- 1College of Veterinary Medicine, Shandong Agricultural University, Tai’an, Shandong, China
- 2The Affiliated Taian City Central Hospital of Qingdao University, Tai`an, Shandong, China
- 3Shandong Zhongnong Puning Pharmaceutical Co.Ltd., Tai’an, Shandong, China
- 4Shandong Province, Pingyi County Animal Husbandry Development Promotion Center, Linyi, Shandong, China
Chromium (Cr) is the seventh most abundant chemical element in the Earth’s crust, and Cr(III) and Cr(VI) are common stable valence states of Cr. Several Cr-containing substances, such as FeOCr2O3 and stainless-steel products, exist in nature and in life. However, Cr(VI) is toxic to soil, microorganisms, and plants and poses a serious threat to human health through direct and indirect exposure. By collecting published journal literature, we found that Cr(VI) can cause acute and chronic toxicity in organisms and has carcinogenic effects, and the mechanisms causing these toxicity include endoplasmic reticulum stress, autophagy and apoptosis. However, the relationship between these mechanisms remains unclear. Many methods have been researched to purify chromium, but each of these methods has its own advantages and disadvantages. Therefore, this review summarizes the hazards of chromium and the mechanisms of chromium toxicity after entering cells and provides a number of methods for chromium contamination management, providing a direction for the next step in chromium toxicology and contamination decontamination research.
1 Introduction
Heavy metal pollution is a thorny issue in current environmental pollution and is a global environmental problem (1). Cr is a heavy metal largely used in industrial activities (leather tanning, wood preservation and metal finishing, etc.). Cr-containing products are ubiquitous in the atmosphere, soil, building materials, household products, and even food (Vincent and Lukaski, 2018). Chromium pollution mainly comes from tannery, textile production and printing and dyeing leading to pollution of the atmosphere and water bodies, food processing equipment, packaging may also lead to chromium pollution. However, Cr is also a constant threat to people’s lives. Therefore, a clear and comprehensive understanding of the environmental toxicity of this heavy metal is urgently needed.
Cr can exist in chemical valence states 0–6, but only the trivalent [Cr(III)] and hexavalent [Cr(VI)] states are generally stable (Sharma et al., 2020). For decades, Cr(III) has been considered essential for mammals; however, no clear animal model of Cr deficiency has been established to prove this idea (Xu et al., 2018). Studies on the toxicity of Cr(III) showed that large amounts of Cr(III) orally affect the absorption of trace elements in animals and damage the brain, kidney, and liver. Compared with Cr(III), Cr(VI) is more toxic to soil, microorganisms, plants, animals, and humans. Cr(VI) affects soil pH and enzyme activities, leading to reduced soil fertility; causes oxidative damage, DNA damage, and death of Cr-intolerant microorganisms; affects plant enzyme activity and photosynthesis, leading to stunted growth and reduced yields (Ao et al., 2022); and accumulates in animal tissues and organs, leading to acute or chronic toxicity and carcinogenesis. Direct exposure of humans to Cr (VI) causes poisoning and cancer, but Cr (VI) also accumulates in plants and animals and enters the human body indirectly through the food chain and other forms, causing harmful effects on the human body.
The mechanisms by which Cr poisoning produces these hazards are gradually being revealed. For instance, Cr (VI) can cause cellular oxidative stress, autophagy, apoptosis, pyroptosis, endoplasmic reticulum (ER) stress, and inflammatory response, resulting in cellular damage due to overproduction of reactive oxygen species (ROS) (Sharma et al., 2020). In conclusion, Cr contamination leads to a wide range of toxic effects to the ecosystem and human health. Therefore, physical, chemical, and biological means to remediate Cr contamination have been investigated. However, previous studies on Cr toxicity are scattered and do not form a system.
This review summarizes the toxic effects and mechanisms of Cr and the methods to manage Cr pollution. It also discusses the interconnection between these mechanisms and provides ideas for further research on Cr toxicity and pollution management.
2 Hazards of Cr exposure
2.1 Ecotoxicity
2.1.1 Toxicity to soil and plants
Plants play a vital role in the overall ecological cycle, and Cr (VI) contamination in soil can inhibit plant growth and metabolism and even lead to plant death. In nature, Cr (III) exists as FeOCr2O3, and Cr(VI) combines with oxygen to form CrO42- or Cr2O72-. Cr (III) is the most stable while Cr(VI) is the most phytotoxic form of Cr (Sharma et al., 2020). When Cr slag accumulates, Cr redox consumes a large number of anions, causing the pH to rise and the soil to become alkaline (Liu et al., 2019), which can inhibit soil alkaline phosphatase activity and soil dehydrogenase activity. Cr in the soil is eventually absorbed by green plants growing in the soil and accumulates in plant tissues.
The heavy metals accumulated in plants can affect nutrient uptake and physiological balance, thereby disrupting development and growth. Metal uptake in plants is concentrated in the roots, but some species also redistribute a greater proportion of metals to aboveground tissues, especially leaves. Specifically, Cr(VI) disrupts plant metabolic functions and enzyme activities, leading to oxidative stress, photosynthesis and respiration inhibition, delayed germination, premature defoliation, and reduced yields. It may also induce genotoxicity and even cause plant death (Wang and Chao, 2020). Cr(VI) toxicity affects plant growth and impedes its essential metabolic processes. It reduces plant growth and productivity, photosynthetic pigments, and antioxidant enzyme activity (Ali et al., 2015). The reduced growth rate and smaller leaves after treatment with potassium dichromate may be due to oxidative damage to photosynthetic pigments caused by Cr (VI), thereby decreasing photosynthesis. In peas, excessive Cr (VI) exposure disrupts the structure and function of the pea root plasma membrane, leading to reduced photosynthesis and poor plant growth (Kushwaha and Singh, 2020). In cotton, Cr (VI) toxicity significantly reduces plant height, root length, leaf, stem, and root fresh and dry weights (Farooq et al., 2016). In maize, Cr (VI) stress reduces leaf area, rachis formation, 100-grain weight, and shoot biomass (Anjum et al., 2017). These heavy metals that accumulate in plants can eventually harm animals and humans through the food chain.
2.1.2 Toxicity to microorganisms
Microorganisms play an important role in soil material cycle, and soil Cr (VI) contamination inhibits the growth and metabolism of microorganisms. When assessing the toxicity of Cr (VI), microorganisms are more sensitive indicators of ecotoxicity than soil invertebrates and plants (Zhang et al., 2022). The alpha diversity (species richness and evenness in samples) of microbial communities is usually associated with ecosystem stability and function, and Cr-contaminated soils exhibit reduced bacterial alpha diversity (Sheik et al., 2012). The significant differences in bacterial abundance and diversity between the 0 and >20.00 cm depth layers suggest that the heavy metal content in the soil has a certain depth gradient that affects the bacterial groups at different depths (Liu et al., 2019). High concentrations of Cr(VI) can be lethal to microorganisms that are not tolerant to Cr(VI) growth. However, microorganisms that are Cr tolerant can resist Cr toxicity and convert the more toxic Cr(VI) to the less toxic Cr(III). Thus, people have thought of using Cr-tolerant strains to clean Cr-contaminated soil and water.
2.2 Danger of Cr pollution to humans and animals
2.2.1 Accumulative poisoning
In general, Cr poisoning can be divided into acute and chronic poisoning. Cr(VI) and Cr(III) can cause poisoning, but Cr(VI) is more toxic than Cr(III). China stipulates that the maximum allowable concentration of chromium in ground water is Cr(III) 0.50 mg L-1 and Cr(VI) 0.05 mg L-1 (Ministry of Ecology and Environment of the People’s Republic of China, GB5749-85), and the maximum allowable discharge standard of Cr(VI) and its compounds in industrial wastewater is 0.50 mg L-1 (The People’s Republic of China Ministry of Ecology and Environment Integrated Wastewater Discharge Standards GB 8978-1996).
The role of Cr(III), which was once considered essential for mammals, is controversial. Some believe that Cr deficiency is associated with impaired glucose tolerance, fasting hyperglycemia, increased body fat, dyslipidemia, and impaired fertility (Jomova and Valko, 2011). Cr(III) may regulate the metabolism of carbohydrates, lipids, and even proteins by enhancing the function of insulin. However, this claim is not supported by sufficient evidence. The mainstream view is that excessive intake of Cr(III) can cause some harm to the body. For example, excessive intake of Cr(III) may disrupt the absorption of trace elements in the serum and brain (Liu et al., 2017). Cr accumulates in large quantities in the heart, liver, and kidneys over a long period of time and leads to impaired metal absorption and metabolism in chickens (Liu et al., 2016). Oral administration of CrCl3 can reduce the growth performance of chickens, cause pathological lesions such as renal tubular atrophy and structural degeneration of the glomerulus, and affect the antioxidant capacity of the kidney (Liu et al., 2015). The addition of different concentrations of CrCl3 to drinking water induces oxidative stress in the chicken brain, and Cr(III) at doses up to 50.00% can significantly increase the levels of malondialdehyde and hydrogen peroxide in brain tissue (Cheng et al., 2016). High doses and prolonged exposure to Cr(III) induce oxidative stress and hepatocyte injury (Fan et al., 2015).
The acute oral toxicity of Cr(VI) (1900.00 mg kg-1–3,300.00 mg kg-1) is significantly higher than that of Cr(III) and can damage several tissues throughout the body, and post-mortem examination of rats receiving lethal doses of Cr(VI) results in diffuse hemorrhage, gastritis, and enteritis (Katz and Salem, 1993). Effects of occupational and non-occupational Cr(VI) ingestion include gastrointestinal symptoms, hypotension, and liver and kidney failure (Alvarez et al., 2021). Cr(VI) poisoning can damage multiple tissues throughout the body, including liver, kidney, reproductive organs. Cr(VI) can cause oxidative damage to the liver in chickens (Chen et al., 2017), inflammatory heart tissue damage, mitochondrial impairment, autophagy (Wang et al., 2022a), and oxidative damage to the kidney in roosters (Wan et al., 2017). Cr(VI) enters the body through drinking water and accumulates in the brain and serum over time, disrupting the absorption of trace elements in the brain and serum of animals (Zhu et al., 2018). Excessive intake of Cr(VI) can cause inflammatory brain damage in chickens (Guo et al., 2021). Damage to the male reproductive system, including sperm, has been observed in experimental animals exposed to Cr(VI) (Jomova and Valko, 2011). Cr(VI) can damage dog hearts by causing oxidative damage and altering A-TPase content (Lu et al., 2019). Cr(VI) is also known to have toxic effects on the immune system, causing a series of changes such as a dramatic decrease in the levels of leukocytes, erythrocytes and neutrophils and an increase in lymphocytes in mice (Monga et al., 2022a). Cr accumulation in fish may enter the body through food and cause serious health hazards (Rahman et al., 2012). Cr can also cause hair loss, headaches, diarrhea, nausea, and vomiting in humans. In addition, Cr(VI) increases the expression of tumor necrosis factor-alpha, interleukin 6, and heat shock proteins in chicken liver, triggering inflammatory damage (Wang et al., 2020a). Recently, the neurotoxicity caused by Cr(VI) exposure has also received attention. Rats exposed to Cr(VI) exhibit long aggressive behavior toward “intruder” rats and take long to recognize previously encountered rats, thus confirming the social memory deficit observed in humans (Wise et al., 2022). In addition, Cr(VI) is neurotoxic, and Cr(VI) exposure can cause various behavioral abnormalities in fish, such as irritability and rapid surfacing. The abnormal behavior may be due to the neurotoxic effects and stimulation of the body’s sensory system after Cr(VI) exposure (Ahmed et al., 2013).
2.2.2 Carcinogenicity
The carcinogenicity of Cr(VI) has also been studied experimentally, and chronic or high exposure to Cr in animals and humans can increase the risk of cancer.
In humans, long-term exposure to Cr-containing environments can lead to an increased probability of developing stomach, lung, bladder, and pancreatic cancers. In 1987, investigators in Liaoning Province, China studied the association between Cr exposure and cancer. Data showed that between 1970 and 1978, the mortality rate from stomach cancer was higher in Liaoning Province than in nearby uncontaminated areas, and the mortality rate from lung cancer also increased (Beaumont et al., 2008). Similarly, exposure to Cr(VI) in drinking water increases the likelihood of stomach tumors in humans and animals (Jomova and Valko, 2011). Cancer mortality or incidence rates in industries associated with Cr(VI) exposure have been analyzed, and results showed that workers exposed to Cr(VI) have an approximately 7.00% higher risk of cancer and an increased risk of respiratory, oral, throat, prostate, and gastric cancers compared with the general population of the same age and sex (Deng et al., 2019).
In animals, Cr toxicity increases the risk of developing cancer. Animal experiments showed that exposure to Cr(VI) alone does not cause skin tumors but increases the sensitivity of mice to UV light, whereas the presence of Cr(VI) in drinking water increases the frequency of skin tumors in UV-irradiated hairless mice (Zhitkovich, 2011). The National Toxicology Program designed a 2-year rodent study to determine the potential hazards of human exposure to Cr(VI) in drinking water. The study found a significant increase in the incidence of oral squamous cell carcinoma in F344/N rats and in the combined incidence of adenoma and small intestine cancer in B6C3F1 mice in animals exposed to Cr-containing compounds (Mezencev and Auerbach, 2021).
2.2.3 Genotoxicity
Cr(III) cannot enter the cell through the cell membrane, but Cr(VI) can enter the cell through non-specific ion channels and is eventually reduced to Cr(III). The reduction of Cr(VI) generates unstable radicals, such as thiol groups, hydroxide radicals, hydrogen peroxide, and superoxide anions, which have a wide range of DNA-damaging effects. Cr-DNA formation adducts can lead to chromosome fragmentation and mutagenesis (Zhitkovich, 2011). Earlier studies have shown that Cr(VI) compounds can produce different types of DNA damage, such as single-strand breaks, base instability sites, and DNA–protein crosslinks, while selectively inhibiting the activity of enzymes, such as glutathione reductase (VonHandorf et al., 2021). An increase in Cr(VI) level leads to oxidative damage to tissues, and oxidative damage generates large amounts of ROS; overproduction of ROS an lead to deleterious biological effects and irreversible damage by promoting the oxidation of proteins, lipids, carbohydrates, and DNA (Scharf et al., 2014). Cr(VI) is genotoxic, and nearly 400 out of 450 samples from the experiment showed that soluble Cr(VI) compounds are mutagenic in bacteria. In addition, soluble Cr(VI) compounds can induce a wide range of genetic effects in yeast and insects.
3 Mechanisms of Cr toxicity
3.1 ER stress
Cr(VI) induces ER stress in cells. When Cr(VI)-induced ER stress occurs, protein kinase R-like endoplasmic reticulum kinase (PERK) is activated (Alvarez et al., 2021), eukaryotic translation initiation factor 2α is phosphorylated, and transcription factor 4 (ATF4) is activated (Cho et al., 2011), accompanied by changes in glucose regulatory protein 78 (GRP78 or BiP). Elevated PERK, a typical indicator of ER stress, has been detected after 20 μM Cr(VI) treatment of chicken embryonic fibroblasts (DF-1) for 10, 20, and 30 h, suggesting that Cr(VI) induces ER stress in DF-1 cells (Chen et al., 2019). The addition of Cr(VI) upregulates the expression of GRP78 and p-PERK in A549 cells and causes ER stress. The addition of an ER stress inhibitor (4PBA) reduces apoptosis and autophagy, and ER stress has been hypothesized to occur earlier than autophagy and apoptosis. In a previous study (Ge et al., 2019), an inhibitor of apoptosis (Z-VAD-FMK) and an inhibitor of autophagy (3-MA) were added separately to investigate the relationship between autophagy and apoptosis. Results showed that Z-VAD-FMK can inhibit autophagy, but 3-MA cannot inhibit apoptosis. Thus, many connections exist between Cr(VI)-induced ER stress, autophagy, and apoptosis. Cr(VI) entry into cells increases ROS production, resulting in mitochondrial damage and mitochondrial ROS release, disruption of ER protein folding, and ER stress (Liang et al., 2019). Exposure to Cr(VI) elevates the expression levels of ER stress-related proteins (GRP78/Bip and PERK) and COX-2 in chicken liver cancer cell line (LMH), demonstrating that Cr(VI) can induce ER stress phase in LMH cells (Liu et al., 2020).
3.2 Autophagy
Autophagy is a lysosomal catabolic pathway that degrades damaged and aged cytosolic macromolecules and organelles. Induced autophagy can maintain cell survival under nutrient depletion and oxidative stress conditions. The expression of LC3-II, a typical indicator of autophagy, is elevated after treatment of chicken embryonic fibroblasts (DF-1) with 20 μM Cr(VI), indicating that Cr(VI) induces autophagy in DF-1 cells (Chen et al., 2019). Exposure of LMH cells to Cr(VI) increases the expression of autophagy-related proteins Beclin1 and LC3-II (Liu et al., 2020). Cr(VI) can also lead to mitochondrial dysfunction and imbalance in mitochondrial dynamics, triggering severe mitochondrial autophagy (Wang et al., 2021a). Cr(VI) treatment can damage mitochondrial morphology and function and decrease mitochondria-related index TOMM20, demonstrating that Cr(VI) causes mitochondrial damage in DF-1 cells. Moreover, damaged mitochondria may be cleared by autophagy, leading to autophagy of mitochondria (Xu et al., 2020). Different doses of Cr(VI) intake induce Parkin-mediated mitochondrial autophagy in chicken brain tissue (Guo et al., 2021). Cr(VI) treatment induces the formation of autophagosomes, increases the expression of autophagy-related proteins, such as LC3 II, and decreases the expression of P62 in cells. Silencing the HMGA2 gene with siRNA successfully prevents these changes, demonstrating that HMGA2 is involved in Cr(VI)-induced autophagy and plays a key role, leading to the speculation that HMGA2 is an important marker of Cr(VI)-induced autophagy or other toxic mechanisms (Yang et al., 2017).
3.3 Interactions between ER stress, autophagy, and apoptosis
ER stress has many links to autophagy and apoptosis, suggesting that various intracellular pathways are integral and collaborate with each other to maintain cellular homeostasis. Cr(VI) induces autophagy in DF-1 cells through ER stress and can reduce Cr(VI) damage through the ER stress-COX-2 pathway, suggesting a relationship between ER stress and autophagy with COX-2 (Liu et al., 2022). In addition, the molecular mechanism of Cr(VI)-induced autophagy and damage in DF-1 cells is dependent on ER stress-regulated COX-2 overexpression. Cr(VI) can increase COX-2 levels, but this increase can be inhibited by ER stress inhibitors (NS-398), suggesting that COX-2 plays an important role in ER stress-induced autophagy. Cr(VI) can induce ER stress by upregulating ER stress-related proteins GRP78 and PERK. However, the expression of COX-2 is also upregulated after ER stress, which further increases the expression of Beclin1 and LC3-II and eventually triggers autophagy. These results suggest that COX-2 plays a key role between Cr(VI)-induced ER stress and autophagy (Chen et al., 2019). Cr(VI) induces autophagy and ER stress in LMH cells, and autophagy improves after the addition of the ER stress inhibitor 3-MA, suggesting an association between ER stress and autophagy (Liu et al., 2020).
Autophagy can alleviate Cr(VI)-induced apoptosis to some extent, and the underlying mechanism may be due to the fact that autophagy reduces apoptosis by removing damaged mitochondria to reduce intracellular stress. Cr(VI) induces elevated LC3-II and activates autophagy in L-02 hepatocytes, and the ROS-AKT-mTOR pathway is associated with Cr(VI)-induced autophagy, whereas inhibition of autophagy promotes Cr(VI)-induced apoptosis (Liang et al., 2018). Cr(VI)) activates the Akt, NF-kB, and MAPK pathways and induces cell death through the action of ROS (Lee et al., 2014). Cr(VI) entry into cells induces ROS overproduction, causing mitochondrial damage and mitochondrial ROS release. Part of the mitochondria are degraded by the autophagic pathway, whereas the mitochondria that are not degraded show destruction of membrane lipids, leading to outer membrane rupture, triggering the release of CytC and other substances to the cell membrane and causing apoptosis (Liang et al., 2019). The reduction of Cr disrupts the redox balance in cancer cells, which induces intracellular DNA damage and activation of the p53 pathway, ultimately leading to aspartase-dependent and mitochondria-mediated apoptotic cell death (Chen et al., 2021). Cr(VI) treatment increases the levels of glycolysis-related proteins, such as HK2, GLUT1, PKM2, and LDHA in A549 cells. It also increases the rates of glucose consumption and lactate and ATP production, indicating that Cr(VI) can induce aerobic glycolysis in A549 cells. ER stress and autophagy play important roles in Cr(VI)-induced aerobic glycolysis. While aerobic glycolysis plays an important role in resistance to Cr(VI)-induced apoptosis, ATF4 is involved in Cr(VI)-induced aerobic glycolysis in A549 cells. Therefore, ATF4 may be involved in Cr(VI)-induced apoptosis (Gao et al., 2020). Cr(VI)-induced apoptosis in DF-1 cells attenuates the decrease in mitochondrial membrane potential (MMP) and ER stress indicators, demonstrating that apoptosis attenuates mitochondrial damage and ER stress. ATF-6 plays a key role in this process (Zhang et al., 2021a). The relationship between ER stress, apoptosis, and autophagy may be that Cr(VI)-induced autophagy rescues Cr(VI)-induced mitochondrial damage by phagocytosis of damaged mitochondria, which consequently reduces apoptosis due to mitochondrial damage, and ER may be the intersection of autophagic and apoptotic pathways (Ge et al., 2019).
3.4 Others
Cr(VI) causes cellular ER stress, autophagy, and apoptosis. It can also cause oxidative stress, induce an inflammatory response, and pyroptosis. Cr(VI) increases inflammation-related markers, such as TNF-α, COX-2, and NF-κB/p65, in human bronchial epithelial cells (BEAS-2B), demonstrating an inflammatory response (Roy et al., 2016). A study in 2019 found that Cr(VI) induces the pyroptosis of DF-1 cells, and the mechanism of interaction is possibly related to the activation of CaSR (Zhu et al., 2019). Long-term exposure to Cr(VI) induces oxidative stress in the chicken brain (Hao et al., 2017). Cr(VI) exposure activates the Nrf2 pathway and induces oxidative damage in the mouse small intestine (Zhu et al., 2021a). In addition, Cr(VI) can bind directly to proteins, causing the loss of their biological functions (Scharf et al., 2014).
In conclusion, Cr(VI) entry into cells induces a series of responses, including ER stress, autophagy, and apoptosis, and a close connection exists between these responses. However, the mechanisms underlying these connections are still not well defined and need further investigation (Figure 1).
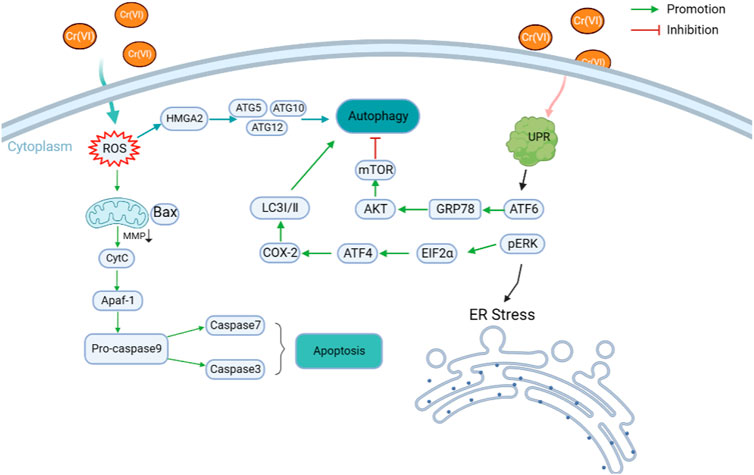
FIGURE 1. Mechanism of chromium toxicity in cells. The entry of Cr(VI) into cells induces a series of responses, including ER stress, autophagy and apoptosis, and these pathways also interact with each other through certain mechanisms.
4 Remediation of Cr contamination
4.1 Physical remediation
Physical remediation refers to the use of physical technologies, including adsorption, coagulation, and nanotechnology, to effectively separate and transform harmful substances from the soil into non-toxic or less toxic substances. Physical adsorption is considered to be an effective technique, but the cost of the adsorbent affects the feasibility of the process. Finding or synthesizing an adsorbent material with high adsorption efficiency and reusability will be the next research direction for this method. The higher the concentration of chromium contamination the faster the adsorption rate, and generally the efficiency of physical adsorption is between 80.00% and 99.00%.
El-Mehalmey et al. constructed a composite of amino-derived zirconium carboxylate metal-organic backbone and silica using silica as a porous solid carrier. The experimental data showed that the composite has good Cr(VI) adsorption capacity and potential for industrial wastewater treatment (El-Mehalmey et al., 2018). The use of rhizobia and yeast as adsorbents and their combination with nanomaterials to form multi-walled carbon nanotubes provide an ideal adsorption of Cr(VI) (Sathvika et al., 2018). Ionic solids (ethyl cetyl dimethyl ammonium bromide) impregnated with phosphorylated chitosan (ISPC) were applied for the adsorption of Cr(VI) in industrial wastewater. ISPC shows a high adsorption capacity of 266.67 mg g-1 at pH 3.0 with a total Cr recovery of 94.00% (Kahu et al., 2016). Lotus seed pods (LSPs) are an excellent and low-cost biomaterial for the removal of Cr(VI) from aqueous solutions by absorption, redox, and reabsorption. Data show that the maximum removal of Cr(VI) by LSPs in aqueous solution is 153.85 mg g-1 (Shi et al., 2020). Polypyrrole/sugarcane bagasse composites (PPy/SCB) can adsorb and reduce Cr(VI) in wastewater with adsorption capacities of 156.00–251.00 mg g-1 (Chen and Pan, 2021). Nitrogen-doped hydrocarbon prepared from bamboo and NH4Cl is a cheap and efficient adsorbent for Cr(VI) removal from water. The main mechanisms are electrostatic attraction, reduction, and complexation (Li et al., 2021). A novel nano-biosorbent was constructed by encapsulating a nanoscale metal-organic framework (Cu-TMA) in a chitosan matrix coated with vanadium pentoxide, and this material was found to possess excellent adsorption and a removal capacity of 92.43%–96.95% toward Cr(VI) from wastewater (Mahmoud et al., 2021). Palladium nanoparticles 3.00–25.00 nm in size were synthesized for the reduction of Cr(VI). The material is reusable and provides a novel method for the detoxification of Cr(VI) in wastewater treatment (Tripathi and Chung, 2020). In general, physical remediation is often applied only to highly polluted soils or waters. However, this technique is labor and resource intensive and reduces soil fertility.
4.2 Chemical reduction
Chemical reduction involves the use of chemical redox reactions to convert harmful substances in soil and wastewater into non-toxic or less toxic substances. Oxidation–reduction reactions are common remediation strategies for converting Cr(VI) to Cr(III). The chemical reduction method is simple, low cost, and has the advantage of short cycle time, and is generally not limited by the pollution concentration for Cr(VI) The reduction efficiency of Cr(VI) can reach up to 100.00%. However, its use is limited due to its tendency to cause secondary pollution. The combination of this technology and bioremediation technology can be considered, which can make up for the shortcomings of the long bioremediation cycle and reduce secondary pollution.
Li et al. found that 100.00% removal of Cr(VI) could be achieved within 60 min under acidic conditions by using pyrite as a reducing agent and catalyst (Li et al., 2020). S-nZVI composites supported by chitosan-stabilized biochar were used to remediate Cr(VI) contamination, with removal effects of 221.84 and 244.07 mg g-1 at 25°C for 15 and 120 min, respectively. The main mechanism of action of this composite decontamination is redox reaction, supplemented by electrostatic attraction and chelation reaction (Xu et al., 2021). Cr(VI) can be converted to Cr(III) by electrochemical reduction in acidic sodium alginate solution using a gold electrode. Acidic pH also favors the electrochemical reduction of Cr(VI), and the current intensity is proportional to Cr(VI) concentration (Butter et al., 2021). Molasses can remediate Cr(VI)-contaminated groundwater chemically and biologically; in specific, 3 g L-1 of molasses can biologically restore 100 mg L-1 of Cr(VI), and adding a small amount of ascorbic acid (0.05 L-1) promotes Cr(VI) bioreduction (Yang et al., 2021). Chemical leaching and reduction involve the removal of water-soluble and acid-soluble components by initial chemical leaching, followed by the reaction of the reducing agent with the residual Cr(VI) in the soil and in the deep soil by electron transfer. These techniques can reduce the Cr(VI) content in the soil to below the screening value (3.00 mg kg-1) at a low cost, which is effective for the remediation of Cr(VI)-contaminated soil. The removal rate of Cr(VI) is 62.70% at 0.02 mol L-1 oxalic acid and citric acid with a leaching time of 45 min using a 5:1 liquid–solid ratio (Wang et al., 2021b). For Cr(VI), nature also has certain restorative functions. For instance, Cr(VI) can be reduced to Cr(III) in soils or water containing small amounts of Fe(III). In many cases, iron-rich soils contain stable Fe(II) even in an aerobic environment. Thus, the chemical reduction of Cr(VI) may be promoted regardless of the environment (Whitaker et al., 2018). Chemical conversion reduction is commonly used to remediate Cr contamination with high conversion efficiency. However, a large amount of toxic solid sludge is generated during the precipitation of Cr(III).
4.3 Bioreduction
Bioreduction removes contaminated Cr(VI) using microorganisms, plants, and other organisms. Bioreduction is environment friendly and economical. Therefore, it is considered effective for the remediation of heavy metal-contaminated environments. Compared with traditional remediation techniques, bioremediation has many advantages, but still has some shortcomings, such as time-consuming, limited to moderately polluted sites, and susceptible to external environmental interference. The removal rate of Cr(VI) (50.00 mg/L) reached 100.00% and Cr(VI) (100.00 mg/L) reached 92.00% within 24 h after co-culture of bacteria and algae (Roestorff and Chirwa, 2019). Therefore, we should make full use of the synergistic effect of native and exogenous organisms to achieve the best bioremediation effect.
4.3.1 Microbial reduction
Microorganisms have multiple metal chelating mechanisms and have a large metal uptake capacity. Microorganisms use various toxic compounds as a source of energy for growth and development through respiration, fermentation, and metabolism (Ayangbenro and Babalola, 2017). The mechanisms involve active and passive processes, including transport, accumulation, biosorption, and redox reactions (Gutierrez-Corona et al., 2016). A large number of strains with Cr(VI)-reducing activity have been identified. For example, the application of Bacillus subtilis can mitigate the toxic effects of Cr on wheat by converting Cr(VI) to Cr(III) in wheat (Seleiman et al., 2020). Strain G161, isolated from CrO42-contaminated soil near a tannery in Wenzhou, China, exhibits CrO42 tolerance and can reduce Cr(VI) to Cr(III) (Ge et al., 2016). Cr11 strains isolated from tannery wastewater in Kanpur district exhibits Cr(VI)-reducing activity; in addition, Cr11 enhances seed germination and promotes wheat growth in the presence of Cr(VI) in the soil (Sagar et al., 2012). Rhodobacter sphaeroides SC01 strain can remove Cr(VI) from wastewater. The highest removal rate is achieved under the following conditions: temperature of 35°C, pH of 7.20, NaCl concentration of 5.00 g L-1, light intensity of 4,000 lx, and initial cell concentration (OD680) of 0.15. Cr is mostly enriched in the cell membrane as Cr(III) after reduction. The addition of Na4P2O7 and (NaPO3)6 salts increases the Cr(VI) reduction capacity by 15.00%. The combination of biological and chemical techniques provides an efficient solution that can be used for the remediation of Cr(VI)-containing industrial wastewater (Su et al., 2021). The microorganism Lysinibacillus sp. JLT12 can remove Cr(VI) from wastewater. In addition to adsorption and direct reduction, Lysinibacillus sp. JLT12 an also remove passive layers on magnetite to facilitate the reduction of Cr(VI) (Zhu et al., 2021b). Six species of bacteria belonging to the genera Bacillus and Pseudomonas were identified by Aashna Monga et al. that can tolerate up to 20.00 mg L-1 Cr(VI), and demonstrated that the in vitro metal removal capacity of this culture ranged from 90.00% to 96.00% at pH 7.0, 4.00 mg L-1 Cr(VI), and 37°C (Monga et al., 2022b).
CrO42 uptake via sulfate transporter proteins is a common process in bacteria and fungi, although it is slightly different in fungi. Fungal organisms are effective biosorbents for the removal of toxic metals from wastewater. Fungi have a unique property that their cell walls have a strong affinity for metals. Consequently, various enzymes produced by fungi are effective in heavy metal removal from wastewater (Ukhurebor et al., 2021). The enzyme glucose oxidase from Aspergillus niger produces molecules such as gluconolactone and hydrogen peroxide, which can reduce Cr(VI) to Cr(III) (Gutierrez-Corona et al., 2016). Sharma et al. show good purification of Cr from Cr-containing wastewater using a complex of two fungi (Cladosporeum perangustum and Penicillium commune) and two bacteria (Paecilomyces lilacinus and Fusarium equiseti) and using nylon mesh as a support material. The germination rate of seeds in the treated wastewater is close to 50.00% (0 for untreated wastewater under the same conditions) (Sharma and Malaviya, 2016).
In general, the remediation of Cr contamination using microorganisms, such as bacteria and fungi, is an economical and environment-friendly solution, but the application is slow and limited by the contamination concentration and environmental factors (pH, nutrients used for bacterial growth, etc.).
4.3.2 Phytoremediation
The advantages of phytoremediation are in situ remediation, no secondary pollution, low cost, low soil disturbance, and the ability to combine remediation effects with economic effects, making it suitable for treating long-term contaminated areas. However, the efficiency of the action depends on the growth rate of plants and generally has a long cycle time.
Plants can take up soluble metal ions from water and soil during growth, especially during photosynthesis. In addition to the uptake of metal ions, plants can detoxify metals into less harmful forms by chelating heavy metals or changing their oxidation state. Ranieri and Gikas constructed a model of Cr(VI)-contaminated soil (irrigated with 10.00 mg L-1 of Cr(VI) water) and studied the effectiveness of three plants (reed, willow, and stinkhorn) growing in Cr(VI)-contaminated soil to remove Cr(VI) from the soil. Results showed that the total removal of Cr(VI) from water ranges from 56.00% (reed) to 70.00% (willow) (Ranieri et al., 2016). Both macrophytes (Pistia stratiotes and Eichhornia crassipes) show high removal efficiency of Cr from different metal solutions without affecting growth and dry biomass. The mean accumulation amounts of Cr in the roots and leaves of P. stratiotes are 85.00 mg and 56.00 mg, respectively, whereas those in the roots and leaves of E. crassipes are 90.00 mg and 53.00 mg, respectively (Tabinda et al., 2020). Brassica napus can effectively remove 98.00% of 10.00 mg L-1 Cr(VI), and the main mechanisms involved are accumulation (55.00%–60.00%) and adsorption (30.00%) (Perotti et al., 2020). Microalgae can be used as bio-sorbents to remove heavy metals from water, and their mechanisms of action are rapid biosorption and slow bioaccumulation (Leong and Chang, 2020). The microalgae species Chlorella sorokiniana can remove 99.67% of Cr from a 100 ppm Cr(VI) containing effluent in 3 days (Hamouda et al., 2019). The principle of action is that microalgae have high antioxidant activity and can synthesize various antioxidant enzymes for counteracting the oxidative effect of Cr(VI) (Balaji et al., 2016).
4.3.3 Plant-based microbial fuel cell
Plant-based microbial fuel cell (PMFC) is a renewable and sustainable energy technology that generates energy from wastewater by combining a microbial-catalyzed reduction reaction with a biological cathode. PMFC systems can improve the removal of Cr(VI) through bioelectrochemical reduction. It provides a new idea for the removal of Cr(VI) from wastewater or soil (Zhang et al., 2021b). Wetland plants with PMFCs can remediate Cr(VI)-contaminated soils, with plants and electrode materials being the two larger influencing factors. PMFC systems can achieve 99.00% removal of Cr(VI) from soil through different mechanisms, including soil background reduction, plant uptake, and bioelectrochemical processes. Among these mechanisms, bioelectrochemical processes contribute the most to Cr(VI) removal. The use of higher biomass plants (Chinese bromeliads) PMFC is more effective than the use of common ones (Guan et al., 2019). Plants can provide a carbon source for microbial fuel cells by secreting root exudates, and bioelectrochemical reduction is the main mechanism for the removal of Cr(VI). The generation of current is related to the initial Cr(VI) concentration, and a high Cr(VI) concentration leads to a higher current. However, due to the high toxicity of Cr(VI), excessively high Cr(VI) concentrations can affect plant growth and bacterial activity. Therefore, Cr-tolerant strains and plants need to be identified (Habibul et al., 2016). Cr(VI) gradually decreases when strain Lsc-8 isolated from rumen contents is inoculated into growth medium containing 9.44 mg L-1 Cr(VI), and the Cr(VI) concentration decreases from 9.44 mg L-1 to 1.67 ± 1.05 mg L-1 within 24 h. This strain can also be used to produce microorganisms, using carboxymethylcellulose as a carbon source fuel cell with the highest output power density of 3.47 ± 0.28 mWm2 (Cao et al., 2020). Dual-chamber MFCs with air cathodes have been applied to remediate soils contaminated with heavy metals. The metal ions migrate toward the cathode driven by the electrode reaction and the electric field, creating a concentration gradient. Remediation performance can also be improved by lowering the pH and extending the operating time (Wang et al., 2020b). Iron-containing activated carbon particles have been synthesized and incorporated into soil to construct an enhanced microbial fuel cell system, which effectively reduces the resistance of soil and improves the power generation efficiency and remediation efficiency of Cr(VI)-contaminated soil (Wang et al., 2022b).
Microbial fuel cell technology has the advantage of simultaneously remediating Cr(VI) pollutants and generating renewable energy. However, the remediation efficiency of this technology can vary greatly under different hydrogeological conditions, and the relevant conditions (pH, electrode distance, strain selection, etc.) need to be clarified in concrete practice according to actual situations.
In summary, we have discussed the more common and effective remediation methods in physical, chemical and bioremediation, and there are some methods not mentioned in our study, which may be due to the fact that the method is too inefficient or requires a lot of human and financial resources and is not economical. Of course, we do not rule out the possibility that there may be more effective restoration methods that we have not yet discovered. However, we believe that by combining these restoration methods, a more universal restoration method can be developed in the near future.
4.4 Detoxifying substances
In addition to finding methods to remove Cr from contaminated environments, substances that can be used to mitigate Cr toxicity should also be determined. Low amounts of selenium form complexes with Cr(VI) and inhibit the accumulation of Cr(VI) in organs (Chen et al., 2017). Hydrogen-rich medium can reduce Cr(VI) damage via the ER stress-COX-2 pathway (Liu et al., 2022). Tomato anthocyanin ameliorates Cr(VI)-induced autophagy by inhibiting ER stress (Liu et al., 2020). Lycium ruthenicum Murr anthocyanin can inhibit autophagic activity and reduce mitochondrial damage through downregulation of the PINK1/Parkin pathway, resulting in a protective effect on DF-1 cells (Guo et al., 2022), Platycodon grandiflorus polysaccharides could improve Cr(VI)-induced mitochondrial autophagy by inhibiting ROS and restoring MMP (Hao et al., 2020). These studies could serve as bases for the treatment of Cr poisoning.
5 Conclusion
Cr(VI) is a widely used heavy metal and its pollution of the environment has been a worldwide concern. Cr(VI) has multiple toxicities (cumulative, ecotoxic and genotoxic) to plants, animals and the environment. Cr(VI) can cause ER stress, autophagy, apoptosis and inflammation, but there are many unclear questions about the mechanisms of its toxic effects, such as the interrelationship between ER stress, autophagy, apoptosis and inflammation. So the next step of research we should carry out around these issues. In addition, researchers have used various techniques (including physical, chemical and biological methods) for the purification of Cr(VI) from the environment, but each purification method has its own advantages and disadvantages. Therefore, a feasible research direction is to combine multiple decontamination methods to develop an efficient, reproducible and cost-effective solution that can be used to treat different forms of Cr contamination.
Author contributions
Conceptualization, PZ, YL, and JL; data curation, RZ and XT; formal analysis, GY; funding acquisition, YL; investigation, PZ; methodology, GY and YG; project administration, JL, YL, and PZ; resources, YQ and YF; software, XT; supervision, JL, YL, and PZ; validation, JW and YL; visualization, GY; writing—original draft, GY and YG; writing—review and editing, GY and JL.
Funding
This project was supported by the National Nature Science Foundation of shandong (No. ZR2021MH089), the Shandong Province Medical and Health Technology Development Plan (202003010792).
Conflict of interest
Author JW was employed by company Shandong Zhongnong Puning Pharmaceutical Co.Ltd.
The remaining authors declare that the research was conducted in the absence of any commercial or financial relationships that could be construed as a potential conflict of interest.
Publisher’s note
All claims expressed in this article are solely those of the authors and do not necessarily represent those of their affiliated organizations, or those of the publisher, the editors and the reviewers. Any product that may be evaluated in this article, or claim that may be made by its manufacturer, is not guaranteed or endorsed by the publisher.
References
Ahmed, M. K., Kundu, G. K., Al-Mamun, M. H., Sarkar, S. K., Akter, M. S., and Khan, M. S. (2013). Chromium (vi) induced acute toxicity and genotoxicity in freshwater stinging catfish, heteropneustes fossilis. Ecotoxicol. Environ. Saf. 92, 64–70. Epub 2013/03/12. doi:10.1016/j.ecoenv.2013.02.008
Ali, S., Bharwana, S. A., Rizwan, M., Farid, M., Kanwal, S., Ali, Q., et al. (2015). Fulvic acid mediates chromium (Cr) tolerance in wheat (Triticum aestivum L.) through lowering of Cr uptake and improved antioxidant defense system. Environ. Sci. Pollut. Res. Int. 22 (14), 10601–10609. Epub 2015/03/07. doi:10.1007/s11356-015-4271-7
Alvarez, C. C., Bravo Gomez, M. E., and Hernandez Zavala, A. (2021). Hexavalent chromium: Regulation and health effects. J. Trace Elem. Med. Biol. 65, 126729. Epub 2021/02/21. doi:10.1016/j.jtemb.2021.126729
Anjum, S. A., Ashraf, U., Khan, I., Tanveer, M., Shahid, M., Shakoor, A., et al. (2017). Phyto-toxicity of chromium in maize: Oxidative damage, osmolyte accumulation, anti-oxidative defense and chromium uptake. Pedosphere 27 (2), 262–273. doi:10.1016/s1002-0160(17)60315-1
Ao, M., Chen, X., Deng, T., Sun, S., Tang, Y., Morel, J. L., et al. (2022). Chromium biogeochemical behaviour in soil-plant systems and remediation strategies: A critical review. J. Hazard Mater 424, 127233. Epub 2021/10/01. doi:10.1016/j.jhazmat.2021.127233
Ayangbenro, A. S., and Babalola, O. O. (2017). A new Strategy for heavy metal polluted environments: A review of microbial biosorbents. Int. J. Environ. Res. Public Health 14 (1), 94. Epub 2017/01/21. doi:10.3390/ijerph14010094
Balaji, S., Kalaivani, T., Sushma, B., Pillai, C. V., Shalini, M., and Rajasekaran, C. (2016). Characterization of sorption sites and differential stress response of microalgae isolates against tannery effluents from ranipet industrial area-an application towards phycoremediation. Int. J. Phytoremediation 18 (8), 747–753. Epub 2015/11/21. doi:10.1080/15226514.2015.1115960
Beaumont, J. J., Sedman, R. M., Reynolds, S. D., Sherman, C. D., Li, L. H., Howd, R. A., et al. (2008). Cancer mortality in a Chinese population exposed to hexavalent chromium in drinking water. Epidemiology 19 (1), 12–23. Epub 2007/12/20. doi:10.1097/EDE.0b013e31815cea4c
Butter, B., Santander, P., Pizarro, G. D. C., Oyarzun, D. P., Tasca, F., and Sanchez, J. (2021). Electrochemical reduction of Cr(vi) in the presence of sodium alginate and its application in water purification. J. Environ. Sci. (China) 101, 304–312. Epub 2020/12/19. doi:10.1016/j.jes.2020.08.033
Cao, L., Ma, Y., Deng, D., Jiang, H., Wang, J., and Liu, Y. (2020). Electricity production of microbial fuel cells by degrading cellulose coupling with Cr(vi) removal. J. Hazard Mater 391, 122184. Epub 2020/02/25. doi:10.1016/j.jhazmat.2020.122184
Chen, M., Huang, X., Shi, H., Lai, J., Ma, L., Lau, T. C., et al. (2021). Cr(V)-Cr(Iii) in-situ transition promotes ros generation to achieve efficient cancer therapy. Biomaterials 276, 120991. Epub 2021/07/09. doi:10.1016/j.biomaterials.2021.120991
Chen, P., Geng, N., Zhou, D., Zhu, Y., Xu, Y., Liu, K., et al. (2019). The regulatory role of cox-2 in the interaction between Cr(Vi)-Induced endoplasmic reticulum stress and autophagy in df-1 cells. Ecotoxicol. Environ. Saf. 170, 112–119. Epub 2018/12/12. doi:10.1016/j.ecoenv.2018.11.120
Chen, P., Zhu, Y., Wan, H., Wang, Y., Hao, P., Cheng, Z., et al. (2017). Effects of the oral administration of K(2)Cr(2)O(7) and Na(2)Seo(3) on Ca, Mg, Mn, Fe, Cu, and Zn contents in the heart, liver, spleen, and kidney of chickens. Biol. Trace Elem. Res. 180 (2), 285–296. Epub 2017/03/30. doi:10.1007/s12011-017-0999-x
Chen, Z., and Pan, K. (2021). Enhanced removal of Cr(vi) via in-situ synergistic reduction and fixation by polypyrrole/sugarcane bagasse composites. Chemosphere 272, 129606. Epub 2021/01/20. doi:10.1016/j.chemosphere.2021.129606
Cheng, J., Fan, W., Zhao, X., Liu, Y., Cheng, Z., Liu, Y., et al. (2016). Oxidative stress and histological alterations of chicken brain induced by oral administration of chromium(iii). Biol. Trace Elem. Res. 173 (1), 185–193. Epub 2016/02/14. doi:10.1007/s12011-016-0640-4
Cho, H. K., Cheong, K. J., Kim, H. Y., and Cheong, J. (2011). Endoplasmic reticulum stress induced by hepatitis B virus X protein enhances cyclo-oxygenase 2 expression via activating transcription factor 4. Biochem. J. 435 (2), 431–439. Epub 2011/01/20. doi:10.1042/BJ20102071
Deng, Y., Wang, M., Tian, T., Lin, S., Xu, P., Zhou, L., et al. (2019). The effect of hexavalent chromium on the incidence and mortality of human cancers: A meta-analysis based on published epidemiological cohort studies. Front. Oncol. 9, 24. Epub 2019/02/20. doi:10.3389/fonc.2019.00024
El-Mehalmey, W. A., Ibrahim, A. H., Abugable, A. A., Hassan, M. H., Haikal, R. R., Karakalos, S. G., et al. (2018). Metal–organic Framework@Silica as a stationary phase sorbent for rapid and cost-effective removal of hexavalent chromium. J. Mater. Chem. A 6 (6), 2742–2751. doi:10.1039/c7ta08281a
Fan, W. T., Zhao, X. N., Cheng, J., Liu, Y. H., and Liu, J. Z. (2015). Oxidative stress and hepatocellular injury induced by oral administration of Cr(3+) in chicken. J. Biochem. Mol. Toxicol. 29 (6), 280–287. Epub 2015/03/25. doi:10.1002/jbt.21697
Farooq, M. A., Ali, S., Hameed, A., Bharwana, S. A., Rizwan, M., Ishaque, W., et al. (2016). Cadmium stress in cotton seedlings: Physiological, photosynthesis and oxidative damages alleviated by glycinebetaine. South Afr. J. Bot. 104, 61–68. doi:10.1016/j.sajb.2015.11.006
Gao, Z., Dlamini, M. B., Ge, H., Jiang, L., Geng, C., Li, Q., et al. (2020). Atf4-Mediated autophagy-dependent glycolysis plays an important role in attenuating apoptosis induced by Cr (vi) in A549 cells. Toxicol. Lett. 331, 178–187. Epub 2020/06/23. doi:10.1016/j.toxlet.2020.06.015
Ge, H., Li, Z., Jiang, L., Li, Q., Geng, C., Yao, X., et al. (2019). Cr (vi) induces crosstalk between apoptosis and autophagy through endoplasmic reticulum stress in A549 cells. Chem. Biol. Interact. 298, 35–42. Epub 2018/11/13. doi:10.1016/j.cbi.2018.10.024
Ge, S., Ai, W., and Dong, X. (2016). High-quality draft genome sequence of leucobacter sp. Strain G161, a distinct and effective chromium reducer. Genome announc. 4 (1), 017600-e1815. Epub 2016/02/20. doi:10.1128/genomeA.01760-15
Guan, C. Y., Tseng, Y. H., Tsang, D. C. W., Hu, A., and Yu, C. P. (2019). Wetland plant microbial fuel cells for remediation of hexavalent chromium contaminated soils and electricity production. J. Hazard Mater 365, 137–145. Epub 2018/11/13. doi:10.1016/j.jhazmat.2018.10.086
Guo, S., Qi, M., Li, H., Cui, Y., Qi, C., Cheng, G., et al. (2022). The protective effect of lycium ruthenicum Murr anthocyanins in Cr (Vi)-Induced mitophagy in df-1 cells. Life (Basel) 12 (8), 1115. Epub 2022/07/28. doi:10.3390/life12081115
Guo, S., Wang, X., Wang, L., Cheng, G., Zhang, M., Xing, Y., et al. (2021). Inflammatory injury and mitophagy of the brain in chicken exposed to Cr(vi). Environ. Sci. Pollut. Res. Int. 28 (31), 42353–42361. Epub 2021/04/05. doi:10.1007/s11356-021-13675-2
Gutierrez-Corona, J. F., Romo-Rodriguez, P., Santos-Escobar, F., Espino-Saldana, A. E., and Hernandez-Escoto, H. (2016). Microbial interactions with chromium: Basic biological processes and applications in environmental biotechnology. World J. Microbiol. Biotechnol. 32 (12), 191. Epub 2016/10/09. doi:10.1007/s11274-016-2150-0
Habibul, N., Hu, Y., Wang, Y. K., Chen, W., Yu, H. Q., and Sheng, G. P. (2016). Bioelectrochemical chromium(vi) removal in plant-microbial fuel cells. Environ. Sci. Technol. 50 (7), 3882–3889. Epub 2016/03/11. doi:10.1021/acs.est.5b06376
Hamouda, A. S., Mahmoud, H. M., El-Belely, E. F., Labena, A., and Husien, S. (2019). Absorption of hexavalent chromium by green micro algae Chlorella sorokiniana: Live planktonic cells. Water Pract. Technol. 14 (3), 515–529. doi:10.2166/wpt.2019.034
Hao, J., Song, Y., Tian, B., Qi, C., Li, L., Wang, L., et al. (2020). Platycodon grandifloras polysaccharides inhibit mitophagy injury induced by Cr (vi) in df-1 cells. Ecotoxicol. Environ. Saf. 202, 110901. Epub 2020/07/01. doi:10.1016/j.ecoenv.2020.110901
Hao, P., Zhu, Y., Wang, S., Wan, H., Chen, P., Wang, Y., et al. (2017). Selenium administration alleviates toxicity of chromium(vi) in the chicken brain. Biol. Trace Elem. Res. 178 (1), 127–135. Epub 2016/12/26. doi:10.1007/s12011-016-0915-9
Jomova, K., and Valko, M. (2011). Advances in metal-induced oxidative stress and human disease. Toxicology 283 (2-3), 65–87. Epub 2011/03/19. doi:10.1016/j.tox.2011.03.001
Kahu, S. S., Shekhawat, A., Saravanan, D., and Jugade, R. M. (2016). Two Fold modified chitosan for enhanced adsorption of hexavalent chromium from simulated wastewater and industrial effluents. Carbohydr. Polym. 146, 264–273. Epub 2016/04/27. doi:10.1016/j.carbpol.2016.03.041
Katz, S. A., and Salem, H. (1993). The toxicology of chromium with respect to its chemical speciation: A review. J. Appl. Toxicol. 13 (3), 217–224. Epub 1993/05/01. doi:10.1002/jat.2550130314
Kushwaha, B. K., and Singh, V. P. (2020). Glutathione and hydrogen sulfide are required for sulfur-mediated mitigation of Cr(vi) toxicity in tomato, pea and brinjal seedlings. Physiol. Plant 168 (2), 406–421. Epub 2019/09/11. doi:10.1111/ppl.13024
Lee, Y. H., Su, S. B., Huang, C. C., Sheu, H. M., Tsai, J. C., Lin, C. H., et al. (2014). N-acetylcysteine attenuates hexavalent chromium-induced hypersensitivity through inhibition of cell death, ros-related signaling and cytokine expression. PLoS One 9 (9), e108317. Epub 2014/09/24. doi:10.1371/journal.pone.0108317
Leong, Y. K., and Chang, J. S. (2020). Bioremediation of heavy metals using microalgae: Recent advances and mechanisms. Bioresour. Technol. 303, 122886. Epub 2020/02/13. doi:10.1016/j.biortech.2020.122886
Li, S. Y., Teng, H. J., Guo, J. Z., Wang, Y. X., and Li, B. (2021). Enhanced removal of Cr(vi) by nitrogen-doped hydrochar prepared from bamboo and ammonium chloride. Bioresour. Technol. 342, 126028. Epub 2021/09/29. doi:10.1016/j.biortech.2021.126028
Li, W., Yang, S., Wang, W., Liu, Q., He, J., Li, B., et al. (2020). Simultaneous removal of Cr(vi) and acid orange 7 from water in pyrite-persulfate system. Environ. Res. 189, 109876. Epub 2020/07/18. doi:10.1016/j.envres.2020.109876
Liang, Q., Xiao, Y., Liu, K., Zhong, C., Zeng, M., and Xiao, F. (2018). Cr(Vi)-Induced autophagy protects L-02 hepatocytes from apoptosis through the ros-akt-mtor pathway. Cell Physiol. Biochem. 51 (4), 1863–1878. Epub 2018/12/07. doi:10.1159/000495713
Liang, Q., Zhang, Y., Huang, M., Xiao, Y., and Xiao, F. (2019). Role of mitochondrial damage in Cr(Vi)-Induced endoplasmic reticulum stress in L-02 hepatocytes. Mol. Med. Rep. 19 (2), 1256–1265. Epub 2018/12/12. doi:10.3892/mmr.2018.9704
Liu, B., Su, G., Yang, Y., Yao, Y., Huang, Y., Hu, L., et al. (2019). Vertical distribution of microbial communities in chromium-contaminated soil and isolation of Cr(Ⅵ)-Reducing strains. Ecotoxicol. Environ. Saf. 180, 242–251. Epub 2019/05/18. doi:10.1016/j.ecoenv.2019.05.023
Liu, K., Chen, P., Lu, J., Zhu, Y., Xu, Y., Liu, Y., et al. (2020). Protective effect of purple tomato anthocyanidin on chromium(vi)-induced autophagy in lmh cells by inhibiting endoplasmic reticulum stress. Biol. Trace Elem. Res. 194 (2), 570–580. Epub 2019/07/03. doi:10.1007/s12011-019-01795-3
Liu, K., Cui, Y., Li, H., Qi, C., Cheng, G., Gao, X., et al. (2022). Hydrogen-rich medium regulates Cr(Vi)-Induced Er stress and autophagy signaling in df-1 cells. Biol. Trace Elem. Res. 200 (5), 2329–2337. Epub 2021/07/31. doi:10.1007/s12011-021-02850-8
Liu, Y., Hao, P., Zhang, X., Zhao, X., Liu, Y., and Liu, J. (2017). Effects of excess Cr(3+) on trace element contents in the brain and serum in chicken. Biol. Trace Elem. Res. 177 (1), 180–186. Epub 2016/10/21. doi:10.1007/s12011-016-0875-0
Liu, Y., Liu, C., Cheng, J., Fan, W., Zhang, X., and Liu, J. (2015). Growth performance and oxidative damage in kidney induced by oral administration of Cr(iii) in chicken. Chemosphere 139, 365–371. Epub 2015/07/25. doi:10.1016/j.chemosphere.2015.07.032
Liu, Y., Zhao, X., Zhang, X., Zhao, X., Liu, Y., and Liu, J. (2016). Effects of oral administration of Crcl3 on the contents of Ca, Mg, Mn, Fe, Cu, and Zn in the liver, kidney, and heart of chicken. Biol. Trace Elem. Res. 171 (2), 459–467. Epub 2015/11/06. doi:10.1007/s12011-015-0559-1
Lu, J., Liu, K., Qi, M., Geng, H., Hao, J., Wang, R., et al. (2019). Effects of Cr(vi) exposure on electrocardiogram, myocardial enzyme parameters, inflammatory factors, oxidative kinase, and atpase of the heart in Chinese rural dogs. Environ. Sci. Pollut. Res. Int. 26 (29), 30444–30451. Epub 2019/08/24. doi:10.1007/s11356-019-06253-0
Mahmoud, M. E., Amira, M. F., Azab, M., and Abdelfattah, A. M. (2021). Effective removal of levofloxacin drug and Cr(vi) from water by a composed nanobiosorbent of vanadium Pentoxide@Chitosan@Mofs. Int. J. Biol. Macromol. 188, 879–891. Epub 2021/08/18. doi:10.1016/j.ijbiomac.2021.08.092
Mezencev, R., and Auerbach, S. S. (2021). Inferred inactivation of the cftr gene in the duodena of mice exposed to hexavalent chromium (Cr(vi)) in drinking water supports its tumor-suppressor status and implies its potential role in Cr(Vi)-Induced carcinogenesis of the small intestines. Toxicol. Appl. Pharmacol. 433, 115773. Epub 2021/10/25. doi:10.1016/j.taap.2021.115773
Monga, A., Fulke, A. B., and Dasgupta, D. (2022). Recent developments in essentiality of trivalent chromium and toxicity of hexavalent chromium: Implications on human health and remediation strategies. J. Hazard. Mater. Adv. 7, 100113. doi:10.1016/j.hazadv.2022.100113
Monga, A., Fulke, A. B., Gaud, A., Sharma, A., Ram, A., and Dasgupta, D. (2022). Isolation and identification of novel chromium tolerant bacterial strains from a heavy metal polluted urban creek: An assessment of bioremediation efficiency and flocculant production. Thalassas Int. J. Mar. Sci. 38 (2), 1233–1244. doi:10.1007/s41208-022-00458-w
Perotti, R., Paisio, C. E., Agostini, E., Fernandez, M. I., and Gonzalez, P. S. (2020). Cr(Vi) phytoremediation by hairy roots of Brassica napus: Assessing efficiency, mechanisms involved, and post-removal toxicity. Environ. Sci. Pollut. Res. Int. 27 (9), 9465–9474. Epub 2020/01/11. doi:10.1007/s11356-019-07258-5
Rahman, M. S., Molla, A. H., Saha, N., and Rahman, A. (2012). Study on heavy metals levels and its risk assessment in some edible fishes from bangshi river, savar, dhaka, Bangladesh. Food Chem. 134 (4), 1847–1854. Epub 2013/02/28. doi:10.1016/j.foodchem.2012.03.099
Ranieri, E., Fratino, U., Petrella, A., Torretta, V., and Rada, E. C. (2016). Ailanthus altissima and phragmites australis for chromium removal from a contaminated soil. Environ. Sci. Pollut. Res. Int. 23 (16), 15983–15989. Epub 2016/05/06. doi:10.1007/s11356-016-6804-0
Roestorff, M. M., and Chirwa, E. M. N. (2019). Cr(Vi) mediated hydrolysis of algae cell walls to release toc for enhanced biotransformation of Cr(vi) by a culture of Cr(vi) reducing bacteria. J. Appl. Phycol. 31 (6), 3637–3649. doi:10.1007/s10811-018-1716-7
Roy, R. V., Pratheeshkumar, P., Son, Y. O., Wang, L., Hitron, J. A., Divya, S. P., et al. (2016). Different roles of ros and Nrf2 in Cr(Vi)-Induced inflammatory responses in normal and Cr(Vi)-Transformed cells. Toxicol. Appl. Pharmacol. 307, 81–90. Epub 2016/07/30. doi:10.1016/j.taap.2016.07.016
Sagar, S., Dwivedi, A., Yadav, S., Tripathi, M., and Kaistha, S. D. (2012). Hexavalent chromium reduction and plant growth promotion by staphylococcusarlettae strain Cr11. Chemosphere 86 (8), 847–852. Epub 2011/12/16. doi:10.1016/j.chemosphere.2011.11.031
Sathvika, T., Soni, A., Sharma, K., Praneeth, M., Mudaliyar, M., Rajesh, V., et al. (2018). Potential application of Saccharomyces cerevisiae and rhizobium immobilized in multi walled carbon nanotubes to adsorb hexavalent chromium. Sci. Rep. 8 (1), 9862. Epub 2018/07/01. doi:10.1038/s41598-018-28067-9
Scharf, B., Clement, C. C., Zolla, V., Perino, G., Yan, B., Elci, S. G., et al. (2014). Molecular analysis of chromium and cobalt-related toxicity. Sci. Rep. 4, 5729. Epub 2014/07/19. doi:10.1038/srep05729
Seleiman, M. F., Ali, S., Refay, Y., Rizwan, M., Alhammad, B. A., and El-Hendawy, S. E. (2020). Chromium resistant microbes and melatonin reduced Cr uptake and toxicity, improved physio-biochemical traits and yield of wheat in contaminated soil. Chemosphere 250, 126239. Epub 2020/02/24. doi:10.1016/j.chemosphere.2020.126239
Sharma, A., Kapoor, D., Wang, J., Shahzad, B., Kumar, V., Bali, A. S., et al. (2020). Chromium bioaccumulation and its impacts on plants: An overview. Plants (Basel) 9 (1), 100. Epub 2020/01/17. doi:10.3390/plants9010100
Sharma, S., and Malaviya, P. (2016). Bioremediation of tannery wastewater by chromium resistant novel fungal consortium. Ecol. Eng. 91, 419–425. doi:10.1016/j.ecoleng.2016.03.005
Sheik, C. S., Mitchell, T. W., Rizvi, F. Z., Rehman, Y., Faisal, M., Hasnain, S., et al. (2012). Exposure of soil microbial communities to chromium and arsenic alters their diversity and structure. PLoS One 7 (6), e40059. Epub 2012/07/07. doi:10.1371/journal.pone.0040059
Shi, X., Gong, B., Liao, S., Wang, J., Liu, Y., Wang, T., et al. (2020). Removal and enrichment of Cr(vi) from aqueous solutions by Lotus seed pods. Water Environ. Res. 92 (1), 84–93. Epub 2019/07/25. doi:10.1002/wer.1187
Su, Y. Q., Yuan, S., Guo, Y. C., Tan, Y. Y., Mao, H. T., Cao, Y., et al. (2021). Highly efficient and sustainable removal of Cr (vi) in aqueous solutions by photosynthetic bacteria supplemented with phosphor salts. Chemosphere 283, 131031. Epub 2021/06/17. doi:10.1016/j.chemosphere.2021.131031
Tabinda, A. B., Irfan, R., Yasar, A., Iqbal, A., and Mahmood, A. (2020). Phytoremediation potential of Pistia stratiotes and Eichhornia crassipes to remove chromium and copper. Environ. Technol. 41 (12), 1514–1519. Epub 2018/10/26. doi:10.1080/09593330.2018.1540662
Tripathi, R. M., and Chung, S. J. (2020). Reclamation of hexavalent chromium using catalytic activity of highly recyclable biogenic Pd(0) nanoparticles. Sci. Rep. 10 (1), 640. Epub 2020/01/22. doi:10.1038/s41598-020-57548-z
Ukhurebor, K. E., Aigbe, U. O., Onyancha, R. B., Nwankwo, W., Osibote, O. A., Paumo, H. K., et al. (2021). Effect of hexavalent chromium on the environment and removal techniques: A review. J. Environ. Manage 280, 111809. Epub 2020/12/29. doi:10.1016/j.jenvman.2020.111809
Vincent, J. B., and Lukaski, H. C. (2018). Chromium. Adv. Nutr. 9 (4), 505–506. Epub 2018/07/23. doi:10.1093/advances/nmx021
VonHandorf, A., Zablon, H. A., and Puga, A. (2021). Hexavalent chromium disrupts chromatin architecture. Semin. Cancer Biol. 76, 54–60. Epub 2021/07/19. doi:10.1016/j.semcancer.2021.07.009
Wan, H., Zhu, Y., Chen, P., Wang, Y., Hao, P., Cheng, Z., et al. (2017). Effect of various selenium doses on chromium(iv)-induced nephrotoxicity in a male chicken model. Chemosphere 174, 306–314. Epub 2017/02/10. doi:10.1016/j.chemosphere.2017.01.143
Wang, D., Li, G., Qin, S., Tao, W., Gong, S., and Wang, J. (2021). Remediation of Cr(Vi)-Contaminated soil using combined chemical leaching and reduction techniques based on hexavalent chromium speciation. Ecotoxicol. Environ. Saf. 208, 111734. Epub 2021/01/06. doi:10.1016/j.ecoenv.2020.111734
Wang, H., Liu, J., Gui, C., Yan, Q., Wang, L., Wang, S., et al. (2022). Synergistic remediation of Cr(vi) contaminated soil by iron-loaded activated carbon in two-chamber microbial fuel cells. Environ. Res. 208, 112707. Epub 2022/01/11. doi:10.1016/j.envres.2022.112707
Wang, H., Zhang, H., Zhang, X., Li, Q., Cheng, C., Shen, H., et al. (2020). Bioelectrochemical remediation of Cr(vi)/Cd(Ii)-Contaminated soil in bipolar membrane microbial fuel cells. Environ. Res. 186, 109582. Epub 2020/05/04. doi:10.1016/j.envres.2020.109582
Wang, P., and Chao, D. (2020). Phytoremediation of heavy metal contamination and related molecular mechanisms in plants. Sheng Wu Gong Cheng Xue Bao 36 (3), 426–435. Epub 2020/04/03. doi:10.13345/j.cjb.190332
Wang, Y., Hao, J., Zhang, S., Li, L., Wang, R., Zhu, Y., et al. (2020). Inflammatory injury and mitophagy induced by Cr(vi) in chicken liver. Environ. Sci. Pollut. Res. Int. 27 (18), 22980–22988. Epub 2020/04/25. doi:10.1007/s11356-020-08544-3
Wang, Y., Wang, L., Wang, X., Cheng, G., Xing, Y., Zhang, M., et al. (2022). Inflammatory injury and mitophagy in the cock heart induced by the oral administration of hexavalent chromium. Biol. Trace Elem. Res. 200 (3), 1312–1320. Epub 2021/04/15. doi:10.1007/s12011-021-02715-0
Wang, Y., Wang, X., Wang, L., Cheng, G., Zhang, M., Xing, Y., et al. (2021). Mitophagy induced by mitochondrial function damage in chicken kidney exposed to Cr(vi). Biol. Trace Elem. Res. 199 (2), 703–711. Epub 2020/05/23. doi:10.1007/s12011-020-02176-x
Whitaker, A. H., Pena, J., Amor, M., and Duckworth, O. W. (2018). Cr(Vi) uptake and reduction by biogenic iron (Oxyhydr)Oxides. Environ. Sci. Process Impacts 20 (7), 1056–1068. Epub 2018/06/21. doi:10.1039/c8em00149a
Wise, J. P., Young, J. L., Cai, J., and Cai, L. (2022). Current understanding of hexavalent chromium [Cr(vi)] neurotoxicity and new perspectives. Environ. Int. 158, 106877. Epub 2021/09/22. doi:10.1016/j.envint.2021.106877
Xu, H., Gao, M., Hu, X., Chen, Y., Li, Y., Xu, X., et al. (2021). A novel preparation of S-nzvi and its high efficient removal of Cr(vi) in aqueous solution. J. Hazard Mater 416, 125924. Epub 2021/09/09. doi:10.1016/j.jhazmat.2021.125924
Xu, X., Nie, S., Ding, H., and Hou, F. F. (2018). Environmental pollution and kidney diseases. Nat. Rev. Nephrol. 14 (5), 313–324. Epub 2018/02/27. doi:10.1038/nrneph.2018.11
Xu, Y., Wang, X., Geng, N., Zhu, Y., Zhang, S., Liu, Y., et al. (2020). Mitophagy is involved in chromium (Vi)-Induced mitochondria damage in df-1 cells. Ecotoxicol. Environ. Saf. 194, 110414. Epub 2020/03/11. doi:10.1016/j.ecoenv.2020.110414
Yang, F., Zhao, L., Mei, D., Jiang, L., Geng, C., Li, Q., et al. (2017). Hmga2 plays an important role in Cr (Vi)-Induced autophagy. Int. J. Cancer 141 (5), 986–997. Epub 2017/05/17. doi:10.1002/ijc.30789
Yang, X., Liu, P., Yao, M., Sun, H., Liu, R., Xie, J., et al. (2021). Mechanism and enhancement of Cr(vi) contaminated groundwater remediation by molasses. Sci. Total Environ. 780, 146580. Epub 2021/05/26. doi:10.1016/j.scitotenv.2021.146580
Zhang, S., Zhao, X., Hao, J., Zhu, Y., Wang, Y., Wang, L., et al. (2021). The role of Atf6 in Cr(Vi)-Induced apoptosis in df-1 cells. J. Hazard Mater 410, 124607. Epub 2020/11/28. doi:10.1016/j.jhazmat.2020.124607
Zhang, X., Liu, Y., and Li, C. (2021). Influence of Cr (vi) concentration on Cr (vi) reduction and electricity production in microbial fuel cell. Environ. Sci. Pollut. Res. Int. 28 (38), 54170–54176. Epub 2021/08/19. doi:10.1007/s11356-021-15889-w
Zhang, X., Zhang, X., Li, L., Fu, G., Liu, X., Xing, S., et al. (2022). The toxicity of hexavalent chromium to soil microbial processes concerning soil properties and aging time. Environ. Res. 204, 111941. Epub 2021/09/03. doi:10.1016/j.envres.2021.111941
Zhitkovich, A. (2011). Chromium in drinking water: Sources, metabolism, and cancer risks. Chem. Res. Toxicol. 24 (10), 1617–1629. Epub 2011/07/20. doi:10.1021/tx200251t
Zhu, Y., Chen, P., Wan, H., Wang, Y., Hao, P., Liu, Y., et al. (2018). Selenium-Chromium(Vi) interaction regulates the contents and correlations of trace elements in chicken brain and serum. Biol. Trace Elem. Res. 181 (1), 154–163. Epub 2017/05/12. doi:10.1007/s12011-017-1038-7
Zhu, Y., He, X., Xu, J., Fu, Z., Wu, S., Ni, J., et al. (2021). Insight into efficient removal of Cr(vi) by magnetite immobilized with Lysinibacillus sp. Jlt12: Mechanism and performance. Chemosphere 262, 127901. Epub 2020/08/18. doi:10.1016/j.chemosphere.2020.127901
Zhu, Y., Wang, L., Yu, X., Jiang, S., Wang, X., Xing, Y., et al. (2021). Cr(Vi) promotes tight joint and oxidative damage by activating the nrf2/ros/notch1 Axis. Environ. Toxicol. Pharmacol. 85, 103640. Epub 2021/03/25. doi:10.1016/j.etap.2021.103640
Keywords: chromium, carcinogenic risk, environmental health hazards, cytotoxicity, heavy metal, remediation strategy
Citation: Yan G, Gao Y, Xue K, Qi Y, Fan Y, Tian X, Wang J, Zhao R, Zhang P, Liu Y and Liu J (2023) Toxicity mechanisms and remediation strategies for chromium exposure in the environment. Front. Environ. Sci. 11:1131204. doi: 10.3389/fenvs.2023.1131204
Received: 24 December 2022; Accepted: 30 January 2023;
Published: 07 February 2023.
Edited by:
Huabin Cao, Jiangxi Agricultural University, ChinaReviewed by:
Limei Zhang, China Agricultural University, ChinaWenjing Sun, Yulin Normal University, China
Copyright © 2023 Yan, Gao, Xue, Qi, Fan, Tian, Wang, Zhao, Zhang, Liu and Liu. This is an open-access article distributed under the terms of the Creative Commons Attribution License (CC BY). The use, distribution or reproduction in other forums is permitted, provided the original author(s) and the copyright owner(s) are credited and that the original publication in this journal is cited, in accordance with accepted academic practice. No use, distribution or reproduction is permitted which does not comply with these terms.
*Correspondence: Jianzhu Liu, bGl1anpAc2RhdS5lZHUuY24=; Pu Zhang, enA4MTk4NDIzQDE2My5jb20=
†These authors have contributed equally to this work