- 1School of Geosciences, Yangtze University, Wuhan, China
- 2Guilin University of Technology at Nanning, Nanning, China
- 3Department of Soil and Environmental Sciences, Amir Muhammad Khan Campus, The University of Agriculture, Peshawar, Pakistan
- 4Department of Soil, Water and Ecosystem Sciences, Indian River Research and Education Center, University of Florida, Fort Pierce, FL, United states
- 5Department of Agronomy, Amir Muhammad Khan Campus, The University of Agriculture, Peshawar, Pakistan
- 6Key Laboratory of Grassland Agroecosystems, Lanzhou University, Lanzhou, China
- 7Department of Plant Breeding and Genetics, Amir Muhammad Khan Campus, The University of Agriculture, Peshawar, Pakistan
- 8Department of Plant Protection, Amir Muhammad Khan Campus, The University of Agriculture, Peshawar, Pakistan
- 9Department of Plant Breeding and Genetics, The University of Agriculture, Peshawar, Pakistan
- 10Guangxi Key Laboratory of Medicinal Resources Protection and Genetic Improvement, Guangxi Botanical Garden of Medicinal Plant, Nanning, China
Introduction: Biochar and biofertilizer have emerged as sustainable soil amendments to improve soil fertility and quality. However, it is necessary to determine how biochar’s various particle sizes and biofertilizers affect soil microbial biomass carbon (MBC), microbial biomass nitrogen (MBN), and CO2 sequestration.
Methods: This research examined two rhizobia inoculum products—Biozote-N and Rhizogold—along with three biochar particle sizes (<2, 2–5, and 5–10 mm) under incubation intervals of 3, 6, and 10 days.
Results: The MBC (µg g−1 soil) and MBN (µg g−1 soil) increased significantly (p < 0.05), however, a significant (p < 0.05) decrease in the organic C and mineralizable C within the <2 mm biochar treatment was observed. With biochar size of 2 mm on day 3, the CO2 evolution was 25% lower, but it increased 4%–19% from day 6–10 compared to the other biochar sizes and the biochar control. Sizes 2 and 2–5 mm were 43% lower in MBN, whereas 5–10 mm was 70% lower (µg g−1 OC). Biozote-N outperformed Rhizogold and no-biofertilizer on days 6 and 10, registering 6% and 20% higher MBC (g g−1 soil/OC), respectively. No biofertilizer increased CO2 production by 14% and 23% on days 3 and 6, respectively. On day 10, however, CO2 production inside Biozote and Rhizogold plots increased by 67% and 45% relative to the control, respectively. Biofertilizers enhanced MBN (µg g−1 OC) by 37%, with the contribution of Biozote-N being 18% more than that of Rhizogold. The Biozote-N and Rhizogold plots had a 13 and 28% increase in mineralizable N compared to the control. After the spring (lentil) crop, there was a 5%–18% higher MBC (g g−1 soil), 6%–17% higher MBC (g g−1 OC), 2%–10% greater CO2 evolution, 23% higher MBN, and 77% higher mineralizable N than after the fall (maize) crop.
Discussion: The results demonstrated the usefulness of Biozote-N for enhanced MBC and MBN, as they decreased C mineralization at the small biochar size (2 mm) even after 1 year of their combined application to lentil crop, showing that biochar-Biozote-N were more successful at boosting soil organic fertility and C sequestration.
1 Introduction
Because of the crucial structural, genetic, and functional contributions that nitrogen (N) makes to plant cells and the considerable growth and developmental processes necessary for increased yield, plants require enormous amounts of N (Bambara and Ndakidemi, 2010). Due to environmental concerns, the extensive use of N-based fertilizers in agriculture is under microscope. As a result, there has been an increased interest in environmentally friendly, sustainable agricultural methods, including inoculation with microorganisms (bio-fertilizers) to lessen the use of industrial fertilizer and its adverse environmental impacts (Lowe and Baldock, 2000). Rhizobia-associated lentils (Lens culinaris M.) boost the soil’s organic fertility and N economy by fixing atmospheric nitrogen. Lentil can enhance yield by up to 2 t ha-1 and meet more than 85% of their nitrogen requirements through N fixation if infected with the suitable rhizobia (Bisen et al., 1980). Rhizobia association changes the rhizospheric environment to impact other nutrients available to the crop and fix the atmospheric N. Consequently, these bacteria are crucial living creatures in agriculture because they help flow plant nutrients, reducing the need for synthetic fertilizers (Mia and Shamsuddin, 2010).
Among different amendments, biochar is a potentially cost-effective carbon source with many agricultural and environmental applications. Biochar, a pyrolytic product of organic material (Lehmann and Joseph, 2009), is anticipated to hold soil carbon and significantly lower its losses. Application of carbon in the soil in the form of biochar (which contains carbon as nanoparticles in grounded form) (Nepal et al., 2023) or carbon nanoparticles (Nepal et al., 2022) offers the potential to improve soil fertility and quality. Each tonne of soil-applied biochar may absorb 1.4–2.9 tonnes of CO2 and has several beneficial effects on soil characteristics and plant nutrition (Glaser et al., 2002; Kulyk, 2012). When used as a soil amendment, biochar improves the soil’s physical and biochemical properties, which in turn increases soil fertility and productivity through increasing soil aggregation, water retention, pH, and microbial activities, thus, improving overall soil quality, potentially helping to reduce chemical fertilizer needs over time (Bhattarai et al., 2015; Nepal et al., 2023). In addition to increasing soil fertility, its ability to store carbon and nitrogen may lessen both immediate and long-term environmental deterioration and its harmful impacts on human and animal health (Verheijen et al., 2010). Biochar is an excellent adsorbent for nutrients and pollutants because of the various functional groups connected with it on its surface (Bilias et al., 2021). As a result, biochar improves the soil’s ability to absorb nutrients and agricultural chemicals while lowering their vaporization and leaching into the surface and groundwater (Nepal et al., 2023). Through weathering and decomposition, biochar may add many crucial nutrients to the soil. Biochar serves to lessen the bulk density of the soil, improves drainage, aeration, and root penetration, as well as the soil’s ability to retain water and workability, thanks to its low density and up to 70% porous structure (Downie et al., 2009). According to Glaser et al. (2002), biochar is a product with a long-lasting impact on soil quality and carbon sequestration due to its greater C half-life (>1,000 years).
Due to its potential for reducing nutrient losses (Downie et al., 2009; Singh et al., 2010) and improving the fertilizer-use efficiency (Widowati et al., 2014), biochar has been embraced under research efforts for crop-nutrient management (Lehmann and Joseph, 2015). Its integration into poor soil enhances soil fertility, crop development, and productivity more than non-biochar soil (Steiner et al., 2007; Major et al., 2010; Zeeshan et al., 2020). However, all of these factors rely on the origin and characteristics of the applied biochar, soil characteristics, environmental plant responses, biochar-soil interactions, and its ability to absorb nutrients (Enders and Lehmann, 2012). It may have an impact on crop development both directly and indirectly depending on its natural nutritional content and capability for nutrient and water retention as well as the reduction of moisture stress (Lehmann et al., 2003; Karhu et al., 2011; Vaccari et al., 2011; Baidoo et al., 2016). In some cases, it is possible to anticipate poor crop performance for the first 30 days following cultivation on acidic soil (pH = 5.5), which may be caused by short-term N retention in N-deficient soil (Clough et al., 2013). According to Widowati et al. (2014), rice husk biochar caused significant N leaching, whereas wood biochar had the maximum water retention and minimized NO3−1 leaching within the first 30 days following application. Jones et al. (2011) claim that biochar alters soil physical characteristics like bulk density, OM breakdown, and the organic carbon it liberates, suggesting a potential method for C sequestration. It effectively delivers nutrients into the soil while reducing nutrient leaching (Biederman and Harpole, 2012). After applying a biochar amendment to the soil, Laird et al. (2010) found a greater content of extractable nutrients, while Ali et al. (2015) reported an increase in soil fertility. Zeeshan et al. (2020) found that compared to the biochar control and the larger particle size fraction, the biochar amendment with small and medium particle size considerably decreased the bulk density of the soil and enhanced overall porosity, pH, and electrical conductivity. The tiny particle size fraction also greatly enhanced the soil’s organic matter (OM) content and saturation.
The biochar’s ability for adsorption depends on its surface area (Uchimiya et al., 2010). When put into the soil, high surface area biochar with a high variable charge component will have a greater surface sorption capacity, affecting the soil’s ability to retain water, nutrients, and organic molecules (Brown et al., 2006). The surface oxygenation of biochar, when it is applied to soil, changes the O2-containing functional groups (carboxyl, OH, phenol, and carbonyl groups) on the vast interior surface area of the biochar, inducing a negative charge and raising the soil pH and cation exchange capacity (CEC) (Cheng et al., 2006; Lee et al., 2010). According to Chintala et al. (2014), biochar-related cation release into the soil reduces soil acidity and may significantly alter the soil’s N (NH4+ and NO3−) and AB-DTPA extractable P and K contents (Zeeshan et al., 2020).
Due to its chemistry and properties, biochar can increase agricultural production, especially in soils with low fertility and soil degradation, where it can be especially beneficial to the world’s poorest farmers. Biochar from biomass waste has been linked to better soil water-holding capacity and a decreased run-off of agricultural pesticides and fertilizers (Woolf et al., 2010). It has improved crop yield by stimulating beneficial soil microbes like mycorrhizal fungi (Warnock et al., 2007), increasing soil base saturation (Glaser et al., 2002; Major et al., 2010), increasing soil water holding capacity (Glaser et al., 2002), and retaining nutrients in the portion of the soil column containing roots and enhancing nutrient use efficiency (Chan et al., 2007; Steiner et al., 2008).
Biochar-treated soil may provide a better environment for beneficial soil bacteria to flourish and perform (Shenbagavalli and Mahimairaja, 2012). Inoculating lentils with rhizobia improved soil OM, micronutrient fertility (Fe, Zn, Mn, and Cu), and soil qualities (Ali et al., 2019). These advantages could change depending on the feedstock and pyrolysis temperature (Yao et al., 2012). The biochar particle size fraction may, however, significantly influence soil properties. The influence of biochar particle size on changes in N and C mineralization and CO2 evolution in a cereal-legume rotation was assessed in the current study. In a silt-loam (fine loamy mixed thermotypic haplustalf) soil, we expected that lowering wood biochar particle size would enhance its advantages in terms of growth, N and C mineralization. The results of our study will broaden and improve farmers’ options for combining appropriate particle size of biochar with fitting rhizobia strains to increase N and C economy, thereby reducing CO2 evolution from agricultural areas.
2 Materials and methods
2.1 Biochar production and soil sampling
Acacia tree branch clippings (3 inches long) were pyrolyzed at 450°C for 48 h in a stainless-steel kiln made locally by the Institute of Mechatronics, the University of Engineering and Technology, Peshawar, Pakistan (Arif et al., 2017). Through crushing and sieving, the final product was divided into fractions of 2 mm, 2–5 mm, and 5–10 mm particle size and was characterized as reported in Table 1.
Soil samples were collected from the experimental site located at the Research Farms of Amir Muhammad Khan (AMK) Campus Mardan, Pakistan (34o,15′,97″N and 72o,.05′,88″E). The soil was silt loam in texture with a bulk density of 1.3 g cm-3, classified as fine loamy, mixed, thermic, typic hapludalfs. The soil’s native moisture level and saturation water content were very low (4% and 8.3%, respectively). The soil was non-saline, alkaline in reaction, low in total N (0.01%) and mineral N (10.3 mg kg-1), low in organic carbon (0.32%), high in C:N ratio (32:1) and moderate in Mehlich 3 extractable K, P, Fe, Cu, Zn, and Mn (81, 5.6, 4.9, 2.5, 1.45, and 3.52 mg kg-1, respectively). Further elaboration of extraction procedure have been reported previously in Ali et al. (2022).
2.2 Experimental design
The study comprised two crops grown in the winter and summer of the same year. The experiment started in November 2019 by growing lentil and using a randomized complete block split-plot design (RCBD split plot) replicated three times. The treatments included two factor; biochar particle size (control, 2 mm, 2–5 mm, and 5–10 mm) applied to main plots whereas second factor, rhizobia inoculum products (Bizote-N and Rhizogold) were arranged in subplot. Biochar was applied at the rate of 1% on soil mass basis and the seeds of lentil were treated with respective biofertilizers at a rate of 0.5%. The recommended basal doses of N (30 kg ha-1) and P2O5 (60 kg ha-1) for the lentil crop were added to all experimental units (4 m * 3 m) uniformly. During field preparation for lentil, soil in the prescribed sub-plots was added and well mixed with biochar particle size fractions (2 mm, 2–5 mm, and 5–10 mm) while keeping one biochar control. Two rhizobia inoculum products, Biozote-N and Rhizogold, were procured from the National Agricultural Research Center (NARC), Islamabad, and the University of Agriculture, Faisalabad (Pakistan), respectively, and used to inoculate lentil seeds before being compared to one biofertilizer control in main plots. The lentil crop was planted on 5 November 2019, and harvested on 5 May 2020 (data not presented in this paper). At the time of lentil harvest, soil samples from all treatment plots were taken at depths ranging from 0 to 30 cm to analyze the soil’s microbial biomass, C and N mineralization, and CO2 evolution. The same experimental setup was used for the subsequent maize crop grown in the summer season, however the soil was supplemented with N, P2O5, and K2O (120, 90, and 60 kg ha-1), respectively, based on maize crop fertilizer recommendations. On 20 June 2020, maize was planted and harvested on 15 October 2020 (data not shown in this paper). To evaluate the residual impact of different biochar particle sizes applied and rhizobia biofertilizer products inoculated to the previous lentil crop on soil microbial biomass C and N, C and N mineralization and CO2 evolution, soil samples from all treatment plots were taken at a depth of 0–30 cm.
2.3 Determination of C, N, and soil microbial biomass
To determine biomass C and N, chloroform fumigation and subsequent incubation techniques were utilized (Brookes et al., 1985; Vance et al., 1987; Horwath and Paul, 1994). Chloroform fumigation was used to kill the microorganisms in 20 g of fresh soil. After that, the soil was inoculated with 1 g of the same fresh soil that had not been fumigated, and it was then incubated for 3, 6, and 10 days intervals with 0.3M NaOH in vials suspended in incubated flasks for CO2 trapping. The same procedure was repeated, simultaneously, for unfumigated soil. The solution was titrated against 0.1N HCl until the pink hue faded after adding 10 mL of 1M BaCl2 solution and 4–5 drops of phenolphthalein. On day 10, after recording the CO2 evolution, total mineral N was examined in both the fumigated and unfumigated samples. Fc = Fumigated sample CO2 and Ufc = Un-fumigated sample CO2 were used to compute the microbial biomass C (Fc-Ufc)/Kc and biomass-N (Fn-Ufn)/Kn (Jenkinson, 1981), where Fn stands for mineral-N from fumigated soil, Ufn for mineral-N from non-fumigated soil, and Kn for 0.54 (Jenkinson, 1988).
For the computation of the quantity of mineralizable C based on 44 g of CO2 containing 12 g of C, the whole amount of CO2 from an unfumigated sample over 10 days of incubation was collected. Before and following the incubation (day 0 and day 10) using the method described above, mineral N in samples was estimated wherein difference at days 0 and 10 represents mineralizable N.
2.4 Statistical analysis
All the data were submitted to an analysis of variance (ANOVA) using the statisticsal software STATISTIX 8.1. The Least Significant Difference (LSD) test was used to determine if the treatment means for each parameter were significantly different (Steel and Torrie, 1980). The MS Excel program was used to create graphs showing the interaction data between biochar particle size and biofertilizers, while the R software (version 3.43.) was used to create the Principal Component Analysis (PCA) graphs and correlation matrix. All the statistical significance level was maintained at p < 0.05, unless otherwise specified.
3 Results
3.1 Microbial biomass carbon
As measured at 3, 6, and 10 days of incubation intervals, microbial biomass carbon (MBC, µg g-1 soil day-1) was substantially (p < 0.05) greater in soil treated with biochar than in soil with no biochar (control). At day 3, MBC with biochar increased 126%, 108%, and 72% over the no biochar treatment. On day 6, the rise was 50%, 24%, and 40%; on day 10, it increased by 29%, 26%, and 25% (Table 2). The order of increased MBC with respect to incubation time was day 3> day 6> day 10, and the efficiency of biochar particle size to raise MBC was; 2 mm > 2–5 mm > 5–10 mm. On days 3 and 6, the microbial biomass C in the treatment with less than 2 mm of biochar was 20% and 7% greater than in the treatment with 2-5 and 5–10 mm of biochar, respectively. On day 10, biochar sizes did not appear to have any impact on MBC; however, when biochar sizes of less than 2 mm were used, MBC increased by 2% and 3% in comparison to treatments using biochar sizes of 2–5, 5–10 mm, respectively.
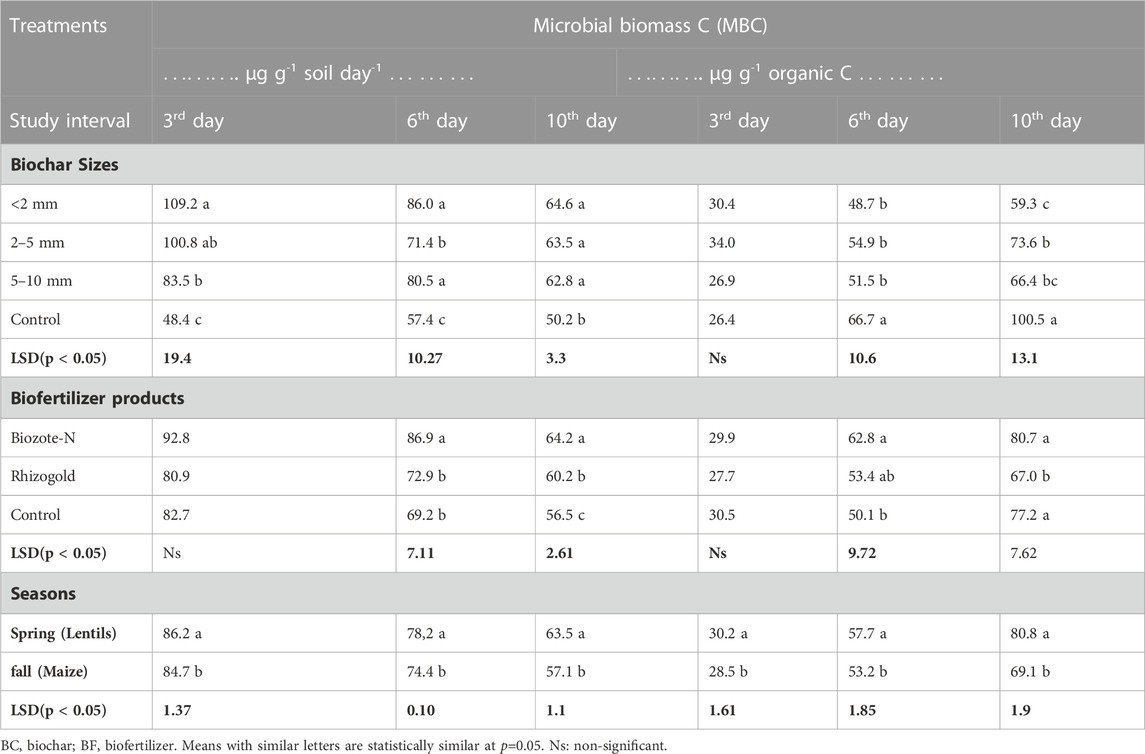
TABLE 2. Soil microbial biomass C (MBC) at different intervals, biochar particle size, and biofertilizers
On day 3, there was little difference in microbial biomass C (µg g-1 organic carbon (OC)) between the treatments for biochar size (Table 2); however, compared to the biochar control, MBC (µg g-1 OC) was 37%, 24%, and 31% lower on day 6 with <2 mm, 2–5 mm, and 5–10 mm particle size of biochar and on day 10, the respective reduction was 69%, 45%, and 58%. Compared to Rhizogold, Biozote-N observed MBC values were 19% higher for µg g-1 soil day-1 and 18% higher for µg g-1 OC on day 6, as well as a 25% increase in each case over the biofertilizer control value (Table 2). On day 10, the MBC in the Biozote-N treatment concerning the units mentioned above increased by 6% and 20% over the Rhizogold treatment and by 13% and 5% over the biofertilizer control, respectively. When examined for 3, 6, and 10 days of incubation, MBC (µg g-1 soil day-1) was 18%, 5%, and 11% greater after lentil than the next maize crop, and MBC (µg g-1 OC) was 6%, 8%, and 17% higher (Table 2). In the interaction (Figure 1), Rhizogold recorded increased MBC (µg g-1 OC) at the smaller particle size fraction at day 3 only, but the Biozote-N considerably enhanced the MBC (µg g-1 soil day-1 and µg g-1 OC) both at days 3 and 10.
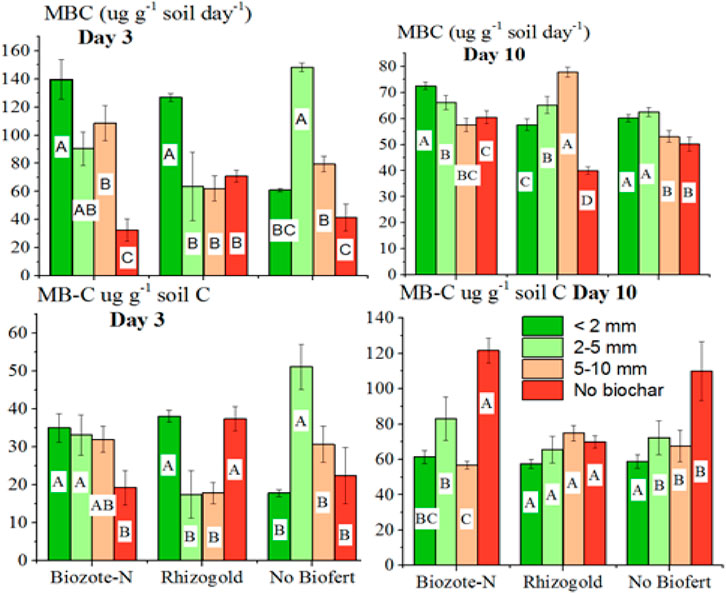
FIGURE 1. Microbial biomass C (µg g-1 soil day-1 and µg g-1 soil OC) at day 3 and 10 as a result of the interaction between biochar particle size and biofertilizers.
3.2 Carbon dioxide evolution
The varying particle size fraction of biochar had a substantial (p < 0.05) impact on the CO2 evolution measured at various time intervals (3, 6, and 10 days) (Table 3). At day 3, biochar size of <2 mm showed significantly low CO2 evolution (by 25%) compared to the no biochar treatment. However, 2–5 mm and 5–10 mm biochar sizes were statistically similar to the no biochar treatment. On day 6, the CO2 evolution with the smallest particle size was substantially greater (by 19%) than the evolution with 5–10 mm biochar size, and it was 9% and 4% higher than the evolution with 2–5 mm biochar size and no biochar, respectively. Similar to day 6, CO2 evolution with the biochar size of <2 mm at day 10 was substantially greater (by 13% and 7%, respectively) than that with 2–5 mm particle size and no biochar. At the beginning (day 3), no-biofertilizer considerably outproduced CO2 production in Biozote-N and Rhizogold plots (14% and 23%, respectively). At day 6, midway through incubation, Rhizogold had a 5% greater CO2 evolution than the biofertilizer control, whereas Biozote-N had a 21% lower evolution. Biozote and Rhizogold had considerably greater CO2 evolution at day 10 (67% and 45%, respectively) than the biofertilizer control.
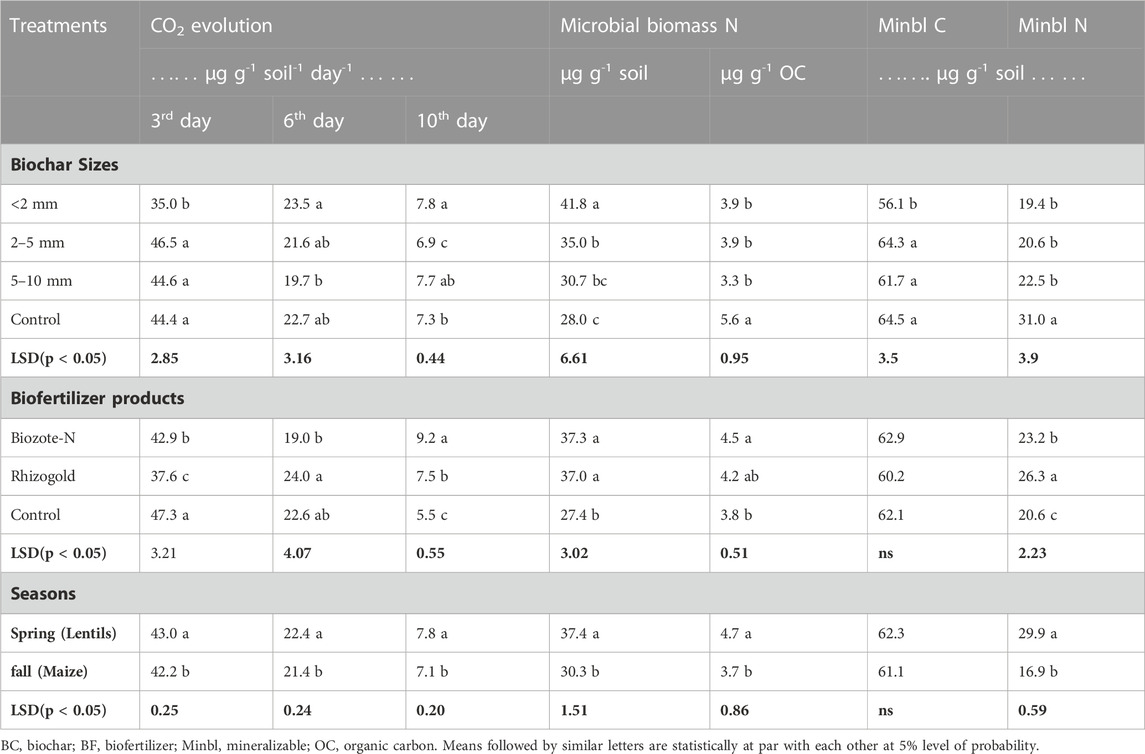
TABLE 3. CO2 evolution, mineralizable C and microbial biomass N (MBN) under different biochar size and biofertilizer treatments.
At all three incubation intervals (day 3, day 6, and day 10), CO2 evolution was strongly influenced by the interaction between biochar size and bio-fertilizer (Figure 2). At the three and 6-day incubations, Biozote and Rhizogold were either at par with or below the bio-fertilizer control; however, at the tenth-day of incubation, Biozote-N was at par with Rhizogold at <2 mm while both significantly higher than the bio-fertilizer control. At the 2-5 and 5–10 mm particles, Biozote was higher in CO2 evolution than Rhizogold and the biofertilizer control. At day 3, day 6, and day 10 of incubation, CO2 evolution was substantially greater in spring after the lentil crop than in fall after the maize crop (by 2, 5, and 10%, respectively).
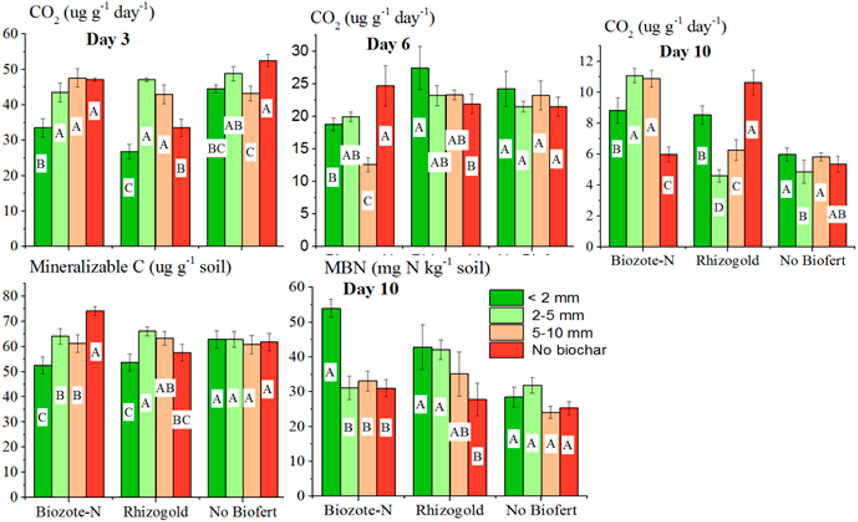
FIGURE 2. CO2 production, microbial biomass N and mineralizable N as a result of interaction between biochar particle size and biofertilizers.
3.3 Microbial biomass nitrogen
Compared to the Microbial biomass N (MBN) in the 2–5 mm, 5–10 mm biochar size treatments and biochar control, the MBN in the <2 mm biochar size treatment was significantly (p < 0.05) higher (by 19%, 36%, and 49%, respectively). While the 5–10 mm biochar particle size induced rise in MBN remained statistically identical with the biochar control, the 2–5 mm biochar particle size treatment also showed a significant edge of a 10% increase in MBN over the biochar control (Table 3). However, MBN (µg g-1 OC) in biochar treatments was significantly (p < 0.05) lower in <2 mm, 2–5 mm (by 43% each), and 5–10 mm (by 70%) than the no biochar plot accounting for the majority of the OC added by biochar and having a more wide-ranging C:N ratio (Table 1). All biochar particle size treatments had identical statistical results for MBN (µg g-1 OC). While both biofertilizers were statistically similar, MBN in the biofertilizer treatments (Biozote N and Rhizogold) was considerably (p < 0.05) greater (by 37% and 36%, respectively) than MBN in control (no biofertilizer) treatment (Table 3). The amount of MBN (µg g-1 OC) in the Biozote-N was significantly higher (by 18%) than that of the no-biofertilizer and registered a non-significant increase over that of the Rhizogold. Furthermore, the no-biofertilizer plot and Rhizogold were statistically comparable in MBN (µg g-1 OC).
Seasons with various crops in the cropping pattern differed noticeably in MBN (µg g-1 soil and µg g-1 OC). A lentil crop in the Spring resulted in 23% higher MBN (µg g-1 soil and µg g-1 OC) measurements than a maize crop in the fall. According to a significant interaction, Biozote-N treatment at the <2 mm size biochar was significantly (p < 0.05) higher in MBN (µg g-1 soil) than the Rhizogold and biofertilizer control at either of the biochar sizes and no biochar plot. Rhizogold’s performance at particle sizes of <2 mm and 2–5 mm was comparable to and much better (p < 0.05) than at particle sizes of 5–10 mm biochar and without biochar.
3.4 Mineralizable carbon
Mineralizable C (µg g-1 soil) at the <2 mm biochar particle size was significantly (p < 0.05) lower (by 15, 10, and 15%) than mineralizable C in 2–5, 5–10 mm size biochar and no biochar treatments, respectively (Table 3). There was no appreciable difference in mineralizable C (µg g-1 soil) between the biofertilizer and no-biofertilizer treatments. Additionally, despite 2% greater mineralizable C (µg g-1 soil) after legumes harvest in the Spring than maize in the fall, mineralizable C remained statistically constant between crop seasons. Mineralizable C was significantly impacted by the interactions between biochar particle size treatments and biofertilizers (Figure 2). Both biofertilizer treatments applied with the smallest size (<2 mm) biochar size had lower mineralizable C than the no-biofertilizer treatment applied with the same size biochar. However, compared to Rhizogold and no-biofertilizer, Biozote had considerably (p < 0.05) greater levels of mineralizable C in the no-biochar treatment. The effects of both biofertilizer products and no biofertilizer for the mineralizable C content of the soil were statistically comparable for the other sizes of biochar additions (2–5 mm and 5–10 mm).
All biochar size (<2 mm, 2–5 mm, and 5–10 mm) treatments’ mineralizable N values were statistically equivalent but significantly (p < 0.05; by 60%, 50%, and 38%) lower than the control values, respectively (Table 3). When compared to the no biofertilizer treatment, mineralizable N increased significantly (p < 0.05) in both Biozote-N and Rhizogold by 13% and 28%, respectively (Table 3). Rhizogold, however, was also significantly (p < 0.05) higher than Biozote-N (by 14%). The results also revealed significant (p < 0.05) seasonal differences (Table 3), with the mineralizable nitrogen content of the soil in the Spring after legume harvest being 77% greater than that of the soil in the fall when maize was grown there.
3.5 Principal component analysis
PCA loading plots were prepared to measure the effects of biochar particle size and bio-fertilizer application on Soil microbial biomass C (MBC), CO2 evolution (C evolution), mineralizable C (Minzbl C), and microbial biomass N (MBN) and microbial biomass N soil organic carbon (MBNOC) (Figure 1, Figure 2). The responses of the different parameters influenced by different biochar sizes and biofertilizer treatments were visualized as PC1 (principal component 1) and PC2 (principal component 2). Figure 3 revealed that PC1 and PC2 explained 64.1% and 22.6% of the total variance. The relation among the above mentioned parameters were further validated using a correlation matrix (Fig: 5) for the spring (a:post-lentil harvest) and fall (b:post-maize harvest) seasons. The PCA results indicated a clear separation among the studied traits under biochar size and biofertilizer treatments. PC1 was positively correlated with mineralizable C and MBCOC and MBNOC under smaller biochar particles with no biofertilizer application, however, MBC and MBN were positively correlated under smaller size of biochar with Rhizogold. Carbon mineralization was negatively associated with MBN, C evolution and MBC. The Minerlized carbon was greater in large particle size with no biofertlization. PC1 was positively correlated with mineralizable C and MBCOC and MBNOC under smaller biochar particles with no biofertilizer application; however, MBC and MBN were positively correlated under smaller size of biochar with Rhizogold. Moreover, PC2 was positively correlated with CO2 evolution under large particles combined with Rhizogold. Figure 4. Represent biplot for 2nd season revealed that PC1 was positively correlated with MBN and MBC under smaller biochar particles with Rhizogold whereas it was negatively correlated under PC2 under large particle size with no biofertilizer application. PC1 was positively correlated with MBN and MBC under smaller particles of biochar with Rhizogold whereas PC2 was negatively correlated with C evolution, minzblC under large particle size with no biofertilizer application. Moreover, C evolution was negatively associated with MBCOC and MBNOC. It was also noted that MBC and MBN were closely associated and were substantially influenced by smaller particle sizes with Biozote.
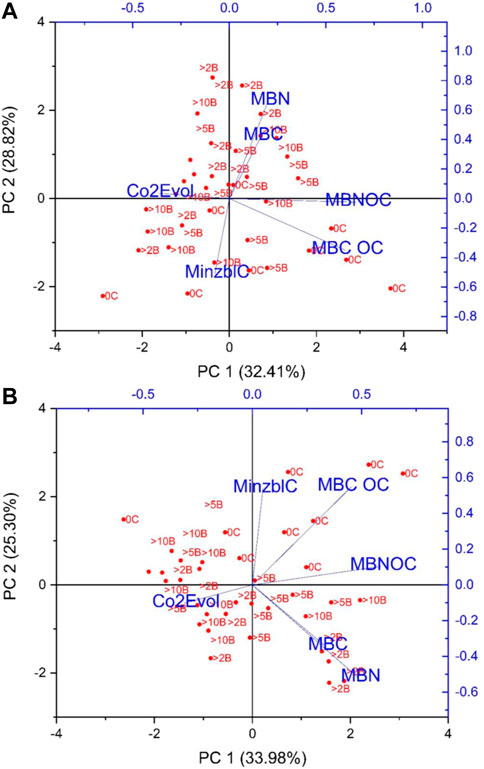
FIGURE 3. Bi-plot (PCA) of (A) first season (B) second season presenting the correlation between various traits including Soil microbial biomass C (MBC) CO2 evolution (C evolution), mineralizable C (Minzbl C) and microbial biomass N (MBN) and microbial biomass N soil organic arbon (MBNOC) under different biochar size and biofertilizer treatments. >2B represent >2 mm Bichar particle plus Biozote-N, >5B = 2–5 mm Bichar particle plus Biozote-N, >>10B = 5–10 mm Bichar particle plus Biozote-N, R represent = Rhizogold and C = control (no biofertilizer).
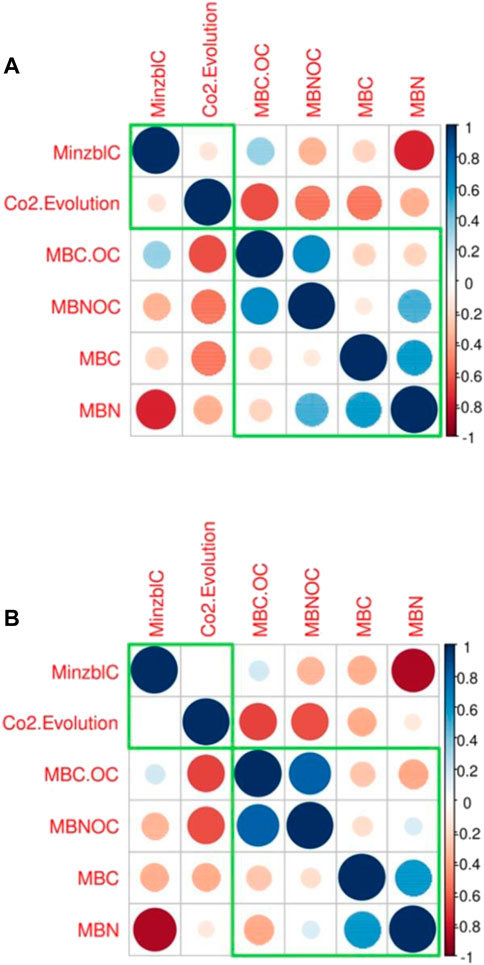
FIGURE 4. Correlation among different variables in (A) first season and (B) second season where; soil microbial biomass C (MBC) CO2 evolution (C evolution), mineralizable C (Minzbl C) and microbial biomass N (MBN) and microbial biomass N soil organic carbon (MBNOC).
4 Discussion
Significantly higher microbial biomass carbon (MBC, g g-1 soil day-1) in the small size (<2 mm) biochar treatment appears to be due to the lowest size fraction having the largest surface area and absorbing most of the nutrients, resulting in increased microbial activity and higher microbial biomass carbon (Ahmad et al., 2014). Similarly, early incubation (day 3) appears to have higher nutritional content than later incubation intervals, resulting in higher MBC. Ascough et al. (2010) noticed a significant increase in microbial population after applying biochar to the soil. Since nutrients are a source of energy for microorganisms (Goyal and Welch, 2006), a decrease in MBC as incubation progresses may be due to nutrient depletion. This demonstrated a greater potential for tiny biochar sizes to sustain MBC for longer than its uses for larger particle-size biochar.
In the present study, lower microbial biomass C (µg g-1 OC) within the <2 mm size biochar is anticipated due to its more consistent mixing with soil matrix and providing more organic carbon to the soil, resulting in an MBC that is lower per Gram of OC in comparison to larger biochar particles. Also, it was noted that as the difference in the MBC (µg g-1 OC) between the different particle size treatments grew, so did the difference in the incubation time. On day 3, the difference was not substantial, however, on days 6 and 10, the <2 mm biochar size had the most significant decrease in MBC (µg g-1 OC) over the no biochar plot, followed by the 2–5 mm and 5–10 mm biochar sizes. The nutrients, energy sources for bacteria (Goyal and Welch, 2006), would be less available for microbial growth over time in the free-soil solution in the <2 mm biochar treatment because they would stick to more exposedsurfaces than on the bigger particles or in the control. This data showing a decrease in MBC (µg g-1 OC) revealed that the microbial community has less effect on the turnover of the biochar C after the easily degradable free C has been used up. This is more noticeable later in the incubation process than at the beginning. Even biochar has been shown to reduce FYM carbon mineralization and CO2 release (Vasu, 2015), which is very similar to what we found. Our results also showed that the ability of biochar to keep soil C safe increased as the particle size fraction decreased which showed the biggest difference between the MBC (µg g-1 OC) and the plot with no biochar (Ali et al., 2022).
The ability of microorganisms to recycle plant nutrients is crucial. In this study, only the Bizote-N showed a significant difference in MBC (µg g-1 soil day-1 and µg g-1 OC) on days 6 and 10. Biozote-N appeared to have adequate activity through atmospheric N fixation and increased substrate for greater microbial growth than Rhizogold and the biofertilizer control. On day 10, Rhizogold exceeded the biofertilizer control in MBC (µg g-1 soil day-1) by 7%, indicating that its onset of N fixation is delayed in relation to the production of N fixation substrates in Biozote-N plots for increased microbial growth. This is supported by Billah et al. (2019), who demonstrated statistically comparable grain and straw nitrogen levels in lentils infected with Rhizogold and Biozote-N, respectively. Rhizogold’s significantly lower MBC µg g-1 OC (by 19%) over the biofertilizer control is due to the higher OC in the Rhizogold treatment compared to the biofertilizer control. It is possible that the increased MBC in Rhizogold-treated plots is not proportional to the increased OC. According to Ahmad et al. (2014), MBC values were higher 10 days after legume exposure. Greater microbial biomass carbon (MBC) after lentil than after maize confirms leguminous N-rich residues and a greater contribution to soil organic matter than cereals. In cropping patterns, legumes have a significantly higher concentration of microbial biomass C (Ahmad et al., 2014). In the present study, increased MBC in both Biozote-N and Rhizogold at the smallest biochar particle size fraction is indicative of its uniform distribution in soil and improved soil physical environment (Ahmad et al., 2014) for favorable microbial inoculation performance and growth are reasons why the smaller biochar particle size is essential for enhancing microbial properties.
At the initial incubation, CO2 evolution with <2 mm biochar size was significantly lower, however, at the mid and final incubation, the CO2 evolution with the smallest particle size was substantially greater (by 19%) than larger biochar size and no biochar. The soil exchanges its microorganisms with biochar once it is put into the soil since the intrinsic microbial community of biochar is often relatively low (Jones et al., 2011). Thus, the organic matter in native soil is negatively primed by biochar (Jones et al., 2011). Since soil matrix, including native organic matter, is anticipated to mix well with <2 mm particles, a more uniform negative priming impact is expected. As a result, the initial rate of CO2 evolution (day 3) was lowered due to poor intrinsic microbial population and a negative priming impact of <2 mm size on soil organic matter. Smaller particles blend well with soil, creating intra-aggregates and particle bridges that increase porosity (Keech et al., 2005) and lower bulk density (Billah et al., 2019). This results in a better soil environment, which increases the aeration of native soil OM and increases CO2 evolution. Since, it would probably take at least 3–6 days to complete, more CO2 liberation was seen on days 6 and 10 rather than at initial interval of day 3. The lower CO2 evolution in our study (Table 3) is consistent with the findings of Jones et al. (2011), who found that a significant portion of the CO2 release from biochar-amended soil comes from a dissolved inorganic portion of the biochar and that the release is, therefore, more abiotically mediated and not related to the extent of microbial activity. This is true even though day 3 (Table 2) showed higher microbial activity. The higher CO2 release at the middle (day 6) and later (day 10) incubation stages (Table 3) seem to be more biotically mediated than abiotic, according to (Jones et al., 2011), who noted that depending on the type of feedstock and temperature of pyrolysis, dissolved organic carbon is approximately equal to dissolved inorganic carbon and their enhancement in CO2 flux is also approximately equal.
At the beginning (day 3), higher CO2 production in biofertilizer control (Table 3) shows the breakdown of native soil organic matter. Compared to Rhizogold and the Control, the soil organic matter level in the Biozote-N plot treated with the previous lentil crop was considerably greater (Ali et al., 2019). The fresh addition of soil organic matter (OM) from legume crops also negatively primes the soil organic matter. Here again, higher microbial activity in the Biozote-N plot than in Rhizogold and biofertilizer control (Table 2) did not increase CO2, indicating that most initial CO2 release is abiotic. Midway through incubation, greater CO2 evolution within Rhizogold and within the Biozote-N and Rhizogold at day 10 (Table 3) are consistent with Table 2’s findings that biofertilizer plots had greater levels of microbial activity than biofertilizer controls seemingly the CO2 release at this stage was biotically driven. Treatments with biofertilizers could need some time before organic matter breaks down. The prolonged availability of organic carbon for decomposition in bio-fertilizer inoculated plots, particularly Biozote-N, is potentially the cause of the extended CO2 evolution duration, whereas, in the no-biofertilizer plot, the same has been maximally exhausted by mid-incubation stage.
The CO2 evolution within Biozote and Rhizogold amended to <2 mm biochar size plots were either at par with or below the bio-fertilizer control at the initial and mid-incubation but outperformed it at the final incubation; however, Biozote was significantly higher than both Rhizogold and the biofertilizer control. Since lentils commonly lose their leaves and leave a thin coating of the letter on the soil surface, this may be due to greater soil organic matter content following lentils than cereal crops (Ahmad et al., 2014). Due to the increased N content of lentil organic matter compared to cereal organic matter (Giller, 2001), which better stimulates microbial activity (Table 2), spring had more CO2 evolution than the fall maize crop (Table 3).
Enhanced microbial biomass N (MBN) inside the <2 mm biochar size followed by the 2–5 mm biochar particle size treatment (Table 3) indicated that biochar had sequestered soil nitrogen by allowing it to be consumed by microorganisms and converted to MBN. According to Table 2, the smallest biochar size had the highest MBC (µg g-1 soil), which denotes a greater microbial population. Therefore, the greatest amount of microbial protein may have been present, which would account for the treatment’s significantly greater MBN. Significantly (p < 0.05) lower MBN (µg g-1 OC) within biochar treatments accounting for most of the OC added by biochar and having a wider C:N ratio. Therefore, it is important to note that biochar may affect nutrient cycles and organo-chemical properties in addition to changing the physical properties of the soil (Billah et al., 2019) and increasing soil microbial biomass (Table 2) (Jin, 2010; Liang et al., 2010; Nguyen et al., 2010; Lehmann et al., 2011).
Biofertilizers are organic products that include live cells of various microorganism types that may biologically change inaccessible forms of nutritionally important nutrients into available forms (Vessey, 2003). An increase in MBN (µg g-1 soil and µg g-1 OC) with biofertilizer inoculation is a testimonial of the N fixation compared to no biofertilizer plot because microorganisms being significant features in agriculture to improve the circulation of plant nutrients and minimize the demand for chemical fertilizers (Pouryousef et al., 2007). The higher MBN (µg g-1 OC) in the Biozote-N indicated the highest potential for N fixation by the Biozote-N and consuming it in their body proteins. Biozote-N provides similar support with a higher MBC than Rhizogold (Table 3). The biofertilizer significantly aided the availability of nitrogen for the crop and the provision of a favorable environment for plant growth. Additionally, nutrient digestion inside microbial biomass improves the nutrients’ retention and recycling. In addition, as a micro-organism dies and decays, it becomes an energy source for more species in the soil food web (Miller et al., 2009).
Season having lentil was significantly higher in MBN than season having maize crop. As was previously indicated, grain crops give less organic matter to soil than legumes. Thus, increased microbial activity and increased N availability from N-rich organic matter may be used to explain greater MBN following legumes. Following the maize harvest in the fall season, soil N-rich organic matter may have significantly decreased compared to the legume harvest in the spring (Ahmad et al., 2014), followed by lower MBN concentration in the soil in the fall than Spring season. Globally, the concentration of CO2 exhaled by legume roots may be comparable to or even greater than CO2 produced during N-fertilizer production. However, the CO2 evolved from the legume root system via photosynthesis, hence, the CO2 concentration that was not recaptured by the plant and eventually escaped from the legume canopy would be C neutral. In contrast, all the CO2 released during the synthesis of fertilizer N is derived from fossil fuels and represents a net contribution to CO2 levels in the atmosphere. In interaction, Biozote-N’s higher potential for N fixation and a more favorable soil environment at the <2 mm size biochar might have resulted in higher MBN (µg g-1 soil) followed by Rhizogold’s performance at the <2 mm and 2–5 mm biochar size than without biochar.
Reduced mineralizable C with the smaller size of biochar alone and in interaction with biofertilizer products could be because of the enhanced microbial population due to the inoculation of biofertilizer products and increased C immobilization by them at the smaller size of biochar because of the improved soil environment. All biochar size fractions had much lower mineralizable N values than the control values. This could be because of biochar’s wide C:N ratio rendering reduced soil mobility and less N availability for mineralization, especially in the short-term experiments (Deenik et al., 2010). Using biochar in the soil is one of the most effective and highly debated aspects to combat climate change and improve soil fertility (Zimmerman et al., 2011). It is claimed that biochar changes how nitrogen (N) moves through soils (Clough and Condron, 2010; Clough et al., 2013). These results demonstrated that biochar application to soil helped keep carbon and nitrogen from escaping the soil. The current study revealed that C and N sequestration is directly linked to the biochar surface area increase when the biochar particle size fraction is decreased. Recent research has shown that adding biochar to mineral soils may directly affect N changes (Castaldi and Aragosa, 2002; Zheng et al., 2013). Mineralizable N increased significantly (p < 0.05) with both the Biozote-N and Rhizogold, and after legumes rather than maize. Microorganisms recycle the plant nutrients, reducing the need for artificial fertilizers (Pouryousef et al., 2007). The results showed that legumes responded to inoculation by increasing the number of micro-organisms and possibly by increasing the rate and composition of exudation (Bashan et al., 2004; Kawasaki et al., 2012) and crop performance (O'Callaghan, 2016), all of which could have led to enhancing soil organic matter. However, Biozote-N was better than Rhizogold at reducing the amount of mineralizable nitrogen. This showed that Biozote-N is better able to fix nitrogen than Rhizogold.
5 Conclusion
Biochar improved microbial biomass C and N concentration (µg g-1 soil) but did not improve their contents g-1 soil OC. The impact of the particle size range of <2 mm was more positive. Because of low MBC (µg g-1 OC) at < 2 mm particle size range, higher CO2 evolution at day 6 and 10 incubation is more likely due to abiotic factors. Similarly, the <2 mm size is better for N sequestration due to its larger MBN and lower mineralizable N. Biozote-N treatment substantially increased MBC and MBN, was low in mineralizable N, and may be advantageous for C sequestration due to its delayed CO2 evolution. All metrics were considerably higher in the Spring after the legume harvest than the maize in the fall, except for mineralizable C. The results showed that for enhanced microbiological characteristics, the grain size of the biochar amendment should be kept below 2 mm. Even after applying Bizote-N and the smallest biochar particle together for a year to a lentil crop, their combined performance was synergistic and beneficial for most of the microbiological parameters of the organic fertility. Further research should focus on nano-char being most effective in a smaller size to evaluate their impact on crop quality, yield, and environment.
Data availability statement
The original contributions presented in the study are included in the article/supplementary material, further inquiries can be directed to the corresponding author.
Author contributions
WA: project conceptualization, resources, writing, editing and review. JN: writing, review and editing, data analysis, validation, ZZ: project administration, supervision, funding acquisition, FM: data analysis, software, review and editing. AK: methodology, review and editing. IA: methodology, review and editing. SZ: review and editing, validation. MK: visualization, methodology, SAJ: review and editing and DT: software, writing and editing and resources. All authors contributed to the article and approved the submitted version.
Conflict of interest
The authors declare that the research was conducted in the absence of any commercial or financial relationships that could be construed as a potential conflict of interest.
Publisher’s note
All claims expressed in this article are solely those of the authors and do not necessarily represent those of their affiliated organizations, or those of the publisher, the editors and the reviewers. Any product that may be evaluated in this article, or claim that may be made by its manufacturer, is not guaranteed or endorsed by the publisher.
References
Ahmad, W., Farmanullah , , Shah, Z., Jamal, M., and Shah, K. A. (2014). Recovery of organic fertility in degraded soil through fertilization and crop rotation. J. Saudi Soc. Agric. Sci. 13, 92–99. doi:10.1016/j.jssas.2013.01.007
Ali, A., Ahmad, W., Munsif, F., Khan, A., Nepal, J., Wójcik-Gront, E., et al. (2022). Residual effect of finely-ground biochar inoculated with bio-fertilization impact on productivity in a lentil–maize cropping system. Agronomy 12 (9), 2036. doi:10.3390/agronomy12092036
Ali, A., Ahmad, W., Zeeshan, M., Khan, F., and Billah, M. M. (2019). Biochar and biofertilizers residual effect on fertility status of soil two crop seasons after their application. Sarhad J. Agric. 35, 727–733. doi:10.17582/journal.sja/2019/35.3.727.733
Ali, K., Arif, M., Ullah, W., Ahmad, W., Rabnawaz Khan, M., Ayeni, L. S., et al. (2015). Influence of organic and inorganic amendments on weeds density and chemical composition. Pak. J. Weed Sci. Res. 21 (1).
Arif, M., Ilyas, M., Riaz, M., Ali, K., Shah, K., Haq, I. U., et al. (2017). Biochar improves phosphorus use efficiency of organic-inorganic fertilizers, maize-wheat productivity and soil quality in a low fertility alkaline soil. Field crops Res. 214, 25–37. doi:10.1016/j.fcr.2017.08.018
Ascough, P. L., Sturrock, C. J., and Bird, M. I. (2010). Investigation of growth responses in saprophytic fungi to charred biomass. Isotopes Environ. health Stud. 46, 64–77. doi:10.1080/10256010903388436
Baidoo, I., Sarpong, D. B., and Bolwig, S. (2016). Biochar amended soils and crop productivity: A critical and meta-analysis of literature. Int. J. Sustain. Dev. 5 (9), 414–432.
Bambara, S. K., and Ndakidemi, P. A. (2010). Changes in selected soil chemical properties in the rhizosphere of Phaseolus vulgaris L. supplied with Rhizobium inoculants, molybdenum and lime. Sci. Res. Essays https://academicjournals.org/journal/SRE/article-full-text-pdf/61C29CB16436.pdf 5, 679–684.
Bashan, Y., Holguin, G., and de-Bashan, L. E. (2004). Azospirillum-plant relationships: Physiological, molecular, agricultural, and environmental advances (1997-2003). Can. J. Microbiol. 50, 521–577. doi:10.1139/w04-035
Bhattarai, B., Neupane, J., and Dhakal, S. P. (2015). Effect of biochar from different origin on physio-chemical properties of soil and yield of garden pea (Pisum sativum L.) at Paklihawa Rupandehi Nepal, World J. Agric. Res. http://article.sciagriculturalresearch.com/pdf/WJAR-3-4-3.pdf 3 (4), 129–138.
Bilias, F., Nikoli, T., Kalderis, D., and Gasparatos, D. (2021). Towards a soil remediation strategy using biochar: Effects on soil chemical properties and bioavailability of potentially toxic elements. Toxics 9 (8), 184. doi:10.3390/toxics9080184
Billah, M. M., Ahmad, W., and Ali, M. (2019). Biochar particle size and Rhizobia strains effect on the uptake and efficiency of nitrogen in lentils. J. Plant Nutr. 42, 1709–1725. doi:10.1080/01904167.2019.1628984
Bisen, C. R., Tomar, S. S., Shivamurthy, R. B., and Kashyap, M. L. (1980). Response of lentil (Lens esculenta) to rhizobium inoculation and fertilization under different moisture regimes. Mysore J. Agric. Sci. https://www.cabdirect.org/cabdirect/abstract/19810723367 14 (4), 483–486.
Brookes, P., Landman, A., Pruden, G., and Jenkinson, D. (1985). Chloroform fumigation and the release of soil nitrogen: A rapid direct extraction method to measure microbial biomass nitrogen in soil. Soil Biol. Biochem. 17, 837–842. doi:10.1016/0038-0717(85)90144-0
Brown, R. A., Kercher, A. K., Nguyen, T. H., Nagle, D. C., and Ball, W. P. (2006). Production and characterization of synthetic wood chars for use as surrogates for natural sorbents. Org. Geochem. 37, 321–333. doi:10.1016/j.orggeochem.2005.10.008
Castaldi, S., and Aragosa, D. (2002). Factors influencing nitrification and denitrification variability in a natural and fire-disturbed Mediterranean shrubland. Biol. Fertil. Soils 36, 418–425. doi:10.1007/s00374-002-0549-2
Chan, K. Y., Van Zwieten, L., Meszaros, I., Downie, A., and Joseph, S. (2007). Agronomic values of greenwaste biochar as a soil amendment. Soil Res. 45 (8), 629–634. doi:10.1071/SR07109
Cheng, C.-H., Lehmann, J., Thies, J. E., Burton, S. D., and Engelhard, M. H. (2006). Oxidation of black carbon by biotic and abiotic processes. Org. Geochem. 37, 1477–1488. doi:10.1016/j.orggeochem.2006.06.022
Chintala, R., Mollinedo, J., Schumacher, T. E., Malo, D. D., and Julson, J. L. (2014). Effect of biochar on chemical properties of acidic soil. Archives Agron. Soil Sci. 60, 393–404. doi:10.1080/03650340.2013.789870
Clough, T. J., Condron, L., Kammann, C., and Müller, C. (2013). A review of biochar and soil nitrogen dynamics. Agronomy 3, 275–293. doi:10.3390/agronomy3020275
Clough, T. J., and Condron, L. M. (2010). Biochar and the nitrogen cycle: Introduction. J. Environ. Qual. 39, 1218–1223. doi:10.2134/jeq2010.0204
Deenik, J. L., McClellan, T., Uehara, G., Antal, M. J., and Campbell, S. (2010). Charcoal volatile matter content influences plant growth and soil nitrogen transformations. Soil Sci. Soc. Am. J. 74, 1259–1270. doi:10.2136/sssaj2009.0115
Downie, A., Crosky, A., and Munroe, P. (2009). “Physical properties of biochar,” in Biochar for environmental management. Editors J. Lehmann, and S. Joseph (London: Earthscan Publications Ltd), 13–32.
Enders, A., and Lehmann, J. (2012). Comparison of wet-digestion and dry-ashing methods for total elemental analysis of biochar. Commun. soil Sci. plant analysis 43, 1042–1052. doi:10.1080/00103624.2012.656167
Glaser, B., Lehmann, J., and Zech, W. (2002). Ameliorating physical and chemical properties of highly weathered soils in the tropics with charcoal–a review. Biol. Fertil. soils 35, 219–230. doi:10.1007/s00374-002-0466-4
Goyal, A., and Welch, I. (2006). A comprehensive look at the empirical performance of equity premium prediction. Rev. Financial Stud. 21, 1455–1508. doi:10.1093/rfs/hhm014
Horwath, W., and Paul, E. (1994). Microbial biomass. Methods Soil Analysis Part 2 Microbiol. Biochem. Prop. 5, 753–773. doi:10.2136/sssabookser5.2.c36
Jenkinson, D. (1988). “Determination of microbial biomass carbon and nitrogen in soil,” in Advances in nitrogen cycling (Southern England: Rothamsted Research), 368–386.
Jenkinson, D. (1981). Microbial biomass in soil: Measurement and turnover. Soil Biochem. https://cir.nii.ac.jp/crid/1571980074289141632 5, 415–471.
Jones, D., Murphy, D., Khalid, M., Ahmad, W., Edwards-Jones, G., and DeLuca, T. (2011). Short-term biochar-induced increase in soil CO2 release is both biotically and abiotically mediated. Soil Biol. Biochem. 43, 1723–1731. doi:10.1016/j.soilbio.2011.04.018
Karhu, K., Mattila, T., Bergström, I., and Regina, K. (2011). Biochar addition to agricultural soil increased CH4 uptake and water holding capacity–Results from a short-term pilot field study. Agric. Ecosyst. Environ. 140, 309–313. doi:10.1016/j.agee.2010.12.005
Kawasaki, A., Watson, E. R., and Kertesz, M. A. (2012). Indirect effects of polycyclic aromatic hydrocarbon contamination on microbial communities in legume and grass rhizospheres. Plant soil 358, 169–182. doi:10.1007/s11104-011-1089-z
Keech, O., Carcaillet, C., and Nilsson, M. C. (2005). Adsorption of allelopathic compounds by wood-derived charcoal: The role of wood porosity. Plant Soil 272, 291–300. doi:10.1007/s11104-004-5485-5
Kulyk, N. (2012). Cost-benefit analysis of the biochar application in the US cereal crop cultivation.
Laird, D. A., Fleming, P., Davis, D. D., Horton, R., Wang, B., and Karlen, D. L. (2010). Impact of biochar amendments on the quality of a typical Midwestern agricultural soil. Geoderma 158, 443–449. doi:10.1016/j.geoderma.2010.05.013
Lee, J. W., Kidder, M., Evans, B. R., Paik, S., Buchanan III, A. C., Garten, C. T., et al. (2010). Characterization of biochars produced from cornstovers for soil amendment. Environ. Sci. Technol. 44, 7970–7974. doi:10.1021/es101337x
Lehmann, J., and Joseph, S. (2015). Biochar for environmental management: An introduction. England, UK: Biochar for environmental management. Routledge, 33–46.
Lehmann, J., Pereira da Silva Jr., J., Steiner, C., Nehls, T., Zech, W., and Glaser, B. (2003). Nutrient availability and leaching in an archaeological anthrosol and a ferralsol of the central amazon basin: Fertilizer, manure and charcoal amendments. Plant soil 249, 343–357. doi:10.1023/a:1022833116184
Lehmann, J., Rillig, M. C., Thies, J., Masiello, C. A., Hockaday, W. C., and Crowley, D. (2011). Biochar effects on soil biota–a review. Soil Biol. Biochem. 43, 1812–1836. doi:10.1016/j.soilbio.2011.04.022
Liang, B., Lehmann, J., Sohi, S. P., Thies, J. E., O’Neill, B., Trujillo, L., et al. (2010). Black carbon affects the cycling of non-black carbon in soil. Org. Geochem. 41, 206–213. doi:10.1016/j.orggeochem.2009.09.007
Lowe, P., and Baldock, D. (2000). Integration of environmental objectives into agricultural policy making, 31–52.
Major, J., Rondon, M., Molina, D., Riha, S. J., and Lehmann, J. (2010). Maize yield and nutrition during 4 years after biochar application to a Colombian savanna oxisol. Plant soil 333, 117–128. doi:10.1007/s11104-010-0327-0
Mia, M. B., and Shamsuddin, Z. (2010). Rhizobium as a crop enhancer and biofertilizer for increased cereal production. Afr. J. Biotechnol. 9, 6001–6009.
Miller, G. T., Spoolman, S. E., Malatesta, K., Yip, L., Marinkovich, A., Hugel, R., et al. (2009). Living in the environment: Concepts, connections, and solutions, 16e. International Student Edition. Belmont: Brooks/Cole, Cengage Learning.
Nepal, J., Ahmad, W., Munsif, F., Khan, A. K., and Zou, Z. (2023). Advances and prospects of biochar in improving soil fertility, biochemical quality, and environmental applications. Front. Environ. Sci. 11, 169. doi:10.3389/fenvs.2023.1114752
Nepal, J., Xin, X., Maltais-Landry, G., Wright, A. L., Stoffella, P. J., Ahmad, W., et al. (2022). Water-dispersible carbon nanomaterials improve lettuce (Latuca sativa) growth and enhance soil biochemical quality at low to medium application rates. Plant Soil 485, 569–587. doi:10.1007/s11104-022-05852-0
Nguyen, B. T., Lehmann, J., Hockaday, W. C., Joseph, S., and Masiello, C. A. (2010). Temperature sensitivity of black carbon decomposition and oxidation. Environ. Sci. Technol. 44, 3324–3331. doi:10.1021/es903016y
O’Callaghan, M. (2016). Microbial inoculation of seed for improved crop performance: Issues and opportunities. Appl. Microbiol. Biotechnol. 100, 5729–5746. doi:10.1007/s00253-016-7590-9
Pouryousef, M., Mohammad, R. C., Mazaheri, D., and Fakhretabatabaii, M. (2007). Effect of different soil fertilizing systems on seed and mucilage yield and seed P content of isabgol (Plantago ovata Forsk), Plant Sci. 6, 1088–1092. doi:10.3923/ajps.2007.1088.1092
Shenbagavalli, S., and Mahimairaja, S. (2012). Production and characterization of biochar from different biological wastes. Int. J. plant, animal Environ. Sci. 2, 197–201.
Singh, B., Singh, B. P., and Cowie, A. L. (2010). Characterisation and evaluation of biochars for their application as a soil amendment. Soil Res. 48 (7), 516–525. doi:10.1071/SR10058
Steel, R. G., and Torrie, J. H. (1980). Principles and procedures of statistics, a biometrical approach. McGraw-Hill Kogakusha: Open-Library.
Steiner, C., Teixeira, W. G., Lehmann, J., Nehls, T., de Macêdo, J. L. V., Blum, W. E. H., et al. (2007). Long term effects of manure, charcoal and mineral fertilization on crop production and fertility on a highly weathered Central Amazonian upland soil. Plant soil 291, 275–290. doi:10.1007/s11104-007-9193-9
Uchimiya, M., Lima, I. M., Thomas Klasson, K., Chang, S., Wartelle, L. H., and Rodgers, J. E. (2010). Immobilization of heavy metal ions (CuII, CdII, NiII, and PbII) by broiler litter-derived biochars in water and soil. J. Agric. Food Chem. 58 (9), 5538–5544. doi:10.1021/jf9044217
Vaccari, F., Baronti, S., Lugato, E., Genesio, L., Castaldi, S., Fornasier, F., et al. (2011). Biochar as a strategy to sequester carbon and increase yield in durum wheat. Eur. J. Agron. 34, 231–238. doi:10.1016/j.eja.2011.01.006
Vance, E. D., Brookes, P., and Jenkinson, D. (1987). An extraction method for measuring soil microbial biomass C. Soil Biol. Biochem. 19, 703–707. doi:10.1016/0038-0717(87)90052-6
Vasu, D. (2015). Effect of biochar addition on soil carbon emission and nitrogen mineralization in some typical Indian soils. Intl J. Emerg. Res. Manage Technol. 4, 17–22.
Verheijen, F., Jeffery, S., Bastos, A. C., Van der Velde, M., and Diafas, I. (2010). “Biochar application to soil: A critical scientific review of effects on soil properties, processes, and functions,” in JRC scientific and technical reports (Italy: Office for the Official Publications of the European Communities), 24099 (162), 2183–2207.
Vessey, J. K. (2003). Plant growth promoting rhizobacteria as biofertilizers. Plant soil 255, 571–586. doi:10.1023/a:1026037216893
Warnock, D. D., Lehmann, J., Kuyper, T. W., and Rillig, M. C. (2007). Mycorrhizal responses to biochar in soil–concepts and mechanisms. Plant soil 300, 9–20. doi:10.1007/s11104-007-9391-5
Widowati, W., Asnah, A., and Utomo, W. H. (2014). The use of biochar to reduce nitrogen and potassium leaching from soil cultivated with maize. J. Degraded Min. Lands Manag. 2 (1), 211. doi:10.15243/jdmlm.2014.021.211
Woolf, D., Amonette, J. E., Street-Perrott, F. A., Lehmann, J., and Joseph, S. (2010). Sustainable biochar to mitigate global climate change. Nat. Commun. 1, 56. doi:10.1038/ncomms1053
Yao, Y., Gao, B., Zhang, M., Inyang, M., and Zimmerman, A. R. (2012). Effect of biochar amendment on sorption and leaching of nitrate, ammonium, and phosphate in a sandy soil. Chemosphere 89 (11), 1467–1471. doi:10.1016/j.chemosphere.2012.06.002
Zeeshan, M., Ahmad, W., Hussain, F., Ahamd, W., Numan, M., Shah, M., et al. (2020). Phytostabalization of the heavy metals in the soil with biochar applications, the impact on chlorophyll, carotene, soil fertility and tomato crop yield. J. Clean. Prod. 255, 120318. doi:10.1016/j.jclepro.2020.120318
Zheng, H., Wang, Z., Deng, X., Herbert, S., and Xing, B. (2013). Impacts of adding biochar on nitrogen retention and bioavailability in agricultural soil. Geoderma 206, 32–39. doi:10.1016/j.geoderma.2013.04.018
Keywords: Biozote-N, biofertilizers, biochar, legumes, organic fertilizer, soil microbiology, soil fertility, soil ecology
Citation: Ahmad W, Nepal J, Zou Z, Munsif F, Khan A, Ahmad I, Zaheer S, Khan MS, Jadoon SA and Tang D (2023) Biochar particle size coupled with biofertilizer enhances soil carbon-nitrogen microbial pools and CO2 sequestration in lentil. Front. Environ. Sci. 11:1114728. doi: 10.3389/fenvs.2023.1114728
Received: 02 December 2022; Accepted: 09 June 2023;
Published: 22 June 2023.
Edited by:
Dionisios Gasparatos, Agricultural University of Athens, GreeceReviewed by:
Farhan Hafeez, COMSATS University Islamabad, PakistanBlaise Pascal Bougnom, University of Yaounde I, Cameroon
Copyright © 2023 Ahmad, Nepal, Zou, Munsif, Khan, Ahmad, Zaheer, Khan, Jadoon and Tang. This is an open-access article distributed under the terms of the Creative Commons Attribution License (CC BY). The use, distribution or reproduction in other forums is permitted, provided the original author(s) and the copyright owner(s) are credited and that the original publication in this journal is cited, in accordance with accepted academic practice. No use, distribution or reproduction is permitted which does not comply with these terms.
*Correspondence: Zhiyou Zou, 410003391@qq.com