- 1Pan African University Life and Earth Sciences Institute, Ibadan, Nigeria
- 2Department of Geology, University of Ibadan, Ibadan, Nigeria
- 3Department of Environmental Sciences, University of Toledo, Toledo, OH, United States
Management of soil and groundwater resources has been recognized as essential to meeting the sustainable development goals of Agenda 2063 of the African Union. As Africa’s fastest growing population with over 200 million people, Nigeria is responsible for leading the continent’s environmental sustainability goal. Nigeria has seen a sizable number of crude oil spillages that have contaminated its soils and groundwater resources, and several of these contaminated sites are to be cleaned up yet. There needs to be more scientific data to design an effective cleanup and to manage the soil and groundwater resources effectively. So far, the only extensive crude oil-contaminated site remediation project documented is on Ogoniland in the Niger Delta region of Nigeria. However, this project resulted in less effective, albeit temporary, cleanup solutions. This review presents a state-of-the-art synthesis of research on soil and groundwater contamination by crude oil. It includes sections on processes, measurements, predictions, and management, as well as an analysis of the state and challenges in Nigeria. In-depth field, laboratory, and computer models for crude oil contamination investigation have been developed with over 60 years of significant research. However, studies and case projects in Nigeria have relied on point sampling to determine the concentration of crude oil contaminants in soil and groundwater. This method offers limited information on the solute concentration and hydraulic distribution, which regulates pollutant mobility within the subsurface. The absence of baseline and high-resolution subsurface characterization data has also resulted in a need for more process-based knowledge to direct the development of site-specific remediation strategies. As a result, it is challenging to design a conceptual model that is detailed enough to help with predictions of the flow dynamics of crude oil contaminants in the unsaturated and saturated zones. It is anticipated that this review will stimulate further multidisciplinary research through site evaluation and monitoring to provide reliable information that can be used to develop appropriate model-based remediation solutions.
1 Introduction
Since the discovery of Oil Creek in Northwestern Pennsylvania by Edwin Drake in 1859 (Wicks, 2009), the demand for crude oil exploration has increased over time. With new advances and technologies in conventional and unconventional exploration of oil and gas resources, crude oil has become the principal source of revenue growth in most oil-rich nations (Adipah, 2018). Following the discovery of crude oil, various exploration, production, and distribution activities have resulted in massive oil spills worldwide (Etkin and Welch, 2005). According to the International Tanker Owners Pollution Federation Limited (ITOPF) oil spill statistics, accidental large-scale oil spills contribute to a significant volume of contamination in the global environment (ITOPF, 2021). The most notable oil spills include the Castillo de Bellver tanker carrying 1.5 million barrels of crude oil that capsized and sank in 420 m of water (Moldan et al., 1985); the release of over 6 million barrels of crude oil into the Arabian Gulf during the Gulf War (Cekirge et al., 1992); and the release of 3.5 million barrels of crude oil from the collision of the Atlantic Empress and Aegan Captain in the Caribbean Sea (Horn and Neal, 2005). Although oil spills have decreased in recent years, the long-term impact of the previously recorded oil spill still affects some productive and vulnerable compartments of the ecosystem (Chen et al., 2012a).
Crude oil spills affect different compartments of the environment, such as air, surface water, plants, soils, and groundwater systems. The near-surface soil and groundwater system (critical zone) is characterized by an elevated level of heterogeneity, which makes the introduction of crude oil with various physical and chemical properties more challenging to investigate (Theodorakis and Walker, 2014). Subsurface fluid flow dynamics are pretty heterogeneous due to variation in the soil’s physical, chemical, and biological properties. For instance, the organic matter content of the soil increases, so does its complexity, which is influenced by abiotic soil structure and biotic variation and results in diverse bacterial communities (Parker, 1989; Pepper et al., 2015). The presence of crude oil contaminants in soil and groundwater generates an additional fluid phase due to their physical qualities, resulting in a multiphase fluid flow system. The partitioning of contaminants between different phases affects the processes that govern the fate and transport of crude oil contaminants in the subsurface (Kim and Swanson, 2001; Brusseau, 2019b). Investigating these processes requires a detailed site conceptualization that involves a multidisciplinary approach (Williams et al., 2006). This approach focuses on investigating the various physiochemical and biological processes and their interplay as they affect the fate and transport of crude oil contaminants (Biswas et al., 2018; Maier, 2019). A well-defined conceptual site model of contaminant flow and transport includes knowledge of the shallow subsurface physicochemical configuration and hydraulic and transport properties (Rolle et al., 2011). The conceptual model is expected to result in a hypothesis and predictions that can be used to understand the future implications of the movement of crude oil contaminants and their interactions with the sources, flow channels, and soil and groundwater systems (Mugunthan et al., 2016).
In Nigeria, thousands of records of crude oil spillage, whose causes include sabotage and theft, equipment failure, and corrosion, have been identified (Ejiba et al., 2016). The threat of crude oil contamination to human health has resulted in multiple lawsuits, crises, and bloodshed (Bodo, 2019). The most recent historic verdict in favor of four farmers and environmental activists declared Shell Company accountable for the oil spill in Ibibio land, with an unknown amount to be paid to the farmers (Doro et al., 2021). The National Oil Spill Detection and Response Agency (NOSDRA) estimated in 2021 that over 92 percent of crude oil-contaminated sites remained unremediated, with minimal scheduled cleanup. Most crude oil spills are more than 10 years old, creating a continuing source of contamination in the soil and groundwater systems (Michel and Fingas, 2016). Crude oil contamination remains a critical component of soil and groundwater contamination in Nigeria, with most oil-producing states recording considerable numbers of crude oil spillages, leading to several land and water systems remaining in deteriorated conditions, unmonitored, and remediation efforts that are currently ineffective. Until recently, there has been little research that summarized the state of knowledge on crude oil contamination in Nigeria, despite many reports of oil spillage as documented by NOSDRA. The current condition of many contaminated sites cannot be ascertained because comprehensive site investigations have not been carried out except for the recent Ogoniland cleanup, the federal government’s only significant remediation effort. Despite the United Nations Environment Programme (UNEP) outlining specific remediation strategies for contaminated soil, no particular strategy was found to be the most efficient for the Niger Delta region. (Zabbey et al., 2017). This can be attributed to a lack of detailed subsurface heterogeneity information that will boost the success rate of any remediation exercise, causing the initiative to fall short of its intended goal. A few contaminated site investigations recorded in the literature tend to focus more on soil and groundwater geochemical characterization by comparing concentration values with acceptable standard values, making a first-order assessment of contaminant sites in the Niger Delta a challenge, particularly on a regional scale (Ebuehi et al., 2005; Osuji & Ezebuiro, 2006; Dago et al., 2018). The lack of interest from the government body is linked to the perceived economic value of groundwater resources in Nigeria. There is a need to review the practical approaches to contaminant site investigation by providing the current status of soil and groundwater processes that control the movement of crude oil contaminants.
In this study, we outline the physical, chemical, and biological processes that determine the fate and movement of crude oil contaminants in soil and groundwater systems using global examples but emphasizing the Nigerian context. This is intended to provide adequate knowledge of crude oil mass transfer, natural attenuation, and the appropriate management technique to stimulate more scientific discussion on Nigeria’s characterization and remediation of crude oil-contaminated sites. In addition, we identify possible knowledge gaps in Nigerian contaminant site characterization and remediation research. This review examines the existing conceptual model and research that explains the processes that govern the fate and transport of crude oil contaminants. We critically analyze this literature and the diverse, more specialized process-based literature that contributes to our understanding of mass transfer, crude oil transport, and attenuation in unsaturated and saturated zones.
2 Crude oil contaminant fate and transport processes in unconsolidated sediments
The evolution of crude oil contamination research begins with the assumption that crude oil introduced into the subsurface gravitationally flows down to the water table and either floats like a pancake on top of the water table, displacing nearly all the water and air in the aquifer’s pore space, or penetrates to the water table’s base before settling, depending on its density properties (Bennett et al., 1999; Mansi et al., 2017, Azimi et al.,2020; Dragun, 2021). However, studies have shown that crude oil coexists with water in the aquifer’s pore network, and the degree of crude oil contaminant saturation is highly dependent on the lithology and fluid properties (Qin et al., 2009; Brusseau, 2019a). Typically, this is determined from water and soil samples collected from wells and boreholes with BTEX (benzene, toluene, ethylbenzene, and xylene) concentrations significantly higher than the standard values (Nwachukwu and Osuagwu, 2014). Upon the release of crude oil into the subsurface, crude oil migrates downward under the influence of gravity and is laterally subjected to capillary forces (Figure 1). The capillary forces caused the contaminant mass to be immobilized within the soil pores, though lateral spreading within the unsaturated zone could be observed depending on the contaminant’s volume and the permeability of such a layer (Sethi and Di Molfetta, 2019). The downward migration is impeded upon getting to the capillary fringe as the buoyancy force and increased water content limit the extent of vertical migration, leading to lateral migration in the direction of the groundwater flow gradient (Essaid et al., 2015).
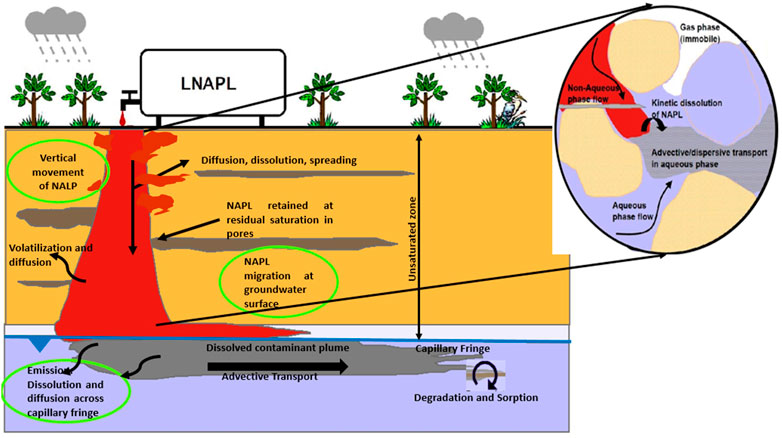
FIGURE 1. Conceptual model of the evolution of hydrocarbon contaminant in the subsurface (modified from Theodorakis and Walker, 2014).
However, the accumulation of crude oil contaminants and radial flow can occur if the rate of crude oil contaminant movement from the surface is greater than that of the lateral migration (Pan et al., 2021). The dissolved plume provides an extended source of contamination as soluble constituents are transformed into water and solid phases (Ossai et al., 2020). This transformation leads to changes in the mass and composition of the contaminant with time, which is directly related to the concentration variation along the flow path. These temporal and spatial transformations are governed by advection, dispersion, adsorption and desorption, volatilization, partitioning, evaporation, and biodegradation (Brusseau and Artiola, 2019).
2.1 Advection, dispersion, and diffusion
Advection is the most dominant solute transport mechanism in soils whose magnitude is primarily controlled by hydraulic gradient distribution along the flow path (de Vries et al., 2017). Advection describes the bulk movement of crude oil contaminants under a hydraulic head gradient (Myers, 2012). In the unsaturated zone, the rate of the advection process has been identified to be controlled by the rate of infiltration, which is directly related to the quantity of contaminant transport in the aqueous phase toward the groundwater (Molins et al., 2010; Basu et al., 2020; Chang et al., 2021). Its effect is significantly observed in layered permeable deposits with predominantly parallel bedding planes (Anderson et al., 2015). This process causes the interlayer solute transfer to decline along the permeable layers, thus increasing the concentration in the less permeable layers. This, in turn, leads to the development of a dispersed concentration gradient in the direction of flow (Güven et al., 1986). Due to composition and temperature variation, advection transport in the unsaturated zone is greatly affected by barometric pressure water, movement through infiltration, water table fluctuation, and density gradient (Petri et al., 2015). Though advection is not the most dominant contaminant transport process in the unsaturated zone, its relevance is observed in the highly permeable, relatively deep unsaturated zone (Šimůnek and Genuchten, 2016). Molins et al. (2010) revealed that a pressure-driven advection contributes up to 15% toward the net flux of crude oil contaminants in the unsaturated zone. Advection-induced flow tends to have more significant horizontal components as it is significantly driven by the lateral groundwater flow, though its effect is extensively seen at an early stage of the crude oil spill. Advection-driven crude oil contaminant migration occurs alongside dispersion and diffusion. Though the spatial influences of the latter two processes are controlled by pore-scale heterogeneity, their importance cannot be neglected (Anderson, 1984; Aziz et al., 2018; Aminnaji et al., 2019).
The irregularities of the soil pores and the flow velocity pattern within a pore influence the dispersion process (Jarvis et al., 2016). Dispersion is commonly considered a second-order process characterized by variations in groundwater velocity around the mean advective velocity (Hadley and Newell, 2014). Under transient flow, dispersion at the pore scale tends to be visible at short intervals and extremely diffuses at long intervals. A more extensive pore size distribution generates more significant velocity differences in solute transport, resulting in a greater spread. This would subsequently contribute to the observed non-ideal behavior of crude oil contaminants. At short and long travel durations, the mixing and spreading of solute plumes traveling through saturated porous media exhibit unexpected dynamics and statistics. Recent research has demonstrated that a non-linear compound-specific parameterization of transverse dispersion is required to define transverse mixing at the Darcy scale (Hochstetler et al., 2013). In addition, transverse mixing in heterogeneous porous media lowers plume fingering caused by longitudinal dispersion (Reidenbach et al., 2007). Diffusion acting through small-scale stratigraphic heterogeneities in porous media can account for the longitudinal dispersion commonly observed in groundwater contaminant plumes and tracer pulse migration (Hadley and Newell, 2014).
Diffusion controls the mass transfer process in a non-equilibrium fluid phase. The diffusion process is the dominant mass transfer process in the unsaturated zone (You et al., 2020). Over time, diffusion tends to diminish acute disparities in concentration distributions. Transverse mixing of hydrocarbon contaminants along the plume margin can influence long-term diffusion-induced migration, posing difficulty in the upscaling of solute transport models (Grathwohl et al., 2020). Diffusion is, therefore, significant not just conceptually since it is the only mechanism that results in an efficient exchange of solute mass in fluids but also quantitatively and practically (Rolle and Kitanidis, 2014). Under normal groundwater flow conditions, the pore diffusion term is quantitatively significant for the local hydrodynamic dispersion coefficients. This negates the assumption of Giadom et al. (2015), who generalized advection and dispersion as the major driving forces in contaminant migration in the Niger Delta region. However, his work forms a base for further investigation to extend the knowledge base of the various processes that control contaminant migration within the area. In addition, when groundwater flow is primarily dominated by advection, diffusion plays a significant role in mechanical dispersion due to the development of poor mixing and compound-specific concentration gradients in the pore channels. Scheibe et al. (2015a) investigated the combined effect of pore-scale advection and intra-granular diffusion on solute transport. They concluded that pore-scale diffusive mass transfer processes between preferential flow paths and relatively immobile zones within the primary porosity might substantially impact transport, particularly in low-concentration tails. Kovalick and Montgomery (2014) reported that if reaction rates or pore-size distribution variation is substantial, the magnitude of the rate of molecular diffusion across pores via the rock matrix can influence the geometry of the concentration gradients. As a result, it is expected that reaction rates and associated microbial growth will increase at pore throats in regions with shorter diffusion zones. More recently, Yoon et al. (2019) utilized calcite dissolution as an example to demonstrate how changes in pore-scale velocity reduce the reaction rate between dissolved and solid phases. They revealed that diffusion-induced contaminant transport is more visible in low-permeability zones. Therefore, diffusion explains poor results from many groundwater remediations because at contaminant sites where transmissive zones are in contact with low-permeability zones, the diffusion process is classified as a first-order process.
The advection and dispersion processes form the basis of crude oil contaminant modeling in groundwater contamination. Over time, there has been concern about the accuracy of the advection and dispersion equation used in contaminant site modeling because this approach depicts an ideal subsurface condition that rarely pictures the true subsurface field heterogeneity. Dong et al. (2018) suggested that solute transport time and pore-scale geometry control the magnitude of the effect of dispersion; thus, it is assumed that advection-induced flow is homogenous most times, leading to an underestimation of the dispersion coefficient. This was further confirmed by Ren et al. (2022), who revealed that at meter-scale heterogeneity, effective dispersion is more sensitive to pore-scale heterogeneity. Solute mixing by hydrodynamic dispersion reflects the heterogeneity of the aquifer at a scale smaller than that associated with the measurement of advection. Thus, the advection–dispersion process is believed to likely overestimate changes in concentration as a dispersion-based attenuation process which sometimes does not accurately simulate release from matrix diffusion storage. The diffusion-dominated process, compared to the dispersion-dominated process, has become essential due to the heterogeneity and anisotropy concept governing crude oil transport (Hadley and Newell, 2014). A new development in the groundwater contamination modeling approach is trending toward a heterogeneity-dominated advection–diffusion approach (Hadley and Newell, 2014). The interaction of the aforementioned processes results in an infinite number of scenarios that control how crude oil contaminants migrate in the subsurface. This poses a significant challenge for environmental managers in identifying primary crude oil controlling factors and considering them in evaluating and optimizing the associated risk (Swartjes, 2011). In addition, the interplay of soil, water, and contaminant poses a substantial challenge for researchers in forecasting important parameters that aid in understanding subsurface dynamics and contamination evolution (Brusseau et al., 2019a). Depending on the scale of the process, the physical, chemical, and biological parameters can be determined through laboratory and field research (Iovenitti et al., 1992; Bruseau and Artiola, 2019).
Until recently, no studies summarized the state of contamination of freshwater and coastal aquifers in Nigeria. Crude oil contamination of soil and groundwater in Nigeria is widespread, with little information about the quantity trapped within the unsaturated zone and the groundwater system, making it difficult to understand the critical process that controls its migration. There is expected to be a paradigm shift in future contaminant research in Nigeria. The current understanding of the behavior of crude oil contaminants is largely deficient for properly optimizing remediation strategies (Almutari, 2018). This new shift should be data-driven (Doro et al., 2020) and involve detailed laboratory experiments simulating the subsurface site conditions from which different processes can be investigated before further upscaling to field-scale research. The initial approach of PINOT sampling investigation needs to be replaced by long-term monitoring of periodic changes in contaminant concentrations, water tables, and biological facies. These will serve as a base for further investigation of knowledge gaps relating to uncontrolled groundwater extraction, climate change, and sea level rise.
3 Approaches to investigate the fate and transport of crude oil contaminants
To better understand the processes in terms of their individual and combined influence on the fate and transport of crude oil pollutants, multiphase and reactive transport processes must be investigated at both the experimental and field scales. The combination of these two approaches has led to the development of predictive models that allow for subsurface modeling of contaminants’ evolution, monitoring their movement, and predicting their final fate (Earl et al., 2003). These long-term estimates help evaluate natural attenuation or cleanup operations and determine the best reaction in the event of a spill mishap. In addition, data from quantitative and qualitative field research are regularly used in the calibration and validation of prediction models, serving as a guide for more effective and efficient remediation implementation. A summary of the selected literature on experimental and field-based approaches is summarized in Table 1 and discussed in the following sections.
3.1 Experimental approach
An experimental technique has been applied in a controlled environment under regulated conditions to improve the understanding of the dynamics of crude oil contaminants in both the saturated and unsaturated zones. Because of physical, chemical, and biological heterogeneity, field-scale research is typically complicated, but this complexity is decreased in an experimental context, allowing for high-resolution, microscopic studies. Multiphase studies have been carried out in sediment-packed one-dimensional (1D) columns, two-dimensional (2D) slabs, and three-dimensional (3D) tanks. Esposito et al. (1999) carried out 2D multiphase flow experiments in variably saturated sand and unsaturated sand with varying porosities, with an emphasis on the effect of the capillary pressure–saturation relationship, the sensitivity of finger pattern development to grain size distribution, spill condition, and contaminant properties on the flow dynamics. At the capillary fringe, a reduction in vertical migration at the capillary fringe was observed as the presence of crude oil contaminants led to the depression of the water table. Also, the injection rate plays a vital role in the magnitude of vertical and horizontal migration under anisotropic hydraulic conductivity conditions; a lower injection rate promotes vertical migration, and a higher rate promotes horizontal migration. Kechavarzi et al. (2005) further carried out a quantitative 2D laboratory experiment to investigate the immiscible flow of crude oil in the vadose zone under transient flow. Their results demonstrate the adequacy of constructive pressure–saturation relations used in multiphase flow models. They proposed that hydraulic relations for three-fluid-phase models should account for residual NAPL saturation occurring after NAPL drainage as residual NAPL can act as a non-negligible constant source of contamination. Haggerty et al. (2004) identified the effect of using a single-rate mass transfer to describe a more extended timescale flow system because the effective dispersivity may increase at a longer scale system, resulting in the underestimation of contaminant spread within the pores.
Experimental characterization of crude oil contaminant entrapment and movement within unsaturated pores has shown that the dispersion process can cause the development of fingers due to pore-scale heterogeneity and fluid interactions (Ovdat and Berkowitz, 2006). Furthermore, residual waters are typically trapped as isolated macroscopic clusters as a result of crude oil displacement of water without buoyancy in water-wet heterogeneous sediments, with the degree of entrapment depending on the heterogeneity structure and crude oil penetration rate (Heib et al., 2011).
3.2 Field-based approach
Field-based measurement is required to obtain in situ subsurface information at contaminated sites. These approaches include, but are not limited to, core sample measurement, partitioning tracer techniques, geophysics measurement, and geochemical analysis. Several studies have provided a quantitative appraisal of the advective property of hydrocarbon contaminants estimated from tracer tests, revealing additional information about the dominant process that controls the migration of contaminants in the subsurface. Although Moreno-Barbero and Illangasekare (2006) discovered that non-equilibrium partitioning could enhance results for high-saturation zones, partitioning tracer studies are mostly utilized for crude oil residual saturation estimation. Using a geochemical method, Liu et al. (2010) described the biodegradation process of crude oil in detail. Their research revealed that aerobic conditions result in a higher rate of degradation than anaerobic settings, and the presence of chlorine atoms enhances biodegradation. Changes in alkalinity, microbial populations, and preferential elimination of BTEX components along the transport path can be used to map subsurface attenuation. Kim et al. (2013) discovered that tidal oscillations in a hydraulically connected water body add complexity to subsurface contaminant models. Also, capillary fringe affects the fraction of benzene biodegraded in saturated and unsaturated zones (Geng et al., 2017). Essaid et al. (1993) observed a complex distribution of crude oil in the subsurface oil, whose saturation distribution has been influenced by sediment heterogeneities and water table fluctuation. Hydrogeologic studies indicated that the crude oil migrated laterally within the capillary fringe, and the center of the oil body depressed the water-saturated zone boundary.
Due to the spatial challenges involved with contaminant movement within the subsurface, in situ field hydrogeological and biogeochemical approaches are limited in capturing the spatial and temporal variation in properties and processes of interest (Doro et al., 2015; Doro et al., 2022a). Tomographic methods, including hydraulic tomography (Cirpka et al., 2014) and tracer tomography (Doro et al., 2015), have been developed in hydrogeology to improve the spatial resolution of flow and transport properties. However, the resolved spatial scale is yet limited to a few meters-scale. Non-destructive geophysical techniques provide high-resolution spatial information about the subsurface condition and the fate of contaminants. Geophysical approaches have been demonstrated to be effective in contaminated site characterization and monitoring as they provide quantitative information on the contaminant flow process in both saturated and unsaturated media (Major et al., 2014). In addition, the geophysical technique is sensitive enough to detect biogeochemical changes caused by degradation and redox reactions, making it useful for environmental monitoring (Doro et al., 2022b). The use of electrical resistivity tomography to determine the spatial distribution of hydrocarbon pollution has received much attention (Mao et al., 2016). Electrical resistivity can be utilized to discover active sources of a dissolved plume by targeting minimal abnormal resistivity levels, identifying changes to the soil matrix, and determining the presence of a dissolved plume (Delgado-Rodiguea et al., 2018). This information can be utilized to create geoelectric models that can be compared with hydrocarbon concentration measurements for high-sensitivity interpretation (Arrubarrena-Moreno and Arango-Galvan, 2013). However, due to the direct relationship between electrical resistivity, water saturation, porosity, and soil texture, the sole usage of the electrical resistivity method in measuring the degree of biodegradation creates either an overestimation or underestimation (Koroma et al., 2015; Noel et al., 2016). According to Che-Alota et al. (2009), for electrical resistivity to image the pollutant distribution, the apparent bulk electrical conductivity of the saturated zone plume must be three to five times higher than the background values. As biodegradation occurs, contaminated zones become more conductive and may be masked by conductive soil (Oyinkuro and Wariebi, 2017).
Furthermore, microbial adaptation to petroleum as a carbon source can significantly impact the surrounding medium’s electrical signature (Allen et al., 2007). Changes in the vertical organization of microbial communities may also result from increased geoelectric measurements. This could guide microbial ecology sampling during natural bioremediation procedures and monitoring (Ehirim et al., 2016). Bacterial biodegradation of hydrocarbons alters groundwater redox conditions, forming zones with varying redox potentials. This results in the formation of redox gradients, which generates an electrochemical source for the SP signal via natural geo-battery processes (Naudet and Revil, 2005; Doro et al., 2022a). Significant negative SP anomalies have been recorded at hydrocarbon-contaminated sites, and a linear association between SP signals and redox potential measurements has been established. Abass et al. (2018) identified a rapid shift in SP signatures from negative values in polluted zones to positive values in clean zones, allowing the contaminated and clean zones to be distinguished and reflecting the extent of alteration at the contaminated site. This method provided an indirect indicator of bacterial activity and the presence of biofilms and mineral precipitates, which are likely to have played a critical role in the electrical current conduction of the plume and the oxidizing conditions outside the plume. Abdel Aal et al. (2006) identified an IP response related to intrinsic biodegradation in contaminated areas. This response was caused by the interfacial properties of microbial and mineral fluids, which caused significant electrical impulses in the polluted zones. With IP, valuable information on the mineralization of hydrocarbon pollutants as a result of contaminant degradation and dissolution can be obtained. These data could show the buildup of metallic minerals in hydrocarbon-contaminated soil (Moreira et al., 2017). Changes in groundwater chemistry observed from spectral-induced polarization data at an ongoing remediation site after acetate input and withdrawal can be utilized to find ideal conditions for contaminant extraction (Chen et al., 2012b). Because IP is sensitive to geochemical changes, the phase response can be used to understand spatial heterogeneity caused by pore size variability and free phase contaminant distribution (Arato et al., 2014).
3.3 Numerical modeling
Multiphase models have proven to help model the fate and transport of crude oil pollutants in soil and groundwater. Several scholars have utilized various numerical methods to solve the multiphase flow. These numerical models were developed to replicate specific environmental processes in the laboratory and field under specified environmental conditions. This approach provides a better understanding of the controlling factors influencing the fate and transport of crude oil contaminants (Peters and Svanstrom, 2019). Such factors include the influence of subsurface heterogeneity and capillary pressure–saturation–relative permeability (Pc-S-kr) relationships. The numerical models successfully reproduced the general features of experimental data sets when appropriate representations of heterogeneity and Pc-S-kr functions were used (Fagerlund et al., 2007). However, the dearth of detailed multiphase field data in different hydrogeological settings has hindered the ability to conduct comprehensive field-scale model testing. The unpredictability of the source-sink scenario in the unsaturated zone can be attributed to the combined actions of dissolution, biodegradation, and volatilization. The coupled system of moisture flow, airflow, and corresponding transport equations modeling demonstrated a gradual decrease in source intensity, corresponding to a decrease in the mass transfer process (Gaonkar et al., 2016). The simulation of a two-phase steady-state flow in the capillary force-dominated regime revealed a low sensitivity of the interfacial area, capillary pressure, and relative permeability to the initial fluid distribution, ruling out the use of capillary pressure measured in a displacement experiment for steady-state infiltration flow analysis (Li et al., 2019). Huang et al. (2020) confirmed this by observing specific differences in the relative permeability prediction and attributing them to inlet pore variation, which regulates the dispersion of pollutants. Multispecies reactive transport modeling results are influenced by variable degrees of heterogeneity and associated uncertainty in the aquifer’s physical parameters (Lu et al., 2005).
Several numerical models have been developed to understand biodegradation and natural attenuation better. For example, Rahbeh and Mohtar (2007) simulated the movement of dissolved crude oils in the 2D domain under the influence of oxygen-limited biodegradation, reaeration, and anaerobic biodegradation. This study discovered that aerobic degradation controls most of the remediation process, with stripping and volatilization accounting for a minor portion of the process. Rifai and Bedient (1990) analyzed crude oil pollutant attenuation in groundwater utilizing first-order solute decay due to advection, dispersion, sorption, and biodegradation processes under aerobic and anaerobic circumstances. Newell and Connor (1998) used estimated flow across the vadose zone to demonstrate the effects of LNAPLs on water table aquifers. Weaver (1996) and Zheng et al. (2010) investigated groundwater seepage as a function of time, precipitation infiltration, and excess pore-water pressure in the saturated and unsaturated zones. Siracusa et al. (2007) investigated subsurface pollutant reactions, microbial metabolisms, and microbial transport kinetics. Because multiphase flow models are computationally expensive, alternatives to continuum-based modeling have been developed, including analytic solutions, invasion-percolation modeling, and dissipative particle dynamics modeling methodologies. This model has been used to simulate steady-state or transient flow and advective and dispersive transport of dissolved oxygen and crude oil plumes.
4 Crude oil contaminant fate and transport implications of soil and groundwater resources
Most crude oil contaminant studies focus on developing an effective remediation plan while considering subsurface heterogeneity and the pollutant’s physical and chemical properties. A good cleanup requires a comprehensive investigation of the polluted location. However, evaluating so many variables is complex and time-consuming. Understanding baseline subsurface conditions before contamination, subsurface hydraulic variation in relation to contaminant distribution, and the impact of external influences such as groundwater fluctuation, climate change, and groundwater extraction are all critical components of crude oil contaminant research (Artiola et al., 2019). In the control of crude oil environmental contaminants, future event mitigation and site rehabilitation are commonly used. Given the harmful effects of crude oil pollutants on the environment and human health, developing an efficient crude oil spill mitigation strategy and responding swiftly are vital. According to Fang et al. (2013), crude oil contamination and soil/aquifer vulnerability are linked to bulk movement advection, and crude oil contamination spreads in saturated and unsaturated zones. In saturated and unsaturated zones, crude oil contamination is linked to groundwater level, net recharge, and hydraulic conductivity on climate change; changes in groundwater levels and increasing sea levels are highlighted since they are critical topics of contaminant research and vital for managing contaminants.
Groundwater accounts for the vast majority of the world’s fresh water. Depending on the recharge rate and underlying quality, groundwater can be found at depths ranging from 1 m in wetlands and marshy regions to several meters in other habitats (Winter, 1999). Groundwater, unlike soil, has a significant degree of mobility. As a result, the contaminant can be transported from one site to another via groundwater. Contaminants can travel from the upper soil layers to the lower soil layers, eventually reaching the groundwater zone and surface waters (Dorner et al., 2006). Because water travels considerably more slowly in aquifers than in surface water systems, it might take years, decades, or millennia for infiltrated water and pollutants to transit from aquifers to the point of discharge (Xia et al., 2010). This duration can vary according to the kind of pollution, aquifer characteristics, and flow path length. As a result, there is ample time for close interactions between the water and the soil and rock material, resulting in mineral composition changes in the water. Because of the significant variations in the geometry of hydrological systems and the soil and rock materials, the movement of contaminants from the source to sink has a varying impact on groundwater quality (natural background concentration) (Ohanmu et al., 2018). Naturally, disturbed groundwater will occasionally pose intolerable risks to human health or the environment (Riccardi et al., 2001). This is demonstrated by the arsenic poisoning of many people in Bangladesh and West Bengal, India, caused by a natural phenomenon rather than industrial contamination (Princewill and Shveda, 2022). In the event of immobile contaminants in the upper soil layers, leaching into groundwater could last for decades. For example, Mandocdoc and David (2008) identified dieldrin in quantities exceeding the drinking water requirements in several wells at the old US military installation Clark Air Base in the Philippines, 16 years after dieldrin was last used there.
5 Scientific challenges in fate and transport of crude oil contaminant research
In the early field investigations of crude oil contaminant flow, it was discovered that the subsurface was heterogeneous due to a variety of variable factors: capillary pressures have been shown to reduce crude oil contaminant relative permeability and prevent infiltration into fine-grained layers, and hydraulic gradients cause down-dip migration, spreading, pooling, and trapping of crude oil contaminants by these fine-grained (frequently water-saturated) layers (Dillard et al., 1997). In addition, it was discovered through experiments with a multiphase flow that fingers occur in crude oil contamination infiltration due to pore-scale and macro-scale variability. All of these factors point to the difficulty in pinpointing crude oil pollution source zones (Swartjes et al., 2012). Many decades have passed without an answer to whether contaminant transport models can be trusted. The reliability of groundwater flow and pollutant transport models is a tough challenge, even in homogenous porous media. It is vital to separate reality from it in order to employ models.
5.1 Hydraulic heterogeneity
The diversity of hydrogeological conditions and changing crude oil chemistry are expected to make field deployment of these systems difficult. The subsurface geometry must be considered in terms of vast amounts of subsoil because plumes frequently traverse soil volumes ranging from several hundred to thousands of cubic meters in a year (Swartjes and Grima, 2011). Even though these enormous volumes are typically formed of numerous irregular layers of different materials and commonly include entities of distinct subsurface material, the employment of contaminant transport models requires the production of a more or less regular-looking profile (Vallero, 2021). The typical, irregular, geochemical input parameters for massive aquifer material must be established. As a result, the description of both hydraulic and geochemical input parameters contributes to uncertainty in expected groundwater quality (Rezael et al., 2017). It is generally recognized that the availability of sufficient input parameters is crucial to the accuracy of model computations in any other model. When attempting to provide a complete picture of heterogeneities, it is vital to consider the consequences of interactions with hydraulically related aquifers and surface water. Because of the high concentration of saltwater in coastal aquifers with a freshwater–saltwater boundary, seawater intrusion becomes a dynamic and three-dimensional phenomenon that causes water quality oscillations on horizontal and vertical scale interactions. Also, contact angle hysteresis, wettability, complex pore geometry, and field-scale heterogeneity may arise, thus adding in another degree of complexity (Essaid et al., 2015). Despite advances in image processing and micro-modeling approaches, turning experimental results into data suitable for evaluating macroscopic, continuum-based multiphase flow models remains challenging, especially for large-scale studies. Multiphase modeling at the pore scale has also revealed how pore-scale processes affect macroscale constitutive relations (Ahrenholz et al., 2011). It is essential to assume a precise hydraulic gradient over a relatively broad area to be accurate in hydraulic situations.
5.2 Spatial and temporal heterogeneity
The concepts of homogeneity and heterogeneity in a system are inextricably linked to the scale at which the investigation is carried out. On a large scale, the same groundwater body can be termed homogeneous, whereas on a small scale, it can be deemed heterogeneous (Anderson, 1989). As a result, the study’s scope must be addressed from the start of any project. This scale contrasts between adjacent clean groundwater and public water supply groundwater sources that are only a few kilometers away when it comes to groundwater. For example, when building a risk management solution, the optimum size is determined by the type of solution and changes based on whether the solution is for source elimination or pathway interception (Cocarta et al., 2017). Field sites must be characterized in various hydrogeological situations to evaluate models that predict the fate of crude oil contaminants. Steefel et al. (2005) described the impact of pore-scale processes on the macroscopic constitutive relationships driving crude oil contaminant saturation, relative porosity (permeability), entrapment, and mass transfer rate. In recent years, non-invasive approaches for determining the source zone architecture of crude oil pollutants have made significant progress.
Due to their complexity, it is still difficult to estimate field concentration distributions and temporal oscillations in crude oil contamination sources. A multi-constituent crude oil contaminant’s chemical composition changes over time as the constituents dissolve and volatilize at varying rates (Fagerlund and Niemi, 2007). As a result, the effluent concentration histories for components dissolved in NAPLs differ. The breakdown of crude oil pollutants and changes in source architecture may result in a nonmonotonic drop in effluent concentration history. It has been proven in laboratory trials that heterogeneity, NAPL saturation, and interaction with moving water affect dissolution. In the presence of a solvent, the degradation of dissolved solutes can also affect the concentration gradient that promotes interphase mass transfer, thus creating a new complexity. However, recently developed hybrid models that dynamically combine pore-scale models for determining constitutive interactions with macroscale flow and transport show promise, but they face several computational and practical hurdles and are limited in their use. The key focus of future research should be on creating techniques for upscaling constitutive relations for field-scale studies.
5.3 Current state of crude oil contamination studies in Nigeria
Crude oil resources continue to be an important and growing source of revenue in Nigeria. A total of 13 multinational firms operate 606 oil fields (355 onshore and 251 offshore), 5,284 oil wells, and 7,000 km of oil and gas pipelines in the country, with the Niger Delta region serving as the epicenter of its production and processing operations (Badejo and Nwilo, 2004). The Niger Delta covers an area of roughly 20,000 square kilometers in southern Nigeria and accounts for approximately 8% of the country’s total land area. It is one of the world’s largest wetland and mangrove forest ecosystems. Sabotage, pipeline vandalism, well blowouts, and engineering failure have caused several crude oil spill accidents in Nigeria. Since the start of the Nigerian oil industry in 1960, 13 million tonnes of crude oil has been reported to have spilled in the Niger Delta due to terrorism, pipeline vandalism, and engineering failure (Figures 2A–C; Figure 3). The Nigerian Oil Spill Detection and Response Agency (NOSDRA) recently revealed statistics indicating that there have been a total of 12,566 confirmed oil spill occurrences from 2006 to the present, resulting in a total of 694,890 barrels of crude oil spilled. Crude oil contamination accounts for 81% of all-known oil leak occurrences (Figure 2D). Not only have agricultural resources in this region been poorly impacted due to crude oil contamination, but also surface water and groundwater resources have been rendered unfit for use in affected areas. The impact of the pipeline rupture that occurred between 1994 and 1996 in Baruwa, Lagos, is still noticeable, according to Uduebor and Ola (2016) and Ola et al. (2019), as a hydrocarbon thickness of 0.72 m was still discovered. Despite the contamination level being known, the reliance on unmanaged natural attenuation as a remediation strategy is grossly insufficient. The contamination still poses a significant risk to the region’s soil and groundwater resources, 20 years after the reported crude oil spill. Several studies on the environmental impact of oil spills in the Niger Delta and other tropical places worldwide have repeatedly revealed that areas immediately exposed to large or recurring oil spills or leaks frequently face long-term environmental challenges. Though there has been a reduction in the occurrence of oil spillage (Figure 4), the long-term effect of the previous spillage has created a continuous effect on land, soil, and the ecosystem at large. Even though the region’s potential for long-term prosperity has to be fully realized yet, its future is jeopardized by a slew of environmental issues, the most serious of which is oil contamination.
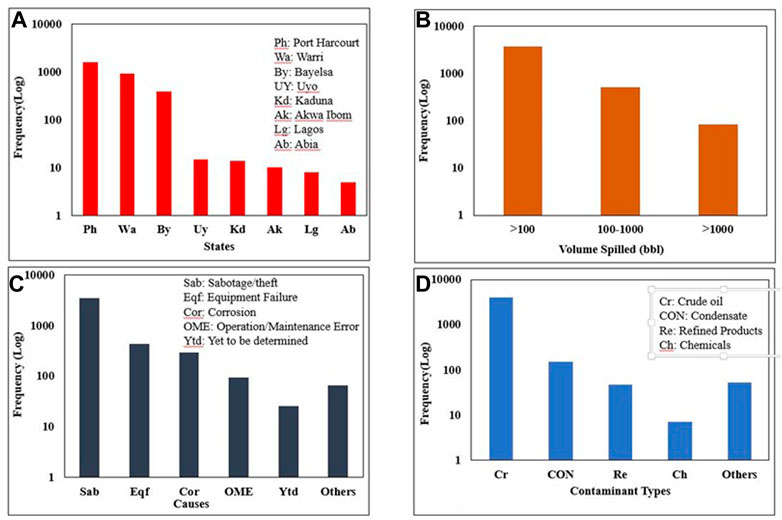
FIGURE 2. Histogram plot of (A) number of oil spills per state, (B) volume of oil spilled, (C) causes of spill, and (D) contaminant types (NOSDRA, 2021).
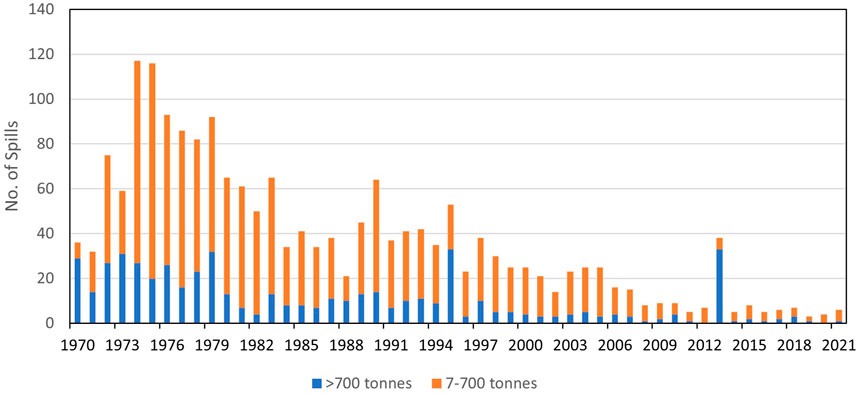
FIGURE 4. Number of medium (7–700 tonnes) and large (>700 tonnes) tanker spills 1970–2021 (ITOPF, 2021).
The influence of crude oil contamination on surface water and aquatic ecosystems in Nigeria, as well as its agriculture, biological diversity, human health, and groundwater sources, has been studied (Chukwu Okeah et al., 2019; Uko et al., 2020). With limited attention paid to the influence on groundwater contamination, the majority of this research concentrated on understanding the advection and dispersion processes of crude oil pollution on surface water (Alinnor and Nwachukwu, 2013). Even yet, dissolved sources of contamination from surface water eventually have an impact on the groundwater system. Most of the investigation that has been carried out on subsurface crude oil contamination uses a point sample approach to assess the total crude oil content in soil and water samples. Doherty and Otitoloju (2013) who measured the residual concentration values of soils and groundwater within the Ijegun oil spills in 2008 between 2009 and 2010 could only confirm the amount of contamination; no information was supplied as connected to the contaminant’s migratory pattern. The unregulated release of crude oil pollutants has increased the number of heavy metals in soils, plants, and groundwater, directly impacting human health and wellbeing (Moslen and Aigberua, 2018). Following a spill, the subsurface properties are likely to change due to the biodegradation process caused by microbial activity. As a result, knowledge of these alterations is crucial in developing a site-specific remediation strategy. The porosity and permeability of impacted soils have been reported to diminish due to the establishment of free-phase crude oil adhesion, generating a ganglion along the pore as a result of the replacement of water molecules by crude oils (Nwankwoala et al., 2020). This has the potential to be very important in shaping migratory patterns throughout the system, among other things.
Furthermore, as demonstrated by various researchers on the high degree of pollution, comprehending the distribution paths of contaminants in the subsurface has not been well investigated. According to Little et al. (2018), the parameters influencing the hazard posed by hazardous substances include their concentrations and distribution, the channels through which they are exposed, and the length of time they are exposed. The flow path of crude oil contamination in the Niger Delta region remains unclear. As of 2020, two scholarly publications have been published exploring the advection and dispersion processes of pollutant migration within the Niger Delta region. According to Giadom et al. (2015) and Ugbena et al. (2020), the groundwater flow velocity is 105 m per day and 121 m per day, respectively. This shows how easily toxins can spread across a large area, harming a more significant number of groundwater supplies. However, the scope of this investigation is constrained by the assumption that the salt tracer’s flow velocity is similar to the flow velocity of crude oil plumes. This non-conformity is thought to be caused by the density and viscosity of the crude oil pollution in the subsoil. Due to a lack of control information, it was difficult to ascertain the baseline subsurface condition and hydraulic distribution before the spill, making it impossible to determine how contamination affected the physical and flow parameters. This knowledge will help carry out a more effective remediation exercise in the real world. It is expected that future research focusing on flow path characterization of contaminant transport will be carried out to provide necessary information for futuristic prediction and identification of a suitable remediation approach.
Attempts have been made to clean up hazardous places. Linden and Palsson (2013) who conducted the first systematic investigation of oil contamination in Ogoniland discovered that, despite the natural conditions for crude oil degradation being favorable due to the region’s high temperature and rainfall, a low recovery rate had been recorded. The most often employed remediation approach, Remediation by Enhanced Natural Attenuation (RENA) (Figure 5), has been inefficient since soil pollution persists even after sites have been certified as remediated. Giadom and Tse (2014) detected residual contamination at a site that had undergone remediation with a 3.6-m smear zone that posed a significant danger to the environment. The applicability of the RENA technique has been demonstrated to be insufficient due to the level of pollution on both the vertical and lateral sides (Sam et al., 2017). The pollution level in the Nigeria Delta region has been estimated to be higher than 5 m, and leachates have been identified in groundwater, raising questions about the effectiveness of the RENA strategy’s tilling activity.
5.4 Management responses to crude oil contamination in Nigeria
The Niger Delta has been identified as one of the world’s most heavily oil-impacted places. The Niger Delta has been labeled “one of the world’s most highly oil-impacted areas” due to oil exploration activities in the region for more than 5 decades (Zabbey and Uyi, 2014). The Restoration by Enhanced Natural Attenuation (RENA) strategy is Nigeria’s popular contaminated land remediation technology. However, RENA is an unsuccessful technique for handling the problem due to the volume and extent of the spills, with oil entering the soil to depths of more than 5 m and leaking into groundwater aquifers (Ebuehi et al., 2005). The most prominent assessment of the level of contamination in the region is the United Nations Environment Programme (UNEP) report, (Environmental Assessment of Ogoniland) (UNEP, 2011). One of this study’s most significant findings was the need to develop and deploy oil pollution mitigation measures. Pollution policies are inadequate in Nigeria, which has been connected to several concerns, including a fragmented government structure, a lack of transparency in decision-making, and ineffective policy implementation, to name a few.
The Petroleum Act of 1969 established comprehensive petroleum regulations to prevent environmental pollution in diverse environmental media such as air, water, and soil. Other notable pieces of legislation were enacted, such as the Harmful Waste Act (1988) and the Environmental Impact Assessment Act (1992), but these were not designed to tackle the problem of polluted land management in the first place. Following an increase in oil production, oil pollution incidents (Nwilo and Badejo, 2005), and community protests, particularly in Ogoniland (Osaghae, 1995), the Nigerian government responded by enacting the Environmental Guidelines and Standards for the Petroleum Industry (EGASPIN) (2002). The EGASPIN spans from 2002 to the present and acts as the regulatory underpinning for Nigeria’s current environmental mandate. Many of EGASPIN’s environmental quality standard criteria were adapted from other countries, especially the United States (mainly from the USA). This has been attributed to Nigeria’s lack of technical ability and expertise in designing such rules and procedures. Concerns have been raised concerning the lack of contextualization in the current guidance and the fact that land use, soil type, and total organic carbon circumstances in the United States differ from those in Nigeria. Due to this disparity, the suitability and effectiveness of the risk assessment and management suggestions may be jeopardized.
6 Conclusion
The current state of research and management strategies in Nigeria, as well as our understanding of the transport processes and modeling conditions that govern the fate of crude oil contaminants in unconsolidated sediments, has been reviewed. Individual processes were discovered to be reasonably understood, and the utility of field and experimental research in estimating model parameters was demonstrated. Despite more than 50 years of research, there are still questions about crude oil contamination. Crude oil contamination is still a hot topic, thanks to complicated field-scale subsurface heterogeneity fueling ongoing research. Most previous studies of processes controlling the fate of crude oil contaminants have relied on laboratory-scaled experiments or various forms of numerical and analytical simulation. Although this provides pore-scale information about individual processes and their interactions, its application to field conditions necessitates further consideration of scaling and field-scale heterogeneity. The accuracy of numerical models has thus become a problem, the solution to which is embedded in detailed subsurface monitoring involving the integration of hydraulics, geophysical, and hydrological information with geological controls and biochemical processes. Due to a lack of active contaminant research and remediation, Nigeria urgently requires a paradigm shift in crude oil contaminant management. In Nigeria, the current understanding of the behavior of crude oil contaminants is woefully inadequate for optimizing management and remediation approaches. According to this review, the most significant shortcoming of crude oil contaminant studies in Nigeria is the lack of intensive baseline and monitoring studies to understand the subsurface heterogeneity and hydraulic variation that control the fate and transport of crude oil contaminants in the subsurface. In addition, no detailed subsurface quantitative framework research was conducted across all known contaminant sites in the country. A long-term perspective on monitoring contaminant behavior is required for a successful remediation exercise. Given how contaminant management is carried out in Nigeria, a national guide to detailed contaminant site management must include the best remediation practices and improve monitoring and evaluation methods.
Author contributions
MA: conceptualization, journal review, framework of the manuscript, and first draft preparation. MO: conceptualization, supervision, and review and editing. KD: conceptualization, supervision, and review and editing.
Funding
This review paper was funded in part by the African Union.
Acknowledgments
The authors wish to thank the African Union for providing financial support (scholarship) through the Pan African University Initiative.
Conflict of interest
The authors declare that the research was conducted in the absence of any commercial or financial relationships that could be construed as a potential conflict of interest.
Publisher’s note
All claims expressed in this article are solely those of the authors and do not necessarily represent those of their affiliated organizations, or those of the publisher, the editors, and the reviewers. Any product that may be evaluated in this article, or claim that may be made by its manufacturer, is not guaranteed or endorsed by the publisher.
References
Abbas, M., Jardani, A., Machour, N., and Dupont, J. P. (2018). Geophysical and geochemical characterisation of a site impacted by hydrocarbon contamination undergoing biodegradation. Near Surf. Geophys. 16 (2), 176–192. doi:10.3997/1873-0604.2017061
Abdel Aal, G. Z. A., Slater, L. D., and Atekwana, E. A. (2006). Induced-polarization measurements on unconsolidated sediments from a site of active hydrocarbon biodegradation. Geophysics 71 (2), H13–H24. doi:10.1190/1.2187760
Adipah, S. (2018). Introduction of petroleum hydrocarbons contaminants and its human effects. J. Environ. Sci. Public Health 3 (1). doi:10.26502/jesph.96120043
Ahrenholz, B., Niessner, J., Helmig, R., and Krafczyk, M. (2011). Pore-scale determination of parameters for macroscale modeling of evaporation processes in porous media. Water Resour. Res. 47 (7), 1–25. doi:10.1029/2010wr009519
Alazaiza, M. Y. D., Ngien, S. K., Bob, M. M., Kamaruddin, S. A., and Ishak, W. M. F. (2018). Non-aqueous phase liquids distribution in three-fluid phase systems in double-porosity soil media: Experimental investigation using image analysis. Groundw. Sustain. Dev. 7, 133–142. doi:10.1016/j.gsd.2018.04.002
Alinnor, I. J., and Nwachukwu, M. A. (2013). Determination of total petroleum hydrocarbon in soil and groundwater samples in some communities in Rivers State, Nigeria. J. Environ. Chem. Ecotoxicol. 5 (11), 292–297.
Allen, J. P., Atekwana, E. A., Atekwana, E. A., Duris, J. W., Werkema, D. D., and Rossbach, S. (2007). The microbial community structure in petroleum-contaminated sediments corresponds to geophysical signatures. Appl. Environ. Microbiol. 73 (9), 2860–2870. doi:10.1128/AEM.01752-06
Almutairi, M. S. (2018). An assessment of remediation strategies for Kuwaiti oil lakes. Environ. Geotech. 5 (6), 345–355. doi:10.1680/jenge.16.00022
Aminnaji, M., Rabbani, A., Niasar, J., and Babaei, M. (2019). Effects of pore-scale heterogeneity on macroscopic NAPL dissolution efficiency: A two-scale numerical simulation study. Water Resour. Res. 55 (11), 8779–8799. doi:10.1029/2019wr026035
Anderson, M. P. (1989). Hydrogeologic facies models to delineate large-scale spatial trends in glacial and glaciofluvial sediments. Geol. Soc. Am. Bull. 101 (4), 501–511. doi:10.1130/0016-7606(1989)101<0501:hfmtdl>2.3.co;2
Anderson, M. P. (1984). Movement of contaminants in groundwater: Groundwater transport-advection and dispersion. Groundw. Contam., 37–45.
Anderson, M. P., Woessner, W. W., and Hunt, R. J. (2015). Applied groundwater modeling: Simulation of flow and advective transport. Massachusetts, United States: Academic Press.
Arato, A., Wehrer, M., Biró, B., and Godio, A. (2014). Integration of geophysical, geochemical and microbiological data for a comprehensive small-scale characterizationof an aged LNAPL-contaminated site. Environ. Sci. Pollut. Res. 21 (15), 8948–8963. doi:10.1007/s11356-013-2171-2
Arrubarrena-Moreno, M., and Arango-Galván, C. (2013). Use of electrical resistivity tomography in the study of soil pollution caused by hydrocarbons: Case study in Puebla (México). Bol. La Soc. Geol. Mex. 65 (2), 419–426. doi:10.18268/BSGM2013v65n2a21
Artiola, J. F., Brusseau, M. L., and Liu, N. (2019). “The role of environmental monitoring in pollution science,” in Environmental and pollution science. 3rd ed., Issue i (Amsterdam, Netherlands: Elsevier Inc). doi:10.1016/B978-0-12-814719-1.00010-0
Atekwana, E. A., Atekwana, E. A., Werkema, D. D., Allen, J. P., Smart, L. A., Duris, J. W., et al. (2004). Evidence for microbial enhanced electrical conductivity in hydrocarbon-contaminated sediments: Microbial enhanced conductivity. Geophys. Res. Lett. 31 (23). doi:10.1029/2004GL021359
Azimi, R., Vaezihir, A., Lenhard, R. J., and Hassanizadeh, S. M. (2020). Evaluation of LNAPL behavior in water table inter-fluctuate zone under groundwater drawdown condition. Water 12 (9), 2337. doi:10.3390/w12092337
Aziz, R., Joekar-Niasar, V., and Martinez-Ferrer, P. (2018). Pore-scale insights into transport and mixing in steady-state two-phase flow in porous media. Int. J. Multiph. Flow 109, 51–62. doi:10.1016/j.ijmultiphaseflow.2018.07.006
Badejo, O. T., and Nwilo, P. C. (2004). Management of oil spill dispersal along the Nigerian coastal areas. Dept.of Surv. Geoinformatics, Univ. Lagos.
Basu, S., Yadav, B. K., Mathur, S., and Gupta, P. K. (2020). In situ bioremediation of toluene-polluted vadose zone: Integrated column and wetland study. CLEAN–Soil, Air, Water 48 (5-6), 2000118. doi:10.1002/clen.202000118
Bennett, P. D., Brumbach, B., Farmer, T. W., IV, P. L. F., and Hatheway, A. W. (1999). Remedy selection for cleanup of uncontrolled waste sites. Pract. Periodical Hazard. Toxic, Radioact. Waste Manag. 3 (1), 23–34. doi:10.1061/(asce)1090-025x(1999)3:1(23)
Biswas, B., Qi, F., Biswas, J. K., Wijayawardena, A., Khan, M. A. I., and Naidu, R. (2018). The fate of chemical pollutants with soil properties and processes in the climate change paradigm—a review. Soil Syst. 2 (3), 51. doi:10.3390/soilsystems2030051
Bodo, T. (2019). Deep issues behind the crisis in the Niger delta region: The case of oil exploration in ogoniland, rivers state, Nigeria. Asian J. Geogr. Res. 2 (1), 1–12. doi:10.9734/ajgr/2019/v2i130078
Brusseau, M. L., and Artiola, J. F. (2019). “Chapter 12 - chemical contaminants,” in Environmental and pollution science. 3rd ed. (Amsterdam, The Netherland: Elsevier Inc). doi:10.1016/B978-0-12-814719-1.00012-4
Brusseau, M. L. (2019b). “Chapter 15 - subsurface pollution,” in Environmental and pollution science. 3rd ed. (Amsterdam, The Netherland: Elsevier Inc). doi:10.1016/B978-0-12-814719-1.00015-X
Brusseau, M. L. (2019a). “Physical processes affecting contaminant transport and fate,” in Environmental and pollution science. 3rd ed. (Amsterdam, The Netherland: Elsevier Inc). doi:10.1016/B978-0-12-814719-1.00007-0
Brusseau, M. L., Walker, D. B., and Fitzsimmons, K., (2019a). “Physical-chemical characteristics of water,” in Environmental and pollution science. 3rd ed. (Amsterdam, The Netherland: Elsevier Inc). doi:10.1016/B978-0-12-814719-1.00003-3
Cerkirge, H. M., Al-Rabeh, A. H., and Gunay, N. (1992). Use of three generations of oil spill models during the Gulf War oil spills. Proc. 15th Arct. Mar. Oil Spill Program Tech. Seminar, 93–105.
Chang, S. W., Chung, I. M., Kim, I. H., Joo, J. C., and Moon, H. S. (2021). Importance of infiltration rates for fate and transport of benzene in high-tiered risk-based assessment considering Korean site-specific factors at contaminated sites. Water 13 (24), 3646. doi:10.3390/w13243646
Che-Alota, V., Atekwana, E. A., Atekwana, E. A., Sauck, W. A., and Werkema, D. D. (2009). Temporal geophysical signatures from contaminant-mass remediation. Geophysics 74 (4), B113–B123. doi:10.1190/1.3139769
Chen, P., Hubbard, S. S., Williams, K. H., Flores Orozco, A., and Kemna, A. (2012b). Estimating the spatiotemporal distribution of geochemical parameters associated with biostimulation using spectral induced polarization data and hierarchical Bayesian models. Water Resour. Res. 48 (5). doi:10.1029/2011WR010992
Chen, P., Li, Y., Lan, G., Liu, B., and Zhou, H. (2012a). “Oil spills detection and monitoring using airborn thermal infrared remote sensing in dalian Xingang oil pipeline explosion,” in Proceedings of the 2012 2nd International Conference on Remote Sensing, Environment and Transportation Engineering, Nanjing, China, 01-03 June 2012. doi:10.1109/RSETE.2012.6260647
Chukwu Okeah, G. O., Ushie Oyegun, L., and Uwadiae, C., (2019). Analysis of soil quality in a deltaic crude oil polluted environment Niger delta Nigeria. South Asian Res. J. Nat. Prod.,2(4).
Cirpka, O. A., Leven, C., Schwede, R., Doro, K., Bastian, P., Ippisch, O., et al. (2014). “Tomographic methods in hydrogeology,” in Tomography of the earth’s crust: From geophysical sounding to real-time monitoring (Cham: Springer), 157–176.
Cocârţă, D. M., Stoian, M. A., and Karademir, A. (2017). Crude oil contaminated sites: Evaluation by using risk assessment approach. Sustainability 9 (8), 1365. doi:10.3390/su9081365
Dago, O., Justin, A., and Harcourt, P. (2018). Groundwater study for the identification of crude oil pollution sources within a hydrocarbon processing facility in Niger delta, Nigeria. Int. J. Appl. Geol. Geophys. 6 (3). doi:10.9790/0990-0603035762
Darnault, C. J. G., DiCarlo, D. A., Bauters, T. W. J., Jacobson, A. R., Throop, J. A., Montemagno, C. D., et al. (2001). Measurement of fluid contents by light transmission in transient three-phase oil-water-air systems in sand. Water Resour. Res. 37 (7), 1859–1868. doi:10.1029/2000WR900380
Delgado-Rodríguez, O., Shevnin, V., Peinado-Guevara, H., and de Guevara-Torres, M. L. (2018). Characterization of Crude oil-contaminated sites based on geoelectrical methods of geophysical exploration. Geophysics, 85–93.
Dillard, L. A., Essaid, H. I., and Herkelrath, W. N. (1997). Multiphase flow modeling of a crude-oil spill site with a bimodal permeability distribution. Water Resour. Res. 33 (7), 1617–1632. doi:10.1029/97wr00857
Doherty, V. F., and Otitoloju, A. A. (2013). Monitoring of soil and groundwater contamination following a pipeline explosion and petroleum product spillage in Ijegun, Lagos Nigeria. Environ. Monit. Assess. 185, 4159–4170. doi:10.1007/s10661-012-2858-8
Dong, S., Dai, Z., Li, J., and Zhou, W. (2018). The scale dependence of dispersivity in multi-facies heterogeneous formations. Carbonates Evaporites 33 (1), 161–165. doi:10.1007/s13146-018-0421-6
Dorner, S. M., Anderson, W. B., Slawson, R. M., Kouwen, N., and Huck, P. M. (2006). Hydrologic modeling of pathogen fate and transport. Environ. Sci. Technol. 40 (15), 4746–4753. doi:10.1021/es060426z
Doro, K. O., Cirpka, O. A., and Leven, C. (2015). Tracer tomography: Design concepts and field experiments using heat as a tracer. Groundwater 53 (1), 139–148. doi:10.1111/gwat.12299
Doro, K. O., Ehosioke, S., and Aizebeokhai, A. P. (2020). Sustainable soil and water resources management in Nigeria: The need for a data-driven policy approach. Sustainability; 12(10):4204. doi:10.3390/su12104204
Doro, K. O., Emmanuel, E. D., Adebayo, M. B., Bank, C. G., Wescott, D. J., and Mickleburgh, H. L. (2022b). Time-lapse electrical resistivity tomography imaging of buried human remains in simulated mass and individual graves. Front. Environ. Sci. 10, 501. doi:10.3389/fenvs.2022.882496
Doro, K. O., Kolapkar, A. M., and Becker, A. M. (2021). “Using shallow subsurface geophysical models to guide restoration of old agricultural fields in northwestern Ohio,” in SEG/AAPG/SEPM first international meeting for applied geoscience & energy (Texas, United States: AAPG).
Doro, K. O., Leven, C., and Cirpka, O. A. (2013). Delineating subsurface heterogeneity at a loop of River Steinlach using geophysical and hydrogeological methods." Environ. earth Sci. 69 (2), 335–348. doi:10.1007/s12665-013-2316-0
Doro, K. O., Stoikopoulos, N. P., Bank, C. G., and Ferris, F. G. (2022a). Self-potential time series reveal emergent behavior in soil organic matter dynamics. Sci. Rep. 12 (1), 13531. doi:10.1038/s41598-022-17914-5
Dragun, J. (2021). “Recovery techniques and treatment technologies for petroleum and petroleum products in soil and groundwater,” in Petroleum contaminated soils (Florida, United States: CRC Press), 211–217.
Earl, N., Cartwright, C. D., Horrocks, S. J., Worboys, M., Swift, S., Kirton, J. A., et al. (2003). Review of the fate and transport of selected contaminants in the soil environment. Draft R and D Technical Report P5-079/TR1, 190.
Ebuehi, O., Abibo, I., Shekwolo, P., Sigismund, K., Adoki, A., and Okoro, I. (2005). Remediation of crude oil contaminated soil by enhanced natural attenuation technique. J. Appl. Sci. Enviromental Manag.
Ehirim, C. N., Adizua, O. F., and Okorie, I. P. C. (2016). Geoelectrical characterization of matured PetroleumHydrocarbon impacted soil in port harcourt, Nigeria. Asian J. Earth Sci. 9 (1), 9–15. doi:10.3923/ajes.2016.9.15
Ejiba, I. V., Onya, S. C., and Adams, O. K. (2016). Impact of oil pollution on livelihood: Evidence from the Niger delta region of Nigeria. J. Sci. Res. Rep. 12 (5), 1–12. doi:10.9734/jsrr/2016/26633
Esposito, G., Allersma, H. G. B., and Selvadurai, A. P. S. (1999). Centrifuge modeling of LNAPL transport in partially saturated sand. J. Geotechnical Geoenvironmental Eng. 125 (12), 1066–1071. doi:10.1061/(asce)1090-0241(1999)125:12(1066
Essaid, H. I., Bekins, B. A., and Cozzarelli, I. M. (2015). Organic contam-inant transport and fate in the subsurface: Evolution of knowledge and understanding. Water Resour. Researcg 51 (7), 4861–4902. doi:10.1002/2015WR017121
Essaid, H. I., Herkelrath, W. N., and Hess, K. M. (1993). Simulation of fluid distributions observed at a crude oil spill site incorporating hysteresis, oil entrapment, and spatial variability of hydraulic properties. Water Resour. Res. 29 (6), 1753–1770. doi:10.1029/93wr00370
Etkin, D. S., and Welch, J. (2005). Oil spill intelligence report international oil spill database: Trends in oil spill volumes and frequency, Int. Oil Spill Conf., 2005. doi:10.7901/2169-3358-1997-1-949
Fagerlund, F., Illangasekare, T. H., and Niemi, A. (2007). Nonaqueous-phase liquid infiltration and immobilization in heterogeneous media: 1. Experimental methods and two-layered reference case. Vadose Zone J. 6 (3), 471–482. doi:10.2136/vzj2006.0171
Fagerlund, F., and Niemi, A. (2007). A partially coupled, fraction-by-fraction modelling approach to the subsurface migration of gasoline spills. J. Contam. hydrology 89 (3-4), 174–198. doi:10.1016/j.jconhyd.2006.08.004
Fang, Y. Z., Ma, Z. M., and Luo, Y. Y. (2013). Research on vulnerability of aquifer to petroleum hydrocarbon basing on the ANN and GIS. Appl. Mech. Mater. 319, 331–336. doi:10.4028/www.scientific.net/amm.319.331
Gaonkar, O. D., Suresh Kumar, G., and Nambi, I. M. (2016). Numerical modelling on fate and transport of coupled adsorption and biodegradation of pesticides in an unsaturated porous medium. ISH J. Hydraulic Eng. 22 (3), 236–246. doi:10.1080/09715010.2016.1166073
Geng, X., Boufadel, M. C., and Cui, F. (2017). Numerical modeling of subsurface release and fate of benzene and toluene in coastal aquifers subjected to tides. J. Hydrology 551, 793–803. doi:10.1016/j.jhydrol.2016.10.039
Giadom, F. D., Akpokodje, E. G., and Tse, A. C. (2015). Determination of migration rates of contaminants in a hydrocarbon-polluted site using non-reactive tracer test in the Niger Delta, Nigeria. Environ. Earth Sci. 74 (1), 879–888. doi:10.1007/s12665-015-4094-3
Giadom, F. D., and Tse, A. C. (2014). Groundwater contamination and environmental risk assessment of a hydrocarbon contaminated site in Eastern Niger Delta, Nigeria. J. Environ. Earth Sci. 5 (14), 123–133.
Grathwohl, P., Eberhardt, C., Klenk, I., Rügner, H., and Maier, U. (2020). “Transverse vertical dispersivity in aquifer materials: Implications on mass transfer across the capillary fringe and the length of steady state plumes,” in Groundwater (Florida, United States: CRC Press), 19–20.
Gupta, P. K., Yadav, B., and Yadav, B. K. (2019). Assessment of LNAPL in subsurface under fluctuating groundwater table using 2D sand tank experiments. J. Environ. Eng. 145 (9). doi:10.1061/(asce)ee.1943-7870.0001560
Güven, O., Molz, F. J., and Melville, J. G. (1986). Comment on “An Advection-Diffusion Concept for Solute Transport in Heterogeneous Unconsolidated Geological Deposits” by Gillham et al. Water Resour. Res. 22 (1), 89–91. doi:10.1029/WR022i001p00089
Hadley, P. W., and Newell, C. (2014). The new potential for understanding groundwater contaminant transport. Groundwater 52 (2), 174–186. doi:10.1111/gwat.12135
Hadley, P. W., and Newell, C. (2014). The new potential for understanding groundwater contaminant transport. Groundwater 52 (2), 656–658. doi:10.1111/gwat.12246
Haggerty, R., Harvey, C. F., Freiherr von Schwerin, C., and Meigs, L. C. (2004). What controls the apparent timescale of solute mass transfer in aquifers and soils? A comparison of experimental results. Water Resour. Res. 40 (1). doi:10.1029/2002wr001716
Heib, V. I., Neuweiler, I., Ochs, S., and Färber, A. (2011). Experimental investigation on front morphology for two-phase flow in heterogeneous porous media. Water Resour. Res. 47 (10). doi:10.1029/2011WR010612
Held, R. J., and Illangasekare, T. H. (1995a). Fingering of dense nonaqueous phase liquids in porous media: 1. Experimental investigation. Water Resour. Res. 31 (5), 1213–1222. doi:10.1029/95WR00428
Hochstetler, D. L., Rolle, M., Chiogna, G., Haberer, C. M., Grathwohl, P., and Kitanidis, P. K. (2013). Effects of compound-specific transverse mixing on steady-state reactive plumes: Insights from pore-scale simulations and Darcy-scale experiments. Adv. water Resour. 54, 1–10. doi:10.1016/j.advwatres.2012.12.007
Horn, S. A., and Neal, C. P., (2005). The Atlantic empress sinking-a large spill without environmental disaster. 2005 International Oil Spill Conference, IOSC, 510
Huang, X., Zhou, W., and Deng, D. (2020). Validation of pore network modeling for determination of two-phase transport in fibrous porous media. Sci. Rep. 10 (1), 20852. doi:10.1038/s41598-020-74581-0
Illangasekare, T. H., Ramsey, J. L., Jensen, K. H., and Butts, M. B. (1995). Experimental study of movement and distribution of dense organic contaminants in heterogeneous aquifers. J. Contam. Hydrology 20 (1–2), 1–25. doi:10.1016/0169-7722(95)00045-W
Iovenitti, J. L., Nitao, J. J., and Bishop, D. J. (1992). Vadose zone investigations at the lawrence livermore national laboratory superfund site: An overview. Washington, D.C: Second USA/CIS Joint Conference Environmental Hydrology and Hydrogeology, 1–17.
ITOPF (2021). Oil tanker spill statistics. Available at: https://www.itopf.org/knowledge-resources/data-statistics/statistics/.
Jarvis, N., Koestel, J., and Larsbo, M. (2016). Understanding preferential flow in the vadose zone: Recent advances and future prospects. Vadose Zone J. 15 (12), 11. doi:10.2136/vzj2016.09.0075
Kechavarzi, C., Soga, K., and Illangasekare, T. H. (2005). Two-dimensional laboratory simulation of LNAPL infiltration and redistribution in the vadose zone. J. Contam. Hydrology 76 (3–4), 211–233. doi:10.1016/j.jconhyd.2004.09.001
Kim, H. S., and Swanson, J. C. (2001). “Fate and transport modeling of contaminants,” in Salem sound. Report to the marine monitoring and research technical series. MMRTS-01-01.
Kim, M., Hong, S. H., Won, J., Yim, U. H., Jung, J. H., Ha, S. Y., et al. (2013). Petroleum hydrocarbon contaminations in the intertidal seawater after the Hebei Spirit oil spill – effect of tidal cycle on the TPH concentrations and the chromatographic characterization of seawater extracts. Water Res. 47 (2), 758–768. doi:10.1016/j.watres.2012.10.050
Koroma, S., Arato, A., and Godio, A. (2015). Analyzing geophysical signature of a hydrocarbon-contaminated soil using geoelectrical surveys. Environ. Earth Sci. 74 (4), 2937–2948. doi:10.1007/s12665-015-4326-6
Kovalick, W. W., and Montgomery, R. H., 2014. Developing a Program for contaminated site management in low and middle income countries.
Li, Z., Galindo-Torres, S., Yan, G., Scheuermann, A., and Li, L. (2019). Pore-scale simulations of simultaneous steady-state two-phase flow dynamics using a lattice Boltzmann model: Interfacial area, capillary pressure and relative permeability. Transp. Porous Media 129 (1), 295–320. doi:10.1007/s11242-019-01288-w
Lindén, O., and Pålsson, J. (2013). Oil contamination in ogoniland, Niger delta. Ambio 42 (6), 685–701. doi:10.1007/s13280-013-0412-8
Little, D. I., Holtzmann, K., Gundlach, E. R., and Galperin, Y. (2018). Sediment hydrocarbons in former mangrove areas, southern ogoniland, eastern Niger delta, Nigeria. Coast. Res. Libr. 25, 323–342. doi:10.1007/978-3-319-73016-5_14
Liu, M., Zhang, Y., and Hu, L. (2010). Modeling the fate and transport of halohydrocarbons contaminants in groundwater aquifers of a northern city. 4th Int. Conf. Bioinforma. Biomed. Eng. ICBBE. doi:10.1109/ICBBE.2010.5515046
Lu, G., Zheng, C., and Wolfsberg, A., (2005). Effect of uncertain hydraulic conductivity on the simulated fate and transport of BTEX compounds at a field site. J. Environ. Eng., 131, 767, 776. doi:10.1061/(asce)0733-9372(2005)131:5(767)
Maier, R. M. (2019). Biological processes affecting contaminants transport and fate. Environ. Pollut. Sci., 131–146. doi:10.1016/b978-0-12-814719-1.00009-4
Major, W., Versteeg, R., Day-Lewis, F. D., Johnson, T. C., and Lane, J. W. (2014). Optimized enhanced bioremediation through 4D geophysical monitoring and autonomous data collection. Process. Analysis ESTCP Proj. 1–98.
Mandocdoc, M., and David, C. P. (2006). Dieldrin contamination of the Groundwater in a former US military Base. Clean 36, 10–11.
Mansi, A. H., Castillo, M. P., and Bernasconi, G. (2017). Controlled laboratory test for the investigation of LNAPL contamination using a 2.0 GHz ground penetrating radar. Boll. Geofis. Teor. Appl. 58 (3).
Mao, D., Lu, L., Revil, A., Zuo, Y., Hinton, J., and Ren, Z. J. (2016). Geophysical monitoring of hydrocarbon-contaminated soils remediated with a bioelectrochemical system. Environ. Sci. Technol. 50 (15), 8205–8213. doi:10.1021/acs.est.6b00535
Michel, J., and Fingas, M. (2016). “Oil Spills: Causes, consequences, prevention, and countermeasures,” in Fossil fuels: Current status and future directions (USA: University of South Carolina), 159–201.
Moldan, A. G. S., Jackson, L. F., McGibbon, S., and Van Der Westhuizen, J. (1985). Some aspects of the Castillo de Bellver oilspill. Mar. Pollut. Bull. 16 (3), 97–102. doi:10.1016/0025-326X(85)90530-2
Molins, S., Mayer, K. U., Amos, R. T., and Bekins, B. A. (2010). Vadose zone attenuation of organic compounds at a crude oil spill site - interactions between biogeochemical reactions and multicomponent gas transport. J. Contam. Hydrology 112 (1–4), 15–29. doi:10.1016/j.jconhyd.2009.09.002
Moreira, A., Innocenti, C., Helene, L. P., and Côrtes, R. P. A. (2017). Integration of geoelectric and geochemical data in the evaluation of natural attenuation in a diesel contaminated site in São Manuel (Brazil). Geofísica Int. 56 (3), 1–15. doi:10.22201/igeof.00167169p.2017.56.3.1814
Moreno-Barbero, E., and Illangasekare, T. H. (2006). Influence of dense nonaqueous phase liquid pool morphology on the performance of partitioning tracer tests: Evaluation of the equilibrium assumption. Water Resour. Res. 42 (4). doi:10.1029/2005WR004074
Moslen, M., and Aigberua, A. (2018). Heavy metals and hydrocarbon contamination of surface water in azuabie Creek within bonny estuary, Nigeria. J. Appl. Sci. Environ. Manag. 22 (7), 1083. doi:10.4314/jasem.v22i7.15
Mugunthan, P., Mohan, R., Thornburg, T., Martin, A., and Mahoney, M. (2016). Considerations for modeling contaminant transport at confined disposal sites. March 1–31.
Myers, T. (2012). Potential contaminant pathways from hydraulically fractured shale to aquifers. Groundwater 50 (6), 872–882. doi:10.1111/j.1745-6584.2012.00933.x
Naidu, R. (2013). Recent advances in contaminated site remediation. Water, Air, & Soil Pollut. 224 (12), 1705–1711. doi:10.1007/s11270-013-1705-z
Naudet, V., and Revil, A. (2005). A sandbox experiment to investigate bacteria-mediated redox processes on self-potential signals. Geophys. Res. Lett. 32 (11), L11405. doi:10.1029/2005gl022735
Newell, C. J., and Connor, J. A. (1998). Characteristics of dissolved petroleum Crude oil plumes. D.C., United States: American Petroleum Institute.
Noel, C., Gourry, J. C., Deparis, J., Blessing, M., Ignatiadis, I., and Guimbaud, C. (2016). Combining geoelectrical measurements and CO2Analyses to monitor the enhanced bioremediation of hydrocarbon-contaminated soils: A field implementation. Appl. Environ. Soil Sci. 2016, 1–15. doi:10.1155/2016/1480976
Nwachukwu, A. N., and Osuagwu, J. C. (2014). Effects of oil spillage on groundwater quality in Nigeria. Am. J. Eng. Res. 3 (6), 271–274.
Nwankwoala, H., Amadi, A., Omofuophu, E., and Ibrahim, H. (2020). Risk evaluation and modeling of soils contaminated with polycyclic aromatic hydrocarbons (PAHs) in parts of bonny island, Niger delta, Nigeria. Ann. Civ. Environ. Eng. 4 (1), 015–026. doi:10.29328/journal.acee.1001021
Nwilo, P. C., and Badejo, O. T. (2005). “Oil spill problems and management in the Niger Delta,” in International oil spill conference (D.C., United States: American Petroleum Institute), 567–570.
Ohanmu, E. O., Bako, S. P., Ohanmu, E., and Ohanmu, O. O. (2018). Environmental implications, properties and attributes of crude oil in the oil-producing states of Nigeria. Ecologia 9 (1), 1–9. doi:10.3923/ecologia.2019.1.9
Ojuri, O. O., Ola, S. A., Rudolph, D. L., and Barker, J. F. (2010). Contamination potential of tar sand exploitation in the Western Niger-delta of Nigeria: Baseline studies. AU J. Technol. 14 (2), 119–128. doi:10.1007/s10064-009-0239-5
Ola, S. A., Fadugba, O. G., Adekoya, J. A., Babatola, J. O., Ajayi, O. O., Ojuri, O. O., et al. (2019). Petroleum hydrocarbon contaminated groundwater remediation using 21st century technology: Baruwa community, Lagos State Nigeria as a case study. IOP Conf. Ser. Mater. Sci. Eng. 640 (1), 012090. doi:10.1088/1757-899x/640/1/012090
Osaghae, E. E. (1995). The Ogoni uprising: Oil politics, minority agitation and the future of the Nigerian state. Afr. Aff. 94 (376), 325–344. doi:10.1093/oxfordjournals.afraf.a098833
Ossai, I. C., Ahmed, A., Hassan, A., and Hamid, F. S. (2020). Remediation of soil and water contaminated with petroleum hydrocarbon: A review. Environ. Technol. Innovation 17, 100526. doi:10.1016/j.eti.2019.100526
Osuji, L. C., and Ezebuiro, P. E. (2006). Hydrocarbon contamination of a typical mangrove floor in Niger Delta, Nigeria. Int. J. Environ. Sci. Technol. 3 (3), 313–320. doi:10.1007/BF03325939
Ovdat, H., and Berkowitz, B. (2006). Pore-scale study of drainage displacement under combined capillary and gravity effects in index-matched porous media. Water Resour. Res. 42 (6). doi:10.1029/2005WR004553
Oyinkuro, O., and Wariebi, K. (2017). Hydrocarbon spill site characterization by electrical resistivity tomography and ground penetrating radar methods - a review. Asian J. Environ. Ecol. 4 (3), 1–9. doi:10.9734/ajee/2017/36742
Pan, Y., Zhang, Q., Yu, Y., Tong, Y., Wu, W., Zhou, Y., et al. (2021). Three-dimensional migration and resistivity characteristics of crude oil in heterogeneous soil layers. Environ. Pollut. 268, 115309. doi:10.1016/j.envpol.2020.115309
Parker, J. C. (1989). Multiphase flow and transport in porous media. Rev. Geophys. 27 (3), 311–328. doi:10.1029/rg027i003p00311
Pepper, I. L., Gerba, C. P., and Gentry, T. J. (2015). Environmental microbiology. San Diego, CA: Academic Press.
Peters, G., and Svanström, M. (2019). “Modelling environmental transport and fate of contaminants,” in Environmental sustainability for engineers and applied scientists (Cambridge, England: Cambridge University Press). doi:10.1017/9781316711408.005
Petri, B. G., Fučík, R., Illangasekare, T. H., Smits, K. M., Christ, J. A., Sakaki, T., et al. (2015). Effect of NAPL source morphology on mass transfer in the vadose zone. Groundwater 53 (5), 685–698. doi:10.1111/gwat.12284
Qin, X. S., Huang, G. H., and He, L. (2009). Simulation and optimization technologies for petroleum waste management and remediation process control. J. Environ. Manag. 90 (1), 54–76. doi:10.1016/j.jenvman.2008.07.002
Rahbeh, M. E., and Mohtar, R. H. (2007). Application of multiphase transport models to field remediation by air sparging and soil vapor extraction. J. Hazard. Mater. 143 (1–2), 156–170. doi:10.1016/j.jhazmat.2006.09.098
Reidenbach, M. A., Koseff, J. R., and Monismith, S. G. (2007). Laboratory experiments of fine-scale mixing and mass transport within a coral canopy. Phys. Fluids 19 (7), 075107. doi:10.1063/1.2752189
Ren, W., Ershadnia, R., Wallace, C. D., LaBolle, E. M., Dai, Z., de Barros, F. P., et al. (2022). Evaluating the effects of multiscale heterogeneous sediments on solute mixing and effective dispersion. Water Resour. Res. 58 (9), e2021WR031886. doi:10.1029/2021wr031886
Rezaei, H., Bozorg-Haddad, O., and Loáiciga, H. A. (2017). Effect of hydraulic conductivity uncertainty on in situ bioremediation of groundwater contaminated with dissolved petroleum Crude oils. J. Irrigation Drainage Eng. 143 (12), 04017049.
Riccardi, C., Berardi, S., Di Basilio, M., Gariazzo, C., Giardi, P., and Villarini, M. (2001). Environmental assessment of a site contaminated by organic compounds. J. Environ. Sci. Health - Part A Toxic/Hazardous Subst. Environ. Eng. 36 (6), 957–970. doi:10.1081/ESE-100104124
Rifai, H. S., and Bedient, P. B. (1990). Comparison of biodegradation kinetics with an instantaneous reaction model for groundwater. Water Resour. Res. 26 (4), 637–645. doi:10.1029/WR026i004p00637
Rolle, M., and Kitanidis, P. K. (2014). Effects of compound-specific dilution on transient transport and solute breakthrough: A pore-scale analysis. Adv. Water Resour. 71, 186–199. doi:10.1016/j.advwatres.2014.06.012
Rolle, M., Maier, U., and Grathwohl, P. (2011). “Contaminant fate and reactive transport in groundwater,” in Dealing with contaminated sites) (Dordrecht: Springer), 851–885.
Sam, K., Coulon, F., and Prpich, G. (2017). Management of petroleum hydrocarbon contaminated sites in Nigeria: Current challenges and future direction. Land Use Policy 64, 133–144. doi:10.1016/j.landusepol.2017.01.051
Scheibe, T. D., Murphy, E. M., Chen, X., Rice, A. K., Carroll, K. C., Palmer, B. J., et al. (2015a). An analysis platform for multiscale hydrogeologic modeling with emphasis on hybrid multiscale methods. Groundwater 53, 38–56. doi:10.1111/gwat.12179
Seigneur, N., Mayer, K. U., and Steefel, C. I. (2019). Reactive transport in evolving porous media. Rev. Mineralogy Geochem. 85 (1), 197–238. doi:10.2138/rmg.2019.85.7
Sethi, R., and Di Molfetta, A. (2019). “Transport of immiscible fluids,” in Groundwater engineering (Cham: Springer), 249–262.
Šimůnek, J., and van Genuchten, M. T. (2016). “Contaminant transport in the unsaturated zone: Theory and modeling,” in The handbook of groundwater engineering (Amsterdam The Nethherland: CRC Press), 221–254.
Siracusa, G., La Rosa, A. D., Musumeci, L., and Maiolino, G. (2007). Modelling of contaminant migration in unsaturated soils. WIT Trans. Ecol. Environ. 102. doi:10.2495/SDP070441
Steefel, C. I., DePaolo, D. J., and Lichtner, P. C. (2005). Reactive transport modeling: An essential tool and a new research approach for the Earth sciences. Earth Planet. Sci. Lett. 240 (3-4), 539–558. doi:10.1016/j.epsl.2005.09.017
Swartjes, F. A., and Grima, J. (2011). “Groundwater-related risk assessment,” in Dealing with contaminated sites (Dordrecht: Springer), 749–785.
Swartjes, F. A. (2011). “Introduction to contaminated site management,” in Dealing with contaminated sites (Dordrecht: Springer), 3–89. doi:10.1007/978-90-481-9757-6
Swartjes, F. A., Rutgers, M., Lijzen, J. P. A., Janssen, P. J. C. M., Otte, P. F., Wintersen, A., et al. (2012). State of the art of contaminated site management in The Netherlands: Policy framework and risk assessment tools. Sci. Total Environ. 427, 1–10. doi:10.1016/j.scitotenv.2012.02.078
Theodorakis, C., and Walker, T. D. (2014). “Environmental processes,” in Encyclopedia of toxicology. Third Edition3 (Amsterdam, Netherlands: Elsevier). doi:10.1016/B978-0-12-386454-3.01011-32
Uduebor, M. A., and Ola, S. A. (2016). Hydrocarbon remediation by natural attenuation at Baruwa, Lagos Nigeria. Electron. J. Geotechnical Eng. 21, 501–512.
Ugbena, K. G., Tse, A. C., and Akpokodje, E. G. (2020). Modeling soluble contaminant migration by advection process in subsurface water in the Eastern Niger Delta, Nigeria. World Sci. News 139 (2), 102–114.
Uko, M., Udotong, I., Ofon, U., Umana, S., and Abraham, N. (2020). Effect of hydrocarbon contamination on the microbial diversity of freshwater sediments within akwa ibom state, Nigeria. J. Chem. Environ. Biol. Eng. 8, 32. doi:10.11648/j.jcebe.20200402.11
Vallero, D. (2021). Environmental systems science: Theory and practical applications. Amsterdam, Netherlands: Elsevier.
Van Geel, P. J., and Sykes, J. F. (1994). Laboratory and model simulations of a LNAPL spill in a variably-saturated sand, 1. Laboratory experiment and image analysis techniques. J. Contam. Hydrology 17 (1), 1–25. doi:10.1016/0169-7722(94)90075-2
Weaver, J. W. (1996). “November. Application of the Crude oil spill screening model to field sites,” in Non-aqueous phase liquids (NAPLs) in subsurface environment: Assessment and remediation, 788–799.
Williams, S. D., Ladd, D. E., and Farmer, J. J. (2006). Fate and transport of petroleum crude oils in soil and ground water at big south fork national river and recreation area. Washington: Tennessee and Kentucky, 2002–2003. U. S. Geological Survey Scientific Investigations Report 2005-5104.
Winter, T. C. (1999). Ground water and surface water: A single resource. Collingdale, Pennsylvania: Diane Publishing, 1139.
Xia, Y., Li, H., Boufadel, M. C., and Sharifi, Y. (2010). Hydrodynamic factors affecting the persistence of the Exxon Valdez oil in a shallow bedrock beach. Water Resour. Res. 46 (10). doi:10.1029/2010WR009179
Yoon, H., Chojnicki, K. N., and Martinez, M. J. (2019). Pore-scale analysis of calcium carbonate precipitation and dissolution kinetics in a microfluidic device. Environ. Sci. Technol. 53 (24), 14233–14242. doi:10.1021/acs.est.9b01634
You, X., Liu, S., Dai, C., Guo, Y., Zhong, G., and Duan, Y. (2020). “Contaminant occurrence and migration between high- and low-permeability zones in groundwater systems: A review,” in Science of the total environment (Ammsterdam Netherland: Elsevier B.V), 743. doi:10.1016/j.scitotenv.2020.140703
Zabbey, N., Sam, K., and Onyebuchi, A. T. (2017). Remediation of contaminated lands in the Niger Delta, Nigeria: Prospects and challenges. Sci. Total Environ. 586, 952–965. doi:10.1016/j.scitotenv.2017.02.075
Zabbey, N., and Uyi, H. (2014). Community responses of intertidal soft-bottom macrozoobenthos to oil pollution in a tropical mangrove ecosystem, Niger Delta, Nigeria. Mar. Pollut. Bull. 82 (1), 167–174. doi:10.1016/j.marpolbul.2014.03.002
Keywords: crude oil, soil, groundwater, contaminant, fate and transport
Citation: Adeniran MA, Oladunjoye MA and Doro KO (2023) Soil and groundwater contamination by crude oil spillage: A review and implications for remediation projects in Nigeria. Front. Environ. Sci. 11:1137496. doi: 10.3389/fenvs.2023.1137496
Received: 04 January 2023; Accepted: 04 April 2023;
Published: 09 May 2023.
Edited by:
Sören Thiele-Bruhn, University of Trier, GermanyReviewed by:
Bassam Tawabini, King Fahd University of Petroleum and Minerals, Saudi ArabiaManuel Cánovas, Catholic University of the North, Chile
Copyright © 2023 Adeniran, Oladunjoye and Doro. This is an open-access article distributed under the terms of the Creative Commons Attribution License (CC BY). The use, distribution or reproduction in other forums is permitted, provided the original author(s) and the copyright owner(s) are credited and that the original publication in this journal is cited, in accordance with accepted academic practice. No use, distribution or reproduction is permitted which does not comply with these terms.
*Correspondence: Margaret A. Adeniran, YWRlbmlyYW5tYXJnYXJldDkzQGdtYWlsLmNvbQ==