- 1Leibniz Centre for Agricultural Landscape Research (ZALF), Müncheberg, Germany
- 2Warsaw University of Life Sciences (SGGW), Department of Soil Science, Warsaw, Poland
For the determination of silicon (Si) contents in plant materials several methods are in use, but as all of these methods exhibit specific advantages and drawbacks, none of them has become prevalent. Alkaline chemicals are widely used to extract Si from plant samples, because this method is comparably simple, fast, and cheap. However, a comprehensive method comparison between different alkaline extractions to different plant organs is still missing, as well as understanding of the relationship between Si content quantified with those methods and soil silicon availability. In our study we performed two different alkaline (sodium carbonate (Na2CO3) and Tiron) extractions to determine Si contents in husk and straw samples of winter wheat (Triticum aestivum). Results obtained from hydrofluoric (HF) acid digestion were used as reference as this method is assumed to completely dissolve Si in a plant material. We evaluated the efficacy of simple, single-step Na2CO3 and Tiron extractions in relation to complex, multistep HF extractions and analyzed the relationships between Si and phytolith contents in plant samples and plant available Si concentrations in corresponding soil samples. Irrespective of the plant material used (husk or straw) we found Tiron to be more efficient in Si extraction compared to Na2CO3. While Na2CO3 extractions systematically underestimated Si contents in the tested plant materials, the results of Tiron extractions were comparable to the results of HF digestions, at least for husk samples. Phytolith contents in plant samples were strongly positively correlated to Si contents obtained from extractions, especially in husk samples. Plant available Si in soil samples was also reflected best in husk Si contents. Based on our results we recommend the use of Tiron for the extraction of Si from plant materials as this method provided more reliable results compared to the Na2CO3 extraction.
1 Introduction
Biosilicification, i.e., the incorporation of inorganic silicon (Si) into living organisms in the form of amorphous, hydrated silica (= biogenic silica, SiO2·nH2O), by various organisms like protists, sponges, and plants has been found to represent a key factor in terrestrial Si cycling (reviewed by Schaller et al., 2021). Biogenic silica precipitates in plants are called phytogenic silica or phytoliths, which also contain organic matter and various elements like aluminum, calcium, iron, manganese, and phosphorus (see Puppe et al., 2022a and references therein). Si accumulation in plants has been shown to enhance their resilience against abiotic [e.g., water stress or metal(loid) toxicity] and biotic (fungal infections or herbivore damage) stressors with consequences for ecosystem functioning (reviewed by Katz et al., 2021).
In agricultural plant-soil systems Si fertilizers are widely used to increase yields of Si accumulating crops like rice, maize, wheat, and sugarcane (reviewed by Haynes, 2017; Savant et al., 1999). Crops’ need for Si supply and Si fertilization efficacy can be evaluated by analyzing Si contents in plant materials. For the determination of Si contents in plants several methods are in use (see Snyder, 2001 and references therein). These comprise i) single-step extractions with alkaline extractants like sodium carbonate (Na2CO3) (Meunier et al., 2014; Clymans et al., 2016), sodium hydroxide (NaOH) (Barão et al., 2015; Clymans et al., 2016), or Tiron (disodium 4,5-dihydroxy-1,3-benzenedisulfonate, C6H4Na2O8S2) (Guntzer et al., 2010; Meunier et al., 2014), and ii) multistep digestions using lithium borate (LiBO2, Li2B4O7) (Alexandre et al., 1997; Nakamura et al., 2020) or hydrofluoric (HF) acid (Sommer et al., 2013; Wehrhan et al., 2021). In addition to these chemical procedures, X-ray fluorescence (XRF) spectrometry has been used for Si content measurements in plant materials (e.g., Evans, 1970; Queralt et al., 2005; Wade et al., 2022). In general, all of these methods exhibit specific advantages and drawbacks, which is why none of these methods has become prevalent. For example, alkaline extractions are destructive, but relatively simple, fast, and cheap. Digestion based methods, which are also destructive, depend on hazardous chemicals and are relatively time-consuming. XRF represents a nondestructive method, but is dependent on a relatively expensive instrumentation and might be hampered by relatively high limits of detection.
The alkaline extractions mentioned above were originally introduced to analyze biogenic silica in marine sediments (Na2CO3/NaOH, see DeMaster, 1981) or to quantify amorphous aluminum, iron, and Si oxides simultaneously in soils (Tiron, see Biermans and Baert, 1977). In this context, it should be noted that the selective extraction of biogenic silica from sediment or soil samples is complicated by the fact that these samples are almost always dominated by inorganic silicates. And although correction methods are used to determine biogenic silica contents in sediment or soil samples, different alkaline extractants can lead to largely differing results (see, e.g., Zhu et al., 2023). In contrast, pure plant samples solely contain biogenic (phytogenic) silica making extractions less complicated. However, although alkaline extractions are widely used to determine Si contents in plant materials nowadays, studies on their efficacy are surprisingly rare. Actually, there are only very few studies published that analyzed the comparability of results derived from different Si determination methods (Guntzer et al., 2010; Meunier et al., 2014; Nakamura et al., 2020), although an accurate quantification of Si contents in plant materials is crucial for assessing the Si status of an agricultural plant-soil system. Interestingly, the previous method comparison studies focused on shoot or leaf samples of different plant species only (Guntzer et al., 2010; Meunier et al., 2014; Nakamura et al., 2020), but did not test different plant materials of a specific plant species simultaneously. However, as Si accumulation can largely differ between plant parts (e.g., Puppe et al., 2021), it is of interest to examine if these differences influence the efficacy of Si extractions.
Moreover, the studies of Guntzer et al. (2010), Meunier et al. (2014), and Nakamura et al. (2020) did not examine potential interactions between extracted Si contents in plants and concentrations of plant available Si in soils. Nevertheless, this interaction is of great interest, as soil Si availability is often deduced from plant Si contents and vice versa in agronomic studies (e.g., Korndörfer et al., 2001; Miles et al., 2014; Wu et al., 2020). In addition, the quantification of Si pools and fluxes in (agricultural) plant-soil systems is also crucial from an agro-ecological point of view. This is because humans largely affect global Si cycling caused by huge Si losses from intensively used land (e.g., Struyf et al., 2010; Vandevenne et al., 2012). Recently, about 35% of total biogenic silica in plants is accumulated in field crops and this proportion is going to increase with increasing agricultural production in the future (Carey and Fulweiler, 2016). Thus, sustainable strategies to overcome anthropogenic desilication are badly needed. In this context, the recycling of organic siliceous materials like straw, husk, and husk ash has been found to represent a promising and cost effective strategy to simultaneously mitigate anthropogenic desilication and improve plant performance (e.g., Meena et al., 2014; Marxen et al., 2016; Puppe et al., 2021).
In our study we focused on two alkaline extractants, namely, Na2CO3 and Tiron, whose suitability for the determination of Si contents in plant materials has already been evaluated in previous studies, albeit with relatively small sample sets (Guntzer et al., 2010; Nakamura et al., 2020). Meunier et al. (2014) analyzed the potential of the Na2CO3 extraction for the quantification of phytolith pools. In their study they also compared their results with the results of Tiron extractions carried out by Guntzer et al. (2010) using fresh (ground shoot samples) and aged (soil) phytoliths. However, the corresponding correlation between Na2CO3 and Tiron results was based on four data points only (cf. Figure 1 in Meunier et al., 2014), hence a comprehensive comparison between these two methods is still missing. We performed single-step Na2CO3 and Tiron extractions using a relatively large sample set (n = 42) of retained husk and straw samples of winter wheat (Triticum aestivum) and correlated the resulting Si contents with i) data on plant available Si concentrations in corresponding soil samples (data from Puppe et al., 2021) as well as ii) phytolith contents in the husk and straw samples. Previous results obtained from multistep HF digestion of the winter wheat samples analyzed in our study were used as reference (see Puppe et al., 2021). Our aims were i) to evaluate the efficacy of the two used alkaline extractants to extract Si from different organs of wheat and ii) to analyze the relationships between Si (Na2CO3 and Tiron extractions, HF digestion) and phytolith contents (ash extraction) in plant samples and plant available Si (calcium chloride extraction) in corresponding soil samples. Assessing the potential of single-step alkaline extractions for the determination of plant Si contents will be of interest for all researchers, who have a focus on biogeochemical Si cycling in terrestrial ecosystems, especially in agricultural plant-soil systems.
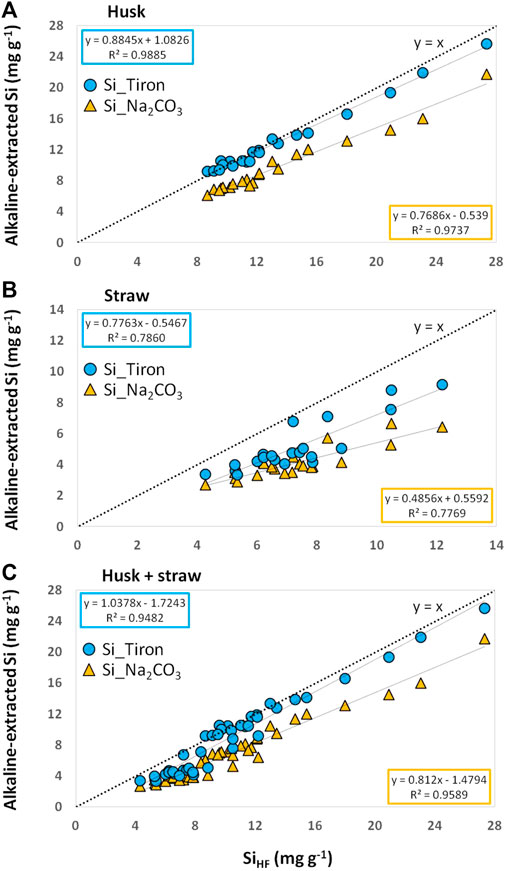
FIGURE 1. Alkaline-extracted Si contents in plant materials [husk (A), straw (B), and husk + straw (C) of winter wheat] plotted against corresponding total Si contents (SiHF). Linear trendlines are given in light blue and corresponding equations and coefficients of determination are stated in blue (Si_Tiron) and orange (Si_Na2CO3) boxes. Dotted lines represent the lines of equality (y = x).
2 Materials and methods
2.1 Plant and soil sampling
In total we used 42 retained winter wheat (T. aestivum, cultivar JB Asano, comprising 21 husk and 21 corresponding straw samples) and corresponding 21 soil samples for the current study. Winter wheat samples were taken in 2018 from an ongoing long-term field experiment in NE Germany (Puppe et al., 2021). Plant samples were washed to remove adhering soil minerals, oven-dried at 45°C for 48 h, and subsequently separated into straw, grain, and husk. The different plant materials of winter wheat were separately homogenized using a knife mill (Grindomix GM 200, Retsch) in two steps: i) for 1 min at 4,000 rpm and ii) for 3 min at 10,000 rpm. As grain samples showed only very low total Si contents (mean 0.2–0.4 mg g-1), we used solely straw (mean total Si 6.3–10.7 mg g-1) and husk (mean total Si 9.5–23.2 mg g-1) samples for our method comparison (total Si concentrations obtained from HF digestion, see Puppe et al., 2021). Soil samples were also taken in 2018 at the identical plots of plant sampling (see Puppe et al., 2021 for details) and were air dried and sieved (2 mm) previous to the extraction of plant available Si.
2.2 Analyses of Si in plant and soil samples
HF digestion was performed in the course of a previous study and the corresponding results can be found in Puppe et al. (2021). Plant sample aliquots of approximately 100 mg were digested under pressure in PFA (Perfluoroalkoxy alkane) digestion vessels using a mixture of 4 mL distilled water, 5 mL nitric acid (65%), and 1 mL HF acid (40%) at 190°C in a microwave digestion system (Mars 6, CEM). A second digestion step was used to neutralize HF acid with 10 mL of a 4% boric acid solution at 150°C. We used the results of HF digestion as a reference (= total Si in a plant sample) for the evaluation of the efficacy of the two alkaline (i.e., Na2CO3 and Tiron) Si extraction methods tested in our study.
For Na2CO3 extraction 30 mg of plant material were mixed with 30 mL of Na2CO3 (1%) and heated at 85°C for 5 h (DeMaster, 1981). Samples were gently shaken by hand directly before heating and subsequently every hour while heating, i.e., after 1, 2, 3, and 4 h in the water bath. Finally, the extracts were filtrated at a pore-size of 0.2 µm (Whatman NL 16).
The Tiron extraction followed the method developed by Biermans and Baert (1977) and modified by Kodama and Ross (1991). It has been used to quantify amorphous biogenic and pedogenic Si (Kendrick & Graham, 2004), although a partial dissolution of primary minerals is well known (Kodama & Ross, 1991; Sauer et al., 2006). The extraction solution was produced by dilution of 31.42 g Tiron with 800 mL of distilled water, followed by addition of 100 mL sodium carbonate solution (5.3 g Na2CO3 plus 100 mL distilled water) under constant stirring. The final pH of 10.5 was reached by adding small volumes of a 4 M NaOH solution. For the extraction, 30 mg of plant samples were weighed into 50 mL centrifuge tubes and a 30 mL aliquot of the Tiron solution was added. The tubes were then heated at 85°C in a water bath for 1 h. The samples were gently shaken by hand directly before heating and after 30 min in the heated water bath. Finally, the extracted solutions were centrifuged at 4,000 rpm for 30 min and filtrated (0.45 μm polyamide membrane filters, Whatman NL 17).
Plant available Si in soil samples (upper 25 cm, Ap horizon) was extracted in the course of the study of Puppe et al. (2021) following the procedure described by Haysom and Chapman (1975); de Lima Rodrigues et al. (2003). Two-gram samples of soil were placed in 50 mL plastic centrifuge tubes, mixed with 20 mL of a 0.01 M calcium chloride (CaCl2) solution, and agitated continuously on a swivel roller mixer for 16 h. Finally, the extracted solutions were centrifuged at 4,000 rpm for 30 min and filtrated using 0.45 μm polyamide membrane filters (Whatman NL 17).
Si concentrations in the HF acid, Na2CO3, Tiron, and CaCl2 extracts were measured at the ZALF Central Laboratory via ICP-OES (ICP-iCAP 6300 Duo, Thermo Fisher Scientific Inc.) using internal calibration standards made from a certified reference material (i.e., Certipur Si ICP Standard, Merck). Accuracy and long-term repeatability of Si concentration measurements via ICP-OES were systematically monitored. The detection limit was 0.6 × 10−3 ppb, the analytical measurement precision was ±1.3%. All analyses were performed in two lab replicates and three single ICP-OES measurements were performed per replicate resulting in six single values (n = 6) for every tested sample. Blank samples (one blank sample per 20 plant/soil samples) were used to analyze Si concentrations in the extraction/digestion chemicals. Blank sample Si concentrations were subtracted from corresponding plant/soil sample Si concentrations before further calculations. To avoid any potential Si contamination only plastic equipment was used during the entire laboratory work.
2.3 Phytolith extraction
Phytoliths were extracted from husk and straw samples by ashing following a protocol modified from Puppe and Leue (2018): i) 2.5 g of dry plant material was weighed, ii) the plant samples were heated in a muffle furnace for 6 h at 450°C to combust organic matter, iii) residual organic matter was oxidized using H2O2 (30%) and HNO3 (65%) at 80°C until the reaction subsided, iv) carbonates were dissolved by the use of hot HCl (10%, 30 min, 80°C), and v) the obtained phytoliths were washed with distilled water, dried at 105°C, and weighed.
2.4 Statistical analyses
Linear and monotonic relationships in the data set were analyzed via Pearson’s (r) and Spearman’s rank (rs) correlations (α level of 0.05), respectively, using the software package SPSS Statistics (version 22.0.0.0, IBM Corp.).
3 Results
Irrespective of the plant material used (husk or straw) we found Tiron to be more efficient in Si extraction compared to Na2CO3 (Figure 1). Regarding husk samples Tiron extracted almost the same amount of Si that was extracted by HF acid, while Na2CO3 extracted lower Si amounts. Regarding straw samples the efficacy of both alkaline extractants was lower compared to the one found for husk samples, which is reflected in the corresponding trendline equations and coefficients of determination. Correlation analyses confirmed these findings (Table 1), with highest correlation coefficients (alkaline-extracted Si vs. SiHF) for husk samples (n = 21), followed by husk + straw (n = 42) and straw (n = 21) samples.
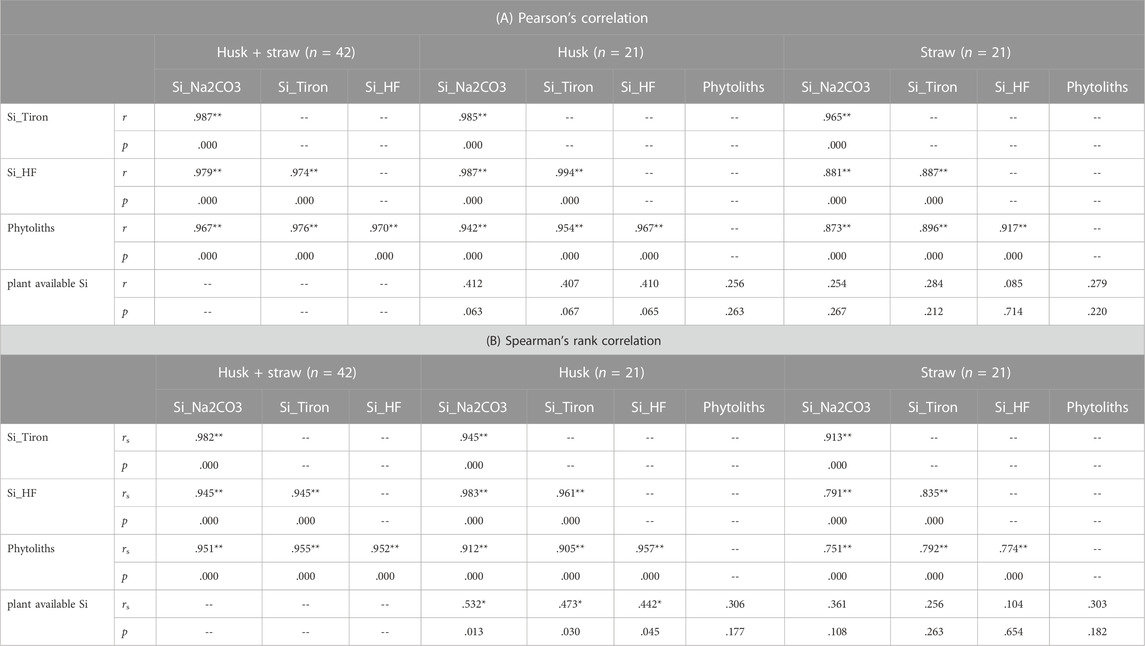
TABLE 1. Correlations between Si in plant materials (winter wheat, Si contents determined by Na2CO3 extraction, Tiron extraction, or HF digestion), phytolith contents (extracted from corresponding plant samples), and plant available Si concentrations in corresponding soil samples (extracted by CaCl2). Significant correlations are marked with asterisks (* indicates p < 0.05, ** indicates p < 0.01).
The differences in the efficacy of Tiron and Na2CO3 in Si extraction is also reflected in the corresponding alkaline-extracted Si-to-SiHF-ratios, which are higher for Tiron compared to Na2CO3 in all the samples (Supplementary Figure S1). Again the efficacy of both extractants was higher for husk samples (Si_Tiron/Si_HF ratio: 0.9–1.1, Si_Na2CO3/Si_HF ratio: 0.6–0.8) compared to straw samples (Si_Tiron/Si_HF ratio: 0.5–0.9, Si_Na2CO3/Si_HF ratio: 0.5–0.7).
Phytolith contents in plant samples were positively correlated to Si contents obtained from the applied extraction methods (Figure 2; Table 1). Especially in husk samples a strong linear relationship between phytolith contents and extracted Si was found, which was strongest for Si_HF (r = 0.967, p < 0.001), followed by Si_Tiron (r = 0.954, p < 0.001) and Si_Na2CO3 (r = 0.942, p < 0.001).
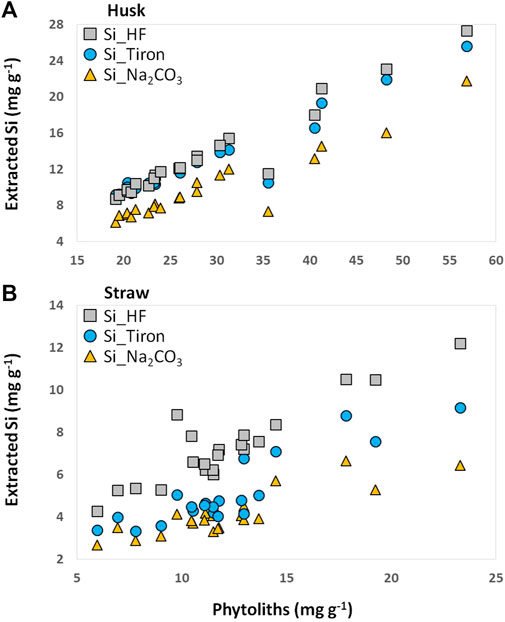
FIGURE 2. Extracted Si in plant materials [husk (A) and straw (B) of winter wheat] plotted against phytolith contents in corresponding plant samples.
Plant available Si in soil samples was reflected best in husk samples, where plant available Si was positively and statistically significantly correlated with Si_Na2CO3 (rs = 0.532, p = 0.013), Si_Tiron (rs = 0.473, p = 0.030), and Si_HF (rs = 0.442, p = 0.045) (Figure 3; Table 1). For straw samples no statistically significant correlations were found. Plant availability of Si in soil samples was not statistically significantly correlated to corresponding phytolith contents in husk and straw samples (Table 1).
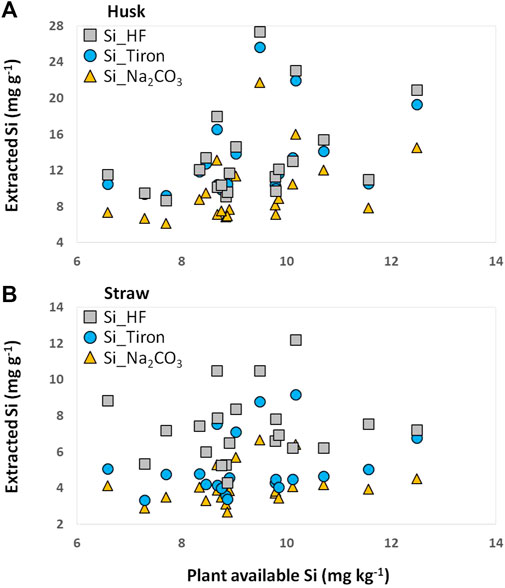
FIGURE 3. Extracted Si in plant materials [husk (A) and straw (B) of winter wheat] plotted against plant available Si in corresponding soil samples.
4 Discussion
4.1 Extraction efficacy and potential factors of influence
In general, our findings clearly show that alkaline extractions with Tiron are more efficient to determine Si contents in plants compared to Na2CO3. This corroborates the results of previous studies. Guntzer et al. (2010), for example, performed Tiron extractions using plant samples of wheat (Triticum durum), horsetail (Equisetum arvense), fern (Dicksonia squarrosa), elm (Ulmus laevis), and larch (Larix gmelinii) and compared their results to the corresponding results of electrothermal vaporization and lithium-metaborate digestion, which were used as reference methods to determine total Si contents. They found the results of electrothermal vaporization to be about 1.3 times higher than the ones obtained from Tiron extraction. This is generally comparable to the results of our study. We found that the results of HF digestion (used as reference for total Si contents) were about 1.04 (husk samples) and 1.4 (straw samples) times higher than the corresponding ones of Tiron extraction. In relation to the results of Na2CO3 extractions the corresponding results of HF digestion were about 1.4 and 1.8 times higher for husk and straw samples, respectively. The results of Tiron extraction were about 1.3 (husk) and 1.2 (straw) times higher than the corresponding ones of Na2CO3 extraction. How can these discrepancies between the results [(i) alkaline extractions vs. HF digestion, (ii) Na2CO3 vs. Tiron extraction, and (iii) husk vs. straw samples] be explained?
The discrepancies between single-step alkaline extractions and multistep HF digestions can be explained best by differences in the methods itself and the corresponding extractant strength. While the HF acid microwave digestion with its strong acids and relatively high temperatures can completely decompose siliceous (including crystalline silica such as quartz) and organic materials, alkaline extractions are comparatively weak. Na2CO3 is assumed to be unable to dissolve crystalline silica quickly, and thus a short-time Na2CO3 extraction is used for the specific extraction of amorphous silica (Meunier et al., 2014; Clymans et al., 2016; Schaller et al., 2022). Nakamura et al. (2020) compared the Na2CO3 method with the borate fusion method (which was used as reference) and found that the Na2CO3 method resulted in 16%–32% lower Si contents than the borate fusion method in five plant species. Based on their results these authors recommended to apply a correction factor to calculate ‘total’ Si contents of plants. However, ideally, this correction factor needs to be considered including a larger number of plant species. Moreover, the findings of Nakamura et al. (2020) are in line with our finding that extraction with Na2CO3 systematically underestimates Si contents in plant materials. Contrary, Tiron is assumed to dissolve noncrystalline (amorphous) silica quicker than Na2CO3 and to slightly attack crystalline silica (see Kodama & Ross, 1991). Thus, differences in the Si contents might also reflect a certain amount of amorphous silica with a very high condensation state in the plant samples, which has been observed in several previous studies (e.g., Dietrich et al., 2003; Schulz-Kornas et al., 2017).
Moreover, differences in phytolith composition likely influence extraction efficacy. Several studies have shown that phytolith surfaces are often associated with cell wall components like (hemi-) cellulose, cutin, or lignin (e.g., Law & Exley, 2011; Soukup et al., 2017; Zancajo et al., 2019). Additionally, organic compounds like proteins or glycoproteins can be found occluded in phytoliths (e.g., Elbaum et al., 2009; Alexandre et al., 2015; Kumar et al., 2020). These differences in phytolith composition are directly linked to the origin of phytoliths: While cell wall phytoliths are associated with a carbohydrate matrix, lumen phytoliths seem to contain more proteins and glycoproteins than cell wall phytoliths with consequences for phytolith dissolution kinetics and carbon sequestration, which is still discussed controversially [see the authoritative reviews of Hodson (2016; 2019) and references therein]. In a previous study Puppe et al. (2022b) analyzed leaf samples from harvest-fresh specimens of the identical winter wheat plants we used in the current study and found that about 62% of winter wheat phytoliths were lumen phytoliths, while 38% were cell wall phytoliths dividable equally in recognizable (in terms of phytolith morphology) cell wall phytoliths (i.e., short cells) and silicified tissue fragments. It can be assumed that the proportion of cell wall and lumen phytoliths in turn is directly linked to Si extraction efficacy as cell wall phytoliths are potentially more stable than lumen phytoliths. Our results on hand indicate that the proportion of cell wall and lumen phytoliths might differ between straw and husk samples resulting in differences of Si extraction efficacy. However, this has to be confirmed in future examinations. In this context, phytolith size and condensation state (potentially influenced by the location of phytolith formation and water evaporation, cf. Kumar et al., 2017) have to be considered as well (Schaller et al., 2021). In a further step we will analyze the morphology and elemental composition of phytoliths extracted from straw and husk samples to underline our assumption (cf. Puppe et al., 2022b).
4.2 Interactions between extractable plant and soil Si
In general, plant-Si-soil-Si relationships are mainly related to plant-specific Si uptake rates and Si availability in soils, factors that can be highly variable. This is why different studies often show inconsistent results. Our results, for example, show that i) phytolith contents in plant samples are not correlated to Si availability in soils at all (discussed in the last paragraph of this subsection) and ii) there are only weak relationships between Si contents in plant samples and plant available Si in corresponding soil samples. In fact, we only found statistically significant (moderate) correlations for husk samples using Spearman’s rank correlation (see Table 1). For straw samples, which showed lower Si contents than husk samples, no statistically significant correlations were detectable at all in our study. Contrary, Wu et al. (2020) found rice straw to better reflect plant available Si concentrations in soils, although rice straw also showed lower Si contents compared to rice husk. Wu and colleagues (2020) assumed the lower Si contents in rice straw to be less subject to variation—caused by fluctuations in concentrations of plant available Si in soils—than the higher Si contents in rice husk. Altogether, these results point to the need of further systematic analyses of plant-specific Si accumulation in different plant organs/materials. In this context, dynamics in plant Si uptake can be covered by analyses of plant samples taken at different plant growth stages (e.g., Schaller et al., 2022).
Regarding Si availability in soils our results partly (straw samples) corroborate the findings of Keeping (2017), who found that the uptake of Si by sugarcane in a shade house pot experiment did neither reflect the concentration of plant available Si in soils nor the Si content of used Si sources (calcium silicate slag, fused magnesium (thermo) phosphate, volcanic rock dust, magnesium silicate, and granular potassium silicate). This is in contrast to the studies of Miles et al. (2014) and Korndörfer et al. (2001), who found close correlations between plant available Si concentrations in soils and corresponding Si contents in sugarcane leaves and rice straw, respectively. These discrepancies between different studies can be explained by i) differences in the study design and ii) the fact that there is no standard extraction method for the determination of Si availability in soils yet. While studies that cover different soil types with widely varying physicochemical properties (e.g., pH, texture, or adsorption capacity) report close relationships between plant Si contents and Si concentrations in soils (e.g., Korndörfer et al., 2001; Miles et al., 2014), studies limited on few or only one study site can show ambiguous results (e.g., Klotzbücher et al., 2017; this study). Regarding Si availability in soils different procedures have been developed for specific plants grown under specific climates, i.e., mainly sugarcane and rice in (sub)tropical zones. In this context, potential correlations between plant available Si concentrations in soils and Si contents in plant materials are not only depending on soil texture, but also on the used extractant (cf. Crusciol et al., 2018) and how this extractant predicts Si availability in soils under a specific crop (potential mobilization of Si by, e.g., root exudates, see Wu et al., 2020). In future studies, measurements of the dynamics of plant available Si concentrations in soils will provide deeper insights into the complex interactions in the soil environment controlling Si availability in soils and corresponding Si uptake by plants (cf. de Tombeur et al., 2021). This knowledge is crucial to better assess (seasonal) human impacts (e.g., harvesting, fertilization, liming) on Si budgets of agricultural plant-soil systems and to implement sustainable strategies (e.g., straw recycling) in existing farming systems to prevent Si losses. In fact, a Si-based sustainable agriculture might be a promising approach to combine the beneficial effects of Si on plant performance with the demand for food security under global change (cf. Keeping and Reynolds, 2009; Van Bockhaven et al., 2013).
The detection of relationships between phytolith contents in plant samples and concentrations of plant available Si in corresponding soil samples is hampered by the fact that Si in plants is not only represented by extractable phytoliths, but also by fragile silica structures (Meunier et al., 2017; Puppe et al., 2017), which are not covered by phytolith extraction techniques like ashing performed in this study. As the majority of phytogenic silica might be stored in these fragile structures, current phytolith extraction techniques (ashing, acid digestion) thus might strongly underestimate the ‘total’ Si content of plants (cf. Puppe et al., 2017). Moreover, such methodological shortcomings might hamper an appropriate interpretation of the role of phytoliths in plant-soil systems (Kaczorek et al., 2019). Thus, we also need detailed analyses of phytogenic silica (extractable phytoliths vs. fragile structures) in different plant species as well as plant organs (e.g., leaves, stems, roots), which will help us to unravel the complex relationships between phytogenic silica in plant/soil samples and Si availability in soils.
5 Conclusion
In general, our results clearly corroborate previous studies that reported the suitability of single-step alkaline extractions for the determination of Si contents in plants. In fact, the relatively simple, fast, and cheap alkaline extractions represent promising alternatives for replacing the highly corrosive and toxic multistep digestion based methods. Concentrations of plant available Si in soils were correlated to Si contents in husk samples, but not in straw samples, which were characterized by lower Si contents compared to husk samples. Regarding extraction efficacy our comprehensive method comparison indicated a systematic superiority of Tiron compared to Na2CO3. This is why we recommend the usage of Tiron for the determination of Si contents in plants in the future. Of course, this should be accompanied by further, detailed non-destructive analyses of phytogenic silica in plants. In this context, the following questions should be in the research focus: i) How is Si stored in different plant species and plant organs (proportion of extractable phytoliths and fragile silica structures)?, ii) How is the proportion of extractable phytoliths and fragile silica structures in plant materials affecting efficacy of alkaline Tiron extraction?, and iii) Which plant material Si content represents best the Si availability in soils (and is this generalizable or plant species-specific)?
Data availability statement
The raw data supporting the conclusion of this article will be made available by the authors, without undue reservation.
Author contributions
DP and JS initialized and designed the experiment. DK and CB conducted the experiment and analyzed the samples. DP analyzed the corresponding data and wrote the manuscript. JS, DK, and CB contributed critically to the draft. All authors contributed to the article and approved the submitted version.
Funding
DP was funded by the Deutsche Forschungsgemeinschaft (DFG) under grant PU 626/2-1 (Biogenic Silicon in Agricultural Landscapes (BiSiAL)—Quantification, Qualitative Characterization, and Importance for Si Balances of Agricultural Biogeosystems).
Acknowledgments
We would like to thank Kristina Holz (head of the Central Laboratory, ZALF) and her team, especially Ellen Janiszewski, for ICP-OES analyses. Last but not least we would like to thank the Associate Editor and the reviewers, whose insightful comments on our manuscript considerably improved its quality.
Conflict of interest
The authors declare that the research was conducted in the absence of any commercial or financial relationships that could be construed as a potential conflict of interest.
Publisher’s note
All claims expressed in this article are solely those of the authors and do not necessarily represent those of their affiliated organizations, or those of the publisher, the editors and the reviewers. Any product that may be evaluated in this article, or claim that may be made by its manufacturer, is not guaranteed or endorsed by the publisher.
Supplementary material
The Supplementary Material for this article can be found online at: https://www.frontiersin.org/articles/10.3389/fenvs.2023.1145604/full#supplementary-material
SUPPLEMENTARY FIGURE S1 | Alkaline-extracted Si-to-SiHF-ratios of husk (left diagram half) and straw (right diagram half) samples of winter wheat.
References
Alexandre, A., Basile-Doelsch, I., Delhaye, T., Borshneck, D., Mazur, J. C., Reyerson, P., et al. (2015). New highlights of phytolith structure and occluded carbon location: 3-D X-ray microscopy and NanoSIMS results. Biogeosciences 12 (3), 863–873. doi:10.5194/bg-12-863-2015
Alexandre, A., Meunier, J.-D., Colin, F., and Koud, J.-M. (1997). Plant impact on the biogeochemical cycle of silicon and related weathering processes. Geochimica Cosmochimica Acta 61 (3), 677–682. doi:10.1016/s0016-7037(97)00001-x
Barão, L., Vandevenne, F., Clymans, W., Frings, P., Ragueneau, O., Meire, P., et al. (2015). Alkaline-extractable silicon from land to ocean: A challenge for biogenic silicon determination. Limnol. Oceanogr. Methods 13 (7), 329–344. doi:10.1002/lom3.10028
Biermans, V., and Baert, L. (1977). Selective extraction of the amorphous Al, Fe and Si oxides using an alkaline Tiron solution. Clay Miner. 12, 127–135. doi:10.1180/claymin.1977.012.02.03
Carey, J. C., and Fulweiler, R. W. (2016). Human appropriation of biogenic silicon - the increasing role of agriculture. Funct. Ecol. 30, 1331–1339. doi:10.1111/1365-2435.12544
Clymans, W., Conley, D. J., Battles, J. J., Frings, P. J., Koppers, M. M., Likens, G. E., et al. (2016). Silica uptake and release in live and decaying biomass in a northern hardwood forest. Ecology 97 (11), 3044–3057. doi:10.1002/ecy.1542
Crusciol, C. A. C., de Arruda, D. P., Fernandes, A. M., Antonangelo, J. A., Alleoni, L. R. F., Nascimento, C., et al. (2018). Methods and extractants to evaluate silicon availability for sugarcane. Sci. Rep. 8 (1), 916. doi:10.1038/s41598-018-19240-1
de Lima Rodrigues, L., Daroub, S. H., Rice, R. W., and Snyder, G. H. (2003). Comparison of three soil test methods for estimating plant-available silicon. Commun. Soil Sci. Plant Analysis 34 (15-16), 2059–2071. doi:10.1081/css-120024048
de Tombeur, F., Roux, P., and Cornelis, J.-T. (2021). Silicon dynamics through the lens of soil-plant-animal interactions: Perspectives for agricultural practices. Plant Soil 467, 1–28. doi:10.1007/s11104-021-05076-8
DeMaster, D. J. (1981). The supply and accumulation of silica in the marine environment. Geochimica Cosmochimica Acta 45 (10), 1715–1732. doi:10.1016/0016-7037(81)90006-5
Dietrich, D., Hinke, S., Baumann, W., Fehlhaber, R., Baucker, E., Ruhle, G., et al. (2003). Silica accumulation in Triticum aestivum L. and Dactylis glomerata L. Anal. Bioanal. Chem. 376 (3), 399–404. doi:10.1007/s00216-003-1847-8
Elbaum, R., Melamed-Bessudo, C., Tuross, N., Levy, A. A., and Weiner, S. (2009). New methods to isolate organic materials from silicified phytoliths reveal fragmented glycoproteins but no DNA. Quat. Int. 193 (1-2), 11–19. doi:10.1016/j.quaint.2007.07.006
Evans, C. C. (1970). X-ray fluorescence analysis for light elements in plant and faecal materials. Analyst 95, 919–929. doi:10.1039/an9709500919
Guntzer, F., Keller, C., and Meunier, J. D. (2010). Determination of the silicon concentration in plant material using Tiron extraction. New Phytol. 188 (3), 902–906. doi:10.1111/j.1469-8137.2010.03416.x
Haynes, R. J. (2017). Significance and role of Si in crop production. Adv. Agron. 146, 83–166. doi:10.1016/bs.agron.2017.06.001
Haysom, M. B. C., and Chapman, L. S. (1975). Some aspects of the calcium silicate trials at Mackay. Proc. Aust. Soc. Sugar Cane Technol. 42, 117–122.
Hodson, M. J. (2016). The development of phytoliths in plants and its influence on their chemistry and isotopic composition. Implications for palaeoecology and archaeology. J. Archaeol. Sci. 68, 62–69. doi:10.1016/j.jas.2015.09.002
Hodson, M. J. (2019). The relative importance of cell wall and lumen phytoliths in carbon sequestration in soil: A hypothesis. Front. Earth Sci. 7. doi:10.3389/feart.2019.00167
Kaczorek, D., Puppe, D., Busse, J., and Sommer, M. (2019). Effects of phytolith distribution and characteristics on extractable silicon fractions in soils under different vegetation – an exploratory study on loess. Geoderma 356, 113917. doi:10.1016/j.geoderma.2019.113917
Katz, O., Puppe, D., Kaczorek, D., Prakash, N. B., and Schaller, J. (2021). Silicon in the soil-plant continuum: Intricate feedback mechanisms within ecosystems. Plants (Basel) 10 (4), 652. doi:10.3390/plants10040652
Keeping, M. G., and Reynolds, O. L. (2009). Silicon in agriculture: New insights, new significance and growing application. Ann. Appl. Biol. 155, 153–154. doi:10.1111/j.1744-7348.2009.00358.x
Keeping, M. G. (2017). Uptake of silicon by sugarcane from applied sources may not reflect plant-available soil silicon and total silicon content of sources. Front. Plant Sci. 8, 760. doi:10.3389/fpls.2017.00760
Kendrick, K. J., and Graham, R. C. (2004). Pedogenic silica accumulation in chronosequence soils, Southern California. Soil Sci. Soc. Am. J. 68, 1295–1303. doi:10.2136/sssaj2004.1295
Klotzbücher, A., Klotzbücher, T., Jahn, R., Xuan, L. D., Cuong, L. Q., Van Chien, H., et al. (2017). Effects of Si fertilization on Si in soil solution, Si uptake by rice, and resistance of rice to biotic stresses in Southern Vietnam. Paddy Water Environ. 16 (2), 243–252. doi:10.1007/s10333-017-0610-2
Kodama, H., and Ross, G. J. (1991). Tiron dissolution method used to remove and characterize inorganic components in soils. Soil Sci. Soc. Am. J. 55, 1180–1187. doi:10.2136/sssaj1991.03615995005500040047x
Korndörfer, G. H., Snyder, G. H., Ulloa, M., Powell, G., and Datnoff, L. E. (2001). Calibration of soil and plant silicon analysis for rice production. J. Plant Nutr. 24 (7), 1071–1084. doi:10.1081/pln-100103804
Kumar, S., Adiram-Filiba, N., Blum, S., Sanchez-Lopez, J. A., Tzfadia, O., Omid, A., et al. (2020). Siliplant1 protein precipitates silica in sorghum silica cells. J. Exp. Bot. 71 (21), 6830–6843. doi:10.1093/jxb/eraa258
Kumar, S., Soukup, M., and Elbaum, R. (2017). Silicification in grasses: Variation between different cell types. Front. Plant Sci. 8, 438. doi:10.3389/fpls.2017.00438
Law, C., and Exley, C. (2011). New insight into silica deposition in horsetail (Equisetum arvense). BMC plant Biol. 11 (1), 112–119. doi:10.1186/1471-2229-11-112
Marxen, A., Klotzbücher, T., Jahn, R., Kaiser, K., Nguyen, V. S., Schmidt, A., et al. (2016). Interaction between silicon cycling and straw decomposition in a silicon deficient rice production system. Plant Soil 398, 153–163. doi:10.1007/s11104-015-2645-8
Meena, V. D., Dotaniya, M. L., Coumar, V., Rajendiran, S., Kundu, S., Rao, A. S., et al. (2014). A case for silicon fertilization to improve crop yields in tropical soils. Proc. Natl. Acad. Sci. India B Biol. Sci. 84, 505–518. doi:10.1007/s40011-013-0270-y
Meunier, J. D., Barboni, D., Anwar-Ul-Haq, M., Levard, C., Chaurand, P., Vidal, V., et al. (2017). Effect of phytoliths for mitigating water stress in durum wheat. New Phytol. 215 (1), 229–239. doi:10.1111/nph.14554
Meunier, J. D., Keller, C., Guntzer, F., Riotte, J., Braun, J. J., and Anupama, K. (2014). Assessment of the 1% Na2CO3 technique to quantify the phytolith pool. Geoderma 216, 30–35. doi:10.1016/j.geoderma.2013.10.014
Miles, N., Manson, A. D., Rhodes, R., van Antwerpen, R., and Weigel, A. (2014). Extractable silicon in soils of the South African sugar industry and relationships with crop uptake. Commun. Soil Sci. Plant Analysis 45 (22), 2949–2958. doi:10.1080/00103624.2014.956881
Nakamura, R., Cornelis, J.-T., de Tombeur, F., Nakagawa, M., and Kitajima, K. (2020). Comparative analysis of borate fusion versus sodium carbonate extraction for quantification of silicon contents in plants. J. Plant Res. 133 (2), 271–277. doi:10.1007/s10265-019-01162-2
Puppe, D., Höhn, A., Kaczorek, D., Wanner, M., Wehrhan, M., and Sommer, M. (2017). How big is the influence of biogenic silicon pools on short-term changes in water-soluble silicon in soils? Implications from a study of a 10-year-old soil–plant system. Biogeosciences 14 (22), 5239–5252. doi:10.5194/bg-14-5239-2017
Puppe, D., Kaczorek, D., Schaller, J., Barkusky, D., and Sommer, M. (2021). Crop straw recycling prevents anthropogenic desilication of agricultural soil–plant systems in the temperate zone – results from a long-term field experiment in NE Germany. Geoderma 403, 115187. doi:10.1016/j.geoderma.2021.115187
Puppe, D., Kaczorek, D., and Schaller, J. (2022a). “Biological impacts on silicon availability and cycling in agricultural plant-soil systems,” in Silicon and nano-silicon in environmental stress management and crop quality improvement (Amsterdam, Netherlands: Elsevier), 309–324.
Puppe, D., and Leue, M. (2018). Physicochemical surface properties of different biogenic silicon structures: Results from spectroscopic and microscopic analyses of protistic and phytogenic silica. Geoderma 330, 212–220. doi:10.1016/j.geoderma.2018.06.001
Puppe, D., Leue, M., Sommer, M., Schaller, J., and Kaczorek, D. (2022b). Auto-fluorescence in phytoliths—a mechanistic understanding derived from microscopic and spectroscopic analyses. Front. Environ. Sci. 10. doi:10.3389/fenvs.2022.915947
Queralt, I., Ovejero, M., Carvalho, M. L., Marques, A. F., and Llabrés, J. M. (2005). Quantitative determination of essential and trace element content of medicinal plants and their infusions by XRF and ICP techniques. X-Ray Spectrom. 34 (3), 213–217. doi:10.1002/xrs.795
Sauer, D., Saccone, L., Conley, D. J., Herrmann, L., and Sommer, M. (2006). Review of methodologies for extracting plant-available and amorphous Si from soils and aquatic sediments. Biogeochemistry 80 (1), 89–108. doi:10.1007/s10533-005-5879-3
Savant, N. K., Korndörfer, G. H., Datnoff, L. E., and Snyder, G. H. (1999). Silicon nutrition and sugarcane production: A review1. J. Plant Nutr. 22 (12), 1853–1903. doi:10.1080/01904169909365761
Schaller, J., Puppe, D., Busse, J., Paasch, S., Katz, O., Brunner, E., et al. (2022). Silicification patterns in wheat leaves related to ontogeny and soil silicon availability under field conditions. Plant Soil 477, 9–23. doi:10.1007/s11104-022-05385-6
Schaller, J., Puppe, D., Kaczorek, D., Ellerbrock, R., and Sommer, M. (2021). Silicon cycling in soils revisited. Plants (Basel) 10 (2), 295. doi:10.3390/plants10020295
Schulz-Kornas, E., Braune, C., Winkler, D. E., and Kaiser, T. M. (2017). Does silica concentration and phytolith ultrastructure relate to phytolith hardness? Biosurface Biotribology 3 (4), 135–143. doi:10.1016/j.bsbt.2017.12.004
Snyder, G. (2001). Methods for silicon analysis in plants, soils, and fertilizers. Stud. plant Sci. 8, 185–196.
Sommer, M., Jochheim, H., Höhn, A., Breuer, J., Zagorski, Z., Busse, J., et al. (2013). Si cycling in a forest biogeosystem – The importance of transient state biogenic Si pools. Biogeosciences 10 (7), 4991–5007. doi:10.5194/bg-10-4991-2013
Soukup, M., Martinka, M., Bosnić, D., Čaplovičová, M., Elbaum, R., and Lux, A. (2017). Formation of silica aggregates in sorghum root endodermis is predetermined by cell wall architecture and development. Ann. Bot. 120 (5), 739–753. doi:10.1093/aob/mcx060
Struyf, E., Smis, A., Van Damme, S., Garnier, J., Govers, G., Van Wesemael, B., et al. (2010). Historical land use change has lowered terrestrial silica mobilization. Nat. Commun. 1, 129. doi:10.1038/ncomms1128
Van Bockhaven, J., De Vleesschauwer, D., and Höfte, M. (2013). Towards establishing broad-spectrum disease resistance in plants: Silicon leads the way. J. Exp. Bot. 64, 1281–1293. doi:10.1093/jxb/ers329
Vandevenne, F., Struyf, E., Clymans, W., and Meire, P. (2012). Agricultural silica harvest: Have humans created a new loop in the global silica cycle? Front. Ecol. Environ. 10, 243–248. doi:10.1890/110046
Wade, R. N., Donaldson, S. M., Karley, A. J., Johnson, S. N., and Hartley, S. E. (2022). Uptake of silicon in barley under contrasting drought regimes. Plant Soil 477, 69–81. doi:10.1007/s11104-022-05400-w
Wehrhan, M., Puppe, D., Kaczorek, D., and Sommer, M. (2021). Spatial patterns of aboveground phytogenic Si stocks in a grass-dominated catchment – results from UAS-based high-resolution remote sensing. Biogeosciences 18 (18), 5163–5183. doi:10.5194/bg-18-5163-2021
Wu, W., Limmer, M. A., and Seyfferth, A. L. (2020). Quantitative assessment of plant-available silicon extraction methods in rice paddy soils under different management. Soil Sci. Soc. Am. J. 84 (2), 618–626. doi:10.1002/saj2.20013
Zancajo, V. M. R., Diehn, S., Filiba, N., Goobes, G., Kneipp, J., and Elbaum, R. (2019). Spectroscopic discrimination of sorghum silica phytoliths. Front. Plant Sci. 10, 1571. doi:10.3389/fpls.2019.01571
Keywords: winter wheat (Triticum aestivum), phytoliths, biogenic silica, plant available Si, husk, straw
Citation: Puppe D, Kaczorek D, Buhtz C and Schaller J (2023) The potential of sodium carbonate and Tiron extractions for the determination of silicon contents in plant samples—A method comparison using hydrofluoric acid digestion as reference. Front. Environ. Sci. 11:1145604. doi: 10.3389/fenvs.2023.1145604
Received: 17 January 2023; Accepted: 10 May 2023;
Published: 22 May 2023.
Edited by:
Jonas Schoelynck, University of Antwerp, BelgiumCopyright © 2023 Puppe, Kaczorek, Buhtz and Schaller. This is an open-access article distributed under the terms of the Creative Commons Attribution License (CC BY). The use, distribution or reproduction in other forums is permitted, provided the original author(s) and the copyright owner(s) are credited and that the original publication in this journal is cited, in accordance with accepted academic practice. No use, distribution or reproduction is permitted which does not comply with these terms.
*Correspondence: Daniel Puppe, daniel.puppe@zalf.de