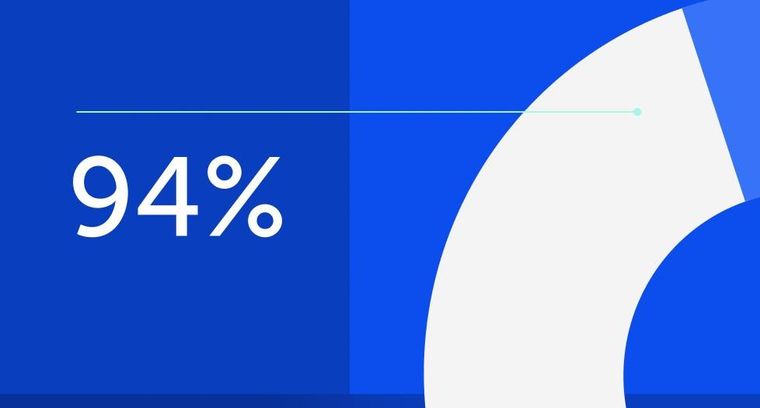
94% of researchers rate our articles as excellent or good
Learn more about the work of our research integrity team to safeguard the quality of each article we publish.
Find out more
ORIGINAL RESEARCH article
Front. Environ. Sci., 25 May 2023
Sec. Water and Wastewater Management
Volume 11 - 2023 | https://doi.org/10.3389/fenvs.2023.1111199
This article is part of the Research TopicWastewater Treatment: Removal of Recalcitrant CompoundsView all 7 articles
To reduce the hazard of sulfamethoxazole (SMX) to the water ecology, the removal mechanism of SMX in wastewater was revealed. This study evaluated the PVDF/DA modified membrane in the membrane bioreactor (MBR) toward the removal and degradation of SMX as a recalcitrant micropollutant. A strategy of dopamine for surface modification of polyvinylidene fluoride (PVDF) membrane was proposed, and PVDF/DA membrane was prepared. Subsequently, PVDF/DA was run in the MBR for 60 days, and the reactor could produce good removal ability for SMX after certain acclimation. When the SMX concentration increased from 0.5 to 2 mg/L, liquid chromatography was used for analysis, and the results showed that the average removal rate was above 85%. The removal effect of COD and NH4+-N and the degree of membrane fouling were also evaluated. By measuring the extracellular polymer (EPS) content, the results showed that after 30 days of operation, the protein and polysaccharide contents in the cake layer on the surface of the PVDF membrane were 1.35 times and 1.49 times that of PVDF/DA membrane, respectively, and the PVDF/DA membrane could alleviate membrane fouling, thereby improving the service life of the membrane and reducing its operating cost. Finally, degradation by-products are evaluated to achieve deeper insight into the degradation mechanisms in the MBR process.
In recent years, antibiotics have received more and more attention and are considered emerging micropollutants due to their relatively stable structure and anti-biodegradability (Bilal et al., 2020; Bungau et al., 2021; Mohapatra et al., 2023a). Although they are present in low concentrations, when antibiotics enter water, they not only induce antibiotic resistance genes, but also combine with other pollutants, producing compound pollutants that are more toxic to aquatic organisms and humans, which may pose a serious threat to human health and environment (Carvalho and Santos., 2016; Felis et al., 2020; Kovalakova et al., 2020).
Indeed, many studies indicate the detection of antibiotics in both wastewater treatment plants, as well as surface and groundwater (Patel et al., 2019; Quesada et al., 2019; Mohapatra et al., 2023b). Sulfonamides are one of the oldest antibiotics groups that is still widely used (White et al., 2019; Priyanka and Mohapatra., 2020). For example, SMX, a widely used sulfonamide antibiotic, usually prescribed to treat various infectious diseases in humans (Ryan et al., 2011; Johnson et al., 2015; Prasannamedha and Sennthil Kumar, 2020), has been detected at concentrations of up to 1.1 μg/L in surface water (Barnes et al., 2008; Su et al., 2016) and up to 2.8 μg/L in wastewater treatment plants (Batt et al., 2007). Wastewater treatment plants are often recognized as the main source of antibiotics discharge into the environment (Prieto-Rodriguez et al., 2012; Sabri et al., 2020).
For the removal of antibiotics, a large number of technologies have been investigated, such as adsorption, advanced oxidation, membrane separation, activated sludge and membrane bioreactor (MBR) (Bartolomeu et al., 2018; Yang et al., 2019; Phoon et al., 2020). Among the various processes, MBR seems to be the most interesting one since the use of biological treatment can lead to an integrated green solution. Biological treatment is simple and easy to operate, cost-effective and does not generate toxic by-products (Chaturvedi et al., 2020; Cheng et al., 2020; Lu et al., 2020). For example, the removal rates of ofloxacin and SMX in conventional activated sludge are 23.8% and 55.6%, the removal rates in the MBR process is 94% and 60.5% (Radjenovic et al., 2007). The difference between the two processes is that the MBR process has a higher biomass and a longer sludge retention time (Schaar et al., 2010). They include, a combination of biological treatment with membrane filtration (Xiao et al., 2019). The removal efficiency of the MBR remains stable, and the development of biofilm on the membrane can play a role in reducing the intercepted pore size, realizing the retention of micropollutants or forcing them to enter the biofilm for biodegradation (Luo et al., 2014).
However, further improvements are needed, mainly in the development of modified methods for membrane fouling, to make these processes cost-effective while retaining high efficiency. Moreover, the modified membrane has gained a great deal of attention recently as shows synergistic roles in membrane filtration by enhancing pollutant degradation, improving filtration performance, and mitigating membrane fouling (Zhao et al., 2015; Zhang et al., 2020). Therefore, it is important to modify the membranes with necessary hydrophilic modifications to improve the overall performance. In 2007, Lee et al. (2007) discovered that marine mussels can firmly adhere to rocks and even submarine reef surfaces. It was found that the adhesion of mussel adhesion protein played a crucial role, and dopamine-containing both catechol hydroxyl and lysine amino groups are used instead of adhesion protein. DA coating was formed on the surface of the object, which could improve the hydrophilicity and anti-fouling ability of the membrane.
DA coating has the advantages of simple operation, mild and controllable conditions, and green environmental protection, which makes this modification method the prospect of wide application (Huang et al., 2020). Liu et al. (2013) modified a low-cost polyester filter cloth with DA coating under milder conditions, and the flux increased steadily by 36% in the MBR short-term test, and the irreversible pollution resistance of the modified membrane was low and the filtration cycle was longer. Xiang et al. (2015) immersed the PVDF membrane in a Tris-HCl solution of 2 g/L dopamine (pH = 8.5), exhibited excellent resistance to protein fouling, and quickly recovered throughput after membrane cleaning. Most studies have focused on the effect of membrane fouling, and less attention has been paid to the operation of PVDF/DA membranes to remove antibiotics in MBR.
This study evaluated the utility of PVDF/DA membrane in MBR. In the experiment, changes in COD, NH4+-N, and EPS were monitored and the degradation of SMX was identified by high-performance liquid chromatography (HPLC) technology. To detect SMX, which is used for the evaluation of removal efficiencies in MBR, the intermediate products of microbial degradation were further explored by using mass spectrometry (MS).
The PVDF flat membrane (0.22 μm) was purchased from Zhongke Ruiyang Membrane Technology Co., Ltd. in Beijing, China. SMX, DA, and Tris (hydroxymethyl)aminomethane-hydrochloric acid (Tris-HCl) were purchased from Aladdin Bio-Chem Technology Co., Ltd. in Shanghai, China. The chemicals, isopropanol, Glucose, starch, peptone, sodium bicarbonate, dipotassium hydrogen phosphate, ammonium chloride, magnesium chloride, calcium chloride, and hydrochloric acid (HCl) were obtained from Comio Chemical Reagent Co., Ltd. in Tianjin, China. Deionized (DI) water was provided by the Milli-Q Water Purification System.
The preparation of PVDF/DA modified membrane was prepared by the surface modification method after the optimization of the response surface of the research group: PVDF membrane was immersed in isopropanol for 2 h, and soaked in deionized water for 24 h. Prepare 0.01 mol/L, pH = 8.5 Tris-HCl buffer solution, configure into a mass concentration of 1.65 g/L DA solution, immerse the pretreated PVDF membrane in DA solution, put it into a shaker constant temperature at 120 r/min oscillation, end the self-polymerization reaction process, take out the membrane material and rinse it with deionized water, put it into a vacuum drying oven (DHG-9140A, Shanghai Precision Scientific Instrument Co., China) at 25°C, and obtain PVDF/DA membrane after drying.
Several characterizations were conducted to study the effect of the modification on the resulting membranes. The morphology of the membrane surface was characterized by scanning electron microscopy (SEM, S4800, Hitachi, Japan). The chemical groups of the membranes were characterized by fourier infrared spectrometer (FTIR, Nicolet 6700, Thermo Fisher Scientific, United States). Proteins and polysaccharides were stained by fluorescent dyes FITC and Calcofluor white, and polysaccharides and proteins on the surface of the contaminated membrane were observed by laser scanning confocal microscopy (CLSM, Leica SP8 SR, Germany), which showed fluorescence of different colors. The hydrophilicity of the membrane surface was measured by a contact angle goniometer (Model No.250, Ram-Hart, United States). The samples were cut into approximately 5 cm × 1 cm for contact angle testing.
In this experiment, an MBR integrated reactor was used, as shown in Figure 1, the reactor was made of plexiglass, with an effective volume of 10.6 L. In parallel comparison experiments, two sets of flat membrane modules were submerged in the reactor, and an aeration tube was set under the membrane module, which used microporous aeration to provide oxygen and generate cross-flow on the membrane surface to reduce the deposition of contaminants on the membrane surface. The experimental sewage enters the reactor from the high water tank, the inlet water tank volume is 50 L, and the effluent is filtered by the membrane module and sucked out by the peristaltic pump. The dissolved oxygen is about 2.5 mg/L, the pump-stop ratio is 8: 2, the pH is between 7 and 8, the temperature is maintained at (25 ± 1)°C, and no sludge discharge is carried out during the whole experiment.
The experimental synthetic water quality will be artificially simulated synthesis according to the typical domestic sewage water quality, the water distribution components are shown in Table 1, the activated sludge inoculated in this experiment comes from Shenzhen Deep Water Bright Water Environment Co., China, second sedimentation tank return sludge, the initial concentration of activated sludge in MBR is 2,571 mg/L. To explore the effect and degradation pathway of removing SMX from the dopamine-modified membrane in MBR application. The test is run in three stages, the antibiotic concentration of stages I-III is 0.5, 1, and 2 mg/L, the I. and II. stages are run for 15 days, and the III. stage is run for 30 days, and the inlet and outlet water samples are taken once a day and stored at 4°C after passing the 0.22 μm filter membrane, waiting for HPLC (Shimadzu LC-40, Japan) detection. COD is measured by using the potassium dichromate method. NH4+-N is measured according to the standard method by using an ultraviolet-visible spectrophotometer (UV-7504 (A), Shanghai Huyueming Scientific Instrument Co., China). MLSS is measured by the gravimetric method. EPS were determined by 60°C thermal extractions.
SMX samples were detected using HPLC, in this case, mobile phase A is 0.1% formic acid aqueous solution, and mobile phase B is methanol, the ratio of the two is A: B (V: V) = 60: 40. The column was C18 analytical column (1.7 μm, 2.1 × 150 mm, Agilent, US), the injection volume was 20 μL, the flow rate was 0.5 mL/min, the column temperature was 40°C, the detection wavelength was 269 nm, the SMX peak time was about 5.52 min, the peak output was complete without tailing and the peak type was intact.
To test the MBR degradation pathway of SMX, the intermediate products of the SMX degradation process were detected by liquid chromatography-mass spectrometry (LC-MS/MS, Thermo Fisher Q Exactive, United States), mobile phase A was acetonitrile solution of 0.1% formic acid, mobile phase B was 0.1% formic acid aqueous solution, the column was C18 column (2.6 μm, 2.1 × 100 mm), column temperature was 25°C, the flow rate was 0.2 mL/min, injection volume 20 μL.
As shown in Figure 2, the contact angle of the PVDF membrane modified by dopamine is reduced from 69° to 33.9°, which is due to the oxidation of dopamine in a weak base solution, and through covalent or non-covalent bonds, a polydopamine coating is formed on the membrane surface, which contains a large number of hydrophilic groups, which improves the hydrophilicity of the PVDF membrane surface. The chemical structures of the PVDF membrane and PVDF/DA modified membrane were analyzed by Fourier infrared spectroscopy, and the infrared spectra are shown in Figure 3. Compared with the original PVDF membrane, the modified flat membrane showed new absorption peaks: at 3,600 cm−1 to 3,200 cm−1 was the -OH stretching vibration peak, which was due to the -OH structure of dopamine itself; the absorption peak at 1,610 cm−1 was the C = C double bond stretching vibration, which was related to the benzene ring structure contained in dopamine; the absorption peak at 1,510 cm−1 was the N-H bending vibration, which was related to the -NH2 contained in dopamine. These are all characteristic peaks belonging to DA, indicating that DA has indeed formed a polymer through self-polymerization, covering the surface of the PVDF membrane.
In addition, the morphological structure before and after the modification of the membrane surface was further characterized by SEM. As shown in Figure 4A, the SEM images on the surface of the PVDF membrane were magnified a thousand times, and the membrane surface could be seen to be very smooth. In Figure 4B, the membrane pores in the PVDF/DA membrane were covered by dopamine polymer, and the number of dopamine particles was large and evenly distributed. Compared with the PVDF membrane, the surface of the PVDF/DA membrane has relatively good roughness, and this surface morphology will be more conducive to the attachment and growth of microorganisms, and more conducive to the process of the biological hanging membrane in the early stage. Due to the short polymerization time, the coating did not completely cover the membrane pores, indicating that the dopamine-modified PVDF membrane did not destroy the membrane pore structure and could ensure a certain water flux.
It can be seen from Figure 5 that at the beginning of stage I, the concentration of SMX in the influent water is 0.43–0.53 mg/L, the removal rate of PVDF membrane and PVDF/DA membrane is 56.12% and 56.93%, SMX is a toxic refractory organic pollutant, in the early stage of the test because the sludge has not been domesticated by antibiotics, the removal effect of SMX is poor, but the removal rate of about 50% also indicates that activated sludge has a certain removal ability for SMX. After a period of domestication, SMX began to be rapidly removed in stage II and stage III, although the concentration of SMX in the influent increased, it did not cause a large fluctuation in the concentration of SMX in the effluent, and the removal rate of PVDF membrane and PVDF/DA membrane are 83.73% and 85.22%, indicating that the domesticated MBR reactor had a high removal capacity for SMX and could withstand a certain impact load. The results show that the activated sludge system can adapt to the high concentration of SMX by changing its community structure within a certain period, and can degrade it, because PVDF/DA membrane is much better than PVDF membrane in hydrophilicity and roughness so that microorganisms can grow better on the membrane and have a better biological affinity, so the SMX removal effect of PVDF/DA membrane is better than that of PVDF membrane.
During the experimental operation, the COD concentration of the influent water was 346.74–456.33 mg/L, and the COD removal effect of the two membrane modules changed with time as shown in Figure 6. After the operation of stage I, the COD of the effluent of the PVDF membrane and PVDF/DA membrane is stable, and the next concentration experiment is carried out after the stable operation. At the start of stage II, the average removal rate of the PVDF membrane is 93.41%, and the removal rate of the PVDF/DA membrane is 95.07%, and the results showed that even when the antibiotic dosage concentration increased during operation, the final effluent value of the membrane module could still be kept at a low level, and the removal rate was not affected. After the end of stage III, the average removal rate of COD from PVDF/DA membrane effluent reached 95.37%, which was better than 93.93% of PVDF membrane, compared with PVDF membrane, PVDF/DA membrane showed certain interception advantages, but with the extension of running time, the removal rate of PVDF membrane gradually increased, from the overall change trend, in the early stage of start-up, the difference between the two is large, PVDF/DA membrane on COD removal effect is better.
The ammonia nitrogen in the influent water is mainly generated by the NH4Cl added during water distribution, the concentration of NH4+-N in the influent is 15.93–22.54 mg/L, it can be seen from Figure 7 that the removal rate of ammonia nitrogen in PVDF membrane and PVDF/DA membrane reactor in stage I shows an upward trend, the removal rate of the two in stage II (after 15 days) tends to be stable and not much different, the ammonia nitrogen in the effluent is below 2.5 mg/L, the removal rate is above 93%, and the removal rate of PVDF/DA membrane is slightly higher. Mainly because, the removal of NH4+-N is based on aerobic nitrification reaction, the presence of a dopamine coating on the membrane surface does not provide the gain effect of removing NH4+-N.
The change of EPS content of the two membrane modules with time is shown in Figure 8, the cultured mature biofilm is scraped off the membrane surface, three parallel samples are performed, and the sum of protein, polysaccharide, and DNA content is used as the EPS content. It can be seen from Figure 8 that the EPS in the cake layer on the surface of the PVDF membrane increased from 37.75 mg/gVSS to 86.13 mg/gVSS, but the EPS content increased rapidly within 20–30 days, and the changes in protein and polysaccharides also increased to varying degrees. With the extension of the running time, the proportion of polysaccharides increased from 23.89% to 29.44%, and the polysaccharide molecules are easy to form gels, and it was easy to form a filter cake layer on the membrane surface, which improved the resistance of membrane separation. The changing trend of EPS and components of the PVDF/DA membrane was similar to that of the PVDF membrane, but the content of EPS is lower. The EPS in the cake layer on the surface of the PVDF/DA membrane increased from 29.03 mg/gVSS to 62.70 mg/gVSS, and the contents of protein and polysaccharides in the cake layer on the surface of the PVDF membrane are 57.23 mg/gVSS and 25.36 mg/gVSS, respectively, which are 1.35 times and 1.49 times that of PVDF/DA membrane. Proteins bind to the membrane surface due to their amphoteric characteristics by dipole action or in the form of colloidal forming gels, but after DA surface modification, the adsorption capacity of protein molecules is reduced. The EPS content can indirectly indicate the degree of membrane pollution, and it can be seen that the DA coating can slow down membrane fouling.
Through CLSM, fluorescence staining is performed on the contaminated membrane samples, and then the membrane surface contamination is observed more intuitively. Compare Figure 9, after fluorescence staining of the contaminated membrane, the protein and polysaccharide appear green and blue, respectively. Compared with PVDF membrane, PVDF/DA membrane has less protein and polysaccharide attached to the surface, because the water distribution components are easily biodegradable soluble starch and glucose, the microorganisms in the reactor grow vigorously, and the high level of organic pollution makes the PVDF membrane seriously contaminated by the membrane, and at the same time indicates that the pollution degree of PVDF/DA membrane is light.
The main removal mechanism for SMX in MBR under aerobic conditions is biodegradation (Gao et al., 2014). This is mainly because the formation of biofilm on the PVDF/DA membrane. To gain a better understanding of removal mechanisms in MBR, the tentative degradation products (DPs) of SMX were identified by LC-MS/MS (Mohapatra et al., 2023c). Figure 10 shows four main DPs a are identified based on their characteristic peaks at different m/z values. The initial degradation of SMX is due to microbial degradation of the isoxazole ring. In addition, the parent compound SMX mainly undergoes rearrangement of the aminobenzene moiety, cleavage of the S-N bond and isoxazole ring, and loss of the SO2 group, which produce 4-Aminobenzenesulfinic acid (m/z = 156).
FIGURE 10. LC-MS/MS spectra of SMX degradation at 12 h of reaction. (four peaks are identified with retention times in minutes). (A) 1.782, (B) 2.007, (C) 3.241, and (D) 5.7.
The degradation pathway with structures and m/z values is proposed in Figure 11. In the main pathway, the m/z 200.0495 DP was produced from m/z 258.0903 DP through loss of hydroxyl and subsequent C-C bond breaking. Under the action of microorganisms, the DP with m/z 156.0418 was produced by breaking the S-N bond in m/z 200.0495. It is speculated that the second degradation pathway, the C-N bond break of m/z 258.0903 is oxidized to a ketone group to generate m/z 215.0235, which is reduced to hydroxyl groups under the action of aldehyde ketone reductase to generate m/z 201.0079. The DP with m/z 156.0418 was produced by breaking the S-N bond in m/z 201.0079, which is further converted to aniline (m/z = 92) and degraded by Anaerolineae (Wolfson et al., 2018).
The MBR process needs to be domesticated to remove SMX stably and efficiently. At an SMX of 0.5 mg/L, the removal rate increased rapidly after 15 days of acclimation. With the later acclimation with higher concentrations of SMX, the removal rate remained at an average of 85%. The degradation by-products analysis revealed possible SMX degradation pathways with four main oxidation intermediates. Biodegradation was the main removal path of SMX, and the high biodegradation potential of sludge in MBR will reduce the adsorption of antibiotics in sludge, reduce sludge yield and antibiotic contamination, thereby reducing sludge treatment and disposal costs and environmental pollution risks.
The original contributions presented in the study are included in the article/Supplementary Material, further inquiries can be directed to the corresponding authors.
MZ: Investigation, data curation, and writing-original draft; SL: writing-review, funding acquisition and editing; JS: Investigation and validation; JpS: Methodology and resources; LW: Supervision, editing and writing-review; RZ: resources and writing-review. All authors listed have made a substantial, direct, and intellectual contribution to the work and approved it for publication.
This work was kindly supported by the Guangdong Provincial Department of Education, municipal and department-level key science and technology projects (6020210087K), and the Shenzhen Science and Technology Innovation Commission Innovation and Entrepreneurship Project (KCXFZ20201221173203010).
The authors declare that the research was conducted in the absence of any commercial or financial relationships that could be construed as a potential conflict of interest.
The reviewer MH declared a shared affiliation with the authors to the handling editor at the time of review.
All claims expressed in this article are solely those of the authors and do not necessarily represent those of their affiliated organizations, or those of the publisher, the editors and the reviewers. Any product that may be evaluated in this article, or claim that may be made by its manufacturer, is not guaranteed or endorsed by the publisher.
Barnes, K. K., Kolpin, D. W., Furlong, E. T., Zaugg, S. D., Meyer, M. T., and Barber, L. B. (2008). A national reconnaissance of pharmaceuticals and other organic wastewater contaminants in the United States—I) groundwater. Sci. Total Environ. 402 (2-3), 192–200. doi:10.1016/j.scitotenv.2008.04.028
Bartolomeu, M., Neves, M. G. P. M., Faustino, M. A. F., and Almeida, A. (2018). Wastewater chemical contaminants: Remediation by advanced oxidation processes. Photoch Photobio Sci. 17 (11), 1573–1598. doi:10.1039/c8pp00249e
Batt, A. L., Kim, S., and Aga, D. S. (2007). Comparison of the occurrence of antibiotics in four full-scale wastewater treatment plants with varying designs and operations. Chemosphere 68 (3), 428–435. doi:10.1016/j.chemosphere.2007.01.008
Bilal, M., Mehmood, S., Rasheed, T., and Iqbal, H. M. N. (2020). Antibiotics traces in the aquatic environment: Persistence and adverse environmental impact. Curr. Opin. Environ. Sci. Health 13, 68–74. doi:10.1016/j.coesh.2019.11.005
Bungau, S., Tit, D. M., Behl, T., Aleya, L., and Zaha, D. C. (2021). Aspects of excessive antibiotic consumption and environmental influences correlated with the occurrence of resistance to antimicrobial agents. Curr. Opin. Environ. Sci. Health 19, 100224. doi:10.1016/j.coesh.2020.10.012
Carvalho, I. T., and Santos, L. (2016). Antibiotics in the aquatic environments: A review of the European scenario. Environ. Int. 94, 736–757. doi:10.1016/j.envint.2016.06.025
Chaturvedi, P., Giri, B. S., Shukla, P., and Gupta, P. (2020). Recent advancement in remediation of synthetic organic antibiotics from en-vironmental matrices: Challenges and Perspective. Bioresour. Technol. 319, 124161. doi:10.1016/j.biortech.2020.124161
Cheng, D., Ngo, H. H., Guo, W., Chang, S. W., Nguyen, D. D., Liu, Y., et al. (2020). A critical review on antibiotics and hormones in swine wastewater: Water pollution problems and control approaches. J. Hazard Mater 387, 121682. doi:10.1016/j.jhazmat.2019.121682
Felis, E., Kalka, J., Sochacki, A., Kowalska, K., Bajkacz, S., Harnisz, M., et al. (2020). Antimicrobial pharmaceuticals in the aquatic environment - occurrence and environmental implications. Eur. J. Pharmacol. 866, 172813. doi:10.1016/j.ejphar.2019.172813
Gao, S., Zhao, Z., Xu, Y., Tian, J., Qi, H., Lin, W., et al. (2014). Oxidation of sulfamethoxazole (SMX) by chlorine, ozone and permanganate-a comparative study. J. Hazard Mater 274, 258–269. doi:10.1016/j.jhazmat.2014.04.024
Huang, Q., Chen, J., Liu, M., Huang, H., Zhang, X., and Wei, Y. (2020). Polydopamine-based functional materials and their applications in energy, environmental, and catalytic fields: State-of-the-art review. Chem. Eng. J. 387, 124019. doi:10.1016/j.cej.2020.124019
Johnson, A. C., Keller, V., Dumont, E., and Sumpter, J. P. (2015). Assessing the concentrations and risks of toxicity from the antibiotics ciprofloxacin, sulfamethoxazole, trimethoprim and erythromycin in European rivers. Sci. Total Environ. 511, 747–755. doi:10.1016/j.scitotenv.2014.12.055
Kovalakova, P., Cizmas, L., McDonald, T. J., Marsalek, B., Feng, M., and Sharma, V. K. (2020). Occurrence and toxicity of antibiotics in the aquatic environment: A review. Chemosphere 251, 126351. doi:10.1016/j.chemosphere.2020.126351
Lee, H., Dellatore, S. M., Miller, W. M., and Messersmith, P. B. (2007). Mussel-inspired surface chemistry for multifunctional coatings. Science 318 (5849), 426–430. doi:10.1126/science.1147241
Liu, L., Shao, B., and Yang, F. (2013). Polydopamine coating - surface modification of polyester filter and fouling reduction. Sep. Purif. Technol. 118, 226–233. doi:10.1016/j.seppur.2013.07.003
Lu, Z., Ma, Y., Zhang, J., Fan, N., Huang, B., and Jin, R. (2020). A critical review of antibiotic removal strategies: Performance and mechanisms. J. Water Process Eng. 38, 101681. doi:10.1016/j.jwpe.2020.101681
Luo, Y., Guo, W., Ngo, H. H., Nghiem, L. D., Hai, F. I., Zhang, J., et al. (2014). A review on the occurrence of micropollutants in the aquatic environment and their fate and removal during wastewater treatment. Sci. Total Environ. 473-474, 619–641. doi:10.1016/j.scitotenv.2013.12.065
Mohapatra, S., Bhatia, S., Senaratna, K. Y. K., Jong, M., Lim, C. M. B., Gangesh, G. R., et al. (2023a). Wastewater surveillance of SARS-CoV-2 and chemical markers in campus dormitories in an evolving COVID − 19 pandemic. J. Hazard Mater 446, 130690. doi:10.1016/j.jhazmat.2022.130690
Mohapatra, S., Snow, D., Shea, P., Gálvez-Rodríguez, A., Kumar, M., Padhye, L. P., et al. (2023b). Photodegradation of a mixture of five pharmaceuticals commonly found in wastewater: Experimental and computational analysis. Environ. Res. 216, 114659. doi:10.1016/j.envres.2022.114659
Mohapatra, S., Yutao, L., Goh, S. G., Ng, C., Luhua, Y., Tran, N. H., et al. (2023c). Quaternary ammonium compounds of emerging concern: Classification, occurrence, fate, toxicity and antimicrobial resistance. J. Hazard Mater 445, 130393. doi:10.1016/j.jhazmat.2022.130393
Patel, M., Kumar, R., Kishor, K., Mlsna, T., Pittman, C. J., and Mohan, D. (2019). Pharmaceuticals of emerging concern in aquatic systems: Chemistry, occurrence, effects, and removal methods. Chem. Rev. 119 (6), 3510–3673. doi:10.1021/acs.chemrev.8b00299
Phoon, B. L., Ong, C. C., Mohamed, S. M., Show, P. L., Chang, J. S., Ling, T. C., et al. (2020). Conventional and emerging technologies for removal of antibiotics from wastewater. J. Hazard Mater 400, 122961. doi:10.1016/j.jhazmat.2020.122961
Prasannamedha, G., and Sennthil Kumar, P. (2020). A review on contamination and removal of sulfamethoxazole from aqueous solution using cleaner techniques: Present and future perspective. J. Clean. Prod. 250, 119553. doi:10.1016/j.jclepro.2019.119553
Prieto-Rodriguez, L., Miralles-Cuevas, S., Oller, I., Agüera, A., Puma, G. L., and Malato, S. (2012). Treatment of emerging contaminants in wastewater treatment plants (WWTP) effluents by solar photocatalysis using low TiO2 concentrations. J. Hazard Mater 211-212, 131–137. doi:10.1016/j.jhazmat.2011.09.008
Priyanka, M. S., and Mohapatra, S. (2020). Shifts and trends in analysis of contaminants of emerging concern: Sulfonamides. Resilience, response, and risk in water systems. Springer: Springer Transactions in Civil and Environmental Engineering.
Quesada, H. B., Baptista, A., Cusioli, L. F., Seibert, D., Bezerra, C. D. O., and Bergamasco, R. (2019). Surface water pollution by pharmaceuticals and an alternative of removal by low-cost adsorbents: A review. Chemosphere 222, 766–780. doi:10.1016/j.chemosphere.2019.02.009
Radjenovic, J., Petrovic, M., and Barceló, D. (2007). Analysis of pharmaceuticals in wastewater and removal using a membrane bioreactor. Anal. Bioanal. Chem. 387 (4), 1365–1377. doi:10.1007/s00216-006-0883-6
Ryan, C. C., Tan, D. T., and Arnold, W. A. (2011). Direct and indirect photolysis of sulfamethoxazole and trimethoprim in wastewater treatment plant effluent. Water Res. 45 (3), 1280–1286. doi:10.1016/j.watres.2010.10.005
Sabri, N. A., Holst van, S., Schmitt, H., Zaan der van, B. M., Gerritsen, H. W., Rijnaarts, H. H. M., et al. (2020). Fate of antibiotics and antibiotic resistance genes during conventional and additional treatment technologies in wastewater treatment plants. Sci. Total Environ. 741, 140199. doi:10.1016/j.scitotenv.2020.140199
Schaar, H., Clara, M., Gans, O., and Kreuzinger, N. (2010). Micropollutant removal during biological wastewater treatment and a subsequent ozonation step. Environ. Pollut. 158 (5), 1399–1404. doi:10.1016/j.envpol.2009.12.038
Su, T., Deng, H., Benskin, J. P., and Radke, M. (2016). Biodegradation of sulfamethoxazole photo-transformation products in a water/sediment test. Chemosphere 148, 518–525. doi:10.1016/j.chemosphere.2016.01.049
White, D., Lapworth, D. J., Civil, W., and Williams, P. (2019). Tracking changes in the occurrence and source of pharmaceuticals within the River Thames, UK; from source to sea. Environ. Pollut. 249, 257–266. doi:10.1016/j.envpol.2019.03.015
Wolfson, S. J., Porter, A. W., Villani, T. S., Simon, J. E., and Young, L. Y. (2018). The antihistamine diphenhydramine is demethylated by anaerobic wastewater microorganisms. Chemosphere 202, 460–466. doi:10.1016/j.chemosphere.2018.03.093
Xiang, Y., Liu, F., and Xue, L. (2015). Under seawater superoleophobic PVDF membrane inspired by polydopamine for efficient oil/seawater separation. J. Membr. Sci. 476, 321–329. doi:10.1016/j.memsci.2014.11.052
Xiao, K., Liang, S., Wang, X., Chen, C., and Huang, X. (2019). Current state and challenges of full-scale membrane bioreactor applications: A critical review. Bioresour. Technol. 271, 473–481. doi:10.1016/j.biortech.2018.09.061
Yang, L., Wen, Q., Zhao, Y., Chen, Z., Wang, Q., and Burgmann, H. (2019). New insight into effect of antibiotics concentration and process configuration on the removal of antibiotics and relevant antibiotic resistance genes. J. Hazard Mater 373, 60–66. doi:10.1016/j.jhazmat.2019.03.060
Zhang, J., Xiao, K., and Huang, X. (2020). Full-scale MBR applications for leachate treatment in China: Practical, technical, and economic features. J. Hazard Mater 389, 122138. doi:10.1016/j.jhazmat.2020.122138
Keywords: SMX, PVDF/DA membrane, MBR, biodegradation, membrane fouling
Citation: Zhang M, Li S, Sun J, Sun J, Wang L and Zhao R (2023) Effect and degradation pathway of sulfamethoxazole removal in MBR by PVDF/DA modified membrane. Front. Environ. Sci. 11:1111199. doi: 10.3389/fenvs.2023.1111199
Received: 11 January 2023; Accepted: 04 May 2023;
Published: 25 May 2023.
Edited by:
Dunia E. Santiago, University of Las Palmas de Gran Canaria, SpainReviewed by:
Muhammad Hassan, Southwest Jiaotong University, ChinaCopyright © 2023 Zhang, Li, Sun, Sun, Wang and Zhao. This is an open-access article distributed under the terms of the Creative Commons Attribution License (CC BY). The use, distribution or reproduction in other forums is permitted, provided the original author(s) and the copyright owner(s) are credited and that the original publication in this journal is cited, in accordance with accepted academic practice. No use, distribution or reproduction is permitted which does not comply with these terms.
*Correspondence: Shaofeng Li, bHNoYW9mZW5nQHN6cHQuZWR1LmNu; Lei Wang, d2FuZ2xlaWNyYWVzQDE2My5jb20=
Disclaimer: All claims expressed in this article are solely those of the authors and do not necessarily represent those of their affiliated organizations, or those of the publisher, the editors and the reviewers. Any product that may be evaluated in this article or claim that may be made by its manufacturer is not guaranteed or endorsed by the publisher.
Research integrity at Frontiers
Learn more about the work of our research integrity team to safeguard the quality of each article we publish.