al., 2019). Thus, no new land has been put into agricultural production.
TABLE 1. Characteristics of irrigated study regions within the Henrys Fork watershed by irrigation year (November–October). The standard deviation for mean annual precipitation and ET are reported parenthetically. We report data for two periods of time, 1978–2000 and 2001–2022. The year division for these time periods was determined through analysis in this paper. Diversion data are from Idaho Water District 01. Average annual precipitation and evapotranspiration were calculated from gridMET for alfalfa reference within each irrigated study region (Abatzoglou, 2013). The gridMET period of record begins in 1980 and has 4 km resolution. We assume a constant value for total irrigable land.
Our study considers two irrigation efficiency scales: on-farm and project. At the farm scale, efficiency is related to mode of irrigation application. Four modes of irrigation application are currently used in the watershed: flood irrigation and sprinkler irrigation via hand-line, wheel-line, and center-pivot (Table 2). In the Henrys Fork watershed, the estimated 1980–2010 average for on-farm irrigation efficiency (evapotranspiration divided by water applied) was 60% for North Fremont and 55% for each of the Egin Bench and Lower Watershed (U.S. Bureau of Reclamation, 2012b). Project-scale efficiency for the entire Henrys Fork watershed from 1979 to 2008 was 26% (U.S. Bureau of Reclamation, 2012b). Project-scale irrigation efficiency is water consumptively used by crops (i.e., evapotranspiration) divided by total water withdrawn and includes loss within canal conveyance.
TABLE 2. Irrigation type definitions adapted from Bjorneberg and Sojka (2005) and Lonsdale et al. (2020) and irrigation type application efficiencies with appropriate citations. Application efficiency is defined as the fraction of average irrigation water applied that meets a target irrigation depth for an irrigation event (Burt et al., 1997).
Each irrigated region differs in terms of its gradient and soil type, important factors for irrigation application. Flood irrigation requires flatter terrain (0.5%–4% gradient), whereas wheel-line and center-pivot sprinklers are appropriate for steeper slopes ≤15% and hand-line sprinklers can handle slopes ≤20% (Brown, 2008; Barnhill et al., 2009). Egin Bench and the Lower Watershed have predominantly flat terrain (≤0.5% slope), whereas the North Fremont region is steeper with greater heterogeneity (0%–20% slope; Supplementary Figure S2). Regarding soil, Egin Bench is almost exclusively loamy fine sand, noted for its high infiltration and low runoff rates (Supplementary Figure S2). North Fremont has soils that range from moderate infiltration and runoff to soils that are near-impervious with high runoff potential. Hydrologic soil groups in the Lower Watershed are heterogeneous (Supplementary Figure S2).
We conducted 20 semi-structured phone interviews in July 2022 to 1) identify sociological, economic, and geographic factors that prompt farmers to convert to more efficient irrigation in the Henrys Fork watershed and 2) extend temporal flood-to-sprinkler conversion data beyond the period aerial and satellite imagery were available. Staff at the Henry’s Fork Foundation, a local watershed conservation organization and sponsor of this research, developed a key informants list for initial contact; additional participants were identified using the snowball method (Hay, 2005). We interviewed current and former agricultural irrigators with a variety of farm acreage, irrigation district and canal company representatives, and second- or third-generation irrigators with knowledge of historic family operations related to surface-water irrigation. Our study area is rural, with a population of ∼28,500 (United States Census Bureau, 2022a; United States Census Bureau, 2022b; United States Census Bureau, 2022c). Most farms in our study area are family-owned and operated. Eighty percent of farm operations in the study area are <500 acres, 10% are 500–999 acres, and the remaining 10% are ≥1,000 acres (USDA National Agricultural Statistics Service, 2017a; USDA National Agricultural Statistics Service, 2017b). It is likely our sample was biased towards individuals who are highly active in and knowledgeable about local and regional water management. Participation rate may have been negatively impacted by conducting interviews during the irrigation season when irrigators have limited capacity, drought limiting water rights allocation and contributing to high tension around water conversations, and perceptions of the Henry’s Fork Foundation and its intent in conducting this research.
Interview data were collected in field notes and summarized in analytical memos (Hay, 2005)—a reflexive activity where researchers explore topics in a narrative structure (Birks et al., 2008). We used these analytical memos for inductive coding and thematic analysis (Attride-Stirling, 2001; Saldana, 2016). See the Supplementary Material for interview instrument.
We used aerial photography and Landsat satellite imagery from 1986 to 2020 to evaluate spatiotemporal trends in irrigation practices (Supplementary Table S2). From satellite imagery, it was difficult to differentiate fields that were flood irrigated versus those that were irrigated via hand- or wheel-line sprinkler. Thus, we visually assigned irrigation type as pivot vs. not-pivot in June or July for each field using imagery from 1988 to 2002 (every 2 years) and 2005–2020 (every 5 years). We assigned pivots to circular fields and quantified pivot acres, assigning full pivot circles 0.63 km2, three-quarter circles 0.47 km2, and half pivot circles 0.32 km2.
To verify the presence and extent of flood irrigated land currently in production, we identified eighteen fields in the Lower Watershed and two fields on the Egin Bench that appeared to be flood irrigated in Google Earth imagery from September 2015 and June 2017. We traveled to these sites in July 2021 to verify irrigation type.
We used statistical model selection and multi-model inference with Akaike’s Information Criterion (AIC) to analyze annual time series data for five key measures of water supply and use: 1) surface-water irrigation diversion, 2) river reach gain, 3) unregulated streamflow, 4) total diversion minus reach gain (net watershed withdrawal), and 5) total watershed inflow minus watershed outflow (net watershed export). We conducted our analysis at two spatial scales—watershed and subreach. We conducted the watershed-scale analysis for irrigation years 1978–2022, where the irrigation year is defined as November 1 through October 31. The 1978–2022 period is the longest over which complete daily data are available. Some sub-reach analysis was done for irrigation years 2004–2022, the longest period over which streamflow data were available for the sub-reaches.
The primary hydrologic data used in the analysis were daily streamflow from U.S. Geological Survey (USGS) monitoring stations, surface-water diversion and exchange well injection reported by Idaho Water District 01 (the basin-wide water administration agency), reservoir volume from the U.S. Bureau of Reclamation, and precipitation and evapotranspiration data from U.S. Bureau of Reclamation and Natural Resources Conservation Service. Exchange wells inject groundwater directly into the Teton River (Olenichak, 2020). The exchange wells are operated only during very dry years, as are other exchange wells in the watershed, which inject water into the Henrys Fork (U.S. Bureau of Reclamation and Idaho Water Resource Board, 2015). Of the five key measures assessed, all but surface-water diversion required computation (detailed below).
We estimated reach gain on reaches of the Henrys Fork and Teton River that interact with the ESPA (Figure 2). These reaches do not gain appreciable water from tributary streams and do not contain storage reservoirs. Hence the net gain from a combination of surface-irrigation return flow and groundwater input into these reaches can be calculated as:
FIGURE 2. U.S. Geological Survey stream gages used in the water balance and reach gain calculations.
Negative reach gains indicate a reach loss.
Unregulated streamflow for the three sub-watersheds was calculated for upper Henrys Fork, Fall River, and Teton River as:
Regulated streamflow data for Equation 2 used three long-term USGS stream gaging stations downstream of all source tributaries and immediately upstream of interactions with the ESPA (Supplementary Figure S3 and Supplementary Table S3). The reservoir evaporation term in Equation 2 is the net difference between evaporation and precipitation on reservoir surfaces. If positive, this represents a loss via evaporation, and if negative represents a gain via direct precipitation in reservoirs. Eqs 1, 2 largely coincide with those used by Water District 01 to administer water rights in the watershed (Olenichak, 2020). Total watershed unregulated flow is the sum of unregulated flow in the three sub-watersheds.
For the watershed-scale water balance (total inflow minus outflow; net basin export), we included all sources of inflow available for surface-water diversion, which is given by:
Note: We define net basin export as the sum of consumptive use and water that exits the basin as groundwater flow to the ESPA.
Annual watershed outflow is regulated streamflow at the downstream-most gage on the Henrys Fork near the bottom of the watershed at the confluence with the main Snake River (Figure 2). Eq. 1 can be rearranged to yield:
At the watershed scale, Equations 1–3 can be used to obtain an alternate derivation of Equation 4 showing that net withdrawal of water from the watershed can be calculated either as the difference between diversion and unregulated flow or as the difference between total watershed inflow and watershed outflow. We analyze both to demonstrate this equivalence and better interpret the role of reach gains in the watershed-scale water balance.
We used an AIC-based approach to statistically model each of our five key hydrologic measures through the 1978–2022 study period and quantify changes through time. The basic AIC method is to propose a set of candidate models, rank them according to AIC, and then use a measure of relative evidence for the models in the candidate set to calculate a final model that is a weighted average of all models in the set (Burnham and Anderson, 2002; Anderson, 2008; Claeskens and Hjort, 2008). We used a modification of AIC known as AICc (AIC with small-sample correction), which includes an additional term that increases the overfitting penalty when the number of fitted parameters becomes large relative to the sample size.
All of the data analyzed here occur in a time series of 45 annual values, and all models were fit in the framework of autoregressive time series models using the arima function in the R programming environment (R Core Team, 2022). We proposed five types of structural models describing potential temporal trends in the data:
1. Null model: data described by a single mean (one structural parameter).
2. Piecewise constant: data described by two means, one for each of two distinct time periods (two structural parameters describing the means plus a third defining the time period breakpoint).
3. Linear trend (two structural parameters).
4. Piecewise trend: data described by linear trend over the first time period and constant mean over the second (three structural parameters plus a fourth defining the time period breakpoint).
5. Quadratic (three structural parameters).
The breakpoints in models 2 and 4 were not specified a priori but were determined through the maximum-likelihood model-fitting process. However, to avoid the possibility of a few extreme water years at the beginning or end of the time series artificially introducing a breakpoint near the endpoints of the study period, we restricted the range of breakpoints to 1991–2009. This ensured that each of the two time periods was at least 13 years long.
For each of the above, we proposed two sub-models, one in which unregulated flow was used as a covariate (one additional parameter) and another without the covariate. We included unregulated flow as a covariate because diversion in prior appropriation systems is generally greater in years of greater water supply. Incorporation of water supply as a covariate removes the confounding effect of short-term variability in water supply on actual long-term trends. For each of the models described so far, we proposed one each with and without first-order serial autocorrelation (one additional parameter). Finally, we fit one set of models to normally distributed residuals and another with lognormally distributed residuals, the latter achieved by log-transforming the response variable. Because reach gains could be negative and were on the order of 125 Mm3, we used the transformation
Lastly, we calculated Pearson correlations (
From the twenty irrigator interviews, some had experience across irrigation study regions and could describe practices across the watershed. Thus, we received a total of 24 responses: 9 from North Fremont, 6 from Egin, and 9 from the Lower Watershed. Nineteen irrigators reported experience with either flood-to-sprinkler conversion or increasing sprinkler mechanization (i.e., converting from hand- or wheel-line to center pivot irrigation). Five irrigators continue to flood irrigate to a degree and mostly in the Lower Watershed. We recognize small sample size can carry bias, particularly with our non-random interviewee selection. However, we prioritized representation within each irrigated area given limited resources and previous work identifying each area as different in their irrigation practices, due to differences in physical geography and water rights priority (U.S. Bureau of Reclamation and Idaho Water Resource Board, 2015).
Across the study regions, economic efficiency and physical geography were primary motivators for converting irrigation practices. Responses about economic efficiency centered on water and labor, separately. Irrigators with flood irrigation experience noted how pivot irrigation reduced water lost to seepage and evaporation. Other irrigators noted that hand- and wheel-line sprinklers are subject to water loss through wind, sometimes double-watering crops while leaving others dry. With the water savings earned through increased irrigation efficiency, irrigators noted their ability to harvest an additional crop during the growing season—producing higher crop yields and crops of better quality. Conversion to pivot irrigation also significantly reduced the labor required to successfully irrigate via flood, hand-line, or wheel-line, improving economic efficiency.
Responses about physical geography noted how irrigation conversion better accommodated for land slope and soil profiles. Some regions are not conducive to flood irrigation. For North Fremont irrigators, steeper terrain prevented flood irrigation success and motivated increased sprinkler mechanization in the 1950s and 1960s as technology became available. In the Lower Watershed, irrigators with land impacted by the 1976 Teton Dam Failure noted that sediment deposition altered land slope and reduced flood irrigation efficiency, thus motivating their conversion to sprinkler irrigation. Irrigators on the Egin Bench coalesced around one story: the region has sandier soils (Supplementary Figure S2) and historically used subirrigation—subsurface application that raises the water table to crop roots (Bjorneberg and Sojka, 2005)—until a single irrigator converted to sprinkler application in the late 1970s/early 1980s, thus lowering the local water table and making subirrigation untenable. This initiated a conversion to sprinkler irrigation on the Egin Bench, where initial adopters converted to sprinkler application due to the physical limitations of subirrigation and secondary adopters converted to sprinklers to participate in the increased yield experienced by their neighbors. We do not know why one irrigator in Egin Bench first converted from subirrigation to sprinkler.
Topics related to environmental stewardship were evoked as justification for both converting and not converting to more efficient irrigation. Irrigators who converted to sprinkler application noted its benefit for minimizing soil erosion and improving soil health, oftentimes pairing these benefits with mention of higher yield and crop quality. Irrigators who continue to flood irrigate drew attention to its benefits for wildlife, aquifer recharge, and maintenance of groundwater springs.
Respondents noted cost, water right seniority, and land composition as factors limiting their ability to convert to more mechanized application and/or center-pivot sprinklers. Irrigators identified the high upfront cost of center-pivot sprinklers as the primary barrier to conversion, with the applications for federal cost-sharing programs to purchase equipment described as “a pain in the ass” by one interviewee. Irrigators also highlighted that those with senior water rights lack incentive to convert to more efficient sprinkler application, as they are less likely to face curtailment. Irrigators with rocky and vegetated land noted center-pivot installation is infeasible.
In terms of conversion through time, interviewees in the North Fremont region converted from flood to sprinkler irrigation prior to the 1970s. Irrigators from the Egin Bench and Lower Watershed lagged in their flood-to-sprinkler conversion by at least a decade, with conversion beginning largely in the 1970s. Conversion to sprinkler on the Egin Bench was completed by 2000, whereas respondents in the Lower Watershed reported converting their flood operations through to 2010. Increased sprinkler mechanization continued through the 2000s in all regions. However, Egin Bench mechanized prior to the 1990s while North Fremont and the Lower Watershed mostly increased their sprinkler mechanization prior to the 2000s.
Overall, center-pivot sprinkler irrigation increased between 1988 and 2020. On the Egin Bench, total acres irrigated by pivots increased rapidly between 1988 and 2000—from 22.1% to 73.1% (Figure 3B). This rate of pivot expansion slowed after 2000, with 87.2% of irrigated acres using center-pivot sprinklers by 2020 (Figure 3B). The rate of conversion on the Egin Bench, where water users have senior water rights of the three study regions, did not align with commentary in irrigator interviews about senior water rights holders lacking incentive to convert to more efficient irrigation application. However, slowed expansion after 2000 aligns with irrigator interviews, where none of our interviewees on the Egin Bench reported conversion after 2000. In contrast, the rate of conversion from non-pivot irrigation to center-pivot sprinklers has been consistent through time in the Lower Watershed. Between 1988 and 2020, the percentage of irrigated acres with center-pivot sprinklers increased from 5.9% to 47.0%—an average annual rate of 1.3% (Figure 3). This result also aligns with irrigator interviews, particularly given some irrigators in the Lower Watershed continue to flood irrigate. Flood irrigation has been negligible in North Fremont since sprinkler irrigation became available because of the steeper terrain. The rate of center-pivot installation in North Fremont paralleled that of the Lower Watershed and, as of 2020, 36.7% of North Fremont was irrigated with center-pivot sprinklers. However, much of the land with irrigation rights cannot be irrigated due to its gradient, rocky substrate, and wetlands. Therefore, we estimate center-pivot sprinklers are used on ∼80% of the total land area that is regularly irrigated from year to year.
FIGURE 3. Panel A is change in pivot-irrigated acres for Egin Bench (1987–1998) and the Lower Watershed (1987–2021) (Imagery is from USDA FSA NAIP, July 2019). Panel B is percentage of acres irrigated with pivots for all three irrigation study areas for 1988–2020.
Lastly, ground-truthing 2015 and 2017 satellite imagery confirmed the presence of flood irrigation as of July 2021. Of the twenty fields observed, fifteen were flood irrigated and five were irrigated by wheel-line sprinklers. Of the fifteen flood irrigated parcels, thirteen were growing barley, hay or alfalfa and two were pasture fields. This exercise confirmed that aerial imagery could not be used to distinguish wheel-line sprinkler irrigation from flood irrigation, as both have rectangular irrigation patterns.
The AICc analysis provided strong evidence for a steady decline in diversion from the late 1970s until 2000, followed by a sharp drop to a much lower, but constant level of diversion from 2001 to 2022 (Figure 3). Six models accounted for 99.5% of the AICc weight, and all six included terms quantifying the continuous decline from 1978 to 2000 (Supplementary Table S4). Four of those, accounting for 87.9% of the AICc weight, identified the step-wise drop between 2000 and 2001. Watershed-total unregulated streamflow appeared as a covariate in the top four models, accounting for 98.7% of the model weight. Annual watershed-total diversion dropped from a mean of 1,374 Mm3 in the 1978–2000 period to 1,063 Mm3 in 2001–2022, a decrease of 311 Mm3 (23%). The pattern and relative magnitude of decrease in diversion was uniform across all irrigated areas (Table 2; Supplementary Figure S4). Within the irrigation year, diversion was similar between the two time periods early and late in the irrigation season—April/May and October—but greater in the 1978–2000 period during June–September and during the winter. Winter diversion is allowed under water rights for stock water and other non-irrigation uses.
Evidence was equally strong that watershed-total reach gain has declined. Eight models accounted for 99.5% of the model weight, and all eight included terms modeling a decrease from 1978 until the early 2000s (Figure 4; Supplementary Table S5). Watershed-total unregulated streamflow appeared as a covariate in four of these models, accounting for 94.3% of model weight. Models containing a step-wise drop in the early 2000s accounted for 98.3% of model weight, but the location of the step differed across models. The top two models (93.1% of model weight) identified the step-wise drop as occurring between irrigation years 2002 and 2003; three other models (5.2% of weight) fit the step-wise drop between 1999 and 2000 or 2000 and 2001. The averaged model thus shows that the decline in reach gains lags that of diversion and is slightly more gradual (Figure 4). Using the 1978–2000 vs. 2001–2022 time division identified by the diversion trends, reach gain dropped from an annual mean of 322 Mm3 in the 1978–2000 period to 23.1 Mm3 in 2001–2022, a decrease of 299 Mm3. We cannot calculate percent decrease in reach gains because reach gains can sometimes be zero or negative. Watershed-total reach gain was negative in 8 years in the recent period, whereas gain was positive in each year prior to 2001. Mid-summer reduction in reach gain between the two time periods averaged ∼11 m3/s.
FIGURE 4. Trends in Henrys Fork watershed total diversion, reach gains, and unregulated streamflow for irrigation years 1978–2022.
Even though unregulated streamflow was a strong and positive covariate in all models of diversion and reach gain through time, on its own, it showed only a very modest decrease since 1978 (Figure 4). Six models accounted for 99.4% of the model weight, and the top model (34.2% of model weight) included only a constant term and first-order autocorrelation (Supplementary Table S6). Three of the models (37.2% of weight) identified a step-wise decline, and in all three, the step occurred between 2000 and 2001. Annual unregulated streamflow averaged 3,234 Mm3 in the 1978–2000 period and 2,738 Mm3 in the later time period, a decline of 496 Mm3 (15.3%). Unregulated flow was nearly constant during the early period but has decreased at a rate of 3.9 Mm3 per year since 2001, for a total reduction of 82.1 Mm3 (2.9%) in the last 20 years.
Net watershed withdrawal—the difference between watershed-total diversion and reach gain—showed no evidence of change since 1978. The top two models accounted for ∼100% of model weight, and both were models of a constant over the entire study period (Figure 5; Supplementary Table S7). As expected from the mathematical definitions, net watershed export—the difference between total watershed inflow and outflow—was equivalent to net withdrawal, excluding differences from reservoir evaporation/precipitation, which is highly variable at the daily scale. Net watershed withdrawal averaged 1,052 Mm3 in 1978–2000 and 1,041 Mm3 in 2001–2022, a 1% decline. Over the entire study period, the net annual withdrawal of water from the watershed, measured either as diversion minus gain or inflow minus outflow, averaged 1,046 Mm3 with an interannual coefficient of variation of 8.3%. Despite much higher winter and mid-summer diversion in the 1978–2000 period (Figure 4), net basin export showed little difference between the two time periods across the irrigation year (Figure 5).
FIGURE 5. Net watershed withdrawal and export in the Henrys Fork watershed for irrigation years 1978–2022.
Pearson correlations among the three primary response variables were strong only between reach gain and diversion and then only at the watershed scale and only over the entire study period (Table 3). Correlations between diversion and reach gain were weak otherwise. Correlations between diversion and unregulated flow were positive and moderate for all reaches and time periods except the watershed total over 1978–2022. Reach gain and unregulated flow showed little correlation, other than a correlation of 0.55 for the watershed total over 1978–2022. Thus, reach gains were largely independent of unregulated streamflow whereas diversions were generally higher in wet years.
TABLE 3. Correlation coefficients between diversion, unregulated flow, and reach gains within a given subreach or spatial extent (ex. Comparing diversion upstream of the middle Henrys Fork to unregulated flow into that node). Cell shading uses light to dark to signify weak to strong correlations. Correlations were computed based on data availability; subreach data for the Teton River were limited to 2004–2022.
On-farm irrigation efficiency in the Henrys Fork watershed has increased over the last 70 years. Local irrigators began converting flood irrigation to more mechanized sprinkler application in the 1950s in North Fremont and in the 1970s in the Egin Bench and Lower Watershed to improve their economic efficiency and accommodate for land composition. As of 2020, 87% of the Egin Bench, 47% of the Lower Watershed, and ∼80% of North Fremont used center-pivot sprinkler application. Those changes to irrigation efficiency have altered Henrys Fork hydrology. Between 1978 and 2000, surface-water diversion and reach gains both decreased substantially and by about the same volume—311 Mm3 and 299 Mm3—then stayed relatively constant from 2001 to 2022. Hydrologic changes have been largest in the lower Henrys Fork/Teton River—most likely in response to rapid changes in irrigation practices on the Egin Bench through 2000. Although reach gains declined through the period of record, stream gage data show that net watershed export—the sum of consumptive use and water that exits the basin as groundwater flow to the ESPA—has not changed, despite a 3% decrease in unregulated streamflow during 2001–2022 from extended drought in the West (Williams et al., 2020). This result, in combination with interpretation of additional regional studies, indicate consumptive use has increased with irrigation efficiency in the Henrys Fork watershed. Furthermore, our data show that prior to 2001, reach gains in our system were equivalent to irrigation return flows, i.e., water diverted from the river in excess of what could be consumed by crops or recharged to the regional aquifer.
Farm-scale decisions in irrigation application have changed the irrigated landscape within the Henrys Fork watershed. The timing and rate of sprinkler adoption on the Egin Bench aligns with previous work in the watershed documenting conversion to mostly center-pivot sprinkler irrigation by the mid-1990s (Contor, 2004). The conversion of 61% of total irrigable land to center-pivot irrigation in the Egin Bench and Lower Watershed combined also aligns with irrigation conversion to more precise application elsewhere in the United States (Maupin et al., 2014). Irrigator motivations and inhibitors toward adopting more efficient irrigation application in the Henrys Fork are similar to those of irrigators elsewhere in the United States and globally. The irrigators we interviewed noted a desire to reduce water loss, a common perspective when water intended for a specific beneficial use is apparently “lost” or “wasted” to seepage or evaporation (Lankford, 2012; Cantor, 2017).
Reduced labor costs were also a factor in the adoption of more irrigation-efficient application technologies in the Henrys Fork. Flood irrigation can take 12–24 h to execute, depending on crop, soil, field size, and slope, and requires monitoring to move tarp dams (Bjorneberg and Sojka, 2005). Hand-line sprinklers need to be connected, disconnected, and moved to their new application location every 8–24 h (Bjorneberg and Sojka, 2005). Center-pivot sprinklers, on the other hand, uniformly water large areas with little labor (Bjorneberg and Sojka, 2005; Brown, 2008), and can be operated remotely (Avello Fernández et al., 2018)—reducing labor costs up to 90% (Brown, 2008). Irrigators elsewhere in the world have also switched from surface to sprinkler irrigation due to labor costs. In Spain, Lecina et al. (2010) documented that irrigation modernization partially occurred due to the high labor requirement of surface application and a diminishing workforce. Irrigators surveyed in Alberta, Canada also reported reduced labor cost as a factor in adopting more efficient irrigation technologies (Wang et al., 2015).
In addition to labor, Henrys Fork irrigators noted the benefit of increased irrigation efficiency to crop yield and quality, which directly affect income. Globally, irrigators report adopting more efficient irrigation technology to improve crop yield and quality too. For example, onion and potato farmers in Morocco’s Saïss plain largely adopted drip irrigation to increase their yield (Benouniche et al., 2014). Irrigators of low-value crops like wheat and barley in Alberta, Canada also reported yield as a motivator for improving their irrigation efficiency (Wang et al., 2015). English vegetable farmers for high-value grocery markets receive higher financial benefit from crop quality than crop yield and make irrigation decisions accordingly (Knox et al., 2012).
In our study, soils informed decisions regarding flood versus sprinkler application and, in combination with local geology, soils contributed to the lagged response of reach gains to surface-water diversion. In regions where soil salinity and nutrient loading are concerns, increasing irrigation efficiency may be a worthwhile pursuit to address water quality degradation created by return flows to streams, as has been documented in Spain’s Ebro Basin (Causapé et al., 2006), in the Chiredzi and Runde Rivers in Zimbabwe (Nhiwatiwa et al., 2017), and in the Murray-Darling Basin in Australia (Walker et al., 2021).
Irrigators in the Henrys Fork who have yet to increase their irrigation efficiency noted the high cost of sprinklers. The financial barriers to increasing irrigation efficiency are documented in farming communities worldwide (Koech et al., 2021; Babin et al., 2022). Advocates for increased irrigation efficiency acknowledge these financial barriers and sponsor subsidies to promote access to more efficient irrigation application technologies (Huffaker, 2008; Molle and Tanouti, 2017; Jordan et al., 2023). Critics of these subsidies argue that they facilitate increased consumptive use (Huffaker, 2008; Wheeler et al., 2020), favor larger farms (Jordan et al., 2023), and may put irrigators at greater financial risk as these subsidies enable operation expansion (Scott et al., 2014; Schirmer, 2017). We were unable to determine the role of subsidies in local irrigation conversion. However, we did receive separate comments on the nuisance of cost-share applications, general wariness of government influence, and a concern that larger farms were more adaptable than smaller operations. Although we do not necessarily advocate for subsidies to increase irrigation efficiency, when creating watershed-scale water conservation or irrigation intervention programs, we recommend assessing local attitudes towards the program and program sponsors, as well as their accessibility to diverse farm operations (e.g., Ricart and Clarimont, 2016; Sanchis-Ibor et al., 2021).
Overall, most irrigators in the Henrys Fork watershed who we interviewed revealed that they made decisions regarding irrigation efficiency based on economic efficiency. These results adhere to the common framing of irrigators as economically rational actors who seek to maximize their individual benefit (Qureshi et al., 2011; Contor and Taylor, 2013; Graveline, 2016). Boelens and Vos (2012) note that adopting irrigation efficiency for economic gain is a settler-colonial standard and ignores the values of social efficiency that inform Indigenous irrigation practices, with examples from the Andes. Similar characterizations have been made regarding irrigation modernization in Spain (Oyonarte et al., 2022) and the southwestern United States (Hicks and Peña, 2003; Fernald et al., 2007). Ultimately, the framing that irrigators pursue irrigation efficiency as part of their journey toward economic efficiency holds in highly productive agricultural regions like the Henrys Fork.
In the Henrys Fork watershed, farm-scale decisions to increase irrigation efficiency caused surface-water diversion to decrease by 23% between 1978 and 2000 then remain stable at reduced levels from 2001 to 2022 (Figure 4). We were unable to definitively identify the cause for the abrupt decline in 2001 with our methods. However, two factors may have contributed: drought and irrigation conversion on the Egin Bench. The year 2001 was a severe drought year in the Henrys Fork. State water managers have observed increases in on-farm irrigation efficiency in Idaho in drought years (Mathew Weaver 2023; personal communication, 18 May) and studies elsewhere document drought as a catalyst for increasing irrigation efficiency in the early 2000s (Schuck et al., 2005; Scott et al., 2014). Nonetheless, senior water users like those on the Egin Bench were almost always in priority for water allocation (U.S. Bureau of Reclamation and Idaho Water Resource Board, 2015) and still reduced their surface-water diversion as they converted to more efficient irrigation application (Table 2; Figure 3). The rapid rate of conversion on the Egin Bench from 1978 to 2000 coincides with the decrease in surface-water diversions in the watershed. Conversion on Egin Bench slowed after 2000 (Figure 3) for reasons unknown, coinciding with the stable surface-water diversions 2001–2022. Therefore, the dynamics of irrigation conversion on the Egin Bench may have also been a factor in the dynamics of surface-water diversion through time. Our statistical analysis confirmed a reduction in watershed-total diversion and provided strong evidence for temporal change in diversion even after accounting for the confounding effect of reduced unregulated flow identified within our correlation analysis (Table 3). Reduced diversion as a result of irrigation efficiency improvements have also been observed in other studies (e.g., Sando et al., 1988; Bigdeli Nalbandan et al., 2023).
As irrigation efficiency improved and diversion decreased in the Henrys Fork watershed, reach gains decreased by 299 Mm3. Elsewhere in the upper Snake River basin, reach gain decline was largely attributed to decreased surface return, but the potential for changes in groundwater use to affect reach gains was acknowledged (Olenichak, 1998). Although we did not specifically investigate groundwater use, groundwater pumping was ∼25% of total irrigation withdrawal in 2015, and the 299 Mm3 decrease we observed in reach gains was larger than the 200 Mm3 of total groundwater withdrawal from our study area in 2015 (Lovelace et al., 2020). Based on statewide data, we estimate that groundwater use for irrigation in our study area increased by ∼24 Mm3 between 1978 and 2022 (see Supplementary Material). Thus, we conclude that the decline in reach gains in 1978–2000 were from flood-to-sprinkler irrigation conversion. Effectively, then, reach gains prior to 2000 were irrigation return flows to the river. Our result aligns with other studies that have modeled 23%–77% declines in return flows following conversion to sprinkler or drip irrigation (Cai et al., 2003; Toloei, 2015; Hu et al., 2017; Malek et al., 2021).
Return flows are the combination of surface and groundwater returns to the river, where seepage from field application and canal conveyance contribute to groundwater returns specifically. Olenichak (1998) documented return flows were typically supplemented by surface return in river reaches downstream of the Henrys Fork watershed. However, based on field work done in the late 2000s, very little return flow occurs via surface return in the Henrys Fork (U.S. Bureau of Reclamation, 2012b). Our results suggest that return flows at least partially travel through shallow groundwater. The AICc analysis identified diversion decreasing from 1978 to 2000 before dropping abruptly in 2001, whereas reach gains continued to diminish more gradually through 2002 before stabilizing in 2003–2022. The 2-year lag between diversion and reach gain decline likely reflects attenuation in the groundwater system, further emphasizing the relationship between surface-water diversion and reach gains that is also demonstrated in our correlations (Table 3). A lag in streamflow response to groundwater recharge has been documented elsewhere in the Snake River basin (Miller et al., 2003) as well as in other systems (e.g., Kendy and Bredehoeft, 2006; Stoelzle et al., 2014). Given the increase in irrigation efficiency at the field scale, seepage from earthen canals is likely a major contributor in maintaining return flows at present. Thus, when considering a basin-scale shift in irrigation efficiency, it is important to assess the roles of soil, local geology, and conveyance seepage in both farm-scale decisions and the resulting basin-scale hydrology.
Critics of the effort to increase irrigation efficiency as a means for basin-scale water conservation specifically cite how these economically rational decisions at the farm-scale lead to higher consumptive water use and negate water conservation efforts (Ward and Pulido-Velazquez, 2008; Grafton et al., 2018). Overall, our analysis of streamflow data from 1978 to 2022 demonstrated no change in net basin export—the sum of consumptive use and water that exits the basin as groundwater flow to the ESPA. Our study did not include detailed groundwater data. Thus, we cannot quantify how consumptive use and groundwater stored in the ESPA individually contribute to net basin export. However, regional studies have documented a decline in ESPA storage and discharge from 1950 to present (Stewart-Maddox et al., 2018; Sukow, 2021)—suggesting a likely decrease in groundwater export from the watershed. If groundwater export in the Henrys Fork has declined, consumptive use would need to increase to maintain the average annual 1,046 Mm3 net basin export. Our documented wide-spread conversion to center-pivot sprinklers (Figure 3) demonstrate a mechanism for increased consumptive use within the watershed. Furthermore, the observed reduction of 11 m3/s in mid-summer reach gain is equivalent to previous scenario modeling predicting a 11.1 m3/s reach gain decline from 1980 to 2002 due to irrigation efficiency improvements (Contor et al., 2004). Consumptive use of irrigation water by crops in the study area was estimated at 350 Mm3 in 1980–2010 (U.S. Bureau of Reclamation, 2012b), around one-third of the total water exported from the watershed.
Thus, increases in irrigation efficiency in the Henrys Fork watershed may have increased consumptive use of surface water diversion and decreased return flows available to downstream users. The observed reduction of 11 m3/s in mid-summer reach gain is the same order of magnitude as a 2020 irrigation-season flow target of ∼10 m3/s in the lower Henrys Fork (Morrisett et al., 2023) and is approximately one-third of the 31 m3/s average mid-summer streamflow in the Henrys Fork at Rexburg for 2001–2022. Return flows can provide streamflow to downstream users (Simons et al., 2015; Owens et al., 2022), and irrigation systems may be managed with inherent assumptions of return flow reuse downstream (e.g., Boelens and Vos, 2012; Simons et al., 2020). Similar assumptions were made throughout the western United States until a 2007 Supreme Court case determined that the doctrine of recapture within prior appropriation does not require an irrigator to return unused water to its original source. Thus, irrigators are allowed to improve their irrigation efficiency and consumptive use as part of their original water right (MacDonnell, 2011). The loss of return flows has particular implications for downstream users, as they may have junior water rights and be especially sensitive to climate-induced water scarcity (Null and Prudencio, 2016). In the Henrys Fork watershed, the lower Teton River would be a losing reach without irrigation return flows (Apple, 2013). In mid-summer, when upstream users are diverting administrative storage water, the downstream-most water users on the lower Teton River have rights only to reach gains, and the river is managed so that the only physical water available to them are reach gains (Olenichak, 2020). Historically, irrigation return flows were likely a major source of water for lower Teton River irrigators, and return flow reduction has since diminished water availability for these downstream users—an issue that has been discussed numerous times by the local watershed council.
It is not apparent if the loss of irrigation return flows to the lower Henrys Fork watershed has impacted local aquatic ecosystems. Morrisett et al., 2023 did not identify a reduction in trout habitat for 1978–2021 that aligned with the declining reach gains observed in this study; the uniform flow-dependent habitat is consistent with our results that net diversion and streamflow have not changed despite decreased reach gains. However, another study has documented a shift in fish demographics that may be partially explained by thermal stress (Moore et al., 2016), due to a loss of cool groundwater inflow.
Irrigation return flow may be a beneficial climate adaptation tool in many types of systems. In the semi-arid western United States, reduced streamflow and warmer stream temperatures are expected with climate change (Ficklin et al., 2018). In irrigated watersheds, return flows can add resilience by mediating low streamflow and providing cool water refugia (Fernald and Guldan, 2006; Dzara et al., 2019; Van Kirk et al., 2020). Although increasing irrigation efficiency for aquatic ecosystem conservation was not a motivating factor for irrigation conversion in the Henrys Fork, our work provides an example for how increasing irrigation efficiency alone is not a successful tool for increasing streamflow for aquatic habitat. To best benefit aquatic ecosystems, managers and policymakers need to formally allocate water for environmental purposes (Batchelor et al., 2014; Pérez-Blanco et al., 2021; Anderegg et al., 2022). Otherwise, conserved water will continue to be allocated for human demands (Scott et al., 2014; Linstead, 2018). These ideas and methods are broadly applicable to other systems. For example, return flow reduction as a result of increased irrigation efficiency has made wetlands more vulnerable to change (Burke et al., 2004; Peck et al., 2004; Downard et al., 2014), diminished inland lake volume and habitat (Scott et al., 2014; Micklin, 2016; Parsinejad et al., 2022), and degraded delta ecosystems (Frisvold et al., 2018).
Options for recovering return flows in the lower Henrys Fork watershed include 1) conducting managed aquifer recharge and 2) maintaining and expanding flood irrigation for incidental recharge. In Idaho, managed aquifer recharge is appropriated through water rights administration and incidental recharge occurs incidental to standard irrigation operations (i.e., seepage via canal conveyance and flood irrigation). Within the scientific literature, agricultural managed aquifer recharge (Ag-MAR) generally references the practice of using irrigation infrastructure or fields for recharge (Levintal et al., 2023) and captures both incidental and managed aquifer recharge as defined by Idaho’s state water law.
Managed aquifer recharge is already being conducted in the watershed. In an effort to increase aquifer levels and spring discharge in the ESPA, the Idaho Water Resources Board recently invested over $1M USD to expand managed aquifer recharge infrastructure in the lower Henrys Fork (Patton, 2018). Managed aquifer recharge may only occur when its water rights are in priority and is thus conducted from November to March using existing irrigation infrastructure (i.e., canals) to route streamflow to the Egin Lakes recharge site—8 km from the river near the Egin Bench irrigation study area—for aquifer infiltration and percolation (Idaho Department of Water Resources, 1999). Groundwater models have shown that water recharged at Egin Lakes returns as base flow to the lower Henrys Fork in 3 months (Contor et al., 2009), and if effectively timed, recharge can supplement summer low-flow periods when irrigation diversion peaks (Idaho Department of Water Resources, 1999; Van Kirk et al., 2020).
Achieving recharge incidental to standard irrigation operations will be challenging. Given the economic inertia of irrigation development in the Henrys Fork watershed, it is unlikely irrigators will revert from center-pivot sprinkler application to flood irrigation. Flood irrigation continues to be conducted on some parcels within the Lower Watershed, as evidenced by our 2021 ground-truthing, and has potential to continue given relationship building and proper incentives. Implementing incidental recharge in the Henrys Fork at a scale meaningful for irrigation return flows will require irrigator buy-in.
To incentivize and collaborate with irrigators appropriately, managers and water conservation interests must understand and consider irrigator values and limitations, as well as the impact of climate change and market forces on agricultural production (Ricart and Clarimont, 2016). Our interviews suggested that irrigators who continue to flood irrigate may do so due to financial and land limitations, but also because of their values towards maintaining wildlife habitat and groundwater springs. Ag-MAR needs and constraints are inherently local (Levintal et al., 2023). Honing in on land parcels suitable for Ag-MAR using GIS-based multi-criteria decision analysis (Kazakis, 2018; Sallwey et al., 2019) or computer modeling (Behroozmand et al., 2019) and characterizing irrigator values, constraints, and enablers can identify potentially effective partnerships (Alonso et al., 2019; Sketch et al., 2020; Zuo et al., 2022). Given the economic incentives for increasing on-farm irrigation efficiency highlighted in our interviews, as well as the subsidies in place locally and globally to facilitate adoption of more efficient irrigation, economic incentives will likely be a key factor for implementing incidental recharge. Once the legal and regulatory framework are in place to allow Ag-MAR, economic incentives to conduct Ag-MAR include compensating irrigators for taking on risk through their participation (Dahlke et al., 2018; Gailey et al., 2019), access to the groundwater recharged via property rights or credit (Niswonger et al., 2017; Hanak, 2018; Reznik et al., 2022), and rebates on subsequent groundwater pumping fees (Miller et al., 2021). Lastly, social capital, civic engagement, and capacity building are important for developing cooperative partnerships with irrigators (Lubell, 2004; Alston and Whittenbury, 2011; Sketch et al., 2020) and should be a valued part of Ag-MAR pursuits.
However, the ability to conduct Ag-MAR may be limited by agricultural land availability as irrigators decide to sell their land for residential, urban, and commercial development. Conversion of agricultural land is increasing in the Henrys Fork watershed and is shifting water use to groundwater resources (Baker et al., 2014). Generally, increased groundwater withdrawal combined with decreased groundwater recharge further contribute to diminishing groundwater contributions to the river (Venn et al., 2004; Essaid and Caldwell, 2017). Furthermore, urban encroachment on surface water canals can disrupt their function and hinder local irrigation operations (Hicks and Peña, 2003; Cox and Ross, 2011). Mixed residential and agricultural neighborhoods may also limit the ability of an irrigator to flood irrigate due to the proximity of residential basements (Deng and Bailey, 2020). Thus, residential development within an irrigated landscape can indirectly limit groundwater recharge activities.
Hence, managers and water conservation interests must also be aware of how agricultural land development and conservation play a role in the hydrologic cycle. Li, Endter-Wada and Li (2019) analyzed agricultural land conversion in Utah (United States) and noted that irrigable lands are more likely to be developed due to their proximity to urban areas and flatter terrain, compared to non-irrigated agricultural land that is more rural and on hill slopes. In a nearby Idaho watershed, Huang et al. (2019) found that conservation of agricultural land with riparian buffers may indeed reduce water scarcity, nutrient loading, and sediment export under climate change.
Ag-MAR is not a panacea, however. Water rights priority, irrigator interests, and continued development of irrigable agricultural land may limit its implementation and effectiveness. Therefore, it is imperative water managers and policymakers consider how farm-scale decisions can compound to have watershed-scale hydrologic impacts. Ricart and Clarimont (2016) offer an approach for mapping stakeholder priorities in changing irrigation systems. Lankford et al. (2020) propose the ‘irrigation efficiency matrix’ framework in which multiple spatial scales and social dimensions are classified for consideration to prevent unintended consequences of changing irrigation landscapes. Numerous scholars urge accounting for basin-scale hydrology in water conservation policy, rather than focusing on maximizing on-farm irrigation efficiency alone (Huffaker, 2008; Ward and Pulido-Velazquez, 2008; Lankford et al., 2020).
Increasing irrigation efficiency is an economically attractive option to irrigators in the semi-arid Henrys Fork region to reduce water lost to seepage and improve their agricultural production under water scarcity. However, watershed-wide adoption of more efficient irrigation application has increased consumptive use and reduced return flows. Loss of cool groundwater return flow may exacerbate the effects of climate change on summer streamflow and stream temperature—and Ag-MAR may be a tool to mitigate such loss. Here, we demonstrate an interdisciplinary approach that combines interviews, geospatial analysis, and statistical streamflow analysis to identify the historical motivations and progression of irrigation conversion through time and investigate the watershed-scale response to these farm-scale decisions. Moving forward, when considering water conservation strategies within an irrigated watershed, we recommend managers and policymakers assess current and possible interactions between irrigation efficiency and irrigator behavior, as well as irrigation efficiency and basin-scale hydrology to identify and anticipate potential hydrologic outcomes. A holistic approach that seeks to understand how irrigator priorities contribute to landscape-scale changes in hydrologic regimes will allow watershed management to adapt to water scarcity accordingly.
The datasets generated and analyzed for this study can be found on Hydroshare in the following repository: https://www.hydroshare.org/resource/5bf4e21aa33d4e7b8a65f0791396d30c/.
The study involving humans was approved by Utah State University Institutional Review Board under Protocol #12846. The study was conducted in accordance with the local legislation and institutional requirements. The ethics committee/institutional review board waived the requirement of written informed consent for participation because interviews were not recorded and interview notes were collected with unique participant IDs (rather than names). Instead, interviewers read a Letter of Information to the participant that included the minimum elements for exempt applications and asked participants if they agreed and wished to continue.
Conceptualization: CM, RVK; methodology: RVK, CM, LB, AH, and CP; formal analysis: RVK, CM, LB, AH, and CP; investigation: RVK, CM, LB, AH, and CP; resources: RVK and SN; data curation: RVK, CM, LB, AH, and CP; writing—original draft preparation: CM; writing—review and editing: RVK, SN, LB, AH, and CP; visualization: CM, RVK, AH, and LB; supervision: RVK, CM, SN; project administration: RVK; funding acquisition: RVK and CM. As part of 10-week internships with the Henry’s Fork Foundation: LB specifically contributed to the hydrologic time-series analysis; AH the geospatial analysis; CP the irrigator interviews. All authors contributed to the article and approved the submitted version.
The funding was provided by a U.S. Bureau of Reclamation WaterSMART Applied Science Grant (R21AP10036) and individual donations to the Henry’s Fork Foundation. CM received support from the National Science Foundation grant no. 1633756 and SN received funding from a USDA National Institute for Food and Agriculture grant on Secure Water Future. LB was supported by an internship fund at St. Lawrence University. AH and CP were supported by an internship program at the Henry’s Fork Foundation tied to its Farms and Fish Program. Publishing fees were subsidized by the Utah State University Open Access Funding Initiative.
All interviews were conducted under Protocol #12846 approved by the Utah State University Institutional Review Board. We thank the Institutional Review Board at Utah State University for their feedback on our research protocol, Daniel Wilcox for his guidance in developing and executing our interview protocol, Sarah Newcomb for processing the gridMET precipitation and ET data as well as the Landsat imagery for North Fremont, Gregory Goodrum and Eryn Turney for their ArcGIS assistance, and the irrigators who shared their time, perspective, and networks during a busy irrigation season.
The authors declare that the research was conducted in the absence of any commercial or financial relationships that could be construed as a potential conflict of interest.
All claims expressed in this article are solely those of the authors and do not necessarily represent those of their affiliated organizations, or those of the publisher, the editors and the reviewers. Any product that may be evaluated in this article, or claim that may be made by its manufacturer, is not guaranteed or endorsed by the publisher.
The Supplementary Material for this article can be found online at: https://www.frontiersin.org/articles/10.3389/fenvs.2023.1188139/full#supplementary-material
Abatzoglou, J. T. (2013). Development of gridded surface meteorological data for ecological applications and modelling. Int. J. Climatol. 33 (1), 121–131. doi:10.1002/joc.3413
Alger, M., Lane, B. A., and Neilson, B. T. (2021). Combined influences of irrigation diversions and associated subsurface return flows on river temperature in a semi-arid region. Hydrol. Process. 35 (8), e14283. doi:10.1002/hyp.14283
Alonso, A., Feltz, N., Gaspart, F., Sbaa, M., and Vanclooster, M. (2019). Comparative assessment of irrigation systems’ performance: case study in the Triffa agricultural district, NE Morocco. Agric. Water Manag. 212, 338–348. doi:10.1016/j.agwat.2018.08.033
Alston, M., and Whittenbury, K. (2011). Climate change and water policy in Australia’s irrigation areas: a lost opportunity for a partnership model of governance. Environ. Polit. 20 (6), 899–917. doi:10.1080/09644016.2011.617175
Anderegg, W., Buttars, C., Ferry, J., Gochnour, N., Shelley, K., Steed, B., et al. (2022). Great Salt Lake policy assessment: a synthesized resource document for the 2023 General Legislative Session. The Great Salt Lake Strike Team.
Anderson, R. (2022). Efficiency in the Upper Deschutes Basin: understanding the hydrosocial implications of irrigation canal piping. Portland: M.S. Portland State University. Available at: https://www.proquest.com/docview/2694927145/abstract/A8545ED4CC1F487CPQ/1 (Accessed February 28, 2023).
Apple, B. D. (2013). Predicting groundwater effects due to changing land practices in the intermountain west. Arcata: M.S. Humboldt State University. doi:10.1145/3132847.3132886
Armstrong, A., and Jackson-Smith, D. (2017). Connections and collaborations of local water management organizations of Utah. Soc. Nat. Resour. 30 (11), 1343–1357. doi:10.1080/08941920.2017.1347972
Attride-Stirling, J. (2001). Thematic networks: an analytic tool for qualitative research. Qual. Res. 1 (3), 385–405. doi:10.1177/146879410100100307
Avello Fernández, L., Izaguirre Castellanos, E., Vidal Díaz, M. L., Martínez Laguardia, A. S., and Hernández Santana, L. (2018). Remote supervision and control based on wireless technology to operation of central pivot irrigation machine. Sist. Telemática 16 (44), 63–74. doi:10.18046/syt.v16i44.2788
Babin, N., Klier, C., and Singh, A. (2022). Understanding and promoting adoption of irrigation efficiency practices in Paso Robles, California vineyards: the importance of farm typology and grower sustainability networks. Curr. Res. Environ. Sustain. 4, 100143. doi:10.1016/j.crsust.2022.100143
Baker, J. M., Everett, Y., Liegel, L., and Van Kirk, R. (2014). Patterns of irrigated agricultural land conversion in a western U.S. watershed: implications for landscape-level water management and land-use planning. Soc. Nat. Resour. 27 (11), 1145–1160. doi:10.1080/08941920.2014.918231
Barnhill, J. V., Hill, R. W., and Patterson, R. (2009). Small acreage irrigation system selection. Logan: Utah State University Cooperative Extension. Available at: https://digitalcommons.usu.edu/cgi/viewcontent.cgi?article=2387&context=extension_curall.
Batchelor, C., Reddy, V. R., Linstead, C., Dhar, M., Roy, S., and May, R. (2014). Do water-saving technologies improve environmental flows? J. Hydrology 518, 140–149. doi:10.1016/j.jhydrol.2013.11.063
Bayrd, G. B. (2006). The influences of geology and water management on hydrology and fluvial geomorphology in the Henry’s Fork of the Snake River, Eastern Idaho and Western Wyoming. Pocatello: M.S. Idaho State University.
Behroozmand, A. A., Auken, E., and Knight, R. (2019). Assessment of managed aquifer recharge sites using a new geophysical imaging method. Vadose Zone J. 18 (1), 1–13. doi:10.2136/vzj2018.10.0184
Benouniche, M., Kuper, M., Hammani, A., and Boesveld, H. (2014). Making the user visible: analysing irrigation practices and farmers’ logic to explain actual drip irrigation performance. Irrigation Sci. 32 (6), 405–420. doi:10.1007/s00271-014-0438-0
Bigdeli Nalbandan, R., Delavar, M., Abbasi, H., and Zaghiyan, M. R. (2023). Model-based water footprint accounting framework to evaluate new water management policies. J. Clean. Prod. 382, 135220. doi:10.1016/j.jclepro.2022.135220
Birks, M., Chapman, Y., and Francis, K. (2008). Memoing in qualitative research: probing data and processes. J. Res. Nurs. 13 (1), 68–75. doi:10.1177/1744987107081254
Bjorneberg, D. L., and Sojka, R. E. (2005). ‘Irrigation methods’. United States Department of Agriculture. Available at: https://eprints.nwisrl.ars.usda.gov/id/eprint/822/1/1136.pdf.
Boelens, R., and Vos, J. (2012). The danger of naturalizing water policy concepts: water productivity and efficiency discourses from field irrigation to virtual water trade. Agric. Water Manag. 108, 16–26. doi:10.1016/j.agwat.2011.06.013
Brown, P. W. (2008). “Flood vs. pivot irrigation for forage crops: what are the advantages and disadvantages?,” In California alfalfa and forage symposium and western seed conference, San Diego, CA, USA.
Burke, S. M., Adams, R. M., and Wallender, W. W. (2004). Water banks and environmental water demands: case of the Klamath Project. Water Resour. Res. 40 (9). doi:10.1029/2003WR002832
Burnham, K. P., and Anderson, D. R. (2002). Model selection and multimodel inference. 2nd edn. New York, NY: Springer.
Burt, C. M., Clemmens, A. J., Strelkoff, T. S., Solomon, K. H., Bliesner, R. D., Hardy, L. A., et al. (1997). Irrigation performance measures: efficiency and uniformity. J. Irrigation Drainage Eng. 123(6), 423–442. doi:10.1061/(asce)0733-9437(1997)123:6(423)
Cai, X., Rosegrant, M. W., and Ringler, C. (2003). Physical and economic efficiency of water use in the river basin: implications for efficient water management. Water Resour. Res. 39 (1). doi:10.1029/2001WR000748
Cantor, A. (2017). Material, political, and biopolitical dimensions of “waste” in California water law. Antipode 49 (5), 1204–1222. doi:10.1111/anti.12314
Causapé, J., Quílez, D., and Aragüés, R. (2006). Irrigation efficiency and quality of irrigation return flows in the Ebro River Basin: an overview. Environ. Monit. Assess. 117 (1), 451–461. doi:10.1007/s10661-006-0763-8
Claeskens, G., and Hjort, N. L. (2008). Model selection and model averaging. Camridge: Cambridge University Press.
Contor, B. A. (2004). Delineation of sprinkler and gravity application systems, Eastern Snake Plain Aquifer model enhancement project scenario document DDW-022. Moscow: University of Idaho: Idaho Water Resource Research Institute. Technical Report 04-005.
Contor, B. A., and Taylor, R. G. (2013). Why improving irrigation efficiency increases total volume of consumptive use. Irrigation Drainage 62 (3), 273–280. doi:10.1002/ird.1717
Contor, B. A., Taylor, S. L., and Quinn, G. W. (2009). Monitoring of Egin, Idaho recharge experiment, Fall 2008. IWRRI technical completion report 200901.
Contor, B., Cosgrove, D., and Johnson, G. (2004). Snake River Plain Aquifer Model Scenario: hydrologic effects of changes in surface-water irrigation ‘No surface-water changes scenario’. Moscow, Idaho: University of Idaho. Idaho Water Resource Research Institute Technical Report 04-003.
Cox, M., and Ross, J. M. (2011). Robustness and vulnerability of community irrigation systems: the case of the Taos valley acequias. J. Environ. Econ. Manag. 61 (3), 254–266. doi:10.1016/j.jeem.2010.10.004
Dahlke, H. E., LaHue, G. T., Mautner, M. R. L., Murphy, N. P., Patterson, N. K., Waterhouse, H., et al. (2018). “Chapter eight - managed aquifer recharge as a tool to enhance sustainable groundwater management in California: examples from field and modeling studies,” in Advances in chemical pollution, environmental management and protection. Editors J. Friesen, and L. Rodríguez-Sinobas (Elsevier Advanced Tools for Integrated Water Resources Management), 215–275. doi:10.1016/bs.apmp.2018.07.003
Deng, C., and Bailey, R. T. (2020). Assessing causes and identifying solutions for high groundwater levels in a highly managed irrigated region. Agric. Water Manag. 240, 106329. doi:10.1016/j.agwat.2020.106329
Downard, R., Endter-Wada, J., and Kettenring, K. M. (2014). Adaptive wetland management in an uncertain and changing arid environment. Ecol. Soc. 19 (2), art23. doi:10.5751/ES-06412-190223
Dunham, J. B., Angermeier, P. L., Crausbay, S. D., Cravens, A. E., Gosnell, H., McEvoy, J., et al. (2018). Rivers are social–ecological systems: time to integrate human dimensions into riverscape ecology and management. Wiley Interdiscip. Rev. Water 5 (4), e1291. doi:10.1002/wat2.1291
Dzara, J. R., Neilson, B. T., and Null, S. E. (2019). Quantifying thermal refugia connectivity by combining temperature modeling, distributed temperature sensing, and thermal infrared imaging. Hydrology Earth Syst. Sci. 23 (7), 2965–2982. doi:10.5194/hess-23-2965-2019
English, M. (1990). Deficit irrigation. I: analytical framework. J. Irrigation Drainage Eng. 116 (3), 399–412. doi:10.1061/(ASCE)0733-9437(1990)116:3(399)
Essaid, H. I., and Caldwell, R. R. (2017). Evaluating the impact of irrigation on surface water – groundwater interaction and stream temperature in an agricultural watershed. Sci. Total Environ. 599 (600), 581–596. doi:10.1016/J.SCITOTENV.2017.04.205
Ferencz, S., and Tidwell, V. (2022). Physical controls on irrigation return flow contributions to stream flow in irrigated alluvial valleys. Front. Water 4. doi:10.3389/frwa.2022.828099
Fernald, A. G., Baker, T. T., and Guldan, S. J. (2007). Hydrologic, riparian, and agroecosystem functions of traditional acequia irrigation systems. J. Sustain. Agric. 30 (2), 147–171. doi:10.1300/J064v30n02_13
Fernald, A. G., and Guldan, S. J. (2006). Surface water–groundwater interactions between irrigation ditches, alluvial aquifers, and streams. Rev. Fish. Sci. 14 (1–2), 79–89. doi:10.1080/10641260500341320
Fernald, A., Guldan, S., Boykin, K., Cibils, A., Gonzales, M., Hurd, B., et al. (2015). Linked hydrologic and social systems that support resilience of traditional irrigation communities. Hydrol. Earth Syst. Sci. 19, 293–307. doi:10.5194/hess-19-293-2015
Ficklin, D. L., Abatzoglou, J. T., Robeson, S. M., Null, S. E., and Knouft, J. H. (2018). Natural and managed watersheds show similar responses to recent climate change. Proc. Natl. Acad. Sci. 115 (34), 8553–8557. doi:10.1073/pnas.1801026115
Frisvold, G., Sanchez, C., Gollehon, N., Megdal, S., and Brown, P. (2018). Evaluating gravity-flow irrigation with lessons from Yuma, Arizona, USA. Sustainability 10 (5), 1548. doi:10.3390/su10051548
Gailey, R. M., Fogg, G. E., Lund, J. R., and Medellín-Azuara, J. (2019). Maximizing on-farm groundwater recharge with surface reservoir releases: a planning approach and case study in California, USA. Hydrogeology J. 27 (4), 1183–1206. doi:10.1007/s10040-019-01936-x
Grafton, R. Q., Williams, J., Perry, C. J., Molle, F., Ringler, C., Steduto, P., et al. (2018). The paradox of irrigation efficiency. Science 361 (6404), 748–750. doi:10.1126/science.aat9314
Graveline, N. (2016). Economic calibrated models for water allocation in agricultural production: a review. Environ. Model. Softw. 81, 12–25. doi:10.1016/j.envsoft.2016.03.004
Hamidov, A., Kasymov, U., Djumaboev, K., and Paul, C. (2022). Rebound effects in irrigated agriculture in Uzbekistan: A stakeholder-based assessment. Sustainability 14 (14), 8375. doi:10.3390/su14148375
Hanak, E. (2018). Replenishing groundwater in the San Joaquin Valley. San Francisco: Public Policy Institute of California.
Hicks, G. A., and Peña, D. G. (2003). Community acequias in Colorado’s Rio Culebra watershed: a customary commons in the domain of prior appropriation. Univ. Colo. Law Rev. 74 (2), 387–486.
Hipke, W., Thomas, P., and Stewart-Maddox, N. (2022). Idaho’s Eastern Snake Plain Aquifer managed aquifer recharge program. Groundwater 60 (5), 648–654. doi:10.1111/gwat.13214
Hu, Q., Yang, Y., Han, S., Yang, Y., Ai, Z., Wang, J., et al. (2017). Identifying changes in irrigation return flow with gradually intensified water-saving technology using HYDRUS for regional water resources management. Agric. Water Manag. 194, 33–47. doi:10.1016/j.agwat.2017.08.023
Huang, L., Liao, F. H., Lohse, K. A., Larson, D. M., Fragkias, M., Lybecker, D. L., et al. (2019). Land conservation can mitigate freshwater ecosystem services degradation due to climate change in a semiarid catchment: the case of the Portneuf River catchment, Idaho, USA. Sci. Total Environ. 651, 1796–1809. doi:10.1016/j.scitotenv.2018.09.260
Huffaker, R. (2008). Conservation potential of agricultural water conservation subsidies. Water Resour. Res. 44 (7). doi:10.1029/2007WR006183
Idaho Department of Water Resources (1999). Feasibility of large-scale managed recharge of the Eastern Snake. Boise, ID: In cooperation with U.S. Bureau of Reclamation and Navigant Consulting, Inc.
Idaho Water Resources Board (2009). Eastern Snake Plain Aquifer. Boise: Comprehensive Aquifer Management Plan, 31.
Jensen, M. E. (2007). Beyond irrigation efficiency. Irrigation Sci. 25 (3), 233–245. doi:10.1007/s00271-007-0060-5
Jordan, C., Donoso, G., and Speelman, S. (2023). Irrigation subsidy policy in Chile: lessons from the allocation, uneven distribution and water resources implications. Int. J. Water Resour. Dev. 39 (1), 133–154. doi:10.1080/07900627.2021.1965964
Kazakis, N. (2018). Delineation of suitable zones for the application of managed aquifer recharge (MAR) in coastal aquifers using quantitative parameters and the analytical hierarchy process. Water 10 (6), 804. doi:10.3390/w10060804
Kendy, E., and Bredehoeft, J. D. (2006). Transient effects of groundwater pumping and surface-water-irrigation returns on streamflow. Water Resour. Res. 42 (8). doi:10.1029/2005WR004792
King, B. A., and Kincaid, D. C. (1997). Optimal performance from center pivot sprinkler systems. BUL 797. Moscow, Idaho: Cooperative Extension System-Agricultural Experiment Station, Univesity of Idaho. Available at: https://www.lib.uidaho.edu/digital/uiext/items/uiext24736.html.
Knox, J. W., Kay, M. G., and Weatherhead, E. K. (2012). Water regulation, crop production, and agricultural water management—understanding farmer perspectives on irrigation efficiency. Agric. Water Manag. 108, 3–8. doi:10.1016/j.agwat.2011.06.007
Koech, R., Haase, M., Grima, B., and Taylor, B. (2021). Barriers and measures to improve adoption of irrigation technologies: a case study from the bundaberg region in queensland, Australia. Irrigation Drainage 70 (4), 909–923. doi:10.1002/ird.2583
Lam, W. F. (2004). “Institutional evolution and social-ecological resilience: a study of irrigation institutions in taiwan,” in Workshop in political theory and policy analysis.
Lankford, B., Closas, A., Dalton, J., López Gunn, E., Hess, T., Knox, J. W., et al. (2020). A scale-based framework to understand the promises, pitfalls and paradoxes of irrigation efficiency to meet major water challenges. Glob. Environ. Change 65, 102182. doi:10.1016/j.gloenvcha.2020.102182
Lankford, B. (2012). Fictions, fractions, factorials and fractures; on the framing of irrigation efficiency. Agric. Water Manag. 108, 27–38. doi:10.1016/j.agwat.2011.08.010
Lecina, S., Isidoro, D., Playán, E., and Aragüés, R. (2010). Irrigation modernization and water conservation in Spain: the case of Riegos del Alto Aragón. Agric. Water Manag. 97 (10), 1663–1675. doi:10.1016/j.agwat.2010.05.023
Levidow, L., Zaccaria, D., Maia, R., Vivas, E., Todorovic, M., and Scardigno, A. (2014). Improving water-efficient irrigation: prospects and difficulties of innovative practices. Agric. Water Manag. 146, 84–94. doi:10.1016/j.agwat.2014.07.012
Levintal, E., Kniffin, M. L., Ganot, Y., Marwaha, N., Murphy, N. P., and Dahlke, H. E. (2023). Agricultural managed aquifer recharge (Ag-MAR)—a method for sustainable groundwater management: a review. Crit. Rev. Environ. Sci. Technol. 53 (3), 291–314. doi:10.1080/10643389.2022.2050160
Li, E., Endter-Wada, J., and Li, S. (2019). Dynamics of Utah’s agricultural landscapes in response to urbanization: a comparison between irrigated and non-irrigated agricultural lands. Appl. Geogr. 105, 58–72. doi:10.1016/j.apgeog.2019.02.006
Linstead, C. (2018). The contribution of improvements in irrigation efficiency to environmental flows. Front. Environ. Sci. 6. doi:10.3389/fenvs.2018.00048
Lonsdale, W. R., Cross, W. F., Dalby, C. E., Meloy, S. E., and Schwend, A. C. (2020). Evaluating irrigation efficiency: toward a sustainable water future for Montana. Technical Report. The Montana University System Water Center. Available at: https://scholarworks.montana.edu/xmlui/handle/1/16067 (Accessed: January 18, 2023).
Lovelace, J. K., Nielsen, M. G., Read, A. L., and Murphy, C. J. (2020). Estimated groundwater withdrawals from principal aquifers in the United States--County level data for 2015. Virginia: U.S. Geological Survey. doi:10.5066/P9EI0KMR
Lubell, M. (2004). Collaborative watershed management: a view from the grassroots. Policy Stud. J. 32 (3), 341–361. doi:10.1111/j.1541-0072.2004.00069.x
MacDonnell, L. J. (2011). Montana v. Wyoming: sprinklers, irrigation water use efficiency and the doctrine of recapture perspectives on Montana v. Wyoming. Gold. Gate Univ. Environ. Law J. 5 (2), 265–296.
Malek, K., Adam, J., Yoder, J., Givens, J., Stockle, C., Brady, M., et al. (2021). Impacts of irrigation efficiency on water-dependent sectors are heavily controlled by region-specific institutions and infrastructures. J. Environ. Manag. 300, 113731. doi:10.1016/j.jenvman.2021.113731
Maupin, M. A., Kenny, J. F., Hutson, S. S., Lovelace, J. K., Barber, N. L., and Linsey, K. S. (2014). Estimated use of water in the United States in 2010. Circular 1405. Virginia: U.S. Geological Survey. doi:10.3133/cir1405
Micklin, P. (2016). The future Aral Sea: hope and despair. Environ. Earth Sci. 75 (9), 844. doi:10.1007/s12665-016-5614-5
Miller, K., Fisher, A. T., and Kiparsky, M. (2021). Incentivizing groundwater recharge in the Pajaro Valley through recharge net metering (ReNeM). Case Stud. Environ. 5 (1), 1222393. doi:10.1525/cse.2021.1222393
Miller, S. A., Johnson, G. S., Cosgrove, D. M., and Larson, R. (2003). Regional scale modeling of surface and ground water interaction in the Snake River Basin. J. Am. Water Resour. Assoc. 39 (3), 517–528. doi:10.1111/j.1752-1688.2003.tb03673.x
Molle, F., and Tanouti, O. (2017). Squaring the circle: agricultural intensification vs. water conservation in Morocco. Agric. Water Manag. 192, 170–179. doi:10.1016/j.agwat.2017.07.009
Moore, V. K., Flinders, J., Keen, D., High, B., and Garren, D. (2016). Fishery management annual report, Upper Snake region 2014, report No. 16-108. Boise, Idaho: Idaho Department of Fish and Game.
Morrisett, C. N., Van Kirk, R. W., and Null, S. E. (2023). Assessing downstream aquatic habitat availability relative to headwater reservoir management in the Henrys Fork Snake River. River Research and Applications.
Neibling, H. (1997). Irrigation systems for Idaho agriculture. CIS 1055. Moscow, Idaho: Cooperative Extension System-Agricultural Experiment Station, University of Idaho.
Nhiwatiwa, T., Dalu, T., and Brendonck, L. (2017). Impact of irrigation based sugarcane cultivation on the Chiredzi and Runde Rivers quality, Zimbabwe. Sci. Total Environ. 587–588, 316–325. doi:10.1016/j.scitotenv.2017.02.155
Niswonger, R. G., Morway, E. D., Triana, E., and Huntington, J. L. (2017). Managed aquifer recharge through off-season irrigation in agricultural regions. Water Resour. Res. 53 (8), 6970–6992. doi:10.1002/2017WR020458
Null, S. E., and Prudencio, L. (2016). Climate change effects on water allocations with season dependent water rights. Sci. Total Environ. 571, 943–954. doi:10.1016/J.SCITOTENV.2016.07.081
Ochoa, C. G., A. G. Fernald, , S. J. Guldan, , and M. K. Shukla, (2007). Deep percolation and its effects on shallow groundwater level rise following flood irrigation. Trans. ASABE 50 (1), 73–81. doi:10.13031/2013.22413
Olenichak, T. (1998). Changes to the Snake River Blackfoot-to-Milner reach gains. Idaho Falls: Idaho Water District 01.
Olenichak, T. (2020). Concepts, practices, and procedures used to distribute water within water district #1: Upper Snake River Basin, Idaho. Idaho Falls: Idaho Department of Water Resources Water District 01.
Owens, K., Carmody, E., Grafton, Q., O'Donnell, E., Wheeler, S., Godden, L., et al. (2022). Delivering global water security: embedding water justice as a response to increased irrigation efficiency. WIREs Water 9, e1608. doi:10.1002/wat2.1608
Oyonarte, N. A., Gómez-Macpherson, H., Martos-Rosillo, S., González-Ramón, A., and Mateos, L. (2022). Revisiting irrigation efficiency before restoring ancient irrigation canals in multi-functional, nature-based water systems. Agric. Syst. 203, 103513. doi:10.1016/j.agsy.2022.103513
Parsinejad, M., Rosenberg, D. E., Ghale, Y. A. G., Khazaei, B., Null, S. E., Raja, O., et al. (2022). 40-years of Lake Urmia restoration research: review, synthesis and next steps. Sci. Total Environ. 832, 155055. doi:10.1016/j.scitotenv.2022.155055
Patton, B. (2018). ESPA managed recharge program: legislative natural resources interim committee. Boise: Idaho Water Resource Board.
Peck, D. E., McLeod, D. M., Hewlett, J. P., and Lovvorn, J. R. (2004). Irrigation-dependent wetlands versus instream flow enhancement: economics of water transfers from agriculture to wildlife uses. Environ. Manag. 34 (6), 842–855. doi:10.1007/s00267-004-3085-z
Pérez-Blanco, C. D., Hrast-Essenfelder, A., and Perry, C. (2020). Irrigation technology and water conservation: a review of the theory and evidence. Rev. Environ. Econ. Policy 14 (2), 216–239. doi:10.1093/reep/reaa004
Pérez-Blanco, C. D., Loch, A., Ward, F., Perry, C., and Adamson, D. (2021). Agricultural water saving through technologies: a zombie idea. Environ. Res. Lett. 16 (11), 114032. doi:10.1088/1748-9326/ac2fe0
Qureshi, M. E., Grafton, R. Q., Kirby, M., and Hanjra, M. A. (2011). Understanding irrigation water use efficiency at different scales for better policy reform: a case study of the Murray-Darling Basin, Australia. Water Policy 13 (1), 1–17. doi:10.2166/wp.2010.063
R Core Team (2022). R: a language and environment for statistical computing. Available at: https://www.r-project.org/(Accessed: April 14, 2022).
Reznik, A., Dinar, A., Bresney, S., Forni, L., Joyce, B., Wallander, S., et al. (2022). Institutions and the economic efficiency of managed aquifer recharge as a mitigation strategy against drought impacts on irrigated agriculture in California. Water Resour. Res. 58 (6), e2021WR031261. doi:10.1029/2021WR031261
Ricart, S., and Clarimont, S. (2016). Modelling the links between irrigation, ecosystem services and rural development in pursuit of social legitimacy: results from a territorial analysis of the Neste System (Hautes-Pyrénées, France). J. Rural Stud. 43, 1–12. doi:10.1016/j.jrurstud.2015.09.012
Richter, B. D., Brown, J. D., DiBenedetto, R., Gorsky, A., Keenan, E., Madray, C., et al. (2017). Opportunities for saving and reallocating agricultural water to alleviate water scarcity. Water Policy 19 (5), 886–907. doi:10.2166/wp.2017.143
Saldana, J. (2016). in The coding manual for qualitative researchers. Editor J. Seaman (London: SAGE Publications LTD).
Sallwey, J., Bonilla Valverde, J. P., Vásquez López, F., Junghanns, R., and Stefan, C. (2019). Suitability maps for managed aquifer recharge: a review of multi-criteria decision analysis studies. Environ. Rev. 27 (2), 138–150. doi:10.1139/er-2018-0069
Sanchis-Ibor, C., Ortega-Reig, M., Guillem-García, A., Carricondo, J. M., Manzano-Juárez, J., García-Mollá, M., et al. (2021). Irrigation post-modernization. Farmers envisioning irrigation policy in the region of Valencia (Spain). Agriculture 11 (4), 317. doi:10.3390/agriculture11040317
Sando, S. K., Borrelli, J., and Brosz, D. J. (1988). Hydrologic impacts of improved irrigation efficiencies. J. Irrigation Drainage Eng. 114 (2), 334–342. doi:10.1061/(ASCE)0733-9437(1988)114:2(334)
Schirmer, D. J. (2017). Water Reform: socio-economic effects of investment in water infrastructure. Australia: Department of Agriculture and Water Resources. Available at: https://www.agriculture.gov.au/sites/default/files/sitecollectiondocuments/water/rws-report-2016.pdf.
Schuck, E. C., Frasier, W. M., Webb, R. S., Ellingson, L. J., and Umberger, W. J. (2005). Adoption of more technically efficient irrigation systems as a drought response. Int. J. Water Resour. Dev. 21 (4), 651–662. doi:10.1080/07900620500363321
Scott, C. A., Vicuña, S., Blanco-Gutiérrez, I., Meza, F., and Varela-Ortega, C. (2014). Irrigation efficiency and water-policy implications for river basin resilience. Hydrology Earth Syst. Sci. 18 (4), 1339–1348. doi:10.5194/hess-18-1339-2014
Simons, G. W. H., Bastiaanssen, W., Cheema, M., Ahmad, B., and Immerzeel, W. (2020). A novel method to quantify consumed fractions and non-consumptive use of irrigation water: application to the Indus Basin Irrigation System of Pakistan. Agric. Water Manag. 236, 106174. doi:10.1016/j.agwat.2020.106174
Simons, G. W. H., Bastiaanssen, W. G. M., Immerzeel, W. W., and Walter, G. (2015). Water reuse in river basins with multiple users: a literature review. J. Hydrology 522, 558–571. doi:10.1016/j.jhydrol.2015.01.016
Sketch, M., Dayer, A. A., and Metcalf, A. L. (2020). Western ranchers’ perspectives on enablers and constraints to flood irrigation. Rangel. Ecol. Manag. 73 (2), 285–296. doi:10.1016/j.rama.2019.12.003
Stoelzle, M., Stahl, K., Morhard, A., and Weiler, M. (2014). Streamflow sensitivity to drought scenarios in catchments with different geology. Geophys. Res. Lett. 41 (17), 6174–6183. doi:10.1002/2014GL061344
Sukow, Je. (2021). Model calibration report: Eastern Snake Plain Aquifer model version. Idaho Department of Water Resources. Available at: https://research.idwr.idaho.gov/files/projects/espam/browse/ESPAM22_Reports/ModelCalibrationRpt/ModelCalibration22_Final.pdf (Accessed: February 6, 2023).
Thompson, S. A. (1988). Patterns and trends in irrigation efficiency. JAWRA J. Am. Water Resour. Assoc. 24 (1), 57–63. doi:10.1111/j.1752-1688.1988.tb00880.x
Toloei, Z., Delavar, M., Morid, S., and Ahmadzadeh, H. (2015). Uncertainty analysis of pressurized irrigation impact on Urmia Lake basin outflow, case study: Zarinehrud Basin, Iran. Iran-Water Resour. Res. 11 (2), 135–145.
Trimmer, W., and Hansen, H. (1994). Irrigation scheduling. PNW0288. Washington State University Extension. Available at: http://irrigation.wsu.edu/Content/Fact-Sheets/pnw288.pdf.
United States Census Bureau (2022a). U.S. Census Bureau QuickFacts: Fremont County, Idaho. Available at: https://www.census.gov/quickfacts/fremontcountyidaho (Accessed: May 19, 2023).
United States Census Bureau (2022b). U.S. Census Bureau QuickFacts: Madison County, Idaho. Available at: https://www.census.gov/quickfacts/fact/table/madisoncountyidaho/PST045222 (Accessed May 23, 2023).
United States Census Bureau (2022c). U.S. Census Bureau QuickFacts: Rexburg City, Idaho. Available at: https://www.census.gov/quickfacts/fact/table/rexburgcityidaho/PST045222 (Accessed: May 23, 2023).
U.S. Bureau of Reclamation and Idaho Water Resource Board (2015). Henrys Fork Basin Study final report.
U.S. Bureau of Reclamation (2012a). Henry’s Fork Basin Study, Teton Dam storage alternative technial series No. PN-HFS-004. Boise, Idaho: U.S. Department of Interior.
U.S. Bureau of Reclamation (2012b). Henrys Fork watershed basin study water needs assessment technical series No. PN-HFS-001. Boise, Idaho: U.S. Department of Interior.
USDA National Agricultural Statistics Service (2017a). USDA/NASS Census of agriculture chapter 2, table 8, Fremont county Idaho. Available at: https://www.nass.usda.gov/Quick_Stats/CDQT/chapter/2/table/8/state/ID/county/043/year/2017 (Accessed: May 19, 2023).
USDA National Agricultural Statistics Service (2017b). USDA/NASS Census of agriculture chapter 2, table 8, Madison county Idaho. Available at: https://www.nass.usda.gov/Quick_Stats/CDQT/chapter/2/table/8/state/ID/county/065/year/2017 (Accessed: May 23, 2023).
Van Kirk, R. W., Contor, B. A., Morrisett, C. N., Null, S. E., and Loibman, A. S. (2020). Potential for managed aquifer recharge to enhance fish habitat in a regulated river. Water 12 (3), 673. doi:10.3390/w12030673
Van Kirk, R. W., Hoffner, B., Verbeten, A., and Yates, S. (2019). “New approaches to providing streamflow for fisheries in the American West: embracing prior appropriation and the marketplace,” in Multispecies and watershed approaches in freshwater conservation. Editors D. C. Dauwalter, T. W. Birdsong, and G. P. Garrett (Bethesda, MD: American Fisheries Society), 515–564.
Van Kirk, R. W., and Griffin, C. B. (1997). “Building a collaborative process for restoration: henrys Fork of Idaho and Wyoming,” in Watershed restoration: principles and practices (Bethesda, MD: American Fisheries Society), 253–276.
Van Kirk, R. W., Allison, K., Nowell, C., Oldemeyer, B., Contor, B., Nelson, F., et al. (2021). The economic value of fishing in the Henrys Fork watershed. Ashton: Henry’s Fork Foundation.
Venn, B. J., Johnson, D. W., and Pochop, L. O. (2004). Hydrologic impacts due to changes in conveyance and conversion from flood to sprinkler irrigation practices. J. Irrigation Drainage Eng. 130 (3), 192–200. doi:10.1061/(ASCE)0733-9437(2004)130:3(192)
Vonde, A. Y., Bromley, C. M., Carter, M. M., Courtney, A. L., Hamlin Nygard, S. E., Hensley, H. A., et al. (2016). Understanding the Snake River Basin adjudication. Ida. Law Rev. 52 (1), 53–222.
Walker, G. R., Horne, A. C., Wang, Q. J., and Rendell, R. (2021). Assessing the impact of irrigation efficiency projects on return flows in the south-eastern Murray-Darling basin, Australia. Water 13 (10), 1366. doi:10.3390/w13101366
Wang, J., Klein, K. K., Bjornlund, H., Zhang, L., and Zhang, W. (2015). Changing to more efficient irrigation technologies in southern Alberta (Canada): an empirical analysis. Water Int. 40 (7), 1040–1058. doi:10.1080/02508060.2015.1086257
Ward, F. A., and Pulido-Velazquez, M. (2008). Water conservation in irrigation can increase water use. Proc. Natl. Acad. Sci. U. S. A. 105 (47), 18215–18220. doi:10.1073/pnas.0805554105
Welsh, L. W., Endter-Wada, J., Downard, R., and Kettenring, K. M. (2013). Developing adaptive capacity to droughts the rationality of locality. Ecol. Soc. 18 (2), art7. doi:10.5751/ES-05484-180207
Wheeler, S. A., Carmody, E., Grafton, R., Kingsford, R., and Zuo, A. (2020). The rebound effect on water extraction from subsidising irrigation infrastructure in Australia. Resour. Conservation Recycl. 159, 104755. doi:10.1016/j.resconrec.2020.104755
Williams, A. P., Cook, E. R., Smerdon, J. E., Cook, B. I., Abatzoglou, J. T., Bolles, K., et al. (2020). Large contribution from anthropogenic warming to an emerging North American megadrought. Science 368 (6488), 314–318. doi:10.1126/science.aaz9600
Xu, H., and Song, J. (2022). Drivers of the irrigation water rebound effect: a case study of Hetao irrigation district in Yellow River basin, China. Agric. Water Manag. 266, 107567. doi:10.1016/j.agwat.2022.107567
York, R., and McGee, J. A. (2016). Understanding the Jevons paradox. Environ. Sociol. 2 (1), 77–87. doi:10.1080/23251042.2015.1106060
Zalidis, G., Dimitriadis, X., Antonopoulos, A., and Gerakis, A. (1997). Estimation of a network irrigation efficiency to cope with reduced water supply. Irrigation Drainage Syst. 11 (4), 337–345. doi:10.1023/A:1005872200842
Keywords: groundwater-surface water, aquifer recharge, Idaho, Eastern Snake Plain Aquifer, irrigation efficiency, return flow, reach gain
Citation: Morrisett CN, Van Kirk RW, Bernier LO, Holt AL, Perel CB and Null SE (2023) The irrigation efficiency trap: rational farm-scale decisions can lead to poor hydrologic outcomes at the basin scale. Front. Environ. Sci. 11:1188139. doi: 10.3389/fenvs.2023.1188139
Received: 17 March 2023; Accepted: 08 August 2023;
Published: 28 August 2023.
Edited by:
Saket Pande, Delft University of Technology, NetherlandsReviewed by:
Joao Paulo Moura, University of Trás-os-Montes and Alto Douro, PortugalCopyright © 2023 Morrisett, Van Kirk, Bernier, Holt, Perel and Null. This is an open-access article distributed under the terms of the Creative Commons Attribution License (CC BY). The use, distribution or reproduction in other forums is permitted, provided the original author(s) and the copyright owner(s) are credited and that the original publication in this journal is cited, in accordance with accepted academic practice. No use, distribution or reproduction is permitted which does not comply with these terms.
*Correspondence: Christina N. Morrisett, Y2hyaXN0aW5hLm1vcnJpc2V0dEB1c3UuZWR1
Disclaimer: All claims expressed in this article are solely those of the authors and do not necessarily represent those of their affiliated organizations, or those of the publisher, the editors and the reviewers. Any product that may be evaluated in this article or claim that may be made by its manufacturer is not guaranteed or endorsed by the publisher.
Research integrity at Frontiers
Learn more about the work of our research integrity team to safeguard the quality of each article we publish.