- 1Korea Testing & Research Institute, Changwon, Gyeongsangnam-do, Republic of Korea
- 2Department of Ocean Environmental Sciences, Chungnam National University, Daejeon, Republic of Korea
- 3Korea Marine Equipment Research Institute, Busan, Gyeongsangnam-do, Republic of Korea
Introduction: Disinfection byproducts (DBPs) generated during ballast water treatment using active substances have gained increasing interest owing to their potential impact on the marine environment. This study aimed to investigate the occurrence and distribution of DBPs, which are secondary products generated during electrolysis, using land-based tests.
Methods: DBP levels were compared under various water conditions, including chloride and bromide ion compositions, hold time, and organic matter-related parameters.
Results: After electrolysis, trihalomethane (THM) and haloacetic acid (HAA) levels increased from day 0 to day 5 under all salinities, whereas haloacetonitrile (HAN) level decreased. THMs were found to be the most dominant DBP group, followed by HAAs and HANs. In marine water and brackish water, brominated DBPs were dominant owing to high levels of bromide ions, while chlorinated DBP concentrations were relatively high in fresh water.
Discussion: After electrolysis, the specific ultraviolet absorption of the treated water was >4, indicating a high likelihood of generating carbonaceous DBPs such as THMs and HAA. These findings contribute to a better understanding of the general mechanisms through which physicochemical factors affect the formation of DBPs during electrolysis treatment of ballast water. This understanding is valuable in addressing issues related to the treatment and release of treated ballast water into the marine environment, which is an emerging environmental concern.
1 Introduction
Seaborne trade accounts for 80% of global trade as it enables long-distance mass transportation at a relatively low cost relative to the distances traveled (Ahn et al., 2017; UNCTAD, 2019; Lv et al., 2022). Ballast water constituting 30%–50% of the cargo load is loaded into vessels to control the trim, list, draft, stability, or stress of a ship (Mohammadkhanloo and Ghassemi, 2017; Gollasch and David, 2019; Sayinli et al., 2022). According to the International Maritime Organization (IMO), almost 10 billion tons of ballast water are transported annually by ships, with more than 7,000 species of marine life being moved with the ballast water daily (Tamelander et al., 2010; Zhang et al., 2014). Approximately 3% of these species adapt to their new environment, endangering native organisms and disturbing the ecosystems (Bax et al., 2003). Additionally, they adversely affect local aquatic economic activities (e.g., reducing fishing yields) and human health (e.g., Vibrio cholerae epidemics) (David and Perkovič, 2004; Hess-Erga et al., 2019; Lee et al., 2019; Kurniawan et al., 2022). Other water quality conditions, such as suspended matter, turbidity, and salinity, can further impair the ecosystem balance when ballast water is discharged into foreign seas and ports due to the dissimilar water environments of different sea areas (Kurniawan et al., 2022; Sayinli et al., 2022).
Consequently, to prevent ecological disturbance through regulation of the movement of alien species and prevent and minimize risks to the environment, human health, property, and resources, the International Convention for the Control and Management of Ships’ Ballast Water and Sediments (hereinafter referred to as the Convention) was adopted in 2004 and enforced on 8 September 2017 (International Maritime Organization, 2004, International Maritime Organization, 2018). According to the Convention, all ships operating in international ports must install a type approved ballast water treatment system (BWTS) to treat biological constituents (except bacteria and viruses) in ballast water in accordance with the IMO Regulation D-2 (Ballast Water Performance Standard) before discharging, until regular inspection for International Oil Pollution Prevention on 7 September 2024 (Supplementary Table S1) (International Maritime Organization, 2018).
Various BWTSs have been developed, including physical (membrane filtration and centrifugation), chemical (ozone, chlorine, and chemical injection), and mechanical (ultraviolet and ultrasonication) (Delacroix et al., 2013; Duan et al., 2016; International Maritime Organization, 2019; Sayinli et al., 2022). The BWTS mounted on a ship must be type approved by the flag state to pass land-based and shipboard tests verifying conformity; further, it should conform to both the basic and final approval criteria, depending on treatment type, based on the D-2 Ballast Water Performance Standard (Supplementary Table S1). In particular, BWTSs using active substances must be approved by the IMO based on the assessment of ship and crew safety, human health, and aquatic environmental hazards according to IMO G9 (International Maritime Organization, 2008; International Maritime Organization, 2017). Moreover, type approval of BWTSs using active substances requires technical review by the Group of Experts on the Scientific Aspects of Marine Environmental Protection (GESAMP) and resolution of the Marine Environment Protection Committee (MEPC). A total of 78 BWTSs have been developed and approved by the IMO, of which 47 use active substances (e.g., ozone and chlorine-linked oxidants) (International Maritime Organization, 2022).
Electrolysis is a type of disinfection process that uses activated substances; chlorine disinfectants, including sodium hypochlorite (NaOCl) and hypochlorous acid (HOCl), are produced from salt water (Stocks et al., 2004; David et al., 2018; Mazhar et al., 2020; Sayinli et al., 2022). These substances have strong oxidizing activity, sterilizing persistency after initial treatment, and cost-efficiency for the removal of invasive species (Hess-Erga et al., 2019). Despite these advantages, chlorine is toxic to aquatic organisms and generates toxic and carcinogenic disinfection byproducts (DBPs), such as trihalomethanes (THMs), haloacetic acids (HAAs), haloacetonitriles (HANs), as well as other emerging DBPs, such as 3-chloro-4-(dichloromethyl)-5-hydroxy-2(5H)-furanone and halophenols (Richardson et al., 2007; Gilca et al., 2020; Kali et al., 2021; Pandian et al., 2022). Among these DBPs, some THMs and HAAs are classified as belonging to group 2B (possibly carcinogenic to humans) of the International Agency for Research on Cancer (IARC) Monographs (International Agency for Research on Cancer, 2004).
DBPs have been found in discharged ballast water treated using active substances. David et al. (2018) confirmed 30 compounds among all DBPs (39 compounds) classified as chemicals relevant to the 42 BWTSs approved by the IMO. Moreover, Gonsior et al. (2015) and Romanelli et al. (2019) investigated DBPs produced by ballast water in port, coastal, and estuary water.
These substances have been shown to cause growth inhibition, genotoxicity, mutagenicity, and cytotoxicity in aquatic marine toxicity tests, and their potential risks to human health have been confirmed (Huang et al., 2013; Kali et al., 2021; Pandian et al., 2022). Although there are differences in controlled substances and water quality standards among countries, the United States, Japan, South Korea, and other developed countries have set total or individual water quality standards for THMs, HAAs, and HANs in drinking water (Gilca et al., 2020). However, no standards for the management of DBPs in ballast water discharge from ships have yet been established.
DBP formation is affected by various factors, including the constituents of water (anions and cations), chlorine level, reaction time, organic matter content, temperature, pH, and pipe material (e.g., stainless steel and ductile iron pipes) (Pourmoghaddas and Stevens, 1995; Lu et al., 2009; Zhang et al., 2009; Cha et al., 2015; David et al., 2018; Kali et al., 2021). In particular, organic matter (as a major precursor) may influence specific distribution patterns of DBPs (Zhang et al., 2009; Du et al., 2017; Kali et al., 2021). Therefore, understanding the interaction between water parameters and DBP formation would help clarify the characteristics of DBPs in electrolysis-based BWTSs. Research on DBPs generated in the process of ballast water treatment remains insufficient. Potential hazards of DBPs have been identified, but no discharge control standards for substances have been established, with only basic studies on DBPs and water characteristics reported to date (Huang et al., 2013; Lee et al., 2019; Kurniawan et al., 2022; Sayinli et al., 2022).
Therefore, the aim of this present study was to investigate the generation of DBPs and effects of water quality parameters on the electrolysis process. The main factors involved in the generation of DBPs were identified, which can serve as foundational data for establishing specific measures to reduce the occurrence of DBPs.
2 Materials and methods
2.1 Experimental design
Four electrolysis treatment systems were tested under three salinity conditions—i.e., seawater, brackish water, and fresh water—at a type approved land-based facility. Each test was conducted separately. For the start test (ballasting), 500 tons of test water were used as control (250 tons) and treated water (250 tons). The end test (deballasting) consisted of discharging water from the control and treated water tanks after a predetermined storage time. The challenge water was prepared by adding glucose as a dissolved organic carbon (DOC) source, starch as a particulate organic carbon (POC) source, silicates as total suspended solids, and organisms (microorganisms, phytoplankton, and zooplankton) to 500 tons of natural water under appropriate salinity conditions according to the IMO D-2 regulations for type approval tests. Supplementary Table S2 lists the required conditions for the challenge water according to the Ballast Water Performance Standards. Table 1 presents the specific parameters of the water used and detailed information on the land-based tests conducted in this study.
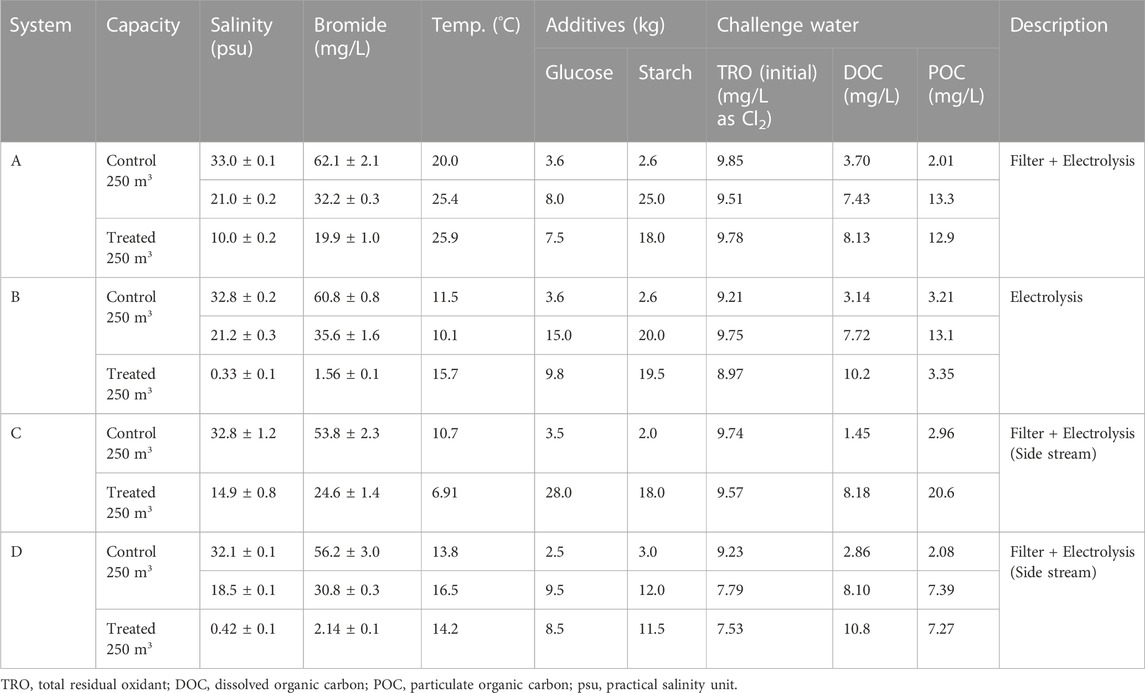
TABLE 1. Specific parameters of challenge water and detailed information on the conducted land-based tests.
Two types of electrolysis treatment were performed: i) an indirect side-stream method involving electrolysis of seawater to produce high-concentration HOCl, which disinfects by diluting the ballast water; ii) an in-stream electrolysis treatment method involving electrolysis of the entire ballast water and treatment with radicals, potential difference, and low-concentration HOCl. In both types, the total residual oxidant (TRO) concentration, which is the sum of free oxidants (such as Cl2) and combined chlorine, was maintained at 10 mg/L. The two types of electrolysis treatments performed in this study are shown in Figures 1A, B.
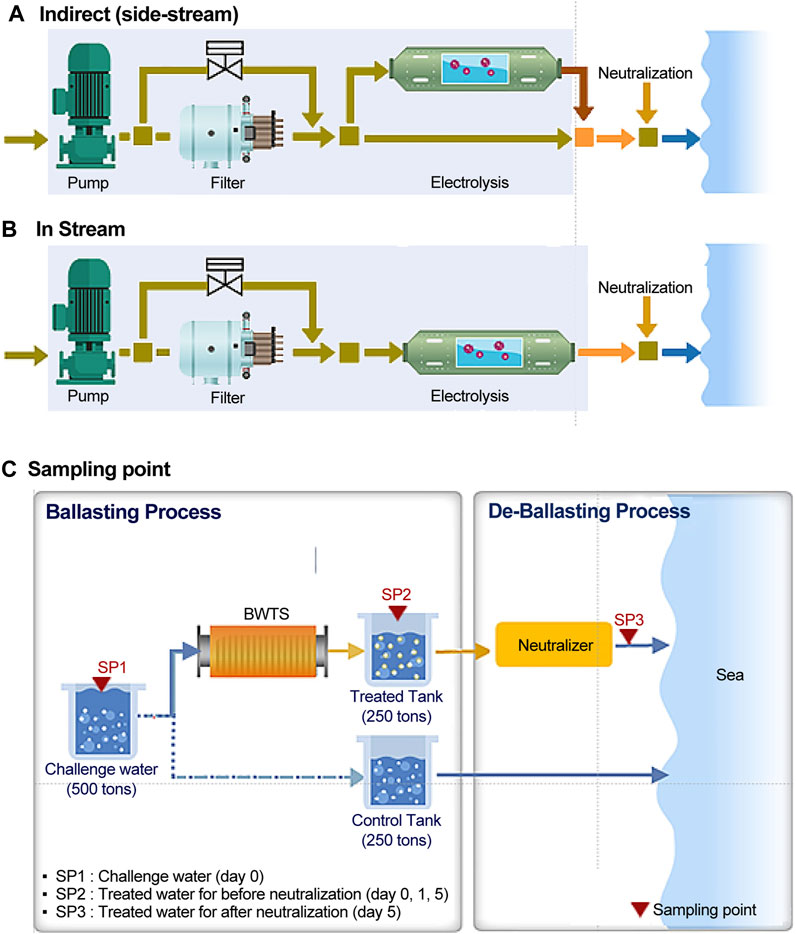
FIGURE 1. The two types of electrolysis treatment and sampling points used in this study: (A) indirect side-stream method; (B) in-stream electrolysis treatment method; (C) sampling points.
2.2 Sampling
To evaluate the generation characteristics and behavior of DBPs in treated ballast water, the IMO G9 requires evaluation of the changes in DBPs according to storage time. In this study, the occurrence and pattern of DBPs were investigated for 120 h after electrolysis treatment, assuming 5 days of ship operation. The samples were collected from the challenge and treated water immediately after electrolysis (ballasting process, day 0), 24 h later (day 1), and 120 h later (day 5) in each salinity zone (seawater, brackish water, and freshwater). On day 5, treated samples were collected both before and after neutralization (referred to as N-treated water) in the deballasting mode. Neutralized treated water was mixed with an appropriate amount of neutralizer to remove residual TROs to attain the maximum allowable discharge limit for TROs (<0.2 mg/L) set by the IMO before being discharged into the receiving water. Table 2; Figure 1C present the details of the sampling points. All analytes were injected with an appropriate preservation reagent for each analysis to prevent changes in properties. Especially for DBPs, sampling preservation and pretreatment were considered to have high TRO concentrations and salinity. The whole procedure from sampling to reporting was performed based on modified United States Environmental Protection Agency (US EPA) methods, ISO standards, or other verified methods while considering a matrix effect for components such as high salinity and particulate matter. For THMs and HAAs, sodium thiosulfate was added to the samples. Ammonium chloride was added to samples for the determination of HAN and chloropicrin (CP). The pH of the samples was adjusted using sulfuric acid for THMs, DOC, and POC, and phosphate buffer for HANs and CP. The samples were transported into the laboratory, stored at < 4°C, and analyzed within 14 days. The details of sampling points and parameters for land-based tests are presented in Figure 1; Table 1, respectively.
2.3 Target compounds
The target compounds for this study were divided into causative substances and DBPs. As causative substances, TRO, free residual oxidant (FRO), DOC, and POC contents as well as ultraviolet absorbance at 254 nm (UV254) were analyzed, and the specific ultraviolet absorption (SUVA) was calculated from the UV254 and DOC values. The TRO and FRO contents were measured to confirm the concentrations of active substances, such as NaOCl, HOCl, hypochlorite (OCl−), and bound chloramine generated in the electrolysis process. DOC and POC contents were measured to determine the concentrations of organic matter in the test waters. UV254 and SUVA were analyzed to investigate the characteristics of organic matter. THM4, HAA10, HAN9, and CP were selected as DBP response variables (Supplementary Table S3).
2.4 Reagents and standards
Reagents unaffected by impurities during the measurement and analysis of analytes were used in this study. Potassium hydrogen phthalate (reference material for DOC and POC) and calcium hypochlorite (technical grade; reference material for TRO and FRO) were purchased from Sigma-Aldrich (St. Louis, MO, United States). A 1000 mg/L stock solution of each reagent was prepared and diluted before use. The THMs, HAAs, HANs, and CP were purchased from AccuStandard (New Haven, CT, United States). Fluorobenzene and 1,2-dichlorobenzene-d4 were used as internal standards for THMs; decafluorobiphenyl (internal standard for HAAs and surrogate standard for HANs), 2-bromobutanoic acid (surrogate standard for HAAs), and p-bromofluorobenzene (internal standard for HANs) were purchased from AccuStandard (New Haven, CT, USA). Artificial seawater was made using Daigo’s artificial seawater SP (Nihon Pharmaceutical, Tokyo, Japan). High-performance liquid chromatography grade solvents [methyl tert-butyl ether (MtBE), methanol, acetone, and n-hexane] were purchased from Burdick & Jackson (Muskegan, MI, United States). Tertiary distilled water was produced using the Milli-Q system (Millipore, Billerica, MA, United States). All reagents used in this study were of guaranteed reagent grade.
2.5 Analytical methods
TROs and FROs are characterized by high reactivity immediately after generation and their concentrations decrease over time. It is therefore crucial to obtain reliable data and minimize the effects of interference. Consequently, TROs and FROs were immediately analyzed in the field using a CLX Online Residual Oxidant and Chlorine Monitor (HF Scientific; Salt Lake City, UT, United States) using the N,N-diethyl-1,4-phenylenediamine method (ISO 7393-2). DOC and POC were analyzed using a total organic carbon analyzer (TOC-VCPH; Shimadzu; Kyoto, Japan) according to ISO 8245. UV254 was measured using a UV/Vis spectrophotometer (V-650; Jasco, Easton, MD, United States) according to US EPA 415.3, and the SUVA was calculated by dividing the UV254 by the DOC values. THM4 was analyzed using purge and trap equipment with a gas chromatograph/mass spectrometer (GC/MS) (7890B GC-5977A MSD; Agilent, Santa Clara, CA, United States) according to US EPA 524.2. HAA10, HAN9, and CP were extracted using MtBE according to US EPA 552.3 for HAA10, and US EPA 551.1 for HAN9 and CP. For HAA10, derivatization was performed using a 10% sulfuric acid/methanol solution. The final extracts for HAA10, HAN9, and CP were determined using a gas chromatograph/electron capture detector (7890A GC; Agilent, Santa Clara, CA, USA) and GC/MS (7890B GC–5977A MSD).
2.6 Quality assessment/quality control (QA/QC)
Quantitative analysis of the analytes was performed using the internal standard method, and quality control was performed in accordance with the certification criteria for each item. Calibration curves were drawn with at least five concentrations. The coefficient of determination of the calibration curve was set to >0.99; the standard deviation of the relative sensitivity coefficient was set to <20%. Supplementary Table S3 lists the verification results, including the method detection limits, accuracy, and precision (relative standard deviation). The accuracy and precision were within the acceptable ranges (accuracy: 81.6%–123%, precision: 0.31%–16.0%). Blank, duplicate, and calibration check samples were analyzed by binning 20 samples together as one batch.
3 Results and discussion
3.1 DBP levels in treated waters in each salinity condition
We assessed the sum of DBPs (Figure 2) and variations in the concentrations of TROs, FROs, and DBPs (Figure 3) in electrolyzed water samples according to holding periods under each salinity condition. The concentrations of THM4, HAA10, and nitrogenous DBPs (N-DBPs) are listed in Supplementary Tables S4–S6. As shown in Figure 3A, during the 5 days of storage, as proposed by the GESAMP Ballast Water Working Group on Active Substances, DBPs formed in the treated water samples after electrolysis via the reaction of TROs with organic matter over time. The initial TRO and FRO concentrations in treated water (before neutralization) at ballasting (day 0) were 7.53–9.85 mg/L and 5.42–9.68 mg/L, respectively. After 5 days (after neutralization), all deballasting water satisfied the maximum allowable discharge concentration of <0.2 mg/L TRO as Cl2 under all salinity conditions. Chlorine produced in the electrolysis process is converted into HOCl, OCl−, OBr−, and HOBr−, which act as free oxidants. These species react with ammonia or organic nitrogen present in the water to form chloramines, such as monochloramine, dichloramine, and trichloramine, also known as combined chlorine (Mazhar et al., 2020). Further, as active substances, TRO and FRO react with organic matter in water (Lu et al., 2009; Zhang et al., 2009; Lee et al., 2017). Both TRO and FRO appeared to decrease during the holding period because of the organic matter added to the challenge water according to Regulation D-2 (Ballast Water Performance Standard) of the Convention (Supplementary Table S2).
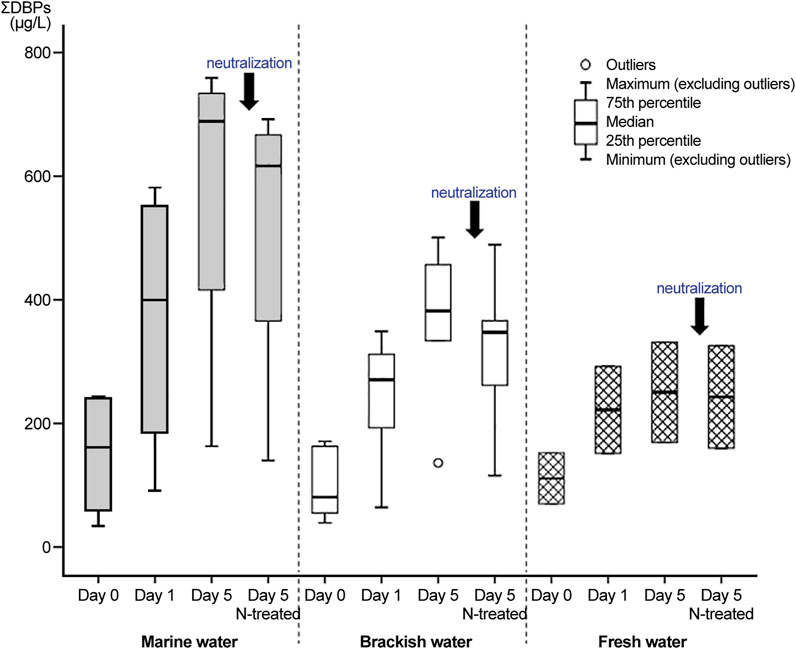
FIGURE 2. Total concentrations of disinfection byproducts (DBPs) in treated water during various holding periods.
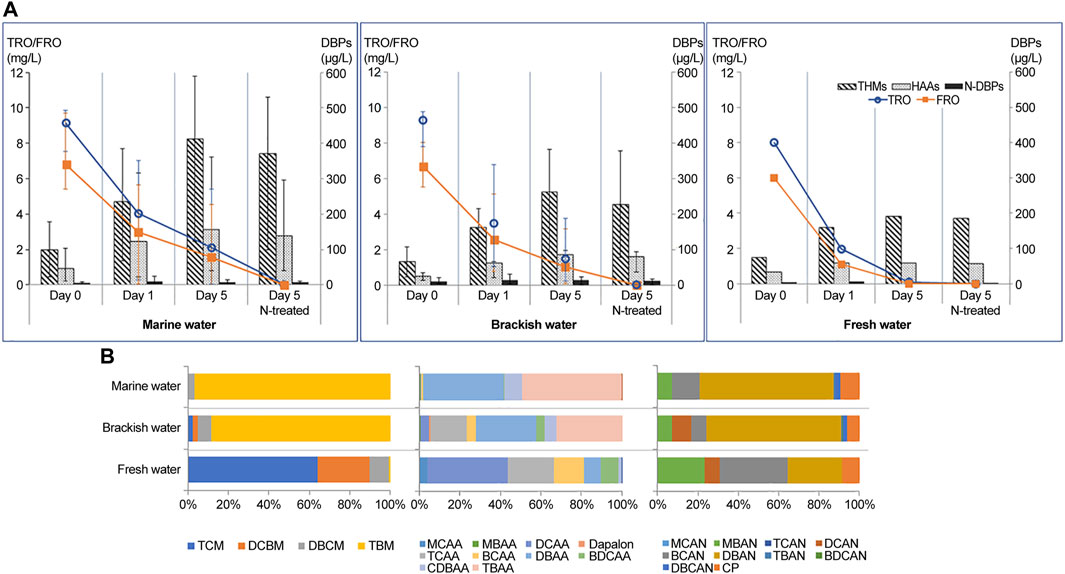
FIGURE 3. (A) Variations in the concentrations of total residual oxidants (TROs), free residual oxidants (FROs), and DBPs in electrolytic ballast water management systems (BWMSs); (B) proportion of DBPs before neutralization of treated water (day 5).
In contrast to the concentrations of TROs and FROs, concentration of DBPs (THM4, HAA10, and HANs) in the treated water following electrolysis increased from day 0–five under all salinity conditions (Figure 3A). The treated samples before neutralization in seawater had the highest levels (day 5: 163–759 μg/L), followed by brackish (day 5: 136–501 μg/L) and fresh water (day 5: 170–332 μg/L); the concentrations were low after neutralization (deballasting). We collected and analyzed challenge water and untreated water as a control, but did not obtain any specific results regarding DBPs.
The concentrations of THMs were significantly higher than those of HAA10 and HAN9. On day 5, the THM4 concentration was highest in seawater (123–584 μg/L), followed by that in brackish (93.3–382 μg/L) and fresh water (117–263 μg/L). Among the THMs, TMB had a 100% detection frequency and was mainly detected in seawater (119–570 μg/L) and brackish water (83.3–361 μg/L). Both dibromochloromethane (DBCM) (seawater: 11.9 μg/L; brackish water: 15.4 μg/L) and dichlorobromomethane (DCBM) (seawater: 1.68 μg/L; brackish water: 6.32 μg/L) were present at relatively low concentrations. In contrast, the trichloromethane (TCM) concentration was highest (21.1–153 μg/L) in fresh water. Before neutralizing the treated water (day 5), tribromomethane (TBM) had the highest concentration, followed by DBCM and DCBM, while TCM was not detected in seawater (Figure 3B). The composition of THMs in brackish water was similar to that in seawater. However, the distribution of THMs in freshwater showed the opposite pattern, with TCMs and DCBMs accounting for 65% and 20%, respectively. HAAs and HANs did not show a clear, substance-specific pattern like THMs did, but were classified as belonging to chlorinated and brominated DBPs.
HAA10 occurred in a pattern similar to that of THM4 under each salinity condition, with 39.6–360 μg/L in seawater, 41.1–144 μg/L in brackish water, and 51.9–67.1 μg/L in fresh water on day 5. Among the HAA10, dibromoacetic acid (DBAA) was detected under all salinity conditions. THM4 (64%–74%) and HAA10 (20%–34%) were the dominant groups, with higher contributions among DBPs in our experiments. Studies have reported that the reaction time positively influences the formation of THMs and HAAs (Pourmoghaddas and Stevens, 1995; Nikolaou et al., 2004; Water Resources Research Institute, 2008; Kali et al., 2021). Consistent with our observations, THMs and HAAs as major byproducts were shown to account for at least 80% of total DBPs formed in chlorinated water (Liu et al., 2011; Delacroix et al., 2013).
By contrast, HAN9 showed a decreasing trend during the holding period. N-DBPs, including HAN9 and CP, were present at lower levels than THM4 and HAA10. On day 5, the N-DBP levels were 1.12–13.5 μg/L in seawater, 1.78–22.8 μg/L in brackish water, and 0.90–1.08 μg/L in fresh water. In treated water, BCAN and DBAN (Dibromoacetonitrile) were detected with a 100% detection frequency under all salinity conditions. N-DBP concentrations did not increase continuously in treated water, and the levels were lower than those of other groups such as THM4 and HAA10.
According to research on the formation and pathway of N-DBPs, such as haloacetonitrile, halonitromethanes, and haloacetamides, they are rapidly generated during chlorine or chloramine disinfection via decarboxylation and undergo hydrolysis reactions (Reckhow et al., 2001; Lui et al., 2011; Shah and Mitch, 2012; Huang et al., 2013). They are produced with the influence of specific nitrogen-containing organic matter such as proteins and amino precursors (Huang et al., 2013). Since the concentration of nitrogenous compounds is typically less than 5% of the DOC level in water, nitrogen-containing DBPs occur at concentrations lower than those of carbonaceous DBPs (THMs and HAAs) and may have different occurrence patterns (Reckhow et al., 2001; Lui et al., 2012; Shah and Mitch, 2012). Kim et al. (2002) reported that HANs decreased with contact time after 48 h as DCAN, as reaction intermediates of DBP formation, such as TCAN, DCAA, or TCAA, disappeared. In addition, HANs are converted to haloacetamides after hydrolysis (Nihemaiti et al., 2017; Vu et al., 2018; Kali et al., 2021; Shao et al., 2022).
In a recent study, 30 DBPs classified as relevant chemicals were detected in treated waters (deballasting) generated from a BWTS (42 in total) using active substances (David et al., 2018). The frequency of occurrence of the four bromine DBPs was over 70%, including TBM (average: 247.14 μg/L), DBAA (average: 48.66 μg/L), DBCM (average: 22.11 μg/L), and monobromoacetic acid (average: 15.48 μg/L). Moreover, analysis of the DBPs (HAA, HAN, and THMs) in ballast water from seven ports in the Adriatic Sea within each medium (seawater, sediment, and transplanted mussels) showed that the highest TBM concentrations were present in seawater. The pattern and distribution of these compounds were similar to those detected in the present study, although the concentrations were slightly lower than those in this study.
3.2 Composition patterns of DBP species
Bromide ions (Br−) play a major role as a catalyst of brominated compounds (Allonier et al., 1999; Taylor, 2006). Consequently, DBP species had different composition characteristics of chlorinated and brominated compounds in treated water during the holding period (Figure 4).
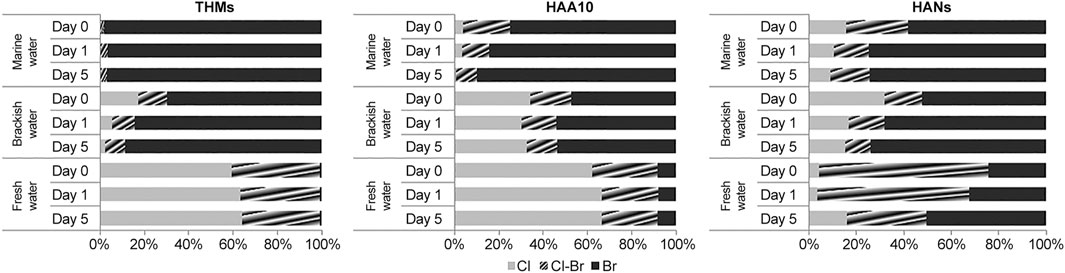
FIGURE 4. Compositional patterns of DBPs in treated water according to chlorinated and brominated species.
In the case of THM4, the brominated fraction (TBM) was greater than the other fractions in seawater (95.0%–100%) and brackish water (39.1%–94.6%). Although the brominated fraction was dominant in brackish water, the composition patterns somewhat differed from those in seawater because of the differences in Br− levels. Compared to seawater, brackish water had higher contents of chlorinated (0.0% compared to 0.0%–47.0%, respectively) and bromochlorinated (0.0%–4.96% compared to 5.4%–29.1%, respectively) species. In particular, the chlorinated compound TCM was not detected in seawater during ballast water electrolysis. The chlorinated fraction (56.5%–69.9%) was the highest in fresh water, followed by the bromochlorinated (30.1%–41.8%) and brominated fractions (0.0%–1.78%); further, relatively higher fractions of chlorinated compounds were present in freshwater than in seawater and brackish water.
The composition of HAA10 showed similar tendencies to that of THM4. In seawater, brominated species (39.9%–100%), such as TBAA and DBAA, were dominant, followed by the bromochlorinated (0.0%–60.1%) and chlorinated fractions (0.0%–16.1%). In brackish water, the brominated fraction (25.1%–67.6%) was found to be lower than in seawater, while chlorinated (12.6%–51.1%) and bromochlorinated species (0.0%–55.0%) showed the opposite trend. In fresh water, the fractions of chlorinated species (60.6%–68.5%), including TCAA and DCAA, were higher than those of both the brominated (6.4%–10.5%) and bromochlorinated species (24.4%–30.2%).
In seawater, brominated N-DBPs (20.4%–87.3%) were dominant, followed by bromochlorinated (6.0%–37.5%) and chlorinated species (2.1%–43.4%). The N-DBP compositions of brominated (29.3%–87.8%), chlorinated (3.9%–50.4%), and bromochlorinated species (4.9%–27.7%) in brackish water were similar to those in seawater, unlike THM4 and HAA10. Although the brominated fraction (21.8%–65.6%) in fresh water was lower than that in seawater and brackish water, brominated N-DBPs were more dominant than bromochlorinated (32.5%–73.0%) and chlorinated species (0.0%–31.9%). N-DBPs had somewhat different tendencies from those of THM4 and HAA10, suggesting that limiting factors such as nitrogen also affected N-DBP formation.
Overall, brominated DBPs increased with increasing Br− levels in ballast water, while chlorinated and bromochlorinated species showed the opposite trend. DBPs such as THMs and HAAs increase as Br− increases (Pourmoghaddas and Stevens, 1995). During ballast water electrolysis, the chlorine produced converts some of the Br− into hypobromous acid (HOBr−). This is because chlorine is a stronger oxidizer than Br−, and can oxidize these ions to form HOBr−. The generated HOBr− then reacts with the dissolved organic matter (DOM) in the water. If there are enough functional groups on the DOM for HOBr− to react with, both electrophilic substitution and electron transfer reactions will actively occur, resulting in the formation of a large amount of organic bromine compounds. Some of the formed organic bromine compounds can be further chlorinated by chlorine to form chlorinated organic compounds. During this process, Br− is re-oxidized by chlorine to form more HOBr−; if sufficient Cl− is present for reactions with organic matter, this cycle will continue (Langsa et al., 2017). In this study, brominated DBPs were dominant in both seawater and fresh water. The types and formation of DBPs influenced by Br− differed from those in freshwater, which is similar to results reported by previous studies (Pourmoghaddas and Stevens, 1995; Taylor, 2006; Sun et al., 2009). Differences in Br− (seawater: 51.3–65.1 mg/L; brackish water: 19.0–37.9 mg/L; and freshwater: 1.49–2.27 mg/L) and Cl− (seawater: 15,200–19400 mg/L; brackish water: 6,230–10900 mg/L; freshwater: 75.4–89.1 mg/L) concentrations due to salinity gradients can cause differences in the distribution of DBPs. Additionally, DBP concentrations during the holding period continuously increased due to chemical reactions between Br− and residual chlorine in the treated water.
3.3 Effects of organic matter on DBP formation
The DOC and POC levels in challenge water were 2.79 ± 0.96 and 2.57 ± 0.61 mg/L (mean ± standard deviation) in seawater, 8.03 ± 0.33 and 13.5 ± 4.70 mg/L in brackish water, and 10.5 ± 0.42 and 5.31 ± 2.77 mg/L in fresh water, respectively (Figure 5).
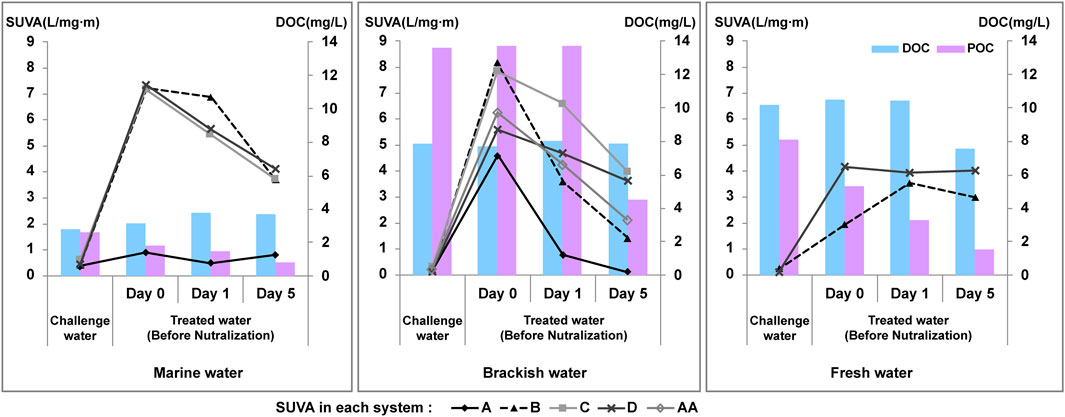
FIGURE 5. Levels of dissolved organic carbon (DOC), particulate organic carbon (POC), and specific ultraviolet absorption (SUVA) in the challenge and treated water for each system.
The SUVA was calculated to investigate the properties of the organic material in the water (Figure 5). In all cases, the values prior to electrolysis were <1 (0.13–0.62). In the treated water after electrolysis on day 0, except for system A, the SUVA values increased by more than 4 (maximum: 7.36), and subsequently decreased over time. Generally, a SUVA value of >4 indicates relatively hydrophobic DOM composed of aromatic, high molecular weight, organic substances (primarily aquatic humic substances). In contrast, a SUVA value of <3 indicates DOM composed of low molecular weight, organic, hydrophilic, non-humic substances. According to previous studies, an increase in SUVA values is indicative of a higher decrease in the DOC concentration and depends on the ratio of glucose to humic substances during microbial cultivation (Hur et al., 2009; Hur et al., 2013; Liu et al., 2014). In this study, the increase in the SUVA value on day 0 after electrolysis may be attributed to a considerable amount of DOM, produced by the decomposition of dead microbes into polymeric and refractory substances (Barker and Stuckey, 1999; Jarusutthirak and Amy, 2007). Furthermore, organic additives such as glucose and fructose added to the water could be converted into organic compounds with double bonds or lead to the enhancement of molecular aromatic substances after electrolysis. The higher the content of unsaturated bonds and aromatic substances in the water, the higher the UV254 value. These substances are highly reactive with oxidants such as ozone and chlorine, and thus the SUVA value appears to decrease over time (Liu et al., 2011).
Figure 6 shows the SUVA and DBP values normalized relative to the DOC concentrations. At SUVA values >3, the DBP formation pattern in water followed the order THM4 > HAA10 > N-DBPs under all salinity conditions. THMs can be generated from highly hydrophobic substances such as humic acids (Malliarou et al., 2005). However, DBP formation may also be affected by other water conditions (e.g., pH), as well as the properties of specific organic materials. For example, THMs are produced in greater amounts than HAAs at a pH of approximately 8 (Pourmoghaddas and Stevens, 1995; Lu et al., 2009). As the pH values of our test waters ranged from 7.6 to 9.2, the pH likely affected the compositions of THM4 and HAA10. Meanwhile, N-DBPs did not have any specific effects on the SUVA values because of their dependency on nitrogen (Liu and Li, 2010).
4 Conclusion
The results of this study suggest that individual DBP composition may be related to differences in Br− levels with respect to water salinity. In seawater and brackish water containing high Br− levels, brominated DBPs were dominant, whereas chlorinated species were more abundant in fresh water than in seawater and brackish water. The SUVA results also suggested the potential conversion of additives into hydrophobic DOCs and subsequent release of intracellular organic material from microorganisms following electrolysis. Furthermore, the results suggested that the compositions and amounts of DBPs formed by electrolysis can be affected by Br− and pH as well as the characteristics of organic matter. This study improves our understanding of the general mechanisms by which physicochemical factors affect the formation of DBPs during electrolysis treatment of ballast water. This can be helpful for addressing issues arising from the discharge of treated ballast water into the marine environment, which is an emerging environmental concern. Further research on DBPs released from treated ballast water and the effects of segmentalized characteristics of organic carbons is required to determine the details of their relationships with chemical functional groups and DBP formation.
Data availability statement
The original contribution presented in the study are included in the article/Supplementary Material, further inquiries can be directed to the corresponding author.
Author contributions
K-HC presided over the entire project and reviewed the manuscript. J-HL conceived the study, wrote the original draft and performed the analyses. JY supported the experiments and funding acquisition.
Funding
This study was supported by the Korea Institute of Marine Science and Technology Promotion funded by the Ministry of Oceans and Fisheries Korea (20210500) and a National Research Foundation of Korea (NRF) grant funded by the Korean Ministry of Science and ICT (No. 2022R1A2C1092562). The funders had no role in study design; in the collection, analysis and interpretation of data; in the writing of the report; or in the decision to submit the article for publication.
Conflict of interest
The authors declare that the research was conducted in the absence of any commercial or financial relationships that could be construed as a potential conflict of interest.
Publisher’s note
All claims expressed in this article are solely those of the authors and do not necessarily represent those of their affiliated organizations, or those of the publisher, the editors and the reviewers. Any product that may be evaluated in this article, or claim that may be made by its manufacturer, is not guaranteed or endorsed by the publisher.
Supplementary material
The Supplementary Material for this article can be found online at: https://www.frontiersin.org/articles/10.3389/fenvs.2023.1114353/full#supplementary-material
References
Ahn, Y. G., Lee, M. K., and Park, J. D. (2017). Factor analysis of seaborne trade volume affecting on the world economy. J. Korea Trade. Rev. 42, 277–296.
Allonier, A. S., Khalanski, M., Camel, V., and Bermond, A. (1999). Characterization of chlorination by-products in cooling effluents of coastal nuclear power stations. Mar. Pollut. Bull. 38, 1232–1241. doi:10.1016/S0025-326X(99)00168-X
Barker, D. J., and Stuckey, D. C. (1999). A review of soluble microbial products (SMP) in wastewater treatment systems. Water Res. 33, 3063–3082. doi:10.1016/s0043-1354(99)00022-6
Bax, N., Williamson, A., Aguero, M., Gonzalez, E., and Geeves, W. (2003). Marine invasive alien species: A threat to global biodiversity. Mar. Poli 27, 313–323. doi:10.1016/S0308-597X(03)00041-1
Cha, H. G., Seo, M. H., Lee, H. Y., Lee, J. H., Lee, D. S., Shin, K., et al. (2015). Enhancing the efficacy of electrolytic chlorination for ballast water treatment by adding carbon dioxide. Mar. Pollut. Bull. 95, 315–323. doi:10.1016/j.marpolbul.2015.03.025
David, M., Linders, J., Gollasch, S., and David, J. (2018). Is the aquatic environment sufficiently protected from chemicals discharged with treated ballast water from vessels worldwide? – a decadal environmental perspective and risk assessment. Chemosphere 207, 590–600. doi:10.1016/j.chemosphere.2018.05.136
David, M., and Perkovič, M. (2004). Ballast water sampling as a critical component of biological invasions risk management. Mar. Pollut. Bull. 49, 313–318. doi:10.1016/j.marpolbul.2004.02.022
Delacroix, S., Vogelsang, C., Tobiesen, A., and Liltved, H. (2013). Disinfection by-products and ecotoxicity of ballast water after oxidative treatment–results and experiences from seven years of full-scale testing of ballast water management systems. Mar. Pollut. Bull. 73, 24–36. doi:10.1016/j.marpolbul.2013.06.014
Du, Y., Lv, X. T., Wu, Q. Y., Zhang, D. Y., Zhou, Y. T., Peng, L., et al. (2017). formation and control of disinfection byproducts and toxicity during reclaimed water chlorination: A review. J. Environ. Sci. (China). 58, 51–63. doi:10.1016/j.jes.2017.01.013
Duan, D., Liu, G., Yao, P., and Wang, H. (2016). The effects of organic compounds on inactivation efficacy of artemia salina by neutral electrolyzed water. Ocean. Eng. 125, 31–37. doi:10.1016/j.oceaneng.2016.08.003
Gilca, A. F., Teodosiu, C., Fiore, S., and Musteret, C. P. (2020). Emerging disinfection byproducts: A review on their occurrence and control in drinking water treatment processes. Chemosphere 259, 127476. doi:10.1016/j.chemosphere.2020.127476
Gollasch, S., and David, M. (2019). “Ballast water: Problems and management,” in World seas: An environmental evaluation (second edition). Elsevier. 237–250. doi:10.1016/B978-0-12-805052-1.00014-0
Gonsior, M., Mitchelmore, C., Heyes, A., Harir, M., Richardson, S. D., Petty, W. T., et al. (2015). Bromination of marine dissolved organic matter following full scale electrochemical ballast water disinfection. Environ. Sci. Technol. 49, 9048–9055. doi:10.1021/acs.est.5b01474
Hess-Erga, O. K., Moreno-Andrés, J., Enger, Ø., and Vadstein, O. (2019). Microorganisms in ballast water: Disinfection, community dynamics, and implications for management. Sci. Total Environ. 657, 704–716. doi:10.1016/j.scitotenv.2018.12.004
Huang, H., Wu, Q. Y., Tang, X., Jiang, R., and Hu, H. Y. (2013). Formation of haloacetonitriles and haloacetamides during chlorination of pure culture bacteria. Chemosphere 92, 375–381. doi:10.1016/j.chemosphere.2013.01.031
Hur, J., Park, M. H., and Schlautman, M. A. (2009). Microbial transformation of dissolved leaf litter organic matter and its effects on selected organic matter operational descriptors. Environ. Sci. Technol. 43 (7), 2315–2321.
Hur, J., Lee, M. H., Song, H., and Schlautman, M. A. (2013). Microbial transformation of dissolved organic matter from different sources and its influence on disinfection byproduct formation potentials. Environ. Sci. Pollut. Res. 20, 4176–4187.
International Agency for Research on Cancer (2004). “IARC Monographs on the evaluation of the carcinogenic risk to humans,” in Drinking-water disinfectants and contaminants, including arsenic (Lyon: IARC Press), 84.
International Maritime Organization (2018). Ballast water management convention and BWMS code with guidelines for implementation. 2018 edition (KA621E). ISBN 978-92-801-17028.
International Maritime Organization (2022). Current lists of ballast water management systems, which received type approval certification, basic and final approval. Available at: https://wwwcdn.imo.org/localresources/en/OurWork/Environment/Documents/Table%20of%20BA%20FA%20and%20TA,%20updated%20December%202021.pdf (Accessed September 28, 2022).
International Maritime Organization (2004). International convention for the control and management of ships’ ballast water and sediments (BWM). Available at: https://www.imo.org/en/About/Conventions/Pages/International-Convention-for-the-Control-and-Management-of-Ships%27-Ballast-Water-and-Sediments-(BWM).aspx (Accessed September 28, 2022).
International Maritime Organization (2019). List of ballast water management systems that make use of active substances which received basic and final approval. Available at: https://wwwcdn.imo.org/localresources/en/OurWork/Environment/Documents/Table%20of%20BA%20FA%20TA%20updated%20January%202020.pdf (Accessed September 28, 2022).
International Maritime Organization (2017). Methodology for information gathering and conduct of work of the GESAMP-BWWG. Available at: https://wwwcdn.imo.org/localresources/en/OurWork/Environment/Documents/BWM.2-Circ.13-Rev-4.pdf (Accessed September 28, 2022).
International Maritime Organization (2008). “Procedure for approval of ballast water management systems that make use of active substances (G9),” in Resolution MEPC, 169. Available at: https://wwwcdn.imo.org/localresources/en/KnowledgeCentre/IndexofIMOResolutions/MEPCDocuments/MEPC.169(57).pdf (Accessed September 28, 2022).
Jarusutthirak, C., and Amy, G. (2007). Understanding soluble microbial products (SMP) as a component of effluent organic matter (EfOM). Water Res. 41, 2787–2793. doi:10.1016/j.watres.2007.03.005
Kali, S., Khan, M., Ghaffar, M. S., Rasheed, S., Waseem, A., Iqbal, M. M., et al. (2021). Occurrence, influencing factors, toxicity, regulations, and abatement approaches for disinfection by-products in chlorinated drinking water: A comprehensive review. Environ. Pollut. 281, 116950. doi:10.1016/j.envpol.2021.116950
Kim, H., Shim, J., and Lee, S. (2002). Formation of disinfection by-products in chlorinated swimming pool water. Chemosphere 46 (1), 123–130. doi:10.1016/S0045-6535(00)00581-6
Kurniawan, S. B., Pambudi, D. S. A., Ahmad, M. M., Alfanda, B. D., Imron, M. F., and Abdullah, S. R. S. (2022). Ecological impacts of ballast water loading and discharge: Insight into the toxicity and accumulation of disinfection by-products. Heliyon 8, e09107. doi:10.1016/j.heliyon.2022.e09107
Langsa, M., Heitz, A., Joll, C. A., Von Gunten, U., and Allard, S. (2017). Mechanistic aspects of the formation of adsorbable organic bromine during chlorination of bromide-containing synthetic waters. Environ. Sci. Technol. 51 (9), 5146–5155. doi:10.1021/acs.est.7b00691
Lee, J., Shon, M. B., Cha, H. G., and Choi, K. H. (2017). The impact of adding organic carbon on the concentrations of total residual oxidants and disinfection by-products in approval tests for ballast water management systems. Sci. Total Environ. 605–606, 852–859. doi:10.1016/j.scitotenv.2017.06.263
Lee, J. S., Hong, S., Lee, J., Choi, T. S., Rhie, K., and Khim, J. S. (2019). Evaluation of residual toxicity of hypochlorite-treated water using bioluminescent microbes and microalgae: Implications for ballast water management. Ecotoxicol. Environ. Saf. 167, 130–137. doi:10.1016/j.ecoenv.2018.10.002
Liu, J. L., and Li, X. Y. (2010). Biodegradation and biotransformation of wastewater organics as precursors of disinfection byproducts in water. Chemosphere 81, 1075–1083. doi:10.1016/j.chemosphere.2010.09.041
Liu, W., Zhao, Y., Chow, C. W., and Wang, D. (2011). Formation of disinfection byproducts in typical Chinese drinking water. J. Environ. Sci. (China) 23, 897–903. doi:10.1016/S1001-0742(10)60493-7
Liu, J. L., Li, X. Y., Xie, Y. F., and Tang, H. (2014). Characterization of soluble microbial products as precursors of disinfection byproducts in drinking water supply. Sci. Total Environ. 472, 818–824.
Lu, J., Zhang, T., Ma, J., and Chen, Z. (2009). Evaluation of disinfection by-products formation during chlorination and chloramination of dissolved natural organic matter fractions isolated from a filtered river water. J. Hazard. Mater. 162, 140–145. doi:10.1016/j.jhazmat.2008.05.058
Lui, Y. S., Hong, H. C., Zheng, G. J., and Liang, Y. (2012). Fractionated algal organic materials as precursors of disinfection by-products and mutagens upon chlorination. J. Hazard. Mater. 209, 278–284. doi:10.1016/j.jhazmat.2012.01.023
Lui, Y. S., Qiu, J. W., Zhang, Y. L., Wong, M. H., and Liang, Y. (2011). Algal-derived organic matter as precursors of disinfection by-products and mutagens upon chlorination. Water Res. 45, 1454–1462. doi:10.1016/j.watres.2010.11.007
Lv, B., Shi, J., Li, T., Ren, L., Tian, W., Lu, X., et al. (2022). Deciphering the characterization, ecological function and assembly processes of bacterial communities in ship ballast water and sediments. Sci. Total Environ. 816, 152721. doi:10.1016/j.scitotenv.2021.152721
Malliarou, E., Collins, C., Graham, N., and Nieuwenhuijsen, M. J. (2005). Haloacetic acids in drinking water in the United Kingdom. Water Res. 39, 2722–2730. doi:10.1016/j.watres.2005.04.052
Mazhar, M. A., Khan, N. A., Ahmed, S., Khan, A. H., Hussain, A., Rahisuddin, F., et al. (2020). Chlorination disinfection by-products in municipal drinking water–a review. J. Clean. Prod. 273, 123159. doi:10.1016/j.jclepro.2020.123159
Mohammadkhanloo, K., and Ghassemi, H. (2017). Critical review of the IMO on ballast water convention and its impact on shipping. IJMSE 8 (2), 29–33.
Nihemaiti, M., Le Roux, J., Hoppe-Jones, C., Reckhow, D. A., and Croué, J. P. (2017). Formation of haloacetonitriles, haloacetamides, and nitrogenous heterocyclic byproducts by chloramination of phenolic compounds. Environ. Sci. Technol. 51 (1), 655–663. doi:10.1021/acs.est.6b04819
Nikolaou, A. D., Golfinopoulos, S. K., Lekkas, T. D., and Arhonditsis, G. B. (2004). Factors affecting the formation of organic by-products during water chlorination: A bench-scale study. Water Air Soil Pollut. 159, 357–371. doi:10.1023/b:wate.0000049189.61762.61
Pandian, A. M. K., Rajamehala, M., Singh, M. V. P., Sarojini, G., and Rajamohan, N. (2022). Potential risks and approaches to reduce the toxicity of disinfection by-product–A review. Sci. Total Environ. 822, 153323. doi:10.1016/j.scitotenv.2022.153323
Pourmoghaddas, H., and Stevens, A. A. (1995). Relationship between trihalomethanes and haloacetic acids with total organic halogen during chlorination. Water Res. 29, 2059–2062. doi:10.1016/0043-1354(95)00026-h
Reckhow, D. A., MacNeill, A. L., Platt, T. L., MacNeill, A. L., and McClellan, J. N. (2001). Formation and degradation of dichloroacetonitrile in drinking waters. J. Water Supply Res. Technol.—AQUA 50, 1–13. doi:10.2166/aqua.2001.0001
Richardson, S. D., Plewa, M. J., Wagner, E. D., Schoeny, R., and DeMarini, D. M. (2007). Occurrence, genotoxicity, and carcinogenicity of regulated and emerging disinfection by-products in drinking water: A review and roadmap for research. Mutat. Res. 636, 178–242. doi:10.1016/j.mrrev.2007.09.001
Romanelli, G., Berto, D., Calace, N., Amici, M., Maltese, S., Formalewicz, M., et al. (2019). Ballast water management system: Assessment of chemical quality status of several ports in Adriatic Sea. Mar. Pollut. Bull. 147, 86–97. doi:10.1016/j.marpolbul.2017.12.030
Sayinli, B., Dong, Y., Park, Y., Bhatnagar, A., and Sillanpää, M. (2022). Recent progress and challenges facing ballast water treatment–a review. Chemosphere 291, 132776. doi:10.1016/j.chemosphere.2021.132776
Shah, A. D., and Mitch, W. A. (2012). Halonitroalkanes, halonitriles, haloamides, and N-nitrosamines: A critical review of nitrogenous disinfection Byproduct Formation pathways. Environ. Sci. Technol. 46, 119–131. doi:10.1021/es203312s
Shao, B., Shen, L., Liu, Z., Tang, L., Tan, X., Wang, D., et al. (2022). Disinfection byproducts formation from emerging organic micropollutants during chlorine-based disinfection processes. Chem. Eng. J. 455, 140476. doi:10.1016/j.cej.2022.140476
Stocks, D. T., O’Reilly, M., and McCracken, W. (2004). “Sodium hypochlorite as a ballast water biocide,” in Second international symposium on ballast water treatment (London: International Maritime Organization), 80–87.
Sun, Y. X., Wu, Q. Y., Hu, H. Y., and Tian, J. (2009). Effect of bromide on the formation of disinfection by-products during wastewater chlorination. Water Res. 43, 2391–2398. doi:10.1016/j.watres.2009.02.033
Tamelander, J., Riddering, L., Haag, F., Matheickal, J., and No, G. M. S. (2010). “Guidelines for development of a national ballast water management strategy,” in GloBallast monograph series No. 18, international Maritime organization (London: GloBallast Partnerships Project Coordination Unit).
Taylor, C. J. (2006). The effects of biological fouling control at coastal and estuarine power stations. Mar. Pollut. Bull. 53, 30–48. doi:10.1016/j.marpolbul.2006.01.004
Vu, T. N., Kimura, S. Y., Plewa, M. J., Richardson, S. D., and Mariñas, B. J. (2018). Predominant N-haloacetamide and haloacetonitrile formation in drinking water via the aldehyde reaction pathway. Environ. Sci. Technol. 53 (2), 850–859. doi:10.1021/acs.est.8b02862
Water Resources Research Institute (2008). Control of haloacetic acid formation in North Carolina drinking water. Available at: https://repository.lib.ncsu.edu/handle/1840.4/4109 (Accessed September 28, 2022).
Zhang, H., Qu, J., Liu, H., and Zhao, X. (2009). Characterization of isolated fractions of dissolved organic matter from sewage treatment plant and the related disinfection by-products formation potential. J. Hazard. Mater. 164, 1433–1438. doi:10.1016/j.jhazmat.2008.09.057
Keywords: active substance, ballast water treatment, disinfection byproduct, electrolysis, water parameter
Citation: Lee J-H, Choi K-H and Yang JH (2023) Effects of physicochemical parameters of water on byproduct formation during electrolysis of ballast water. Front. Environ. Sci. 11:1114353. doi: 10.3389/fenvs.2023.1114353
Received: 05 December 2022; Accepted: 15 March 2023;
Published: 28 March 2023.
Edited by:
Huiyu Dong, Research Center for Eco-environmental Sciences (CAS), ChinaCopyright © 2023 Lee, Choi and Yang. This is an open-access article distributed under the terms of the Creative Commons Attribution License (CC BY). The use, distribution or reproduction in other forums is permitted, provided the original author(s) and the copyright owner(s) are credited and that the original publication in this journal is cited, in accordance with accepted academic practice. No use, distribution or reproduction is permitted which does not comply with these terms.
*Correspondence: Keun-Hyung Choi, a2V1bmhjaG9pQGNudS5hYy5rcg==