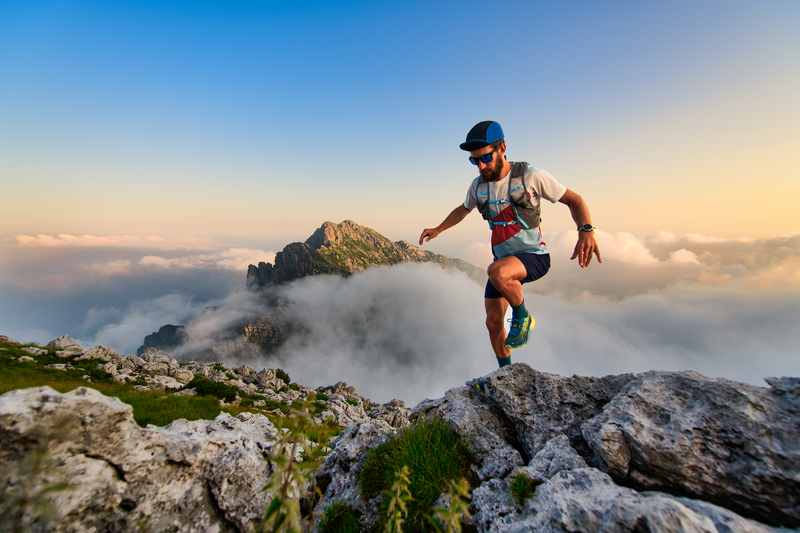
95% of researchers rate our articles as excellent or good
Learn more about the work of our research integrity team to safeguard the quality of each article we publish.
Find out more
ORIGINAL RESEARCH article
Front. Environ. Sci. , 28 July 2023
Sec. Soil Processes
Volume 11 - 2023 | https://doi.org/10.3389/fenvs.2023.1127920
Poor phosphorus utilization is a common limitation of sustainable cotton production. Even so, the intrinsic potential for efficient phosphorus acquisition of the roots remains unclear. In this study, we aimed to explore if phosphorus acquisition in cotton is affected by interactions that could possibly be associated with root morphology and gene expression, between genotype and application rates. A pot experiment was conducted with seven phosphorus application rates: 0, 12.5, 25, 50, 75, 150, and 300 mg P kg−1. The agronomic characters, root morphology, and expression of the PHT1 gene in the roots of cotton varieties with different phosphorus acquisition efficiencies were measured. According to the results, the phosphorus acquisition efficiency in cultivar XLZ19 was higher than in XLZ13 for phosphorus uptake because of the upregulation of GhPTs gene expression that increased the total root length and proportion of fine roots. The Olsen-P levels in the soil ranged from 20 to 30 mg kg−1 at the seedling stage and from 15 to 25 mg kg−1 at the flowering stage, suggesting better root growth and phosphorus supply. The conversion of phosphorus fertilizers to soil Olsen-P decreased when the phosphorus application rate exceeded 50–75 mg kg−1. The phosphorus acquisition–efficient XLZ19 phenotype owes its efficiency to high GhPTs expression and morphological changes in the roots. Finally, with these crops that are highly efficient in phosphorus acquisition, agricultural practices in sustainable cotton production may consume less phosphorus fertilizers.
Satisfactory phosphorus management is mandatory for sustainable agriculture. On the one hand, crops utilize only 15%–30% of applied phosphorus (P) fertilizers because they tend to be fixed in the soil and are difficult for direct use by the crop (Andersson et al., 2013; Lambers, 2022). On the other hand, excessive application of phosphate fertilizers can lead to phosphorus accumulation and eutrophication of water bodies (Sharpley et al., 1994; Conley et al., 2009). In intensive cropping systems, the enhancement of phosphorus efficiency has long been a key challenge (Shenoy and Kalagudi, 2005), and current P management strategies in intensive agriculture focus on both agronomic and environmental concerns, aiming to maintain the soil phosphorus level within the critical values that maximize crop yield and minimize phosphorus loss (Li et al., 2011). Still, limited knowledge is available on whether P management could be improved by the selection of cotton cultivars without compromising yield and quality.
As most of the phosphorus from fertilizers remain in the soil, it has been estimated that residual soil phosphorus from previous intensive fertilization may contribute significantly to future crop production with a considerable time lag (Sattari et al., 2012). Thus, it will be very helpful in crop production to boost the plant capacity of using such residual phosphorus. Specifically, crops have evolved a series of morphological, physiological, and biochemical strategies to improve phosphorus acquisition and utilization (FENG et al., 2019), such as higher root/shoot ratio, root elongation, greater specific root length, smaller root diameter, formation of cluster-root (Eshel and Beeckman, 2013), and modification of root architecture (Williamson et al., 2001; Zhao et al., 2004; Li et al., 2011). Many studies have reported significant differences in the morphological and physiological response mechanisms of plants under low P stress both within and between species (Kumawat et al., 2018; Niu et al., 2013; Yang et al., 2020; Zhang et al., 2013). For instance, when the primary root tip of Arabidopsis reaches a low-P zone, the laryngopharyngeal reflux protein in the root cap alters the activity and distribution of hormones, resulting in the inhibition of main root elongation and the promotion of lateral root growth (Svistoonoff et al., 2007; Müller et al., 2015). Plant behaviors under low phosphorus stress vary among species. Nevertheless, most studies exploring the mechanism of phosphorus acquisition were conducted under phosphorus deficiency. Only a limited number of studies have examined different P supply levels and their relationship with crop varieties.
Cotton is an important natural fiber crop throughout the world (Singh et al., 2012) and a staple economic crop in Xinjiang Autonomous Region (XAR), consuming much more phosphorus fertilizers than do cereals (Feng et al., 2017). Low temperatures in spring and salt return to the ground surface in XAR result in low soil phosphorus availability and weak phosphorus absorption and transportation in cotton crops (Peng et al., 2020). Previous studies have found that phosphate fertilizers struggle to penetrate beyond the top 5 cm of the soil, and since the distributions of crop roots and available phosphorus mismatch significantly, phosphorus fertilizers suffer from a low utilization rate (Yin et al., 2005). Therefore, to maximize this utilization rate, the supply intensity and amount of soil available phosphorus should be controlled within an appropriate range to give full play to the root system and mycorrhiza to absorb the phosphorus (Peng et al., 2020).
When phosphorus deficiency occurs, cotton may develop potential strategies (higher root/shoot ratio, root elongation, enhancement of specific root length, and excretion of phosphatase and H+ ions in the rhizosphere) to improve its phosphorus acquisition efficiency (Chen et al., 2019). When the available phosphorus content in the soil is optimal (15–20 mg kg−1), the root length, hyphal density, and apparent phosphorus recovery are all enhanced simultaneously (Mai et al., 2018). On the other hand, when P supply is deficient, the overall plant growth is depressed due to limited photosynthesis and the root growth is not sufficient as when P supply is adequate (Deng et al., 2018). The intrinsic potential of high efficiency in phosphorus acquisition by plant roots has been extensively investigated with the focus on changes in plant agronomic traits and expression of phosphorus transporters. As of today, the root morphology and PHT1 gene expression in different cotton varieties at different periods of phosphorus deficiency, as well as optimal and excessive conditions, remain unclear. PHT1 is a component in the high-affinity phosphate transporter system and participates in plant phosphorus uptake and transport (Cai et al., 2020). PHT1 transporters are membrane proteins of MFS that serve as the primary entry of Pi from the soil to plants (Nussaume et al., 2011). Therefore, the maximum phosphorus utilization efficiency may be studied by combining different cotton varieties with varying phosphorus acquisition efficiencies, and the root morphology and PHT1 gene expression in the phosphorus acquisition–efficient plants may be directly related to the efficiency in phosphorus uptake.
To investigate the relationship between P efficiency, root morphology, and PHT1 gene expression, we conducted pot experiments on two cotton varieties with different phosphorus efficiencies and seven phosphorus application rates to verify our hypothesis that phosphorus acquisition–efficient cotton has a more favorable root morphology and higher expression level of PHT1 gene expression under both low and adequate phosphorus conditions.
A pot experiment to quantify the effects of gray desert soil with seven different phosphorus concentrations (0, 12.5, 25, 50, 75, 150, and 300 mg P kg−1 soil) on phosphorus absorption of cotton and a triplicate randomized block design were conducted. A total of two cultivars, seven treatments, two periods, and three replicates were involved for each treatment, and three plants were allotted for each pot. The volume of each flowerpot was 12,000 cm3 (30 × 20 × 20 cm). The cotton varieties used were cv. Xinluzao 13 and 19 (half of each) cultivated in 2021 and sown from 1 May to 3 May. Following the bud emergence stage, the plants were irrigated once every 8–10 days with about 1–1.5 L of water, resulting in approximately nine irrigation events over the season. Water was kept at 55%–70% of the field capacity, and its amount was increased as the plants grew. The seasonal irrigation ranged from 3 to 4.5 L according to the local farmer’s practice.
Cotton was planted in gray desert soil collected from a cotton field in Changji, XAR (43°79′N, 87°71′E). The initial soil properties were an organic matter of 14.25 g kg−1, an available nitrogen (N) of 18.0 mg kg−1, an Olsen-P of 6.1 mg kg−1, an exchangeable potassium (K) of 359.0 mg kg−1, a pH of 7.63, and a conductivity of 356.3 μS cm−1 (pH and conductivity were determined in a 1:5 soil/water mixture) (Table 1). Debris, plant residues, films, and other soil impurities were removed, and then the soil was air-dried and ground to pass through a 2-mm sieve. Subsequently, phosphorus supplements were added to the soil as calcium super-phosphate at seven phosphorus concentrations (0, 12.5, 25, 50, 75, 150, and 300 mg P kg−1 soil). Based on previous research experience, these were divided into three gradients: the low level (12.5–50 mg P kg−1), optimum level (75–150 mg P kg−1), and high level (300 mg P kg−1) (Lu et al., 2022).
Nitrogen and K were added to the soil as urea and potassium sulfate at 150 and 100 mg kg−1 soil, respectively. These additives were mixed well with 8 kg of soil and filled into several 5-L pots. Cotton seeds were surface sterilized with 10% hydrogen peroxide (H2O2) for 30 min, rinsed 3–5 times in deionized water, and screened for full-grained ones. Following the screening, the seeds germinated in a humid and dark environment for 72 h at 18°C–20°C. After that, 6–8 seeds were planted in each pot, and the seedlings were laid aside with two plants per pot at the 2-true-leaf stage. The pot experiment was designed as a complete random experiment comprising 14 treatments and 18 repetitions. All pots were watered daily to 75% of field water holding capacity by weights. The cultivation conditions in the glasshouse were a day–night cycle of 14 h/10 h, day/night temperatures of 25°C/18 °C, and relative humidity of 60%–75% (Figure 1).
The plants were harvested 60 and 130 days after planting (DAP) at seedling and flowering stages, respectively. In each treatment, during these two stages, three pots were randomly chosen to sample for shoot, root, and soil. The shoot samples (stems, leaves, and reproductive organs) were washed and heat-inactivated at 105°C for 15 min, dried at 60°C for 72 h, weighed, and ground for phosphorus concentration analysis. All visible roots in each pot were carefully picked out manually and stored in an ice box before laboratory transfer, during which time the soil samples were taken. The root samples were carefully cleaned with tap water and refrigerated at 4°C in the laboratory before the root morphology measurements were taken. The soil samples were air-dried and ground to pass through a 1-mm sieve for the analysis of soil Olsen-P. The remaining three pots in each treatment were sampled for the analysis of Pi transporter expressions in roots. Finally, the roots were gently taken out, immediately washed, and stored in liquid nitrogen for further RNA extraction (Figure 2).
In this study, Olsen-P was used as the indicator of plant-available phosphorus, or soil phosphorus availability, in gray desert soil (Olsen, 1954). Air-dried soil was extracted with 0.5 M NaHCO3 at pH 8.5 (180 rpm, 25°C). Plant P concentration was measured by the molybdo–vanado–phosphate method after the samples were digested with 5 mL concentrated sulfuric acid and 10 mL 30% H2O2 (Bao, 2000). Subsequently, plant phosphorus uptake was calculated from plant dry weight and phosphorus concentration.
To measure the morphological traits of roots, clean root samples were dispersed in water in a transparent array (30 cm × 20 cm × 2 cm) and imaged using a scanner (Epson Expression 1600, Seiko Epson, Nagano, Japan) at a resolution of 800 dpi. The root images were quantitatively analyzed with WinRHIZO Pro software (Regent Instruments, Quebec, Canada). These analyses provided information about the total root length and average root diameter. Root dry weight was determined by weighing the oven-dried samples after scanning. The specific root length was calculated by dividing the total root length by the root dry weight, and the root/shoot ratio was assessed based on root dry weight and shoot dry weight. Finally, the shoot phosphorus uptake was calculated from the shoot dry weight and shoot phosphorus concentration.
A total of 17 phosphorus transporters in the Pht1 family (GhPT1 to GhPT16) have been reported for cotton (Chao et al., 2017). In this report, we selected five highly expressed genes (GhPT5, GhPT6, GhPT7, GhPT15, and GhPT16) in the root system to explore the expression of Pht1 genes in cotton roots with different phosphorus efficiencies. Real-time quantitative RT-PCR (qRT-PCR) (Calderon-Vazquez et al., 2008) was used to analyze the expression of selected genes in cotton root samples. Total RNA was isolated from the frozen root tissues using TRIzol reagent (Biospin Plant Total RNA Extraction Kit). First-strand cDNA was synthesized using the All-In-One 5× RT MasterMix (Cat. No. G592, Shanghai) following the manufacturer’s protocol, and qRT-PCR was performed on the Mastercycler RealPlex 4 Real-Time PCR System (Bio-Rad, United States of America) based on the protocol of BlasTaq™ 2× qPCR MasterMix (Cat. No. G891, Shanghai) in which 20 μL of reaction volume contains 10 μL of SYBR Green PCR Mix, 0.6 μL of each forward and reverse primers, 2 μL of diluted cDNA template, and an appropriate amount of sterile double-distilled water. The program was set as follows: initial polymerase activation at 95°C, 3 min; 40 cycles at 95°C, 15 s; and 60°C, 1 min. The specificity of PCR amplification was evaluated with a melt curve analysis from 60 uC to 95 uC following the final PCR cycle. All reactions were performed with three biological replicates. The UBQ7 gene was used as the internal control as reported by NCBI, and the transcription levels of each gene were normalized to that of UBQ7 by the 2−△△Ct method.
The sequences of gene-specific primers used for the five transporters and UBQ7 were provided by NCBI and CottonGen website (Background of soil physicochemical properties).
Data are presented as means and standard errors (SE). The Olsen-P, shoot biomass, root biomass, shoot phosphorus concentration, and shoot phosphorus uptake in each treatment during the pot experiment were compared by multiple factor ANOVA using SPSS 25.0 (SPSS, Chicago, IL, United States). The differences among the means were tested by Tukey’s test, and those with a p < 0.05 were considered significant. The relationships between soil Olsen-P and shoot phosphorus uptake were determined as exponential equations. The relationships between soil Olsen-P and total root length, average root diameter, specific root length, and root/shoot ratio were visualized using empirical polynomial equations (inverse third order). Finally, the relationships between soil Olsen-P, root morphological traits, expressions of the selected phosphorus transporter genes, and shoot phosphorus uptake were plotted using SigmaPlot 14.0.
Both the above- and below-ground biomass accumulation of the two cotton cultivars at the seedling and flowering stages were positively correlated with the phosphorus application rate up to 150 mg kg−1 (Figure 3).
FIGURE 3. Above - and below-ground biomass accumulation responses of two genotypes of cotton to different phosphorus application rates: (A) 60d shoot biomass of XLZ13 and XLZ19, (B) 130d shoot biomass of XLZ13 and XLZ19, (C) 60d root biomass of XLZ13 and XLZ19, and (D) 130d root biomass of XLZ13 and XLZ19. Each symbol represents the average of three repetitions (±SE). Different lowercase letters indicate the significant difference in biomass of the same cotton variety under different phosphorus supply levels in the same period (p < 0.05).
With phosphorus deficiency treatments, XLZ19 showed slightly slower growth in total biomass than XLZ13 at the seedling stage (Figures 3A, C). At the flowering stage, the shoot dry weight and root dry weight of both cultivars during the pot experiment rose rapidly along with higher phosphorus application up to 150 mg kg−1 soil, where the biomass of both cultivars reached the maximum (Figures 3B, D). XLZ19 grew better than XLZ13 at the flowering stage, and the shoot biomass of XLZ19 was 1.60%, 0.86%, and 11.51% higher than that of XLZ13 under phosphorus deficiency treatments (Figures 3B, D). For the root biomass, the extent of improvement observed in XLZ19 was 21.49%, 21.51%, and 18.36% (Figure 3B). Under optimum phosphorus treatments, the shoot biomass of XLZ19 increased by 6.48% and 9.93% when compared with that of XLZ13 at the flowering stage (Figure 3B). For the root biomass, the improvement was 16.60% and 24.51% (Figure 3B). However, when phosphorus application rates exceeded 150 mg P kg−1, the shoot and root biomass of both cultivars did not show improvements.
From these results, it may be inferred that as the phosphorus application rate increases, P-efficient cotton XLZ19 may form more biomass by absorbing more phosphorus (Figures 3A, B); in the middle and late stages of cotton growth (130 d), the shoot biomass and root biomass of XLZ19 were higher than that of phosphorus-inefficient cotton XLZ13 (Figures 3A, B). XLZ19 was more tolerant to low phosphorus conditions, which confirmed that phosphorus-efficient cotton had a stronger ability to absorb soil phosphorus than phosphorus-inefficient varieties (Figures 3A, B).
Soil Olsen-P was significantly affected by phosphorus application. As the phosphorus application level increased, soil Olsen-P for both cultivars also rose (Figures 4A, B). During the phosphorus deficiency treatment, soil Olsen-P did not differ significantly between the two cultivars at the seedling stage (Figure 4A), but XLZ19 showed higher soil Olsen-P than XLZ13 at the flowering stage by 62.19%, 112.66%, and 3.54% (Figure 4B). During the optimum phosphorus treatments, XLZ19 revealed slightly lower soil Olsen-P than XLZ13 at the seedling and flowering stages (Figures 4A, B). Considering the shoot phosphorus uptake results, the soil Olsen-P findings indicated that the root system of XLZ19 had a higher ability to activate soil phosphorus than did XLZ13 in the middle and late growth stages (Figures 4C, D). Therefore, XLZ19 plants may absorb more phosphorus, and less phosphorus remains in the soil where XLZ19 is planted.
FIGURE 4. Changes in soil Olsen-P content under different phosphorus supply levels: (A) 60d XLZ13 and XLZ19 (seedling stage) and (B) 130d XLZ13 and XLZ19 (flowering stage). Each symbol represents the average of three repetitions (±SE). Each symbol represents the average of three repetitions (±SE). Different lowercase letters indicate the significant difference in soil Olsen-P of the same cotton variety under different phosphorus supply levels in the same period (p < 0.05).
There was a significant exponential relationship between shoot phosphorus uptake and soil Olsen-P until soil Olsen-P surpassed approximately 30 mg kg−1, where the shoot phosphorus uptake leveled off (Figure 5).
FIGURE 5. Response of whole plant P accumulation to soil available P increase in two genotypes of cotton: (A) 60d XLZ13 (seedling stage), (B) 60d XLZ19 (seedling stage), (C) 130d XLZ13 (flowering stage), and (D) 130d XLZ19 (flowering stage). Each symbol represents the average of three repetitions (±SE).
At the seedling stage under phosphorus deficiency treatments, XLZ19 showed 21.79%, 14.01%, and 32.06% higher shoot phosphorus uptake than did XLZ13. Under optimum phosphorus treatment, the improvement became 30.67% and 15.69%. Under high phosphorus treatment, the improvement was merely 0.31% (Figures 5A, B). At the flowering stage, when soil Olsen-P exceeded approximately 20 mg kg−1, the shoot phosphorus uptake plateaued (Figures 5C, D). Under phosphorus deficiency treatment, XLZ19 demonstrated 33.51%, 23.07%, and 18.76% higher shoot phosphorus uptake than did XLZ13. Under optimum phosphorus treatment, the improvement was 46.56% and 37.27%. Under high phosphorus treatment, the improvement was 12.28% (Figures 5C, D).
In summary, XLZ19 was obviously superior to XLZ13 in terms of shoot phosphorus uptake under low and optimum phosphorus application rates (Figure 5).
As soil Olsen-P increased from a low level at the seedling stage, the root/shoot ratio of XLZ13 dropped significantly and then substantially to a minimum when Olsen-P exceeded 35.39 mg kg−1. For XLZ19, the minimum root/shoot ratio appeared at an Olsen-P of 18.82 mg kg−1 (Figure 6A). Both XLZ13 and XLZ19 showed significantly higher root/shoot ratios at the flowering stage, demonstrating varied responses to Olsen-P when compared at the seedling stage (Figure 6B). In this stage, as soil Olsen-P increased, the root/shoot ratio of XLZ13 decreased to a minimum of 0.1 at an Olsen-P of 51.46 mg kg−1. XLZ19 revealed similar responses, but the minimum root/shoot ratio appeared at an Olsen-P of 24.74 mg kg−1 (Figure 6B). At the flowering stage, under phosphorus deficiency treatments, XLZ19 showed a 19.57%, 20.48%, and 6.15% higher root/shoot ratio than did XLZ13. For the optimum phosphorus treatment, the improvement was 9.50% and 13.26% (Figure 6B). For the high phosphorus treatment, the enhancement was 31.02% (Figure 6B). Therefore, the root–shoot ratio of XLZ19 was higher than that of XLZ13 under all phosphorus treatments during the flowering stage (Figure 6B).
FIGURE 6. Response of root/shoot ratio to soil available P increase in two genotypes of cotton: (A) 60d XLZ13 and XLZ19 (seedling stage) and (B) 130d XLZ13 and XLZ19 (flowering stage). Each symbol represents the average of three repetitions (±SE).
The total root length and soil Olsen-P were found to be significantly correlated (Figures 7A, D). During the seedling stage, the total root length of potted plants first increased as soil Olsen-P rose but started to drop when Olsen-P exceeded 25.88–29.82 mg kg-1 (Figure 7A). At the flowering stage, as the soil phosphorus supply rose, the total root length grew first and then became stable after soil Olsen-P rose higher than 21.51 mg kg-1 (Figure 7D). At the flowering stage, under the phosphorus deficiency treatment, XLZ19 showed a 41.53%, 50.12%, and 42.08% longer total root length than XLZ13. For the optimum phosphorus treatment, the improvement was 53.84% and 45.94%. For the high phosphorus treatment, the improvement was 61.18% (Figure 7D). Therefore, XLZ19 demonstrated a longer total root length and better phosphorus absorption (Figures 7A, D).
FIGURE 7. Root morphological traits in response to increasing soil phosphorus supply. (A) Total root length (seedling stage), (B) average root diameter (seedling stage), (C) specific root length (seedling stage), (D) total root length (flowering stage), (E) average root diameter (flowering stage), and (F) specific root length (flowering stage). Each symbol represents the average of three repetitions (±SE).
As soil Olsen-P rose, the root average diameter decreased gradually at the flowering stage and remained stable when Olsen-P exceeded 29.82 mg kg−1 (Figure 7E). At the flowering stage, under phosphorus deficiency treatments, XLZ19 showed 23.90%, 40.57%, and 36.62% smaller root average diameter than XLZ13. For the optimum phosphorus treatment, the shrinkage extent was 41.00% and 26.43%. For the high phosphorus treatment, the shrinkage extent was 24.37% (Figure 7E). Therefore, XLZ19 demonstrated smaller root average diameters and increased phosphorus absorption by increasing the proportion of fine roots (Figure 7E).
As soil Olsen-P increased, the specific root length of XLZ13 began to increase substantially at first during the seedling stage before decreasing at Olsen-P levels over 35.39 mg kg−1 (Figure 7C). At the flowering stage, this specific root length shrank significantly, but the response to Olsen-P was similar to that observed at the seedling stage (the response plateaued for Olsen-P levels over 21.51 mg kg−1) (Figure 7F). A similar relationship between the specific root length and soil Olsen-P was observed with XLZ19 as well. During the seedling stage, the turning point was at soil Olsen-P of 25.88 mg kg−1 (Figure 7C), while during the flowering stage, it plateaued at a soil Olsen-P of 19.23 mg kg−1 (Figure 7F). At the flowering stage, under the phosphorus deficiency treatment, XLZ19 showed 16.49%, 23.54%, and 20.05% longer specific root length than did XLZ13. For the optimum phosphorus treatment, the improvement was 31.94% and 17.21%. For the high phosphorus treatment, the improvement was 18.92% (Figure 7F). At the seedling and flowering stages, XLZ19 demonstrated a longer specific root length than did XLZ13 under both low and normal phosphorus treatments.
We detected the expression of five Pht1 Pi transporter genes at the seedling and flowering stages. In the potted plants, the five genes similarly responded to the soil phosphorus supply and were expressed varyingly during different periods. Particularly, the expression of all five GhPTs genes grew significantly in the flowering stage when compared to the seedling stage. The transcription levels initially decreased with growing phosphorus supply, but above a certain threshold, the gene expression remained high even with low phosphorus supply. Compared with a high level of phosphorus supply, low and normal levels significantly induced expressions of the five GhPTs genes in the roots (Figures 8A–J). Specifically, the GhPT5 expression in XLZ19 showed the highest improvement at 9.50-fold under P0 treatment (Figure 8B).
FIGURE 8. Expression of root Pht1 transporter genes in response to increasing soil phosphorus supply. Roots were sampled at the seedling and florescence stages (60 and 130 days after planting). Relative gene expression level was measured by real-time quantitative RT-PCR. UBQ7 was used as the internal control. For each gene, the lowest treatment was set to 1.0. Each symbol represents the mean (±SE) of three replicates for the pot experiment. Different lowercase letters indicate that the relative expression of the same Pht1 gene of the same cotton variety is significantly different under different phosphorus supply levels (p < 0.05).
The expression of the GhPTs genes varied between XLZ19 and XLZ13 (Figures 8A–J). When harvested at 60d and 130d, the relative expression of the GhPTs genes in XLZ19 under low phosphorus supply was 1.07- to 2.94-fold and 1.24- to 3.24-fold when compared with that in XLZ13. As the phosphorus supply shifted from normal to high levels, the transcription level dropped rapidly, and the gene expression remained low with normal phosphorus supply (Figures 8A–J). When harvested at 60d and 130d, normal phosphorus supply induced GhPTs gene expressions in XLZ19 by 1.47- to 3.21-fold and 1.07- to 2.61-fold when compared to that in XLZ13 (Figures 8A–J). The expression levels of genes responsive to low phosphorus stress suggest that phosphorus-efficient cultivar XLZ19 maintains better growth conditions and accumulates more dry matter in low phosphorus and normal phosphorus environments by regulating the high expression of GhPTs genes in the roots.
Phosphorus fertilizers may help increase soil phosphorus supply (Olsen-P), biomass yield, grain yield, and shoot phosphorus uptake (Jing et al., 2012; Wang et al., 2018; El Mazlouzi et al., 2020). In addition, the relationship among soil phosphorus supply, crop biomass, and shoot phosphorus uptake was reported to be synchronous growth from initial low levels to an asymptote (Marschner et al., 2007; Teng et al., 2013). Based on this finding, it has been proposed that there is a critical level of P supply (either P fertilizer rate or Olsen-P) for optimum shoot phosphorus uptake or crop biomass (Li et al., 2008; Bai et al., 2013). The present study revealed that phosphorus fertilizers may significantly increase Olsen-P, shoot biomass, shoot phosphorus concentration, and shoot phosphorus uptake during the seedling and flowering stages (Figures 1–3), but shoot biomass and shoot phosphorus uptake did not increase further at phosphorus supply levels greater than 150 mg P kg−1 (Olsen-P 30.0–35.4 mg kg−1) at the seedling stage and 75 mg P kg−1 (Olsen-P 19.2–21.5 mg kg−1) at the flowering stage (Figures 1, 3). Therefore, it may be inferred that the critical soil Olsen-P for cotton growth is 20–30 mg kg−1 (Figures 1–3), a value slightly higher than that observed in previous reports (15–25 mg kg−1) (Mai et al., 2018; Huo et al., 2023). In the meantime, this study suggests that the pot experiment is appropriate for characterizing the responses of cotton growth to deficient, adequate, and excessive phosphorus supply. Under all investigated phosphorus supply levels, the shoot biomass and root biomass of phosphorus-efficient XLZ19 were higher than that of phosphorus-inefficient XLZ13 (Figure 1), indicating that XLZ19 could mobilize and take up more phosphorus from the soil than XLZ13.
Roots play an important role in phosphorus uptake. They may alter their morphological traits to adapt to phosphorus supply conditions in the soil (Shen et al., 2013; Wen et al., 2019). Generally speaking, phosphorus deficiency can boost root growth, resulting in longer total root length and fine root length, higher root/shoot ratio and specific root length, greater root surface area and root volume, and more dispersed lateral root (Zhu and Lynch, 2004; Zhang et al., 2012; Deng et al., 2014; Fernandez et al., 2015). However, chronic phosphorus deficiency stunts root growth due to insufficient phosphorus supply (Wissuwa et al., 2005; George et al., 2011; Zhang and Ning, 2022), and excess phosphorus supply may inhibit root elongation and lateral root initiation (Malhotra et al., 2018; Ruiz et al., 2020; He et al., 2021). Only an optimum phosphorus supply may help develop the root system and create sufficient phosphorus use efficiency (Fernandes et al., 2014; Mai et al., 2018; Xia et al., 2021). In the present study, as Olsen-P ranged from 5 mg kg-1 to 10 mg kg-1, root growth was less inhibited than shoot biomass. Both total root length and specific root length rose, but root/shoot ratio and average root diameter dropped (Figures 1, 5), suggesting that cotton roots were more sensitive than shoots to phosphorus deficiency and cotton adapts to low phosphorus conditions by promoting root development, which is consistent with published reports on other crops (Hill et al., 2006; Pang et al., 2009; Teng et al., 2013). At an Olsen-P range of 15 mg kg-1–30 mg kg-1, root morphological parameters (total root length and specific root length) increased significantly, while the root/shoot ratio and average root diameter were relatively low and stable (Figure 5). These findings demonstrate that root growth can be improved by optimizing phosphorus supply in culture conditions, which is also in accordance with published reports on cotton (Mai et al., 2018) and other crops (Teng et al., 2013; Zhang et al., 2016). Finally, the root morphological parameters of XLZ19 were significantly better than that of XLZ13 at an Olsen-P range of 5 mg kg-1–30 mg kg-1. These parameters decreased significantly at excessive phosphorus levels (Olsen-P >30 mg kg-1), which could have resulted from the fact that at high phosphorus supply, soil phosphorus availability was no longer the limiting factor for plant growth. Similar results have been reported in other crops as well (Deng et al., 2014; Malhotra et al., 2018).
In addition to reshaping root morphology to forage for P, plants undergo biochemical changes to promote phosphate uptake (Kumar et al., 2021). These high-affinity phosphate transporters are important participants in phosphate uptake, transport, distribution, and reuse, as well as in activation, efficient uptake, and transport of phosphorus from the root (Xiao et al., 2006). Among the phosphorus transporters in the Pht1 family reported for cotton, GhPT5, GhPT6, GhPT7, GhPT15, and GhPT16 are found to be highly expressed in cotton roots under low phosphorus stress. Many high-affinity phosphate transporters in plants are root-specific or dominantly expressed in roots, and their expression characteristics are hardly inducible by low phosphorus levels (Aslam et al., 2014; Zhang et al., 2016). In the present study, we found that the expression of these five genes is negatively correlated with soil phosphorus supply, and in the case of low phosphorus supply (Olsen-P: 5–10 mg kg−1), upregulation of gene expression was the most significant (Figure 6). On the other hand, the most significant downregulation of the expression of these genes was induced at a soil phosphorus supply higher than the critical level for shoot growth (Olsen-P >30 mg kg-1) (Figure 6), suggesting that compared to shoots, roots respond at the molecular level to increased phosphorus supply at a higher phosphorus level. The expression levels of all five investigated genes were relatively higher and stable under optimum phosphorus supply (Olsen-P 15–20 mg kg-1), and XLZ19 showed an improvement of 1.07- to 3.24-fold in the relative expression level of the GhPTs gene when compared with XLZ13. Also, the overall upregulation of these five genes was more significant at the flowering stage than at the seedling stage (Figure 6). In plants, PHT1 family members are highly expressed in root tissues, such as root cap and external root layers (e.g., root epidermis and root hairs), in response to low Pi conditions to improve Pi uptake (Nussaume et al., 2011; Baker et al., 2015). The expression levels of GhPTs genes in roots increased gradually as the time of low phosphorus treatment prolonged, which is consistent with previous results (Maharajan et al., 2019). Meanwhile, the gradual downregulation of the expression of the five genes with increasing phosphorus supply was consistent with the lower average root diameter at the flowering stage, another phenomenon observed with further increase in phosphorus supply (Figure 7E). XLZ19 showed longer total root length, smaller average root diameter, and higher shoot phosphorus uptake and shoot biomass than did XLZ13. Besides, under low and normal phosphorus treatments, the expression levels of the five GhPTs genes in XLZ19 were also significantly higher than that in XLZ13. Therefore, phosphorus-efficient cotton was capable of adjusting its root morphology by increasing the proportion of fine roots to absorb and utilize more phosphorus. Such responses probably indicate the cooperation of root morphology and biochemistry for efficient phosphorus acquisition in the direct pathway under insufficient soil phosphorus supply (Lambers et al., 2006).
In this report, we discussed the differences in terms of physiological and gene expression responses of cotton with varying phosphorus efficiencies to different phosphorus supply levels. This study helps provide theoretical and technical support for improving cotton phosphorus efficiency, guiding scientific fertilization, breeding new cotton varieties with high phosphorus efficiency, and improving cotton production and planting resources.
However, since this study only focused on morphological and molecular responses of the roots of two cotton cultivars in a pot experiment, further research on phosphorus uptake in the field is required to clarify the genotypic control of phosphorus adsorption. A possible route for future research could be the transfer of GhPTs genes into Arabidopsis thaliana and other model plants. Verification of gene and yeast functions by yeast mutant complementation test could also be performed, and the transcription factors that regulate GhPTs gene expression in upland cotton may also be investigated. Last but not the least, the mechanism of GhPTs’ role in the process of P absorption and utilization in cotton plants can be studied further in detail.
In order to maximize the intrinsic potential of cotton roots to obtain phosphorus, we suggest that the optimal range of P fertilizer supply in the soil should be between 75 and 150 mg P kg−1. The ideal range of soil Olsen-P level at the seedling stage should be between 20 and 30 mg kg−1, while at the flowering stage, it should be between 15 and 25 mg kg−1. These levels may not only promote the good development of cotton roots but also obtain the best phosphorus uptake. Besides, the mechanisms underlying phosphorus uptake differed between the two cotton cultivars. Cultivar XLZ19 showed higher shoot P uptake than cultivar XLZ13. This is primarily due to the fact that shoot P uptake and shoot biomass of XLZ19 benefited from both the modification in root morphology and higher expression of phosphate transporter genes GhPT5, GhPT6, GhPT7, GhPT15, and GhPT16 under varying P conditions (Ar, 2009; Cordell et al., 2009; Cordell et al., 2011; Jasinski, 2011; Li et al., 2022; Misson et al., 2004; Shen et al., 2019; Vaccari, 2009).
The data sets presented in this study can be found in online repositories. The names of the repository/repositories and accession number(s) can be found in the article/Supplementary Material.
FC wrote and edited the original draft and conducted the visualization and formal analysis. BC and FC designed the experiments. FC, YZ, MZ, TH, JW, and BC performed the experiments. FC and BC analyzed the data. BC revised the manuscript. All authors contributed to the article and approved the submitted version.
This work was supported by the Science Foundation for Outstanding Young Scholars of Xinjiang Uygur Autonomous Region, the Project for Young Top-Notch Talents in Science and Technology of Xinjiang Uygur Autonomous Region (2022TSYCCX0085), the National Natural Science Foundation of China (31960629), the Key Research and Development Project in Xinjiang Uygur Autonomous Region (2022B02033-1), and Special Topics of Major Science and Technology in Xinjiang Uygur Autonomous Region (2022A02007-2).
The authors declare that the research was conducted in the absence of any commercial or financial relationships that could be construed as a potential conflict of interest.
All claims expressed in this article are solely those of the authors and do not necessarily represent those of their affiliated organizations, or those of the publisher, editors, and reviewers. Any product that may be evaluated in this article, or claim that may be made by its manufacturer, is not guaranteed or endorsed by the publisher.
The Supplementary Material for this article can be found online at: https://www.frontiersin.org/articles/10.3389/fenvs.2023.1127920/full#supplementary-material
Andersson, H., Bergström, L., Djodjic, F., Ulén, B., and Kirchmann, H. (2013). Topsoil and subsoil properties influence phosphorus leaching from four agricultural soils. J. Environ. Qual. 42 (2), 455–463. doi:10.2134/jeq2012.0224
Aslam, M., Saxena, P., and Sarma, A. K. (2014). Green technology for biodiesel production from Mesua ferrea L. Seed oil. Ener. Environ. res. 4 (2), 11. doi:10.5539/eer.v4n2p11
Bai, Z., Li, H., Yang, X., Zhou, B., Shi, X., Wang, B., et al. (2013). The critical soil P levels for crop yield, soil fertility and environmental safety in different soil types. Plant Soil 372 (1), 27–37. doi:10.1007/s11104-013-1696-y
Baker, A., Ceasar, S. A., Palmer, A. J., Paterson, J. B., Qi, W., Muench, S. P., et al. (2015). Replace, reuse, recycle: Improving the sustainable use of phosphorus by plants. J. Exp. Bot. 66, 3523–3540. doi:10.1093/jxb/erv210
Bao, S. D. (2000). Soil and agricultural chemistry analysis. Sacramento, California, United States: Creative Media Partners, LLC.
Cai, S., Liu, F., and Zhou, B. (2020). Genome-Wide identification and expression profile analysis of the PHT1 gene family in gossypium hirsutum and its two close relatives of subgenome donor species. Int. J. Mol. Sci. 21 (14), 4905. doi:10.3390/ijms21144905
Calderon-Vazquez, C., Ibarra-Laclette, E., Caballero-Perez, J., and Herrera-Estrella, L. (2008). Transcript profiling of Zea mays roots reveals gene responses to phosphate deficiency at the plant-and species-specific levels. J. Exp. Bot. 59 (9), 2479–2497. doi:10.1093/jxb/ern115
Chao, M., Zhang, Z., Song, H., Li, C., Zhang, X., Hu, G., et al. (2017). Genome-wide identification and expression analysis of Pht1 family genes in cotton (Gossypium hirsutum L). Cotton Sci. 29 (1), 59–69. doi:10.3389/fpls.2017.00543
Chen, B. L., Wang, Q. H., Heike, B., Sheng, J. D., Luo, J., Chai, Z. P., et al. (2019). Genotypic differences in phosphorus acquisition efficiency and root performance of cotton (Gossypium hirsutum) under low-phosphorus stress. Crop Pasture Sci. 70, 344–358. doi:10.1071/cp18324
Conley, D. J., Paerl, H. W., Howarth, R. W., Boesch, D. F., Seitzinger, S. P., Havens, K. E., et al. (2009). Controlling eutrophication: Nitrogen and phosphorus. Science 323 (5917), 1014–1015. doi:10.1126/science.1167755
Cordell, D., Drangert, J. O., and White, S. (2009). The story of phosphorus: Global food security and food for thought. Glob. Environ. change 19 (2), 292–305. doi:10.1016/j.gloenvcha.2008.10.009
Cordell, D., Rosemarin, A., Schröder, J. J., and Smit, A. L. (2011). Towards global phosphorus security: A systems framework for phosphorus recovery and reuse options. Chemosphere 84 (6), 747–758. doi:10.1016/j.chemosphere.2011.02.032
Deng, Y., Chen, K., Teng, W., Zhan, A., Tong, Y., Feng, G., et al. (2014). Is the inherent potential of maize roots efficient for soil phosphorus acquisition? PLOS one 9 (3), e90287. doi:10.1371/journal.pone.0090287
Deng, Y., Teng, W., Tong, Y. P., Chen, X. P., and Zou, C. Q. (2018). Phosphorus efficiency mechanisms of two wheat cultivars as affected by a range of phosphorus levels in the field. Front. Plant Sci. 9, 1614. doi:10.3389/fpls.2018.01614
El Mazlouzi, M., Morel, C., Robert, T., Yan, B., and Mollier, A. (2020). Phosphorus uptake and partitioning in two durum wheat cultivars with contrasting biomass allocation as affected by different P supply during grain filling. Plant Soil 449 (1), 179–192. doi:10.1007/s11104-020-04444-0
Eshel, A., and Beeckman, T. (2013). Plant roots: The hidden half. Boca Raton, Florida, United States: CRC Press.
Feng, G., Gai, J., Feng, X., Li, H., Zhang, L., Yi, K., et al. (2019). Strategies for improving fertilizer phosphorus use efficiency in Chinese cropping systems. Front. Agr. Sci. Eng. 6 (4), 341–347. doi:10.15302/J-FASE-2019280
Feng, L., Dai, J., Tian, L., Zhang, H., Li, W., and Dong, H. (2017). Review of the technology for high-yielding and efficient cotton cultivation in the northwest inland cotton-growing region of China. Field crop Res. 208, 18–26. doi:10.1016/j.fcr.2017.03.008
Fernandes, A. M., Soratto, R. P., and Gonsales, J. R. (2014). Root morphology and phosphorus uptake by potato cultivars grown under deficient and sufficient phosphorus supply. Sci. Hortic. 180, 190–198. doi:10.1016/j.scienta.2014.10.035
Fernandez, M. C., and Rubio, G. (2015). Root morphological traits related to phosphorus-uptake efficiency of soybean, sunflower, and maize. J. Plant Nutr. Soil Sci. 178 (5), 807–815. doi:10.1002/jpln.201500155
George, T. S., Fransson, A. M., Hammond, J. P., and White, P. J. (2011). “Phosphorus nutrition: Rhizosphere processes, plant response and adaptations,” in Phosphorus in action (Berlin, Heidelberg: Springer), 245–271. doi:10.1007/978-3-642-15271-9_10
He, H., Wu, M., Su, R., Zhang, Z., Chang, C., Peng, Q., et al. (2021). Strong phosphorus (P)-zinc (Zn) interactions in a calcareous soil-alfalfa system suggest that rational P fertilization should be considered for Zn biofortification on Zn-deficient soils and phytoremediation of Zn-contaminated soils. Plant Soil 461 (1), 119–134. doi:10.1007/s11104-020-04793-w
Hill, J. O., Simpson, R. J., Moore, A. D., and Chapman, D. F. (2006). Morphology and response of roots of pasture species to phosphorus and nitrogen nutrition. Plant Soil 286, 7–19. doi:10.1007/s11104-006-0014-3
Huo, W., Peng, Y., Maimaitiaili, B., Batchelor, W. D., and Feng, G. (2023). Phosphorus fertilizer recommendation based on minimum soil surplus for cotton growing in salt-affected soils. Field crop Res. 291, 108799. doi:10.1016/j.fcr.2022.108799
Jasinski, S. M. (2011). Phosphate rock. Mineral commodity summaries. Reston, Virginia, USA: United States Geological Survey, 122–123.
Jing, J., Zhang, F., Rengel, Z., and Shen, J. (2012). Localized fertilization with P plus N elicits an ammonium-dependent enhancement of maize root growth and nutrient uptake. Field crop Res. 133, 176–185. doi:10.1016/j.fcr.2012.04.009
Kumar, S., Chugh, C., Seem, K., Kumar, S., Vinod, K. K., Mohapatra, T., et al. (2021). Characterization of contrasting rice (Oryza sativa L.) genotypes reveals the Pi-efficient schema for phosphate starvation tolerance. BMC plant Biol. 21 (1), 1–26. doi:10.1186/s12870-021-03015-4
Kumawat, C., Sharma, V. K., Meena, M. C., Dwivedi, B. S., Barman, M., Kumar, S., et al. (2018). Effect of crop residue retention and phosphorus fertilization on P use efficiency of maize (Zea mays) and biological properties of soil under maize-wheat (Triticum aestivum) cropping system in an Inceptisol. Indian J. Agric. Sci. 88 (8), 1184–1189. doi:10.56093/ijas.v88i8.82527
Lambers, H. (2022). Phosphorus acquisition and utilization in plants. Annu. Rev. Plant Biol. 73, 17–42. doi:10.1146/annurev-arplant-102720-125738
Lambers, H., Shane, M. W., Cramer, M. D., Pearse, S. J., and Veneklaas, E. J. (2006). Root structure and functioning for efficient acquisition of phosphorus: Matching morphological and physiological traits. Ann. Bot. 98 (4), 693–713. doi:10.1093/aob/mcl114
Li, H., Huang, G., Meng, Q., Ma, L., Yuan, L., Wang, F., et al. (2011). Integrated soil and plant phosphorus management for crop and environment in China: A review. Plant Soil 349, 157–167. doi:10.1007/s11104-011-0909-5
Li, H., Shen, J., Zhang, F., Tang, C., and Lambers, H. (2008). Is there a critical level of shoot phosphorus concentration for cluster-root formation in Lupinus albus. Funct. Plant Biol. 35 (4), 328–336. doi:10.1071/fp07222
Li, P., Weng, J., Rehman, A., and Niu, Q. (2022). Root morphological and physiological adaptations to low phosphate enhance phosphorus efficiency at melon (cucumis melo L) seedling stage. Horticulturae 8 (7), 636. doi:10.3390/horticulturae8070636
Lu, Z., Sun, H., and Zhang, J. (2022). Study on the abundance and deficiency index of available phosphorus in cotton soil in China and the appropriate phosphorus application rate. China Soil Fertilizer (2), 197–206. doi:10.3969/j.issn.1006-8082.2018.05.001
Maharajan, T., Ceasar, S. A., Krishna, T. P. A., and Ignacimuthu, S. (2019). Phosphate supply influenced the growth, yield and expression of PHT1 family phosphate transporters in seven millets. Planta 250 (5), 1433–1448. doi:10.1007/s00425-019-03237-9
Mai, W., Xue, X., Feng, G., Yang, R., and Tian, C. (2018). Can optimization of phosphorus input lead to high productivity and high phosphorus use efficiency of cotton through maximization of root/mycorrhizal efficiency in phosphorus acquisition? Field crop Res. 216, 100–108. doi:10.1016/j.fcr.2017.11.017
Malhotra, H., Sharma, S., and Pandey, R. (2018). “Phosphorus nutrition: Plant growth in response to deficiency and excess,” in Plant nutrients and abiotic stress tolerance (Singapore: Springer), 171–190. doi:10.1007/978-981-10-9044-8_7
Marschner, P., Solaiman, Z., and Rengel, Z. (2007). Brassica genotypes differ in growth, phosphorus uptake and rhizosphere properties under P-limiting conditions. Soil Biol. Biochem. 39 (1), 87–98. doi:10.1016/j.soilbio.2006.06.014
Misson, J., Thibaud, M. C., Bechtold, N., Raghothama, K., and Nussaume, L. (2004). Transcriptional regulation and functional properties of Arabidopsis Pht1; 4, a high affinity transporter contributing greatly to phosphate uptake in phosphate deprived plants. Plant Mol. Biol. 55 (5), 727–741. doi:10.1007/s11103-004-1965-5
Müller, J., Toev, T., Heisters, M., Teller, J., Moore, K. L., Hause, G., et al. (2015). Iron-dependent callose deposition adjusts root meristem maintenance to phosphate availability. Dev. Cell 33, 216–230. doi:10.1016/j.devcel.2015.02.007
Niu, Y. F., Chai, R. S., Jin, G. L., Wang, H., Tang, C. X., and Zhang, Y. S. (2013). Responses of root architecture development to low phosphorus availability: A review. Ann. Bot. 112 (2), 391–408. doi:10.1093/aob/mcs285
Nussaume, L., Kanno, S., Javot, H., Marin, E., Pochon, N., Ayadi, A., et al. (2011). Phosphate import in plants: Focus on the PHT1 transporters. Front. plant Sci. 2, 83. doi:10.3389/fpls.2011.00083
Olsen, S. R. (1954). Estimation of available phosphorus in soils by extraction with sodium bicarbonate (No. 939). Washington DC, USA: US Department of Agriculture.
Pang, J., Ryan, M. H., Tibbett, M., Cawthray, G. R., Siddique, K. H. M., Bolland, M. D. A., et al. (2009). Variation in morphological and physiological parameters in herbaceous perennial legumes in response to phosphorus supply. Plant Soil 331, 241–255. doi:10.1007/s11104-009-0249-x
Peng, Y., Li, Q. J., Zhang, S. M., Bai, D. S., Aili, M., and Feng, G. (2020). Technical regulation of phosphate fertilizer application for cotton under mulch drip irrigation based on rhizosphere process control. China Cotton (6), 31–38.
Ruiz, S., Koebernick, N., Duncan, S., Fletcher, D. M., Scotson, C., Boghi, A., et al. (2020). Significance of root hairs at the field scale–modelling root water and phosphorus uptake under different field conditions. Plant soil 447 (1), 281–304. doi:10.1007/s11104-019-04308-2
Sattari, S. Z., Bouwman, A. F., Giller, K. E., and van Ittersum, M. K. (2012). Residual soil phosphorus as the missing piece in the global phosphorus crisis puzzle. Proceedings of the National Academy of Sciences 109 (16), 6348–6353. doi:10.1073/pnas.1113675109
Sharpley, A. N., Chapra, S. C., Wedepohl, R., Sims, J. T., Daniel, T. C., and Reddy, K. R. (1994). Managing agricultural phosphorus for protection of surface waters: Issues and options. J. Environ. Qual. 23 (3), 437–451. doi:10.2134/jeq1994.00472425002300030006x
Shen, J., Li, C., Mi, G., Li, L., Yuan, L., Jiang, R., et al. (2013). Maximizing root/rhizosphere efficiency to improve crop productivity and nutrient use efficiency in intensive agriculture of China. J. Exp. Bot. 64 (5), 1181–1192. doi:10.1093/jxb/ers342
Shen, J., Wang, L., Jiao, X., Meng, F., Zhang, L., Feng, G. U., et al. (2019). Innovations of phosphorus sustainability: Implications for the whole chain. Front. Agric. Sci. Eng. 6 (4), 321–331. doi:10.15302/J-FASE-2019283
Shenoy, V., and Kalagudi, G. (2005). Enhancing plant phosphorus use efficiency for sustainable cropping. Biotechnol. Adv. 23, 501–513. doi:10.1016/j.biotechadv.2005.01.004
Singh, D., Singh, K., Hundal, H. S., and Sekhon, K. S. (2012). Diagnosis and recommendation integrated system (DRIS) for evaluating nutrient status of cotton (Gossipium hirsutum). J. plant Nutr. 35 (2), 192–202. doi:10.1080/01904167.2012.636122
Svistoonoff, S., Creff, A., Reymond, M., Sigoillot-Claude, C., Ricaud, L., Blanchet, A., et al. (2007). Root tip contact with low-phosphate media reprograms plant root architecture. Nat. Genet. 39, 792–796. doi:10.1038/ng2041
Teng, W., Deng, Y., Chen, X., Xu, X., Chen, R., Lv, Y., et al. (2013). Characterization of root response to phosphorus supply from morphology to gene analysis in field-grown wheat. J. Exp. Bot. 64, 1403–1411. doi:10.1093/jxb/ert023
Vaccari, D. A. (2009). Phosphorus: A looming crisis. Sci. Am. 300 (6), 54–59. doi:10.1038/scientificamerican0609-54
Wang, J., Chen, Y., Wang, P., Li, Y. S., Wang, G., Liu, P., et al. (2018). Leaf gas exchange, phosphorus uptake, growth and yield responses of cotton cultivars to different phosphorus rates. Photosynthetica 56 (4), 1414–1421. doi:10.1007/s11099-018-0845-1
Wen, Z., Li, H., Shen, Q., Tang, X., Xiong, C., Li, H., et al. (2019). Tradeoffs among root morphology, exudation and mycorrhizal symbioses for phosphorus-acquisition strategies of 16 crop species. New Phytol. 223 (2), 882–895. doi:10.1111/nph.15833
Williamson, L. C., Ribrioux, S. P., Fitter, A. H., and Leyser, H. O. (2001). Phosphate availability regulates root system architecture in Arabidopsis. Plant physiol. 126 (2), 875–882. doi:10.1104/pp.126.2.875
Wissuwa, M., Gamat, G., and Ismail, A. M. (2005). Is root growth under phosphorus deficiency affected by source or sink limitations. J. Exp. Bot. 56 (417), 1943–1950. doi:10.1093/jxb/eri189
Xia, Z., Zhang, S., Wang, Q., Zhang, G., Fu, Y., and Lu, H. (2021). Effects of root zone warming on maize seedling growth and photosynthetic characteristics under different phosphorus levels. Front. Plant Sci. 12, 746152. doi:10.3389/fpls.2021.746152
Xiao, K., Liu, J., Dewbre, G., Harrison, M., and Wang, Z. Y. (2006). Isolation and characterization of root-specific phosphate transporter promoters from medicago truncatula. Plant Biol. 8 (4), 439–449. doi:10.1055/s-2005-873053
Yang, A., Kong, L., Wang, H., Yao, X., Xie, F., Wang, H., et al. (2020). Response of soybean root to phosphorus deficiency under sucrose feeding: Insight from morphological and metabolome characterizations. BioMed Res. Int. 2020, 1–11. doi:10.1155/2020/2148032
Yin, F. H., Kang, J. H., Huang, Z. W., and Ceng, D. C. (2005). A ∼(32)P study on the movement and utilization rate of phosphorus in special fertilizer for drip irrigation in cotton with water application. Northwest Agric. J. 6, 199–204.
Zhang, D., Zhang, C., Tang, X., Li, H., Zhang, F., Rengel, Z., et al. (2016). Increased soil phosphorus availability induced by faba bean root exudation stimulates root growth and phosphorus uptake in neighbouring maize. New Phytol. 209 (2), 823–831. doi:10.1111/nph.13613
Zhang, Y., Chen, F., Chen, X., Long, L., Gao, K., Yuan, L., et al. (2013). Genetic improvement of root growth contributes to efficient phosphorus acquisition in maize (Zea mays L). J. Integr. Agric. 12 (6), 1098–1111. doi:10.1016/s2095-3119(13)60489-x
Zhang, Y., and Ning, P. (2022). Differential root response of maize inbred seedlings to root growth restriction and phosphorus availability. Biologia 78, 31–39. doi:10.1007/s11756-022-01174-9
Zhang, Y., Peng, Y. U., Yun-Feng, P. E. N. G., Xue-Xian, L. I., Fan-Jun, C. H. E. N., and Chun-Jian, L. I. (2012). Fine root patterning and balanced inorganic phosphorus distribution in the soil indicate distinctive adaptation of maize plants to phosphorus deficiency. Pedosphere 22 (6), 870–877. doi:10.1016/S1002-0160(12)60073-3
Zhao, J., Fu, J., Liao, H., He, Y., Nian, H., Hu, Y., et al. (2004). Characterization of root architecture in an applied core collection for phosphorus efficiency of soybean germplasm. Chin. Sci. Bull. 49 (15), 1611–1620. doi:10.1360/04wc0142
Keywords: cotton, phosphorus application rates, soil Olsen-P, phosphorus uptake, dry matter, root morphology, phosphorus transporter, gene expression
Citation: Cai F, Zhang Y, Hou T, Zhang M, Wang J and Chen B (2023) Phosphorus uptake mechanisms associated with phosphorus application levels in two cotton cultivars. Front. Environ. Sci. 11:1127920. doi: 10.3389/fenvs.2023.1127920
Received: 20 December 2022; Accepted: 06 July 2023;
Published: 28 July 2023.
Edited by:
Yuncong Li, University of Florida, United StatesReviewed by:
Aziz Khan Khan, Guangxi University, ChinaCopyright © 2023 Cai, Zhang, Hou, Zhang, Wang and Chen. This is an open-access article distributed under the terms of the Creative Commons Attribution License (CC BY). The use, distribution or reproduction in other forums is permitted, provided the original author(s) and the copyright owner(s) are credited and that the original publication in this journal is cited, in accordance with accepted academic practice. No use, distribution or reproduction is permitted which does not comply with these terms.
*Correspondence: Bolang Chen, Y2hlbndhbmcyMDA5MTBAc2luYS5jb20=
Disclaimer: All claims expressed in this article are solely those of the authors and do not necessarily represent those of their affiliated organizations, or those of the publisher, the editors and the reviewers. Any product that may be evaluated in this article or claim that may be made by its manufacturer is not guaranteed or endorsed by the publisher.
Research integrity at Frontiers
Learn more about the work of our research integrity team to safeguard the quality of each article we publish.