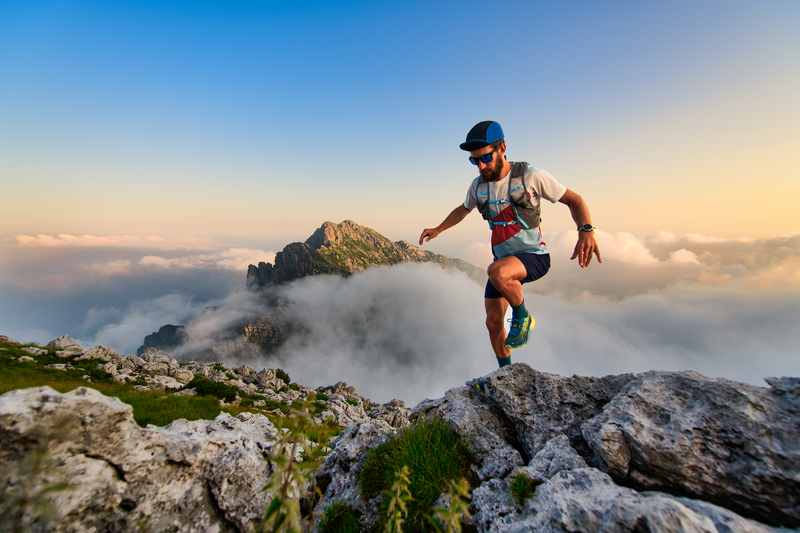
95% of researchers rate our articles as excellent or good
Learn more about the work of our research integrity team to safeguard the quality of each article we publish.
Find out more
REVIEW article
Front. Environ. Sci. , 13 April 2023
Sec. Freshwater Science
Volume 11 - 2023 | https://doi.org/10.3389/fenvs.2023.1162099
This article is part of the Research Topic River Rehabilitation in the Anthropocene View all 7 articles
Recently uplifted, highly erodible rocks, and recurrent high intensity storms, generate exceedingly high erosion and sedimentation rates in the East Coast Region (Tairāwhiti) of Aotearoa New Zealand. Despite the recent nature of the Anthropocene record in global terms (∼650 years since Māori arrival, 250 years of colonial impacts), human disturbance has profoundly altered evolutionary trajectories of river systems across the region. Here we document catchment-by-catchment variability in anthropogenic signature as geomorphic river stories for five catchments (Waiapu, Hikuwai, Waimatā, Waipaoa, Mōtū). We show how targeted, fit-for-purpose process-based rehabilitation programmes that manage at source and at scale are required to facilitate river recovery in each of these catchments. The largest rivers in the region, Waiapu and Waipaoa, comprise steep, highly dissected terrains that are subject to recurrent hillslope failures, including systemic shallow landslides, occasional deep-seated rotational slumps and earthflows. Localised sediment input from large (>10 ha) gully mass movement complexes overwhelms valley floors. Targeted revegetation programmes are required to reduce extreme sediment inputs from these sources. Although there are fewer gully complexes in the Hikuwai, multiple landslips supply vast volumes of fine-grained sediment that aggrade and are recurrently reworked along channel margins in lowland reaches. Waimatā has no gully complexes and a smaller number of landslips, but large areas are subject to sediment input from earthflows. The terrace-constrained flume-like nature of this system efficiently flushes materials ‘from the mountains to the sea’, recurrently reworking materials along channel banks in a similar manner to the lower Hikuwai. Systematic reforestation in the middle-upper catchment and revegetation of riparian corridors is required to reduce sedimentation rates in these catchments. In contrast, terraces buffer sediment delivery from hillslopes in the upper Mōtū catchment, where a bedrock gorge separates large sediment stores along upper reaches from the lower catchment. As reworking of valley floor sediments in response to bed incision and reworking (expansion) of channel margins is the primary contemporary sediment source in this system, bed control structures and revegetation of riparian corridors are required as part of targeted sediment management plans. We contend that geomorphic river stories provide a coherent platform for Anthropocene rehabilitation strategies that work with the character, behaviour and evolutionary trajectories of river systems. Although this generic lens can be applied anywhere in the world, we highlight particular meanings and implications in Aotearoa New Zealand where such thinking aligns directly with Māori values that respect the mana (authority), mauri (lifeforce) and ora (wellbeing) of each and every river.
River histories from centennial to millennial timescales reveal human impact via enhanced sedimentation and catchment sensitisation to climate (e.g., Knox, 1977; Brooks and Brierley, 1997; Wasson et al., 1998; Macklin and Lewin, 2003; Richardson et al., 2014). These understandings are not new (e.g., Marsh, 1864). Concepts of ‘Anthropocene rivers’ recognise the profound extent and scale to which rivers have been transformed by human activity beyond prior reference points (Brown et al., 2013; Large et al., 2022). In many instances, regime shift and state change engender persistent modification (cf. Thoms et al., 2020), as anthropogenic landscape forcing overwhelms the natural process regime (sensu Steffen et al., 2007). In many parts of the world this shift of regime and state reflects direct (planned, purposeful) anthropogenic modification of river systems. In the East Coast Region (Tairāwhiti) of Aotearoa New Zealand it reflects landscape response to deforestation (Fuller et al., 2015), as recognised by Henderson and Ongley (1920).
Global changes in land use play out in differing ways and with differing implications and consequences in different parts of the world, indeed, within any given region. Impacts upon flow/sediment regimes and the balance of impelling and resisting forces along river courses have profound implications for rehabilitation prospects (e.g., Brierley and Fryirs, 2009; Wohl et al., 2015; Brierley and Fryirs, 2016; Poeppl et al., 2020). Putting aside contested meanings of the Anthropocene, especially in socio-political and cultural terms (e.g., Castree, 2014; Malhi, 2017; Hayman et al., 2018), here we show how profound catchment-by-catchment variability in responses to anthropogenic disturbance presents differing prospects for process-based approaches to river rehabilitation for five river systems on the East Coast of Aotearoa New Zealand. Rehabilitation prospects reflect catchment-specific expressions of cumulative (layer upon layer) histories of landscape responses to anthropogenic disturbance. We contend that geomorphic river stories provide an insightful lens to unravel and communicate marked variability in river responses to systematic, rapid deforestation in an area that was already subject to exceedingly high rates of erosion and sedimentation prior to anthropogenic disturbance around 650 years ago (Wilmshurst et al., 1999). We define a river “story” as a succinct account of catchment-specific evolutionary trajectory, based on observed changes over time (decades, at least). By linking together evident causes (e.g., land-use change, altered hydrologic regime) with progressive and persistent documented change in river character (including responses such as floodplain sediment storage, channel widening, progressive channel degradation), we trust that river stories provide readers key insights into the active mechanisms, feedbacks, and/or cumulative effects that lead to this change. In turn, we hope such catchment-specific understandings empower local communities to engage with geomorphologically-informed remediation strategies that are fit-for-purpose.
Initial impacts of Polynesian settlement on river systems in Tairāwhiti did not result in regime shift or state change. At the time of European arrival, streams were clear-flowing and armoured with cobbles (Hamilton and Kelman, 1952). Deforestation following European colonisation to create pastoral landscapes occurred over a matter of decades from the late 1800s to early 1900s (Fuller and Rutherfurd, 2021). Land clearance exposed highly erodible terrains to a range of erosion processes, increasing erosion rates by an order of magnitude relative to estimated rates at the end of the Last Glacial Maximum (Marden et al., 2014). Here we focus on historic trajectories of five major rivers in this region where the anthropogenic signature is especially pronounced (Fuller et al., 2015): Waiapu, Hikuwai, Waimatā, Waipaoa and Mōtū (Figure 1).
FIGURE 1. The Raukumara Ranges are the backbone of Tairāwhiti (East Coast Region of Aotearoa New Zealand). Although the five study catchments (Waiapu (1758 km2), Hikuwai (560 km2), Waimatā (370 km2), Waipaoa (2,208 km2), Mōtū (1,373 km2)) have been subject to similar land use impacts associated with rapid deforestation, marked variability in recovery and rehabilitation prospects reflect significant differences in geomorphic responses to anthropogenic disturbance.
Anthropogenically-induced landscape transformation following European settlement created new river structures, functions, and trajectories in Tairāwhiti. Intriguingly, although removal of indigenous forest was fairly uniform across the region, contrasting channel responses reflect the range and diversity of river types across this relatively small region, often for rivers that share catchment boundaries and land-use histories. Erosion processes such as gully mass movement complexes or ‘badass’ gullies (Marden et al., 2018) evident in some of the study catchments do not conform to any existing gully model, generating profoundly disproportionate inputs into sediment cascades (Phillips, 2015; Fuller et al., 2020). Significant bed aggradation and pulsed sediment movement have ensued in the Waiapu and Waipaoa catchments. Although deforestation also profoundly altered adjacent systems such as the Hikuwai (Uawa), Waimatā and (to a lesser extent) the Mōtū Rivers, geomorphic response rates and extents to the same driver of change were less pronounced. Here we use long-standing principles in applied fluvial geomorphology outlined in Table 1 to characterise these variable river responses to anthropogenic disturbance as geomorphic river stories.
TABLE 1. Key attributes of geomorphic river stories to support rehabilitation programmes that apply nature-based solutions that work with the river (based on Truths of the Riverscape proposed by Brierley and Fryirs (2022)).
Some conceptualisations characterise Anthropocene rivers as homogenous and lacking in geomorphic complexity (De Boer et al., 2020). Such assertions typically reflect anthropogenically modified rivers that have been directly managed for ‘control’. Rivers in Tairāwhiti that we report upon in this study have been subjected to limited direct management. However, transformed boundary conditions following indirect responses to deforestation resulted in rivers that are anything but under ‘control’. We show how process-based understandings expressed as geomorphic river stories can support the design and implementation of catchment-specific river rehabilitation plans for a suite of dynamically adjusting but geomorphologically diverse river systems across Tairāwhiti.
Proactive, precautionary and cost-effective approaches to managing rivers in the Anthropocene ‘work with the river’, applying nature-based solutions that appropriately incorporate place-based understandings of the character, behaviour and evolutionary traits of each river system (Fryirs and Brierley, 2021; García et al., 2021; Brierley and Fryirs, 2022). Process-based measures that manage at source and at scale are key to effective rehabilitation (Beechie et al., 2010; Wheaton et al., 2019). Framed in relation to Anthropocene rehabilitation prospects, geomorphic river stories build upon meaningful description of catchment attributes to guide reliable explanation of the character, behaviour and evolution of the system (Brierley et al., 2021). Inevitably, the river itself is the key character in a geomorphic river story–what it looks like and how it adjusts in its journey from the mountains to the sea (Table 1). Temporal considerations sit alongside these spatial concerns—how the river evolves, its response to disturbance events, and associated magnitude-frequency relations. Geomorphic river stories are seldom linear (cause-and-effect) tales; rather, long periods of relative calm are disrupted by short chaotic intervals, often with surprising outcomes. Such is the inherent unpredictability and uncertainty of innately emergent systems.
Catchment-specific insights expressed through geomorphic river stories are key parts of coherent databases that are required to support and prioritise fit-for-purpose, appropriately targeted, strategic rehabilitation programmes (Table 1; cf., Fryirs et al., 2021). At a regional scale, understandings of controls upon within- and between-catchment variability in geomorphic characteristics appraise the role of boundary conditions set by geologic, climatic and anthropogenic factors, the imprint of past events (landscape, memory, legacy effects) and prospects for geomorphic river recovery (Fryirs and Brierley, 2016). This entails determination of what recovery looks like, how readily it can be achieved, and the sustainability of outcomes. Recognising explicitly that all rivers are important, and it often pays to work with the willing, these geomorphic insights enable cross-regional prioritisation of locally owned and enacted interventions in ways that address things that matter and are financially prudent.
Just as biophysical attributes of the landscapes and ecosystems of Aotearoa New Zealand are globally distinctive (see Gibbs, 2006), so too is the socio-cultural and political history and associated management and governance arrangements, especially attributes shaped by the Treaty of Waitangi (Te Tiriti o Waitangi), signed in 1840 (see Hikuroa et al., 2021; Knight, 2016; Parsons et al., 2021). These framings and the policy settings in which they are embedded create opportunities for novel approaches to rehabilitation in the Anthropocene in Aotearoa New Zealand. Such practices can build upon alignment of geomorphic understandings with principles of Mātauranga Māori (indigenous knowledge construction; Wilkinson et al., 2020). Rivers are conceptualized in Te Ao Māori (the Māori worldview) as the lifeblood of the land, wherein the ora (wellbeing) of the river—encapsulated by its mauri (life force)—is intertwined with the wellbeing of its people (Stewart-Harawira, 2020). For Māori, an awa (river) is not just a resource to be used, it is an interconnected, living system—a force to be lived with, reckoned with, and respected (te awa tupua) (Salmond, 2014). Explicitly, moves towards uptake and embedding of Te Mana o Te Wai in Aotearoa New Zealand’s 2020 National Policy Statement for Freshwater (Ministry for Environment, 2020) emphasise concerns for knowledges-in-place, whereby best available understandings support efforts to enhance the ora (wellbeing) of every waterbody (Te Aho, 2019). We contend that geomorphic river stories provide a helpful resource to support such endeavours.
The climate of Tairāwhiti is strongly maritime influenced with warm moist summers and cool wet winters. Rainfall gradients increase from the south to the north and from the coast inland (Figure 2). Tropical cyclones can occur from November to March (Austral summer December-February). Gisborne is subject to both Tropical and Antarctic weather systems with southeasterlies having the highest impact in the city as the low topography is unable to shield the area (Figure 2; Chappell, 2016). Short-duration, high-intensity rainfall events associated with cyclones, ex-tropical cyclones and sub-tropical depressions induce recurrent flooding and landslides, especially between March and May (Cook et al., 2022). During a storm in March 2022, 345 mm was recorded in 24 h at Te Puia, located towards the coast between the Hikuwai and Waiapu catchments. Cyclone Bola (March 1988) delivered 900 mm in 3 days in the Hikuwai catchment. Tairāwhiti catchments are vulnerable to geomorphic disturbance during these large storm events. Erosion during such events can be severe even under indigenous forest (e.g., Raparapaririki Stream in the Waiapu catchment; Tunnicliffe et al., 2018; see Parkner et al., 2007).
FIGURE 2. Average annual rainfall (mm yr-1) for Tairāwhiti (East Coast Region of Aotearoa New Zealand) from 1981–2020. Note the pronounced gradient from north to south, with much higher annual totals in the Waiapu Catchment relative to the Waimatā Catchment. However, these annual totals mask similarity in the prevalence of the geomorphic role of high intensity storms across the entire region.
Being located on the boundary of the converging Pacific and Indo-Australian lithospheric plates, tectonic uplift and frequent large magnitude earthquakes associated with the Hikurangi Subduction fault are important geomorphic drivers in this region (Mazengarb and Speden, 2000). River incision and terrace formation via knickpoint retreat indicate regional uplift of 0.5–1.1 mm yr-1, (Berryman et al., 2000; Crosby and Whipple, 2006; Marden et al., 2008), with local uplift extending up to 6 mm yr-1 (Litchfield et al., 2020). Two main geologic terrains in the region are distinguished based on lithology, age, and style of deformation (Figure 3). Variably indurated and extensively sheared rocks of the “Cretaceous terrain” mainly comprise mudstone and sandstone of late Cretaceous to Palaeocene age (Mazengarb and Speden, 2000). The “Tertiary terrain” is less tectonically deformed, comprising bedded to massive sandstones and mudstones of early to middle Miocene age (Mazengarb and Speden, 2000). The combined impacts of climatic, tectonic, and lithologic factors make landscapes of the East Coast Region highly erodible.
FIGURE 3. Geology of Tairāwhiti (East Coast Region of Aotearoa New Zealand). Cretaceous terrain is in green, Tertiary terrain is in orange, yellow denotes floodplains and alluvial terraces (note the prominence of Poverty Bay Flats at the mouth of the Waipaoa River). The East Coast Allochthon exerts a significant impact upon sediment flux in this region as it hosts badass gullies (gully-mass movement complexes that induce profound local input of sediment that overwhelms the valley floor—see Marden et al., 2018).
Prior to human settlement vegetation across the region largely comprised tawa (Beilshmiedia tawa), titoki (Alectryon excelus), kohekohe (Dysoxylum spectible) and podocarp forest (Leathwick et al., 1995). Tall forests dominated by kahikatea (Podocarpus dacrydioides) and canopy of totara (Podocarpus totara) and matai (Podocarpus spicatus) characterised terrace and low-lying swampy areas. Māori arrived in the 14th century on two waka (traditional Māori canoes), Horouta and Takitimu (Spedding, 2006). Low-lying fertile flatlands adjacent to river mouths, notably in the vicinity of the Waimatā, have a long and continuous history of Māori settlement and land use (Coombes, 2000; Salmond et al., 2022). Colonisation brought about profound changes to forest cover and land use, as native forest was cleared extensively for pastoral agriculture (Ewers et al., 2006). Later government initiatives under the guise of Land Clearance Grants or Stock Retention Schemes encouraged further clearance of land that had reverted to shrubland in the 1930s Great Depression by providing subsidies for land, developed fertiliser grants, reduced loans, and guaranteed minimum livestock prices. Today, native podocarp forest accounts for only 2.5% of land cover in the region (40% pasture; 19.3% exotic plantation forest; 9.1% regenerating mānuka (Leptospermum scoparium)/kānuka (Kunzia ericoides) scrub).
Disturbance is the norm in the dynamic landscapes of Aotearoa New Zealand (sometimes referred to as the Shaky Isles). Uplift, volcanic activity, earthquakes and recurrent high intensity storms result in steep dissected terrains that are subject to profound rates of sediment generation, erosion and reworking. The tectonically active, steepland relief of the region, coupled with weak, erodible lithologies (Figure 3) and a humid climate (Figure 2) primed these catchments for Anthropocene transformation when indigenous forest cover was removed (Figure 4). In turn, changes to river structure, function and trajectories impact socio-cultural relations to rivers across the region. For their size, these rivers deliver some of the highest sediment yields to the ocean on the planet (Marden et al., 2018). The region contributes 33% of New Zealand’s total suspended sediment yield to the ocean (NZ total 209 Mt yr-1) from 2.5% of the land area (Hicks et al., 2000; Page et al., 2007). The Waipaoa, for example, delivers 15 Mt yr-1, with a specific sediment yield of 6,797 t km-2 yr-1, while the Waiapu delivers 35 Mt yr-1, equating to 20,577 t km-2 yr-1 (Hicks et al., 2000). Although the region has been subjected to near-uniform extents of deforestation (Figure 4), marked variability is evident in catchment-specific geomorphic signatures of the Anthropocene.
FIGURE 4. Contemporary vegetation cover and land use across Tairāwhiti catchments. The region has been subjected to near-systematic deforestation, with remnants of indigenous forest in northern and western headwater tributaries of the Waiapu and the lower course of the Mōtū. Urban development in the region is restricted to Gisborne itself.
The Waiapu is the highest sediment yielding river in Aotearoa New Zealand. Average annual rainfall is around 2,400 mm yr-1, notably higher than 1,470 mm yr-1 in the Waipaoa (Page et al., 2001; see Figure 2). However, average rainfall figures mask the extremes to which the Waiapu has been subjected, as this system has a higher recurrence interval of erosion-generating storms (Hicks, 1995). In 1938, the largest storm since deforestation delivered 762 mm of rainfall in 30 h, initiating extensive hillslope erosion and river bed aggradation (Kasai et al., 2005; Kasai, 2006). Cyclone Bola (March 1988) was the largest rainfall event on record, with 600 mm recorded at the coast and 900 mm in the headwaters over 4 days (Page et al., 2001).
Two major upstream catchments, the Mata (60% of total catchment area) and the Tapuaeroa (19%), feed the lower mainstem Waiapu and its tributaries (21%) (Figures 5A, B, D). Sediment supply varies markedly in the two upstream catchments. The Mata is dominated by Tertiary (mudstone, sandstone) and Cretaceous (greywacke, argillite) terrains, while the Tapuaeroa is underlain by the East Coast Allochthon, a series of moderately dipping, displaced thrust sheets that are structurally much weaker than the other more competent regional lithologies. Tunnicliffe et al. (2018) document a detailed sediment history of this river system.
FIGURE 5. Rapid sediment input from gully mass movement complexes following deforestation and subsequent forest management practices has induced dramatic geomorphic adjustment (aggradation) along the Waiapu River. (A) View upstream on the Tapuaeroa River; (B) Oblique view of the Tapuaeroa; the Mangapekeke (at left) and Mokoiwi (upper centre) have several gullies delivering a long-term supply of weathered material to the river network; (C) A view of the ‘badlands’ topography within Barton’s Gully, one of the largest sediment sources in the catchment; (D) The winding, single-thread morphology of the upper Mata River; (E) Gully stabilisation efforts in the upper Mata River, using Pinus radiata to slow the progression of a gully system; (F) Braided morphology of the lowermost Waiapu River; (G) The fan at the outlet of the Mangaoparo River moderates delivery of material to the mainstem Waiapu River; (H) A view looking upstream on the Waiapu River, showing large gravel bars with silty deposition on bar crests; the river has been subject to industrial gravel extraction in recent years.
The topography of the Mata is more subdued, and the overall gradient of the system is less than that of the Tapuaeroa. The winding, single thread morphology (Figure 5D) stands in contrast to other braided to wandering rivers in the catchment (Figures 5A, B, F–H). Bedrock is commonly exposed along channel margins, especially in confined canyons. In general, although the Mata is a very active sedimentary system, the river is in a supply-limited state. Delivery is episodic, and channels go through cycles of high sediment supply from landslides/gully deliveries (Figure 5E), followed by relatively quiescent episodes of subdued hillslope activity. Although the spatial distribution of gullies is less than the Tapuaeroa, at least two major gully systems contribute notable amounts of fine-grained sediment to the system. Shallow landslides, bank and cliff undercutting flanking incised channels, and minor rotational slumping are the main sources of sediment.
By contrast, the much smaller Tapuaeroa features a broad braided trunk channel (Figures 5A,B), reflecting prolific sediment delivery from numerous (>30) large gullies and gully complexes in the Allochthon terrain (Figure 5C; Betts et al., 2003; Leenman and Tunnicliffe, 2020; Tunnicliffe et al., 2018). A number of homesteads built on low floodplains have been buried in alluvium as the river has built up and widened in the last century. The sedimentary character of the river is strongly influenced by the weathered materials derived from the gullies. These are ongoing, long-term sediment sources, and the volumetric contribution of material greatly exceeds the bedload transport in rivers. Delivery is moderated somewhat by large buffering alluvial fans and rapidly aggrading riverbeds (Betts et al., 2003; Tunnicliffe et al., 2018; Leenman and Tunnicliffe, 2020). By far the greatest management problem in the catchment is abating fine sediment delivery from these gully systems.
Wandering-to-braided reaches along the lower course of the Waiapu River reflect high sediment delivery from the Tapuaeroa and Mangaoparo rivers (Figure 5G), and to a lesser extent, the Poroporo and Maraehara rivers (Figures 5F, H). As with other East Coast systems, the terraces along the river attest to a long-term (millennial-scale) tectonically-driven trajectory of degradation and incision. However, on the scale of the Anthropocene, the story has been one of pronounced aggradation. This points to the disequilibrium that has been introduced by human modification of hillslope landcover, and geologically recent unbalancing of sediment supply to the system.
From a river rehabilitation perspective, the geomorphic river story for the Waiapu emphasises that 1) managing slopes is generally a prerequisite to river rehabilitation efforts; 2) unsynchronised disturbance leads to considerable spatial and temporal variation in river bed-levels and behaviour; 3) every tributary exhibits notably different sensitivities, largely owing to geologic and tectonic factors, land-use and historic disturbance.
The response of the Raparapaririki River to Cyclone Bola is indicative of the vulnerability of tributary subcatchments that drain the highly erodible terrain. Here, an incising, wandering gravel-bed river has been replaced by a rapidly aggrading braided channel system, which has aggraded 20 m in 20 years (Tunnicliffe et al., 2018). The headwaters of this tributary had not, however, been cleared for pastoral agriculture and had remained under native forest. The intensity of Cyclone Bola was such that relatively pristine native forest was unable to protect slopes from intensive mass wasting from short, steep slopes, which efficiently connected eroded sediment to the channels. The Raparapaririki is typical of adjacent north-bank tributaries of the Tapuaeroa, which all rise in terrain underlain by crushed lithologies, subject to intense erosion and the presence of large (‘badass’) gully complexes (Leenman and Tunnicliffe, 2020). Total gully area in these catchments increased from 2 to 11 km2 between 1957 and 1997 (Marden et al., 2012; Leenman and Tunnicliffe, 2020). Over-supply of sediment to the receiving stream network resulted in aggradation and widening of alluvial fans at tributary confluences with the Tapuaeroa (Leenman and Tunnicliffe, 2020). The Tapuaeroa in turn has trimmed these fans, particularly driven by aggradation of the mainstem in response to sediment supplied by the Raparapaririki (Tunnicliffe et al., 2018; Leenman and Tunnicliffe, 2020). This scenario suggests that alluvial fans exert limited buffering capacity upon the Waiapu sediment cascade, unlike equivalent features in the headwaters of the Waipaoa (discussed later). The immediate location and geomorphic context of these features are important considerations when assessing how sediment stores affect longer-term river trajectories.
A small tributary of the Mangaoparo within the highly erodible terrain of the Waiapu does, however, provide some insight into longer-term recovery and potential trajectory of the larger systems in the Waiapu catchment. The Weraamaia was largely cleared of indigenous forest and is underlain by crushed and weathered sandstones and mudstones of the Whangai Formation (Mazengarb and Speden, 2000). Significant channel aggradation occurred in response to the 1938 storm, but formation of tributary junction fans in the Weraamaia reduced the conveyance of sediment through the system, allowing incision to occur prior to Cyclone Bola (Kasai, 2006). Reforestation by pine plantations began within the catchment in 1979, but this new forest cover provided insufficient protection in Cyclone Bola in 1988, which caused extensive landsliding, gullies and a debris flow in the catchment (Kasai et al., 2005; Kasai, 2006). An initial phase of valley floor aggradation was quickly followed by incision and evacuation of sediments by an unstable channel which entrenched and narrowed (Kasai, 2006). The lower reaches of the Weraamaia are now bedrock-controlled, with the Bola sediment remaining as an adjacent terrace (Kasai et al., 2005) (Figure 6). This trajectory has resulted from a combination of channel instabilities and entrenchment together with reforestation of the entire catchment, which has mitigated the impacts of subsequent storms to date. This scenario highlights the importance of contiguous forest cover in managing the sediment-overloaded rivers of the Waiapu catchment.
FIGURE 6. Bedrock exposed in the bed of the Weraamaia Stream, a north bank tributary of Mangaoparo River in the Waiapu Catchment (see Kasai et al., 2005; Kasai, 2006). Valley floor sedimentation during Cyclone Bola (1988) created the terrace visible at the edge of the tree canopy. A major storm in 1938 created similar features in upstream reaches. Photo: ICF, 28 August 2012.
Mostly underlain by Tertiary terrain, the topography in the Hikuwai catchment is steep (20°–40°, mean 32°, in places >40°) has fairly long rectilinear slopes and occurs at an altitude of between 42 and 133 m above sea level (Walley et al., 2018). Lower slopes often connect with very steep, incised bedrock gorges in the upper-middle catchment, while the river course in lowland reaches has a low width-depth ratio channel and a well-developed fine-grained floodplain (Figure 7).
FIGURE 7. Hillslope failures following deforestation and subsequent forest management practices have induced excessive flux of fine-grained sediment and waste wood through the Hikuwai river system. These materials are readily conveyed within a slot-like channel from the Mountains to the Sea. (A) Hikuwai River and floodplain. Photo: 2 February, MM; (B) Wood waste generated from logging activities and delivered to a first-order stream channel by shallow landslides, debris slides and debris flows, Tapaue Stream, Hikuwai catchment. Photo: 11 July 2018. Townsend, GNS Science; (C) Overbank sedimentation and wood deposits, Tapaue Stream floodplain. Hikuwai River labelled (flow left to right). Photo: 11 July 2018 (D). Townsend, GNS Science (with permission); (D) Forestry slash (wood waste) within and adjacent to the Mangatokerau River ∼300 m upstream from its confluence with the Hikuwai (looking upstream). Note the entrenched, narrow channel inset within a fine-grained floodplain. Photo: 29 August 2018, ICF.
Bedrock in the Hikuwai consists of mudstone with intercalated sandstone, conglomerate and tuff (Mazengarb and Speden, 2000), overlain by varying thicknesses of volcanic tephra, and a thin (15 cm) forest topsoil. Soils are well drained, typical of materials reworked by active hillslope processes (Hewitt 2010). Geomorphic mapping of 1997 imagery reveals 290 active gullies with a combined area of 8.44 km2. An estimated annual sediment supply rate of 2.7 Mt yr-1 (4,847 t km-2 yr-1) was derived from these features, equivalent to 54% of the average annual suspended sediment yield (Marden et al., 2008). Despite significant further plantings of exotic forest, 337 actively eroding gullies with a combined area of 769 ha remain in the catchment (Marden et al., 2020).
Mean annual rainfall in the Hikuwai catchment varies from about 1,400 mm at the coast to 1700 mm at higher altitudes (Figure 2). The proximity of steep terrain to the coast induces high rainfall totals. For example, the catchment was subjected to the highest storm total rainfall of 900 mm in the region during Cyclone Bola in 1988. Localised storm cells triggered significant erosion of unstable, steep hill country in 2005, 2009, 2014, 2015, 2017, 2018 and 2023. A storm on 3-4 June 2018 delivered 234 mm of rain in 24 h to the headwaters of the Hikuwai, and a further 270 mm fell on 11-12 June 2018. These storms triggered approximately 6,680 landslides (including shallow translational soil slides, debris slides and debris flows). The total landslide area of 2.4 km2 is estimated to have produced 4.2 M m3 of sediment, of which approximately 2.1 M m3 or 2.6 M tonnes was delivered to the Hikuwai River (Rosser et al., 2019). This is equivalent to nearly 50% of the total 5 M tonnes annual suspended sediment yield from the catchment (Hicks and Shankar, 2003). Between 25-50 mm of fine-grained overbank sediments accumulated across a floodplain area of 426 ha in the lower reaches of the catchment (Rosser et al., 2019).
Delivery of slash (waste debris from forestry operations) to the channel during significant storm events has become a major concern in the Hikuwai (Figure 7D). Collapse of landings where logs are processed on steep slopes delivers large quantities of wood waste to channels (Marden et al., 2023). Slash from former forest practices adjacent to channel margins was readily mobilised during recent (2018-2023) storm events, while landslides on recently harvested (clear-felled) slopes also mobilise wood into the channel network (Figures 7B, C) (Rosser et al., 2019). Connectivity of this wood waste with channels in the Hikuwai resulted in log jams and exacerbated flooding (Figure 7D). This anthropogenic modification of prevailing processes adds a further dimension to the river story and associated needs for rehabilitation. Ironically the measures used to mitigate erosion and intended to reduce sediment connectivity and yields in the Hikuwai (afforestation of steep slopes) have created new issues of river degradation in this Anthropocene river. Rehabilitation requires drastically modified forest harvesting practices which currently clear-fell entire slopes. Particular attention to the management of wood waste is required. Alongside this, native regeneration and economic retirement of steep slopes adjacent to the Hikuwai channel network is recommended as part of a whole-catchment approach that recognizes the connectivity characteristics of the Hikuwai riverscape (see Marden et al., 2023).
The 61 km long Waimatā River extends from dissected headwaters (>300 m asl) in the Raukūmara ranges, draining an area of 370 km2 as it flows through pastoral landscapes prior to joining Taruheru Stream in Gisborne township. Beyond this confluence, Aotearoa New Zealand’s shortest river—the 1.2 km long Tūranganui River—supports the local port before flowing into Poverty Bay (Figure 1). The catchment retains less than 17% native forest cover, with less than 50% of the catchment being forested (exotic or native). Many wetland areas have been drained along low order watercourses (Harvey, 2021).
Waimatā Catchment primarily comprises weak, highly erodible Paleogene sedimentary rocks (Figure 3), with mudstone the dominant lithology and some intrusions of well bedded alternating sandstone and mudstone with shelly muddy sandstone (Mazengarb and Speden, 2000). Weak unconsolidated soils in highly dissected landscapes of steep headwater areas are very susceptible to landslips. Large volumes of tephra originating from the Taupo Volcanic Zone and draped across much of the mid-upper catchment have been reworked and redistributed along valley floors. Pumiceous soils with low clay content, generally less than 10%, result in high macro porosity and deep rooting depths but very low soil strength. Distinct hummocky terrain of moderately-sloped areas downstream reflects shallow (∼0.5 m deep) earthflow activity induced by strata with smectite clay (Mazengarb and Speden, 2000; Marden et al., 2012). Mud volcanoes are present along zones of fault shearing in this area (Leighton et al., 2022).
Hillslope/channel connectivity in Waimatā Catchment is relatively low as alluvial fans, floodplains and wetlands/swamps act as buffers that disconnect sediment conveyance to the trunk stream (Fryirs et al., 2007). Once fine-grained sediments enter river courses, limited accommodation space for sediment storage along terrace-constrained reaches creates efficient sediment delivery pathways. Significant log accumulations on beaches transferred from areas of plantation forestry during major storms attest to the high longitudinal connectivity of this river system. Although terrace-constrained reaches flush significant volumes of fine-grained sediment, analysis of channel outlines from 2000 to 2020 reveals virtually no lateral channel adjustment in this river system (Harvey, 2021). Much of the catchment operates as “transfer” and ‘throughput” reaches (Figure 8), with localised bend adjustment in the former and reworking and redeposition of channel-marginal sediment stores in the latter (cf., Brierley and Fryirs, 2000). The flume-like behaviour of the river promotes efficient biophysical linkages from the mountains to the sea (Orpin et al., 2006; Harvey, 2021). Marden et al. (2014) estimated that 2.6 km3 of sediment has been produced in Waimatā Catchment since the Last Glacial Maximum (LGM).
FIGURE 8. The Waimatā River operates as a terrace-constrained flume-like chute that readily conveys fine-grained sediments (and forestry logs) from the Mountains to the Sea. Throughput reaches dominate the mid-lower course of the river, with a flume (slot-like) channel inset within terraces subject to recurrent reworking of the bed and banks (A, B). Longitudinal connectivity is high, and sediment and logs are readily conveyed through the system. It is only in local transfer reaches that the channel has capacity (space) to laterally adjust (C, D).
Most reaches in Waimatā Catchment are considered to be relatively resilient and have significant capacity for recovery (sensu Reid and Brierley, 2015). Trapping sediment at source, before it enters watercourses, requires catchment-wide management of forestry (reforestation) and pastoral practices that include the planting and management of riparian vegetation, perhaps considering prospects for re-instigation of instream wood and wetland reconstruction to improve ecological habitat. Reforestation using native timber species over longer rotation lengths than exotics is another option (Marden et al., 2023), as is abandonment of the most erosion prone areas to reversion either by passive means (i.e., walk away and allow recolonization to occur) or through planting of early colonising native species such as manuka/kanuka with the view to the production of honey and other associated medicinal products (Marden et al., 2020; Lambie et al., 2021). Such interventions would have considerable benefits for flood management in lowland reaches, reducing delivery of fine-grained sediment and logs to the beach.
High rates of erosion are the defining catchment management issue in the Waipaoa. Marden et al. (2014) estimate that this 2,208 km2 catchment has generated 14.08 ± 2.1 km3 sediment since the last glacial maximum. Since the mid 20th century human activity has accentuated the rate of sediment generation, producing an annual suspended sediment yield of 15 M tonnes yr-1 (Hicks and Shankar, 2003; see Marden, 2012). The primary sources of sediment are hillslopes, especially gullies, which include classic deep, unstable, incising linear channels along incipient drainage lines, as well as much larger gully-mass movement complexes (badass gullies developed in highly weathered and crushed Cretaceous shales associated with the East Coast Allochthon; Figures 9A–D; Fuller et al., 2020; Marden et al., 2018). Gullies often overwhelm receiving streams, creating alluvial fans that temporarily impound drainage, are repeatedly trimmed during floods, and subsequently refill (Fuller and Marden, 2010, 2011; Taylor et al., 2018). Short, steep slopes in the Waipaoa mean that near-ubiquitous shallow landslides (Figure 9G), alongside materials derived from sheet erosion and occasional deep-seated landslides (Figure 9F; see Bilderback et al., 2015), couple effectively with the channel network during large storm events (De Rose and Basher, 2011; Jones and Preston, 2012). Sediment is also fed continuously to channels that intersect with earthflows (Figure 9E; Cerovski-Darriau and Roering, 2016; Marden et al., 2014). The long-term (multi-decadal) Waipaoa sediment yield is dominated by the cumulative effects of low-magnitude events: 75% of sediment produced from landslides occurs during storms with recurrence intervals of less than 27 years (Reid and Page, 2003) and in the Mangatu sub-catchment, half of the long-term average load is transported by events with return periods of less than 1 year (Hicks et al., 2000). This highlights the efficacy of sediment delivered from gully systems in this part of the catchment (Marden et al., 2018).
FIGURE 9. The Waipaoa River is a globally significant example of an overloaded channel with exceedingly high sediment flux. Deforestation and subsequent forest management has created an Anthropocene riverscape that is prone to profound, rapid and recurrent geomorphic adjustment. The river changes downstream from a rapidly aggrading bedload to an aggrading suspended load dominated river. Gully mass movement complexes and landslips induce rapid fan and valley floor aggradation. Despite the buffering effects of terraces and fans, sediment stores along channels are readily reworked in this system that maintains high connectivity from the Mountains to the Sea. Dramatic landscapes of Waipaoa Catchment. (A) upper Tarndale fan, (B) Tarndale gully, (C) Mangatu gully, (D) Mangatu fan, (E) earthflow, (F) deep seated landslide, (G) shallow landslide (courtesy Mike Crozier), (H) overloaded stream bed (Te Weraroa), (I) mid-Waipaoa, (J) Waipaoa at Kanakanaia, (K) Waipaoa at McPhails bend, Poverty Bay Flats.
In response to sediment overload, the Waipaoa has been transformed from a single-thread, clear-flowing, cobble-bedded river, to a multi-thread, sediment-laden, rapidly aggrading channel with matrix-rich gravelly beds in the upper and mid-catchment (Figure 9A, D, G; Hamilton and Kelman, 1952; Gomez et al., 2001; Page et al., 2007; Gomez and Livingston, 2012). The upper river has aggraded by up to 20 m over the last century (Marden, 2011). Beyond the partly-confined mid-catchment reach (Figure 9I), the lower Waipaoa River, once gravel-bedded and laterally active, has become fixed between cohesive, silty banks and floodplain sedimentation is dominated by rapid vertical accretion (Gomez et al., 1998). Marked increases in the supply of fine-grained sediment have resulted in reduced bankfull width and cross-section area, leading to channel contraction (Figures 9J, K; Gomez et al., 2007, 2009). The direct feed of fine sediment from gullies and rapid breakdown of gravel-sized clasts of highly erodible shales and mudstones supplied from hillslope erosion in the upper catchment contribute to high suspended sediment loads (Hicks et al., 2000; Gomez et al., 2001). The Waipaoa Flood Control Scheme, designed in 1949 and completed in 1969 to provide ‘100-year’ (1% AEP) flood protection for over 10,000 ha of intensive horticulture on the Poverty Bay Flats, reduced channel capacity and floodplain accretion in the lower Waipaoa. A ∼$35 million upgrade of the Scheme is underway to maintain ‘100 years’ protection accounting for climate change impact to 2090 (GDC, 2023). The work, due for completion in 2031, will upgrade 64 km of stopbank, increasing its height by 1-2 m and crest width to 4 m (GDC, 2023).
Considering implications for geomorphologically-informed approaches to river rehabilitation, first and foremost a reduction in the incidence and impact of mass movement (gullies, landslides and earthflows) as originators of sediment is required. Secondly, the release of sediment stores associated with large, badass gully complexes in the form of short, steep, fans must be modulated. Thirdly, sediment needs to be retained on hillslopes while allowing for agricultural production. Initial management approaches to control erosion using traditional soil-conservation practices such as space-planted poplar and willow on eroding hillslopes and construction of in-channel debris dams in low-order tributaries such as Tarndale (Figures 9B, C) were largely ineffective (Allsop, 1973; De Rose et al., 1998; Marden et al., 2014). The overwhelming volume of sediment delivered from large gully complexes like Tarndale and Mangatu simply buried in-channel structures (Figures 9A, C; De Rose et al., 1998). In the 1950s the New Zealand government purchased large tracts of the most eroding land for reforestation and planting began in 1961 (Marden et al., 2014). By 1985 96% of the upper Waipaoa and Mangatu catchment had been reforested, principally Pinus radiata (Marden et al., 2014). A second phase of forest planting commenced in the wider catchment and region following extensive damage sustained to large tracts of pastoral hill country during successive storms in the 1980s, most notably Cyclone Bola in 1988. By 1997 a total of ∼137,000 ha of eroding pastoral hill country had been replanted in exotic forestry (Marden et al., 2012). Use of poplar and willow plantings to stabilise hillslopes and reduce channel scour successfully shut down some smaller gullies (Herzig et al., 2011; Marden et al., 2011; Marden, 2012). However, attempts to mitigate sediment production failed for larger amphitheatre fluvio-mass movement gully complexes (>10 ha in area) or ‘badass’ gullies like Tarndale (Figure 9B; Fuller et al., 2020; Marden et al., 2018) and these features remain key sediment generators in the catchment today.
Re-vegetating hillslopes in erosion-prone areas of the Waipaoa with woody vegetation of a sufficient density of stems per hectare (1,250) very effectively reduces off-hillslope sediment delivery from this terrain (Marden et al., 2014, 2018) and disconnects slope-channel coupling. Treatment of gullies using this approach requires early intervention, since large, ‘badass’ gully complexes are beyond remediation (Marden et al., 2018). Despite further efforts to stabilise gullies, 385 remain actively eroding of which 50 are badass gullies (Marden and Seymour, 2022). However, sediment supply does appear to be diminishing from these systems over time, as structural resistance increases with greater system complexity and growth of buffering fans (Fuller and Marden, 2011; Fuller et al., 2020). Targeted management of complex process interactions at the junction between the fan and the channel is critical to the supply of sediment to the channel network (Fuller and Marden, 2011; Taylor et al., 2018; Fuller et al., 2020). Loading of the fan by sediment supplied from the gully complex steepens the fan profile, enhancing connectivity and sediment delivery (Fuller and Marden, 2011; Leenman and Tunnicliffe, 2018; Leenman and Tunnicliffe, 2020). During these phases, the distal fan grows because sediment delivery exceeds transport capacity of the stream channel and this scenario may impede mainstem flow, forming a lake. Sediment from the distal fan is also actively eroded by flood events in the stream channel—the fan in this case is passive since the process conveying sediment to the channel is driven by stream processes. Trimming of the distal fan by lateral stream erosion steepens the lower fan, generating a headcut which recedes up-fan. This re-activates the fan and evacuates sediment to the channel. Enhancing sediment retention in buffers and lowering fan-channel connectivity will reduce sediment delivery from these critical parts of the Waipaoa sediment cascade.
Stabilisation of fans can be achieved by reforestation, which restricts the width of the active channel (Gomez et al., 2003). However, Taylor et al. (2018) suggest that residence times for sediment in the now much smaller active part of the Tarndale fan have likely reduced, enhancing sediment connectivity. Perversely, reforestation of the Tarndale fan may have enhanced sediment volume delivered to the stream channel by some 17,000 t yr-1 (Marden et al., 2005). Similarly, Leenman and Tunnicliffe (2020) observed that the potential for fans in the adjacent Waiapu catchment to act as buffers is dependent on the relative speed of fan trenching (connectivity enhanced) and mainstem incision (disconnecting fan). As gully contribution decreases over time, it will be imperative to stabilise the lower fans to minimize reworking of sediment stores. Gullies and their associated fans are not in sync across the catchment. Considerable year-to-year variability in the volume of sediment supplied from badass gully complexes reflects intrinsic landform adjustment, as well as extrinsic drivers (Fuller and Marden, 2011). The residence time of material delivered from gully to fan may range from decades to months (Taylor et al., 2018). Fuller et al. (2020) highlight the individualistic behaviour of adjacent gully complexes at Tarndale and Mangatu which have a decadal (2005-2019) sediment delivery ratio of 0.65 and 0.78 respectively.
Retention of pastoral production in areas of less erodible terrain will not accentuate problems so long as sediment generated by sheet erosion and shallow landslides is regulated. Slope-channel coupling from shallow landslides in small catchments is dependent on catchment morphology, landslide and triggering event characteristics (Jones and Preston, 2012). Space-planting of poplars and willows and/or a sufficient stem density of native mānuka or kānuka can be effective to reduce the probability of hillslope failure, helping to retain soils on hillslopes and prevent failures in the first place, buffering streams from landslide debris (Ekanayake et al., 1997; Marden et al., 2020). Riparian planting can mitigate remobilization of colluvium stored at the toe of hillslopes into streams. Once materials enter the channel network, it becomes much more difficult to manage sediment accumulation. Hence, managing the problem at source by reducing hillslope erosion is vital. To put Waipaoa sediment sources into perspective, the contribution of sediment from riverbanks and cliff collapse from lateral erosion was estimated at <2% of the total Waipaoa load (De Rose and Basher, 2011). Most of this lateral erosion is in widening (110 mm yr-1) and aggrading (40 mm yr-1) channels upstream of the Waipaoa gorge reach, while in the lower reaches the channel is narrowing (201 mm yr-1) and accreting (17 mm yr-1) (De Rose and Basher, 2011).
Shutting down hillslope-erosion sources in the Waipaoa has already affected the behaviour of the trunk stream, as the upper Waipaoa River and some headwater tributaries are now incising (Rosser and Jones, 2021). The corollary is that this process continues to generate sediment, which is fed downstream. Management of connectivity in this catchment is multi-centennial in scope. Adopting erodible corridor concepts and managing adjacent land tenure and land use is needed to accommodate the ongoing high rates of sediment supply to the lower Waipaoa as the channel continues to adjust to ongoing sediment influx in the coming decades (Brierley et al., 2023). Although gravel extraction could support management of aggrading reaches if carried out carefully and managed in an adaptive manner, in this instance the poor quality of the weak shales and sandstones comprising the bulk of the Waipaoa bedload means this is unviable, and bedload yields are just ∼1% of the suspended load (Gomez et al., 2001).
The Mōtū Catchment drains an area of 1,373 km2 and is located at the apex of the Raukūmara Ranges (Figure 1) comprising late Jurassic to Early Cretaceous sandstone and mudstone (greywacke), and localised conglomerate which forms the Mōtū Falls (Figure 10A; Mazengarb and Speden, 2000). Downstream, the river flows through a deeply-incised bedrock gorge before emerging at the coastline within the Bay of Plenty region.
FIGURE 10. High accommodation space has created opportunity to store large volumes of sediment on valley floors along upper reaches of Mōtū Catchment. Mōtū Falls (A) acts as a local base level, separating this area from a gorge that extends to the coast. Terraces buffer hillslope sediment inputs to the channel and restrict channel migration in upper reaches (B, C). The dominant contemporary sediment source is reworking of valley floor sediments by incision (headcut erosion) and channel expansion of the laterally migrating meandering river (D, E).
Accommodation space on the valley floor in the high-elevation, low-relief upper catchment above Mōtū Falls (Figure 10A) has facilitated sediment accumulation and the development of river terraces during the Quaternary (Berryman et al., 2000). This has developed in response to the creation of a local base level at the Mōtū Falls in combination with unevenly distributed regional uplift, estimated to be 2 -3 mm yr-1 at Mōtū Falls and 1 mm yr-1 upstream at the headwaters (Litchfield and Berryman, 2006). This allowed the river to carve out the space in which Quaternary valley fill sediment has subsequently accumulated (Berryman et al., 2000). The contemporary river is now reworking the sediment on the valley floor, with the lateral migration of the river restricted by the river terraces in the upper reaches which rise up to 40 m above river level upstream (Figure 10B), decreasing to 15 m downstream (Figure 10C).
Notable channel adjustments are restricted to meandering reaches immediately above Mōtū Falls. Anthropogenic disturbance associated with land use change and clearance of riparian vegetation and wood likely brought about a shift in sediment regime in this area, transforming the river from a sediment storage and accumulation zone into a sediment source (cf., Fryirs and Brierley, 2001). Incision and channel expansion reflect acute adjustments of a geomorphologically sensitive river (Figure 10E; sensu Reid and Brierley, 2015). Sediment fingerprinting identifies stream bank erosion as the dominant contemporary sediment source, contributing 95% of instream sediment, with negligible input from hillslope failures (Vale et al., 2021).
Mature forest cover in the Upper Mōtū was progressively cleared in the early 1900s (Twisleton, 2007). This changed the discharge regime, and what was previously inferred to be a wide swampy valley floor has now formed a defined channel which shows evidence of incision and widening (cf. Brooks et al., 2003). The variability in channel entrenchment within the Upper Mōtū suggests that the contemporary river is at different stages of channel evolution and is still adjusting to the change in flow regime (Simon, 1989; Simon and Rinaldi, 2006). Managing sediment flux at source and at scale in this catchment entails rehabilitation of the channel bed and banks as the influx of sediment from hillslopes is largely buffered in this instance (Marden et al., 2014; Leenman & Tunnicliffe, 2018).
Regime shift induced by geomorphic responses to deforestation on the highly erodible terrains of Tairāwhiti over the last 150 years clearly validates conceptions of Anthropocene rivers (Thoms et al., 2020; García et al., 2021; Meybeck et al., 2023 . While profound anthropogenically-induced landscape transformation has been ubiquitous across this region, the geomorphic river stories for the five study catchments highlight profound variability in river diversity, process regimes (sediment sources, connectivity relations) and evolutionary trajectories (Table 2). Unruly rivers on the East Coast present significant management challenges as they respond to massive sediment inputs from rapidly eroding slopes. Other than stopbanks (aka flood-walls, artificial levees) and local bank erosion control structures along lower reaches, indirect, systematic and unintentional responses to deforestation have been the key determinant of contemporary river character and behaviour across the region. However, the physical manifestation of anthropogenic disturbance, and associated prospects for geo-ecological recovery through appropriately targeted rehabilitation practices, vary markedly from catchment to catchment. Managing at source, at scale and in a timely manner are key to the success of proactive and precautionary management plans that promote geomorphic river recovery (Fryirs and Brierley, 2016).
TABLE 2. Application of geomorphic principles (Table 1) to generate river stories that support rehabilitation of Tairāwhiti catchments.
Fit-for-purpose rehabilitation plans build upon catchment-specific understandings of formative processes (sediment sources) and connectivity relationships (Brierley and Fryirs, 2009, 2016, 2022; Poeppl et al., 2020). Management programmes that ‘work with the river’ strive to ensure that appropriate process-based actions are applied at the right place at the right time/sequence (Marden and Seymour, 2022). Rivers across the East Coast region get their sediments from differing sources with variable residence times and material properties. Alongside this, variable connectivity relationships (drainage network configuration, tributary-trunk stream relationships, geomorphic hotspots) drive the operation of the ‘jerky conveyor belt’ in each system. A prescriptive, ‘one-size fits all’ approach to rehabilitation will not address differing issues in these degraded and diverse catchments.
Reaffirming long-held knowledge and foundation principles is sometimes key to effective policies and planning, and outcomes that ensue on-the-ground. For example, coordinated management of land and water at a catchment scale determines the effectiveness of rehabilitation programmes … like the veins on a leaf, the catchment feeds the river (Davis, 1906). Geomorphic stories from the five study catchments highlight differing meanings and implications of this simple premise. Local management of primary sediment sources in gully mass movement complexes is vital in Waiapu and Waipaoa catchments, and together with systematic reafforestation of all five case study rivers, will be required to effectively rehabilitate these catchments. However, sediment flux will not be reduced in the Mōtū system unless targeted measures tackle the reworking of sediments on the valley floor (bed incision and channel widening). In all instances, conservation of remnant indigenous forest and reversion to a continuous native forest cover is to be encouraged, both passively and actively. Region-wide planting of fast growing exotic Pinus radiata to secure an economic return and mitigate erosion at source has proven to be effective in some parts of some catchments, but has failed to shut down the largest gullies, which are beyond remediation by this (and realistically, any) strategy (Marden, 2012; Marden et al., 2014; Marden et al., 2018; Marden and Seymour, 2022). However, re-afforestation of gully-prone Cretaceous terrain in the headwaters of the Waipaoa and Waiapu successfully and quickly stabilised small (1-2 ha) gullies, and gullies up to 10 ha were fully stabilised under mature (∼27 years) P. radiata (Marden and Seymour, 2022). Unfortunately, the success of afforestation in the region’s Cretaceous terrain was offset by new gully development in remaining erosion-prone pastoral hill country and the expansion of untreated gullies, especially those induced by Cyclone Bola, with pronounced responses in the Tertiary terrain of the Waiapu catchment (Tunnicliffe et al., 2018; Marden and Seymour, 2022).
Singular emphasis upon afforestation with P. radiata and conversion of swathes of catchment to production forestry is not the panacea. Gully geomorphology influences the success/failure rate of afforestation to remediate gullies (Marden et al., 2018; Marden and Seymour, 2022). Rehabilitation practices must take into account the geomorphic role of ‘badass’ gully complexes which dominate the sediment flux in the Waipaoa and Waiapu catchments. A staged planting programme, which first targets the higher, less mobile slopes surrounding the gully with P. Nigra, and then once established, the slopes immediately flanking the gully with P. radiata, has been shown to be more effective than a blanket-approach with one species (Marden et al., 2005). This staged approach modulates the connectivity within the gully system and between the gully and stream network (Marden and Seymour, 2022). Catchment-specific insights and location contexts guide determinations of what rehabilitation efforts are likely to work, where, and under what circumstances.
The Hikuwai example demonstrates what can go wrong with production forest in the wrong place and with poor practice. Although exotic forest has reduced sediment supply to the channels, it is clearly not without significant problems. Clear-felling on short-steep slopes adjacent to incised channels heightens the risk of sediment generation and delivery to the river, including any slash (Marden et al., 2023). High volumes of wood waste generated from these forests reflect the poor quality of timber established on unstable slopes (Marden and Seymour, 2022). Selective forestry rather than clear-felling, and retention of riparian strips adjacent to channels, would help mitigate erosion risk and modulate connectivity between slopes and channels in these situations. Alternatives to exotic afforestation to mitigate slope erosion and sediment delivery to these rivers include shrubland reversion, both passive and managed, where fully stocked stands of mānuka and kānuka offer protection equivalent to closed-canopy P. radiata (Marden and Seymour, 2022), and consideration of alternative exotic species with longer rotations including Douglas-fir (Psudotsuga menziesii) and/or coppicing species including coastal redwood (Sequoia sempervirens D.Don) (Marden et al., 2023). Elsewhere, especially in Hikuwai and Waimatā catchments, wetland rehabilitation along low order streams may be helpful in trapping and storing sediment.
Building on past experiences, a commitment to experimentation and adaptive management is recommended. For example, the first attempts at using fascines and check dams for on-site erosion control in gullies were only successful for smaller and linear-shaped gullies but not for controlling larger gullies and especially gully-complexes (Marden et al., 2008). It was failure of these poorly-conceived approaches that led to subsequent broad-scale afforestation. Approaches need to be targeted, staged, and enforced if they are to be effective (Marden and Seymour, 2022). They must also be conducive to the Anthropocene landscape within which they are set.
On the assumption that erosion sources can be treated, or better, prevented, in Tairāwhiti’s catchments, the form and timescale of channel adjustment in these systems must be understood. River bed aggradation will continue for some time, even if all erosion sources could be shut down immediately, because the channel beds themselves comprise such a large sediment store that is being actively reworked, mobilised and delivered downstream (Tunnicliffe et al., 2018). Fine-grained, highly erodible materials stored along valley floors are readily entrained by Tairāwhiti’s rivers during periods of increased stream flow. In addition, sediment stored in fans adjacent to these actively migrating rivers will continue to be actively reworked, and badass gullies will continue to supply sediment to these temporary sediment stores (Fuller and Marden, 2011; Leenman and Tunnicliffe, 2020). The result is multiple decades (if not centuries) of ongoing river aggradation, especially in lower reaches (Gomez et al., 2009). Herzig et al. (2011) estimated that if all present and newly initiating gullies were successfully treated, the annual sediment yield from the region would halve by 2050; but if not treated, sediment yields would double by 2050 in gully dominated catchments such as the Waiapu. The legacy of over a century of intensive and widespread catchment erosion has overloaded these river courses. Although there are signs of sediment exhaustion in the upper parts of some tributaries, where channels have incised, whole-system recovery is a very long-term prospect (e.g., Tunnicliffe et al., 2018). Such understandings help to determine what is realistically achievable in terms of river rehabilitation (Brierley and Fryirs, 2022). Realistic short-medium term targets (50-100 years) cannot be conceived in terms of well-behaved, clear-flowing cobble-armoured rivers. In resilience terms, these rivers have moved into a new basin of attraction, and a return to pre-Anthropocene conditions is simply not achievable (cf., Piégay et al., 2020). Over time, however, the capacity for adjustment is likely to reduce, as re-working and incision of channel beds creates more stable, armoured conditions, reduces bed gradients, and evacuates fine-grained sediments. This will not happen uniformly and discrete reaches will adjust at different times reflecting their position in a catchment, proximity to sediment sources and adjustment of connectivity relationships therein. Accordingly, flexible, open-ended and dynamic rehabilitation goals need to be set (Brierley and Fryirs, 2016). Geomorphic river stories present an appropriately contextualised overview of place-based understandings that document how responses to disturbance and historical impacts (legacy effects) are manifested and communicated through the system. Analyses of evolutionary trajectory framed in relation to understandings of catchment connectivity inform interpretations of what can be expected in the future (Wohl et al., 2019). Collectively, these knowledges-in-place can support scientifically-informed transfer of insights and management applications from one situation/locality to another.
Within-region variability in anthropogenic impacts upon rivers in Tairāwhiti is considered to reflect:
• Dominant sediment sources and associated formative processes that determine the type, form and rate of input
• Sensitivity—capacity for adjustment and range of variability
• Catchment connectivity (hillslope-valley floor and upstream-downstream relations)
Variability in the river stories presented (Table 2) reflects, to a large extent, the location, nature and extent of erosion processes across the Tairāwhiti catchments, coupled with the success, or otherwise of erosion mitigation efforts and approaches. The flume-like nature of the Waimatā reflects the fact that there are no large gully systems in this catchment, the river is not over-supplied with sediment and it efficiently conveys material from slopes to sea as the river has incised deeply into soft mudstone terrain. Similarly, the Mōtū has the largest proportion of comparatively coherent greywacke and no gullies, despite forest clearance from its headwaters (although forest is intact in the lower catchment). Clearance here has affected more of a hydrological change, altering (increasing) runoff, promoting channel incision and reworking of adjacent floodplain sediment stores. As noted, the contrast between the Waipaoa and Waiapu is marked, reflecting the position of the most erodible terrain in each respective catchment. Channels are braided in the upper Waipaoa, but become single-thread and meandering as the over-supply of crushed-bedload material from gullies in the headwaters disintegrates into constituent fine-grained materials. In contrast, the Waiapu River only becomes braided downstream of its confluence with the overloaded (and braided) Tapuaeroa tributary. More coherent lithologies within the Waiapu compared with the Waipaoa also mean the gravel load is coarser. Short, steep slopes in the Hikuwai adjacent to narrow floodplains and incised channels mean that landslides and debris flows effectively convey shallow regolith materials to the valley floor, resulting in rapidly accreting channels choked by fine-grained sediments.
We contend that derivation and use of catchment-specific geomorphic river stories provides a compelling platform to relate scientific understandings of river systems to local and traditional knowledges to co-craft shared approaches to river management. Working with Mātauranga Māori, a living river ethos seeks to protect and enhance the mana (authority), mauri (lifeforce) and ora (wellbeing) of each and every river (Hikuroa et al., 2021). Viewed in this way, river stories can be conceptualised as ‘data with a soul’—a mechanism to relate geomorphologically-informed understandings of rivers to local and traditional knowledges (Brierley et al., 2021). Such conceptualisations recognize contested understandings and meanings of Anthropocene riverscapes, and decisions made regarding their rehabilitation. Abstracting humans from rivers whether by command-and-control ethos or attempted protection practices reduces the mana, mauri and ora of a river (Salmond et al., 2019).
In many parts of the world direct human impacts have transformed the geomorphic character and behaviour of rivers. Such actions and responses are not very pronounced for rivers that make up the East Coast Region (Tairāwhiti) of Aotearoa New Zealand. However, deforestation has induced a profound indirect impact upon these rivers—rivers that already had some of the highest rates of sediment flux per unit area in the world. Massive increases in sediment inputs have induced tens of metres of channel bed aggradation over just a few decades in some instances. However, forms and extents of geomorphic transformation, and associated prospects for rehabilitation, vary markedly from catchment to catchment across the region.
This paper proposes a new conceptual framework which we term geomorphic river stories to synthesize process-based insights into catchment-specific evolutionary trajectories. We show how proactive and precautionary river conservation and rehabilitation plans for five river systems in Tairāwhiti apply understandings of what is realistically achievable in management of a given system to develop fit-for-purpose rehabilitation strategies that work at source and at scale, targeting primary contemporary sediment sources in each instance.
Although a singular focus on geomorphology will not engender sustainable outcomes, conservation planning will NOT be effective unless geomorphic considerations are integrated into management plans (e.g., Brierley and Fryirs, 2022). Within the Waiapu Catchment, the Mata subcatchment is in sediment deficit and incising, while the Tapuaeroa subcatchment is unruly and unpredictable, reflecting rapid overload of sediment from gully mass movement complexes. As a whole, this is a globally significant example of a river subject to significant sediment flux and dramatic geomorphic adjustment (aggradation). The Hikuwai and Waimatā Rivers convey excessive fine-grained sediment (flux), together with forestry slash within a slot-like channel from the mountains to the sea. The Waipaoa is another globally significant example of an overloaded channel with exceedingly high sediment flux that is prone to profound, rapid and recurrent geomorphic adjustment, reflected in marked downstream transition from a rapidly aggrading bedload to an aggrading, suspended load dominated river. High connectivity from the mountains to the sea characterise this system where sediments derived from gully mass movement complexes and landslides readily accumulate as fans and valley floor bedload, yet remain readily and recurrently reworked. In contrast, while alluvial terraces within the upper reaches of the Mōtū River afford considerable accommodation space for the storage of large volumes of sediment, they also buffer hillslope sediment inputs to the channel. The dominant sediment source is therefore derived by incision (headcut erosion), reworking of valley floor sediments, and by channel expansion through bank erosion within a laterally migrating river. Among Tairāwhiti’s rivers, the Mōtū is uniquely different.
In each of the eastward-draining systems the key priority for catchment management is re-vegetation of slopes, which are the key sediment contributor in each catchment. A singular focus on the channels of these Anthropocene rivers cannot result in successful rehabilitation outcomes. Whole-of-catchment approaches are urgently required if rehabilitation is ever to be achieved. The timescale to restore these catchments is multi-generational, if not multi-centennial (Table 1). Rehabilitation started 60 years ago with the establishment of exotic forests, but unfortunately without due consideration given to what species to plant and where to gain maximum effectiveness. The last 60 years’ effort while having success in some parts of the landscape (particularly Cretaceous terrain) have also seen spectacular failures, notably the establishment of production forestry on steep slopes in Tertiary terrain, where vulnerability to shallow landslides increases dramatically during storms and where other vegetative solutions need to be found (see Marden et al., 2023). Cyclones Hale and Gabrielle in 2023 brought problems associated with increasing landslide occurrence and slash mobilisation following production forestry harvesting on Tertiary terrain (in particular) to a head, especially in Hikuwai and Waimatā catchments. Furthermore, these recent storms triggered initiation of new large gully complexes in untreated gully-prone land in the Tapuaeroa (Waiapu), as a consequence of complex land ownership issues and inability of local government to enforce revegetation strategies.
In these endeavours, it pays to listen to the river and learn from it. Geomorphic river stories express catchment-specific understandings that help efforts to work with the river and its connectivity relationships, applying process-based insights that manage at source and at scale, and in a timely manner. Moves towards enhanced catchment management programmes require strong leadership at national government and local levels to support implementation of geomorphologically-informed remediation strategies. To date, piecemeal efforts with insufficient resources have failed to achieve stipulated targets/goals. Accordingly, current practices are barely keeping pace with the rate at which the erosion problem is expanding (the net area affected by gully erosion has only been reduced by 5% in 60-years, Marden and Seymour, 2022). Meanwhile, irrespective of future land use decisions, soil loss from increasing instances of landsliding will result in reduced productivity. Attitudinal shift and improved funding mechanisms are required to facilitate land use change on a large scale and at a greater rate than has been possible in the past, but this time taking into account learnings from past mistakes. Such principles relate geomorphic understandings to issues of social and environmental justice in the Anthropocene, striving to ensure that efforts to minimise harm and maximise geo-ecological, socio-economic and cultural benefits through strategic, cost-effective measures avoid negative off-site impacts and legacy effects, rather than simply transferring problems elsewhere (in space or time).
All authors listed have made a substantial, direct, and intellectual contribution to the work and approved it for publication.
We acknowledge the support of local Tairāwhiti communities in assisting with original work underpinning this review. Helpful comments from two reviewers supported revisions to this paper.
The authors declare that the research was conducted in the absence of any commercial or financial relationships that could be construed as a potential conflict of interest.
The handling editor MT declared a past co-authorship with the author ICF.
All claims expressed in this article are solely those of the authors and do not necessarily represent those of their affiliated organizations, or those of the publisher, the editors and the reviewers. Any product that may be evaluated in this article, or claim that may be made by its manufacturer, is not guaranteed or endorsed by the publisher.
Allsop, F. (1973). The story of Mangatu: The forest which healed the land. Government Printer: AR Shearer.
Beechie, T. J., Sear, D. A., Olden, J. D., Pess, G. R., Buffington, J. M., Moir, H., et al. (2010). Process-based principles for restoring river ecosystems. BioScience 60 (3), 209–222. doi:10.1525/bio.2010.60.3.7
Berryman, K., Marden, M., Eden, D., Mazengarb, C., Ota, Y., and Moriya, I. (2000). Tectonic and paleoclimatic significance of quaternary river terraces of the Waipaoa River, East Coast, north island, New Zealand. N. Z. J. Geol. Geophys. 43 (2), 229–245. doi:10.1080/00288306.2000.9514883
Betts, H. D., Trustrum, N. A., and Rose, R. C. D. (2003). Geomorphic changes in a complex gully system measured from sequential digital elevation models, and implications for management. Earth Surf. Process. Landforms 28, 1043–1058. doi:10.1002/esp.500
Bilderback, E. L., Pettinga, J. R., Litchfield, N. J., Quigley, M., Marden, M., Roering, J. J., et al. (2015). Hillslope response to climate-modulated river incision in the Waipaoa catchment, East Coast north island, New Zealand. Geol. Soc. Am. Bull. 127, 131–148. doi:10.1130/b31015.1
Brierley, G. J., and Fryirs, K. (2000). River styles, a geomorphic approach to catchment characterization: Implications for river rehabilitation in Bega catchment, New South Wales, Australia. Environ. Manag. 25, 661–679. doi:10.1007/s002670010052
Brierley, G., and Fryirs, K. (2009). Don’t fight the site: Three geomorphic considerations in catchment-scale river rehabilitation planning. Environ. Manag. 43, 1201–1218. doi:10.1007/s00267-008-9266-4
Brierley, G. J., and Fryirs, K. A. (2016). The use of evolutionary trajectories to guide ‘moving targets’ in the management of river futures. River Res. Appl. 32, 823–835. doi:10.1002/rra.2930
Brierley, G., and Fryirs, K. (2022). Truths of the Riverscape: Moving beyond command-and-control to geomorphologically informed nature-based river management. Geosci. Lett. 9, 14–26. doi:10.1186/s40562-022-00223-0
Brierley, G., Fryirs, K., Reid, H., and Williams, R. (2021). The dark art of interpretation in geomorphology. Geomorphology 390, 107870. doi:10.1016/j.geomorph.2021.107870
Brierley, G., Fuller, I., Williams, G., Hikuroa, D., and Tilley, A. (2022). Re-imagining wild rivers in Aotearoa New Zealand. Land 11 (8), 1272. doi:10.3390/land11081272
Brierley, G. J., Hikuroa, D., Fuller, I. C., Tunnicliffe, J., Allen, K., Brasington, J., et al. (2023). Reanimating the strangled rivers of Aotearoa New Zealand. Water: Wiley Interdisciplinary Reviews, e1624.
Brooks, A. P., and Brierley, G. J. (1997). Geomorphic responses of lower Bega River to catchment disturbance, 1851–1926. Geomorphology 18 (3-4), 291–304. doi:10.1016/s0169-555x(96)00033-5
Brooks, A. P., Brierley, G. J., and Millar, R. G. (2003). The long-term control of vegetation and woody debris on channel and flood-plain evolution: Insights from a paired catchment study in southeastern Australia. Geomorphology 51 (1-3), 7–29. doi:10.1016/s0169-555x(02)00323-9
Brown, A., Toms, P., Carey, C., and Rhodes, E. (2013). Geomorphology of the Anthropocene: Time-transgressive discontinuities of human-induced alluviation. Anthropocene 1, 3–13. doi:10.1016/j.ancene.2013.06.002
Castree, N. (2014). The Anthropocene and geography I: The back story. Geogr. Compass 8 (7), 436–449. doi:10.1111/gec3.12141
Cerovski-Darriau, C., and Roering, J. J. (2016). Influence of anthropogenic land-use change on hillslope erosion in the Waipaoa River Basin, New Zealand. Earth Surf. Process. Landforms 41, 2167–2176. doi:10.1002/esp.3969
Cook, M. E., Brook, M. S., Tunnicliffe, J., Cave, M., and Gulick, N. P. (2022). Preliminary investigation of emerging suburban landsliding in Gisborne, New Zealand. Q. J. Eng. Geol. Hydrogeology 55. doi:10.1144/qjegh2021-087
Coombes, B. (2000). Ecological impacts and planning history: An environmental history of the turanganui casebook area Waitangi tribunal Wai 814 #A20.
Crosby, B. T., and Whipple, K. X. (2006). Knickpoint initiation and distribution within fluvial networks: 236 waterfalls in the Waipaoa River, north island, New Zealand. Geomorphology 82 (1-2), 16–38. doi:10.1016/j.geomorph.2005.08.023
Davis, W. M. (1906). The geographical cycle in an arid climate. Geogr. J. 27 (1), 70–73. doi:10.2307/1776794
De Boer, J. A., Thoms, M. C., Delong, M. D., Parsons, M. E., and Casper, A. F. (2020). Heterogeneity of ecosystem function in an “Anthropocene” river system. Anthropocene 31, 100252. doi:10.1016/j.ancene.2020.100252
De Rose, R. C., and Basher, L. R. (2011). Measurement of river bank and cliff erosion from sequential LIDAR and historical aerial photography. Geomorphology 126, 132–147. doi:10.1016/j.geomorph.2010.10.037
De Rose, R., Gomez, B., Marden, M., and Trustrum, N. (1998). Gully erosion in Mangatu Forest, New Zealand, estimated from digital elevation models. Earth Surf. Process. Landforms 23, 1045–1053. doi:10.1002/(sici)1096-9837(1998110)23:11<1045::aid-esp920>3.0.co;2-t
Ekanayake, J. C., Marden, M., Watson, A. J., and Rowan, D. (1997). Tree roots and slope stability: A comparison between Pinus radiata and kanuka. N. Z. J. For. Sci. 27, 216–233.
Ewers, R. M., Kliskey, A. D., Walker, S., Rutledge, D., Harding, J. S., and Didham, R. K. (2006). Past and future trajectories of forest loss in New Zealand. Biol. Conserv. 133, 312–325. doi:10.1016/j.biocon.2006.06.018
Fryirs, K. A., and Brierley, G. J. (2016). Assessing the geomorphic recovery potential of rivers: Forecasting future trajectories of adjustment for use in management. Wiley Interdiscip. Rev. Water 3 (5), 727–748. doi:10.1002/wat2.1158
Fryirs, K., and Brierley, G. J. (2001). Variability in sediment delivery and storage along river courses in bega catchment, NSW, Australia: Implications for geomorphic river recovery. Geomorphology 38 (3-4), 237–265. doi:10.1016/s0169-555x(00)00093-3
Fryirs, K., and Brierley, G. (2021). How far have management practices come in ‘working with the river. Earth Surf. Process. Landforms 46 (15), 3004–3010. doi:10.1002/esp.5279
Fryirs, K. A., Brierley, G. J., Preston, N. J., and Kasai, M. (2007). Buffers, barriers and blankets: The (dis) connectivity of catchment-scale sediment cascades. Catena 70 (1), 49–67. doi:10.1016/j.catena.2006.07.007
Fryirs, K., Hancock, F., Healey, M., Mould, S., Dobbs, L., Riches, M., et al. (2021). Things we can do now that we could not do before: Developing and using a cross-scalar, state-wide database to support geomorphologically-informed river management. PloS one 16 (1), e0244719. doi:10.1371/journal.pone.0244719
Fuller, I. C., and Marden, M. (2010). Rapid channel response to variability in sediment supply: Cutting and filling of the Tarndale Fan, Waipaoa catchment, New Zealand. Mar. Geol. 270, 45–54. doi:10.1016/j.margeo.2009.10.004
Fuller, I. C., and Marden, M. (2011). Slope–channel coupling in steepland terrain: A field-based conceptual model from the Tarndale gully and fan, Waipaoa catchment, New Zealand. Geomorphology 128, 105–115. doi:10.1016/j.geomorph.2010.12.018
Fuller, I. C., and Rutherfurd, I. D. (2021). “Geomorphic responses to anthropogenic land-cover change in Australia and New Zealand,”. Treatise on geomorphology. Editor J. J. F. Shroder (Elsevier, Academic Press), 9, 584–619.
Fuller, I. C., Macklin, M. G., and Richardson, J. M. (2015). The geography of the Anthropocene in N ew Z ealand: Differential river catchment response to human impact. Geogr. Res. 53, 255–269. doi:10.1111/1745-5871.12121
Fuller, I. C., Strohmaier, F., McColl, S. T., Tunnicliffe, J., and Marden, M. (2020). Badass gully morphodynamics and sediment generation in Waipaoa Catchment, New Zealand. Earth Surf. Process. Landforms 45, 3917–3930. doi:10.1002/esp.5010
García, J. H., Ollero, A., Ibisate, A., Fuller, I. C., Death, R. G., and Piégay, H. (2021). Promoting fluvial geomorphology to “live with rivers” in the Anthropocene Era. Geomorphology 380, 107649. doi:10.1016/j.geomorph.2021.107649
GDC (2023). Te mahinga arai waipuke o Waipaoa Waipaoa flood control. Available at: https://www.gdc.govt.nz/council/major-projects/waipaoa-river-flood-control-scheme (Accessed March 16, 2023).
Gibbs, G. (2006). Ghosts of Gondwana: The history of life in New Zealand. Nelson, New Zealand: Craig Potton Publishing, 232.
Gomez, B., and Livingston, D. M. (2012). The river it goes right on: Post-glacial landscape evolution in the upper Waipaoa River basin, eastern North Island, New Zealand. Geomorphology 159, 73–83. doi:10.1016/j.geomorph.2012.03.006
Gomez, B., Eden, D. N., Peacock, D. H., and Pinkney, E. J. (1998). Floodplain construction by recent, rapid vertical accretion: Waipaoa River, New Zealand. Earth Surf. Process. Landforms 23, 405–413. doi:10.1002/(sici)1096-9837(199805)23:5<405::aid-esp854>3.0.co;2-x
Gomez, B., Rosser, B. J., Peacock, D. H., Hicks, D. M., and Palmer, J. A. (2001). Downstream fining in a rapidly aggrading gravel bed river. Water Resour. Res. 37, 1813–1823. doi:10.1029/2001wr900007
Gomez, B., Coleman, S., Sy, V., Peacock, D., and Kent, M. (2007). Channel change, bankfull and effective discharges on a vertically accreting, meandering, gravel-bed river. Earth Surf. Process. Landforms 32, 770–785. doi:10.1002/esp.1424
Gomez, B., Cui, Y., Kettner, A., Peacock, D., and Syvitski, J. (2009). Simulating changes to the sediment transport regime of the Waipaoa River, New Zealand, driven by climate change in the twenty-first century. Glob. Planet. Change 67, 153–166. doi:10.1016/j.gloplacha.2009.02.002
Gomez, B., Trustrum, N., Hicks, D., Rogers, K., Page, M., and Tate, K. (2003). Production, storage, and output of particulate organic carbon: Waipaoa River basin, New Zealand. Water Resour. Res. 39, 1161. doi:10.1029/2002wr001619
Hamilton, D., and Kelman, E. (1952). Soil conservation survey of the Waipaoa River catchment, poverty Bay-New Zealand. Wellington, New Zealand: New Zealand Soil Conservation and Rivers. Unpublished Report.
Harvey, K. C. (2021). Integrating process based assessments of river sensitivity through river styles and semi-quantitative sediment connectivity modelling to inform landuse management in the Waimatā catchment, Gisborne, New Zealand. Unpublished MSc thesis. Auckland: School of Environment, Faculty of Science, The University of Auckland.
Hayman, E., James, C., and Wedge, M. (2018). Future rivers of the Anthropocene or whose Anthropocene is it? Decolonising the Anthropocene. Decolonization Indig. Educ. Soc. 7 (1), 77–92.
Henderson, J., and Ongley, M. (1920). The Geology of the Gisborne and Whatatutu subdivisions, raukumara division. Bulletin 21. New Zealand: Geological Survey Branch. Department of Mines.
Herzig, A., Dymond, J. R., and Marden, M. (2011). A gully-complex model for assessing gully stabilisation strategies. Geomorphology 133, 23–33. doi:10.1016/j.geomorph.2011.06.012
Hicks, D. (1995). A way to estimate the frequency of rainfall-induced mass movements (note). J. Hydrology (New Zealand) 33, 59–67.
Hicks, D. M., and Shankar, U. (2003). Sediment from New Zealand rivers, NIWA Chart. Miscellaneous Series No. 79.
Hicks, D. M., Gomez, B., and Trustrum, N. A. (2000). Erosion thresholds and suspended sediment yields, Waipaoa River Basin, New Zealand. Water Resour. Res. 36, 1129–1142. doi:10.1029/1999WR900340
Hikuroa, D., Brierley, G., Tadaki, M., Blue, B., and Salmond, A. (2021)“Restoring sociocultural relationships with rivers,” in River Restoration: Political Social and Economic Perspectives (New York, United States: John Wiley & Sons), 66–88. doi:10.1002/9781119410010.ch3
Jones, K. E., and Preston, N. J. (2012). Spatial and temporal patterns of off-slope sediment delivery for small catchments subject to shallow landslides within the Waipaoa catchment, New Zealand. Geomorphology 141, 150–159. doi:10.1016/j.geomorph.2011.12.037
Kasai, M. (2006). Channel processes following land use changes in a degrading steep headwater stream in North Island, New Zealand. Geomorphology 81, 421–439. doi:10.1016/j.geomorph.2005.11.014
Kasai, M., Brierley, G. J., Page, M. J., Marutani, T., and Trustrum, N. A. (2005). Impacts of land use change on patterns of sediment flux in Weraamaia catchment, New Zealand. Catena 64, 27–60. doi:10.1016/j.catena.2005.06.014
Knight, C. (2016). New Zealand's rivers: An environmental history. Christchurch: Canterbury University Press, 323.
Knox, J. C. (1977). Human impacts on Wisconsin stream channels. Ann. Assoc. Am. Geogr. 67, 323–342. doi:10.1111/j.1467-8306.1977.tb01145.x
Lambie, S. M., Awatere, S., Daigneault, A., Kirschbaum, M. U. F., Marden, M., Soliman, T., et al. (2021). Trade-offs between environmental and economic factors in conversion from exotic pine production to natural regeneration on erosion prone land. N. Z. J. For. Sci. 51, 1–15. doi:10.33494/nzjfs512021x163x
Large, A., Gilvear, D., and Starkey, E. (2022). “Ecosystem service-based approaches for status assessment of Anthropocene riverscapes,” in Rivers of the Anthropocene. Editors J. M. Kelly, P. V. Scarpino, H. Berry, J. Syvitski, and M. Meybeck (University of California Press), 23–42.
Leathwick, J. R., Clarkson, B. D., Burns, B. R., Innes, J. G., and Smale, M. C. (1995). “Waiapu Ecological District: Survey report for the Protected Natural Areas Programme,” in New Zealand Protected Natural Areas Programme (Gisborne: Department of Conservation).
Leenman, A., and Tunnicliffe, J. (2018). Genesis of a major gully mass-wasting complex, and implications for valley filling, East Cape, New Zealand. Geol. Soc. Am. Bull. 130, 1121–1130. doi:10.1130/b31849.1
Leenman, A., and Tunnicliffe, J. (2020). Tributary-junction fans as buffers in the sediment cascade: A multi-decadal study. Earth Surf. Process. Landforms 45, 265–279. doi:10.1002/esp.4717
Leighton, A., Brook, M. S., Cave, M., Rowe, M. C., Stanley, A., and Tunnicliffe, J. F. (2022). Engineering geomorphological reconnaissance of the December 2018 Waimata Valley mud volcano eruption, Gisborne, New Zealand. Q. J. Eng. Geol. Hydrogeology 55 (4), qjegh2021–149. doi:10.1144/qjegh2021-149
Litchfield, N., and Berryman, K. (2006). Relations between postglacial fluvial incision rates and uplift rates in the North Island, New Zealand. J. Geophys. Res. Earth Surf. 111 (F2), F02007. doi:10.1029/2005jf000374
Litchfield, N. J., Clark, K. J., Cochran, U. A., Palmer, A. S., Mountjoy, J., Mueller, C., et al. (2020). Marine terraces reveal complex near-shore upper-plate faulting in the northern Hikurangi margin, New Zealand. Bull. Seismol. Soc. Am. 110, 825–849. doi:10.1785/0120190208
Macklin, M. G., and Lewin, J. (2003). River sediments, great floods and centennial-scale Holocene climate change. J. Quat. Sci. 18, 101–105. doi:10.1002/jqs.751
Malhi, Y. (2017). The concept of the Anthropocene. Annu. Rev. Environ. Resour. 42, 77–104. doi:10.1146/annurev-environ-102016-060854
Marden, M. (2012). Effectiveness of reforestation in erosion mitigation and implications for future sediment yields, East Coast catchments, New Zealand: A review. N. Z. Geogr. 68, 24–35. Available:. doi:10.1111/j.1745-7939.2012.01218.x
Marden, M., and Seymour, A. (2022). Effectiveness of vegetative mitigation strategies in the restoration of fluvial and fluvio-mass movement gully complexes over 60 years, East Coast region, North Island, New Zealand. N. Z. J. For. Sci. 52. doi:10.33494/nzjfs522022x226x
Marden, M., Herzig, A., and Arnold, G. (2011). Gully degradation, stabilisation and effectiveness of reforestation in reducing gully-derived sediment, East Coast region, North Island, New Zealand. J. Hydrology (New Zealand) 50, 19–36.
Marden, M., Herzig, A., and Basher, L. (2014). Erosion process contribution to sediment yield before and after the establishment of exotic forest: Waipaoa catchment, New Zealand. Geomorphology 226, 162–174. doi:10.1016/j.geomorph.2014.08.007
Marden, M., Lambie, S., and Phillips, C. (2020). Potential effectiveness of low-density plantings of manuka (Leptospermum scoparium) as an erosion mitigation strategy in steeplands, northern Hawke’s Bay, New Zealand. N. Z. J. For. Sci. 50. doi:10.33494/nzjfs502020x82x
Marden, M., Rowan, D., and Watson, A. (2023). Effect of changes in forest water balance and inferred root reinforcement on landslide occurrence and sediment generation following Pinus radiata harvest on Tertiary terrain, eastern North Island, New Zealand. N. Z. J. For. Sci. 53. doi:10.33494/nzjfs532023x216x
Marden, M., Arnold, G., Gomez, B., and Rowan, D. (2005). Pre-and post-reforestation gully development in Mangatu forest, East Coast, north island, New Zealand. River Res. Appl. 21, 757–771. doi:10.1002/rra.882
Marden, M., Betts, H., Arnold, G., and Hambling, R. (2008). Gully erosion and sediment load: Waipaoa, Waiapu and uawa rivers, eastern north island, New Zealand. Sediment Dyn. Changing Environ., 339–350.
Marden, M., Arnold, G., Seymour, A., and Hambling, R. (2012). History and distribution of steep-land gullies in response to land use change, East Coast Region, North Island, New Zealand. Geomorphology 153–154, 81–90. doi:10.1016/j.geomorph.2012.02.011
Marden, M., Fuller, I. C., Herzig, A., and Betts, H. D. (2018). Badass gullies: Fluvio-mass-movement gully complexes in New Zealand's East Coast region, and potential for remediation. Geomorphology 307, 12–23. doi:10.1016/j.geomorph.2017.11.012
Marsh, G. P. (1864). Man and nature, or physical Geography as Modified by human Action by george P. Marsh, sampson low, son and marston.
Mazengarb, C., and Speden, I. G. (2000). “Geology of the Raukumara area,” in Institute of Geological and Nuclear Sciences 1:250 000 geological map 6 (Lower Hutt, NZ: Institute of Geological and Nuclear Sciences), 1 sheet + 60.
Meybeck, M., Bouleau, G., Carré, C., Garnier, J., and Lestel, L. (2023). Rivers help us to quantify the socio-ecological functioning of their basin at the Anthropocene: the Seine example (1850–2020). Comptes Rendus. Géosci., 1–19. doi:10.5802/crgeos.140
Ministry for Environment. 2020. essential-freshwater-te-mana-o-te-wai-factsheet. Available at: https://environment.govt.nz/publications/essential-freshwater-te-mana-o-te-wai-factsheet/. (Accessed 14 March 2023).
Orpin, A. R., Alexander, C., Carter, L., Kuehl, S., and Walsh, J. P. (2006). Temporal and spatial complexity in post-glacial sedimentation on the tectonically active, Poverty Bay continental margin of New Zealand. Cont. Shelf Res. 26 (17-18), 2205–2224. doi:10.1016/j.csr.2006.07.029
Page, M., Harmsworth, G., Trustrum, N., Kasai, M., and Marutani, T. (2001). “Waiapu River (north island, New Zealand),” in Source-to-Sink sedimentary Cascades in Pacific Rim geo-systems. Matsumoto sabo work office. Editors T. Marutani, G. J. Brierley, N. A. Trustrum, and M. Page (Matsumoto, Nagano, Japan: Ministry of Land, Infrastructure and Transport), 102–111.
Page, M., Marden, M., Kasai, M., Gomez, B., Peacock, D., Betts, H., et al. (2007). Changes in basin-scale sediment supply and transfer in a rapidly transformed New Zealand landscape. Dev. Earth Surf. Process. 11, 337–356.
Parkner, T., Page, M., Marden, M., and Marutani, T. (2007). Gully systems under undisturbed indigenous forest, East Coast region, New Zealand. Geomorphology 84, 241–253. doi:10.1016/j.geomorph.2006.01.042
Parsons, M., Fisher, K., and Crease, R. P. (2021). Decolonising blue spaces in the Anthropocene: Freshwater management in Aotearoa New Zealand. Book publisher: Palgrave Macmillan, Springer, Cham, Switzerland.
Phillips, C., Marden, M., and Pearce, A. (1990). Effectiveness of reforestation in prevention and control of landsliding during large cyclonic storms. Proc. Int. Union For. Res. Organ. XIX World Congr., 340–350.
Phillips, J. D. (2015). Badass geomorphology. Earth Surf. Process. Landforms 40, 22–33. doi:10.1002/esp.3682
Piégay, H., Chabot, A., and Le Lay, Y. F. (2020). Some comments about resilience: From cyclicity to trajectory, a shift in living and nonliving system theory. Geomorphology 367, 106527. doi:10.1016/j.geomorph.2018.09.018
Poeppl, R. E., Fryirs, K. A., Tunnicliffe, J., and Brierley, G. J. (2020). Managing sediment (dis)connectivity in fluvial systems. Sci. Total Environ. 736, 139627. doi:10.1016/j.scitotenv.2020.139627
Reid, H. E., and Brierley, G. J. (2015). Assessing geomorphic sensitivity in relation to river capacity for adjustment. Geomorphology 251, 108–121. doi:10.1016/j.geomorph.2015.09.009
Reid, L. M., and Page, M. J. (2003). Magnitude and frequency of landsliding in a large New Zealand catchment. Geomorphology 49, 71–88. doi:10.1016/s0169-555x(02)00164-2
Richardson, J., Fuller, I., Holt, K., Litchfield, N., and Macklin, M. (2014). Rapid post-settlement floodplain accumulation in Northland, New Zealand. Catena 113, 292–305. doi:10.1016/j.catena.2013.08.013
Rosser, B. J., and Jones, K. E. (2021). Application of LiDAR differencing to assess sediment load in the Upper Waipaoa River, 2005 to 2019 (Lower Hutt, NZ: GNS Science), 51 Consultancy Report 2021/102.
Rosser, B. J., Ashraf, S., and Dellow, S. (2019). “Assessment of the use of differencing satellite imagery as a tool for quantifying landslide impacts from significant storms - a case study in the Uawa catchment,” in Tolaga Bay (Lower Hutt, NZ: GNS Science), 51 Consultancy Report 2019/93.
Salmond, A. (2014). Tears of rangi: Water, power, and people in New Zealand. HAU J. Ethnogr. Theory 4, 285–309. doi:10.14318/hau4.3.017
Salmond, A., Brierley, G., and Hikuroa, D. (2019). Let the rivers speak: Thinking about waterways in Aotearoa New Zealand. Policy Q. 15 (3). doi:10.26686/pq.v15i3.5687
Salmond, A., Brierley, G., Hikuroa, D., and Lythberg, B. (2022). Tai timu, tai pari, the ebb and flow of the tides: Working with the Waimatā from the mountains to the Sea. N. Z. J. Mar. Freshw. Res. 56, 430–446. doi:10.1080/00288330.2022.2096084
Simon, A. (1989). A model of channel response in disturbed alluvial channels. Earth Surf. Process. landforms 14 (1), 11–26. doi:10.1002/esp.3290140103
Simon, A., and Rinaldi, M. (2006). Disturbance, stream incision, and channel evolution: The roles of excess transport capacity and boundary materials in controlling channel response. Geomorphology 79 (3-4), 361–383. doi:10.1016/j.geomorph.2006.06.037
Spedding, M. (2006). The Tūranganui River: A brief history. Wellington, NZ: Department of Conservation.
Steffen, W., Crutzen, P. J., and McNeill, J. R. (2007). The Anthropocene: Are humans now overwhelming the great forces of nature. AMBIO A J. Hum. Environ. 36, 614–621. doi:10.1579/0044-7447(2007)36[614:taahno]2.0.co;2
Stewart-Harawira, M. W. (2020). Troubled waters: Maori values and ethics for freshwater management and New Zealand's fresh water crisis. Wiley Interdiscip. Rev. Water 7, e1464. doi:10.1002/wat2.1464
Taylor, R. J., Massey, C., Fuller, I. C., Marden, M., Archibald, G., and Ries, W. (2018). Quantifying sediment connectivity in an actively eroding gully complex, Waipaoa catchment, New Zealand. Geomorphology 307, 24–37. doi:10.1016/j.geomorph.2017.10.007
Te Aho, L. (2019). Te Mana o te Wai: An indigenous perspective on rivers and river management. River Res. Appl. 35 (10), 1615–1621. doi:10.1002/rra.3365
Thoms, M., Rose, T., and Dyer, F. (2020). Riverine landscapes, water resource development and management: A view from downunder. River Res. Appl. 36, 505–511. doi:10.1002/rra.3622
Tunnicliffe, J., Brierley, G., Fuller, I. C., Leenman, A., Marden, M., and Peacock, D. (2018). Reaction and relaxation in a coarse-grained fluvial system following catchment-wide disturbance. Geomorphology 307, 50–64. doi:10.1016/j.geomorph.2017.11.006
Vale, S. S., Smith, H. G., and Marden, M. (2021). Upper Mōtū catchment sediment sources study. Palmerston North: Manaaki Whenua - Landcare Research. (Contract Report LC3937).
Walley, Y., Tunnicliffe, J., and Brierley, G. (2018). The influence of network structure upon sediment routing in two disturbed catchments, East Cape, New Zealand. Geomorphology 307, 38–49. doi:10.1016/j.geomorph.2017.10.029
Wasson, R., Mazari, R., Starr, B., and Clifton, G. (1998). The recent history of erosion and sedimentation on the southern tablelands of southeastern Australia: Sediment flux dominated by channel incision. Geomorphology 24, 291–308. doi:10.1016/s0169-555x(98)00019-1
Wheaton, J. M., Bennett, S., Bouwes, N., Maestas, J. D., and Shahverdian, S. M. (2019). Low-tech process-based restoration of riverscapes: Design manual. Logan, UT: Utah State University Restoration Consortium. Version 1.0.
Wilkinson, C., Hikuroa, D. C., Macfarlane, A. H., and Hughes, M. W. (2020). Mātauranga Māori in geomorphology: Existing frameworks, case studies, and recommendations for incorporating indigenous knowledge in earth science. Earth Surf. Dyn. 8 (3), 595–618. doi:10.5194/esurf-8-595-2020
Wilmshurst, J. M., Eden, D. N., and Froggatt, P. C. (1999). Late holocene forest disturbance in Gisborne, New Zealand: A comparison of terrestrial and marine pollen records. N. Z. J. Bot. 37, 523–540. doi:10.1080/0028825X.1999.9512651
Wohl, E., Bledsoe, B. P., Jacobson, R. B., Poff, N. L., Rathburn, S. L., Walters, D. M., et al. (2015). The natural sediment regime in rivers: Broadening the foundation for ecosystem management. BioScience 65, 358–371. doi:10.1093/biosci/biv002
Keywords: catchment transformation, catchment connectivity, river geomorphology, regime shift, sediment flux, recovery, river futures
Citation: Fuller IC, Brierley GJ, Tunnicliffe J, Marden M, McCord J, Rosser B, Hikuroa D, Harvey K, Stevens E and Thomas M (2023) Managing at source and at scale: The use of geomorphic river stories to support rehabilitation of Anthropocene riverscapes in the East Coast Region of Aotearoa New Zealand. Front. Environ. Sci. 11:1162099. doi: 10.3389/fenvs.2023.1162099
Received: 09 February 2023; Accepted: 21 March 2023;
Published: 13 April 2023.
Edited by:
Martin Thoms, University of New England, AustraliaReviewed by:
Kimberly Meitzen, Texas State University, United StatesCopyright © 2023 Fuller, Brierley, Tunnicliffe, Marden, McCord, Rosser, Hikuroa, Harvey, Stevens and Thomas. This is an open-access article distributed under the terms of the Creative Commons Attribution License (CC BY). The use, distribution or reproduction in other forums is permitted, provided the original author(s) and the copyright owner(s) are credited and that the original publication in this journal is cited, in accordance with accepted academic practice. No use, distribution or reproduction is permitted which does not comply with these terms.
*Correspondence: Ian C. Fuller, aS5jLmZ1bGxlckBtYXNzZXkuYWMubno=
†These authors have contributed equally to this work and share first authorship
Disclaimer: All claims expressed in this article are solely those of the authors and do not necessarily represent those of their affiliated organizations, or those of the publisher, the editors and the reviewers. Any product that may be evaluated in this article or claim that may be made by its manufacturer is not guaranteed or endorsed by the publisher.
Research integrity at Frontiers
Learn more about the work of our research integrity team to safeguard the quality of each article we publish.