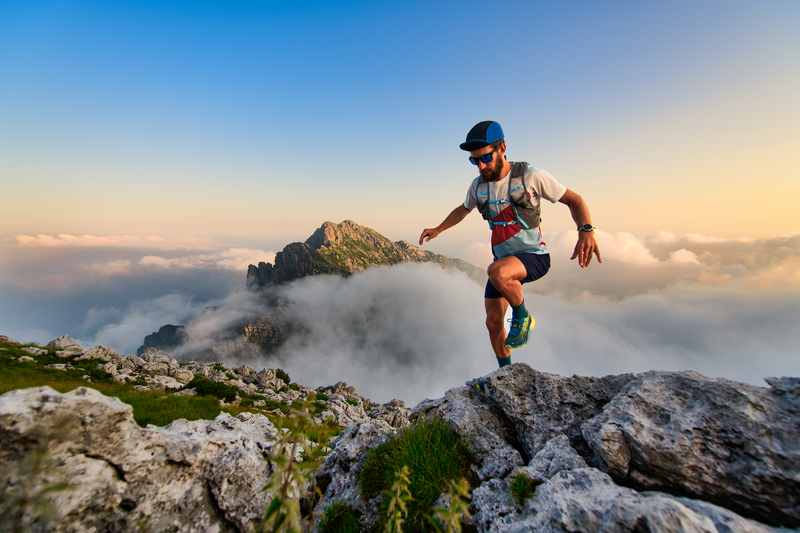
95% of researchers rate our articles as excellent or good
Learn more about the work of our research integrity team to safeguard the quality of each article we publish.
Find out more
ORIGINAL RESEARCH article
Front. Environ. Sci. , 19 June 2023
Sec. Drylands
Volume 11 - 2023 | https://doi.org/10.3389/fenvs.2023.1100552
This article is part of the Research Topic Advances in Soil and Water Management for Dryland Areas View all 8 articles
The olive tree is an iconic tree in the Mediterranean region, traditionally cultivated under rainfed conditions; however, olive cultivars are also found outside the Mediterranean region and are widely used for oil and olive production. However, with the increasing aridity and global changes, olive agroecosystems are facing sustainability challenges. This study aims to evaluate the effect of two deficit irrigation strategies on the agronomic and productive parameters of the Menara, olive cultivar as a tool for operational irrigation water management. For this purpose, an experiment was conducted on an olive orchard for two consecutive years (2021 and 2022), comparing four regulated deficit irrigation (RDI) treatments and two sustained deficit irrigation (SDI) treatments, with fully irrigated trees. The results showed that moderate and controlled water stress under RDI did not significantly affect the yield of the Menara olive cultivar. In addition, by reducing plant water requirement by 20% during sensitive periods and by 40% during normal periods, under RDI, it is possible to save between 25% and 30% of irrigation water and to increase water productivity by 5%–20% with a slight decrease in fruit yield ranging between 10% and 15%. However, the trees subjected to the sustained deficit irrigation strategy exhibited a reduced capacity for shoot growth compared to those under RDI and fully irrigated conditions. Our findings showed that water deprivation during sensitive periods reduced phenological traits and slowed down shoot growth. Furthermore, the water status of the trees was reflected in fruit volume, as a decrease in water supply resulted in a corresponding reduction in fruit volume. Although the study provides important insights into water management strategies for olive cultivation, the short observation period does not allow for long-term plant adaptation evaluation and productivity beyond 2 years. Therefore, it is recommended that future studies extend the observation period to better understand plant adaptation to irrigation regimes.
The olive tree (Olea europaea L.) is an iconic and emblematic fruit tree of the Mediterranean region, with high and valuable cultural and economic significance (Besnard et al., 2018). It belongs to the Olea europaea L. complex, which comprises six olive subspecies distributed across Asia, Africa, and Europe (Medail et al., 2001; Gree, 2002). Of these, only Olea subsp. europaea has been domesticated. Today, both the wild form, O. e. subsp. e. var. sylvestris, known as oleaster, and the cultivated form, O. e. subsp. e. var. sativa, coexist in the Mediterranean Basin (Besnard et al., 2018). The cultivated olive germplasm reflects a large genetic diversity represented by more than 1,200 clonally propagated cultivars grown throughout the Mediterranean region, which accounts for 90% of the globally cultivated area (FAO, 2019). While few of these cultivars are grown in intensive olive systems (Bartolini and Cerreti, 2008), some are grown in restricted areas, especially within traditional agroecosystems (Khadari et al., 2008; Ater et al., 2016). Farmers in various Mediterranean regions have chosen locally adapted varieties, explaining the ongoing process of olive diversification at local and regional scales (Khadari et al., 2008, 2019; El Bakkali et al., 2013; Besnard et al., 2018). Therefore, the observed diversity in olive cultivars is a critical component in preserving and maintaining the productivity of olive-based agroecosystems under various environmental conditions. However, in the face of global changes, it is crucial to understand how olive cultivars will respond to environmental changes, especially drought (Khadari et al., 2019; Kassout et al., 2022). The ability of olive trees to adapt to changing environmental conditions will impact their long-term viability, making this an important area of research.
Olive, an evergreen and sclerophyllous tree species, is well adapted to the Mediterranean climate (Kassout et al., 2021). However, the seasonal variation between hot, dry summers and mild, rainy winters induces stressful conditions for its growth and development (Dichio et al., 2006; Torres-Ruiz et al., 2015). Furthermore, climate change scenarios predict extreme warming and drying trends in the Mediterranean region compared to other climatic ecoregions (Cramer et al., 2018), which are likely to challenge the sustainability of olive-based agroecosystems. The increasing demands for water, for both irrigation and domestic use, may significantly affect the agricultural sector, negatively affecting the growth and productivity of olives (Rallo et al., 2016). Despite the morphological, anatomical, and physiological adaptive traits of the olive tree (Fernandez, 2014; Kassout et al., 2021), the increasing aridity in Mediterranean agroecosystems may potentially interrupt progress toward an efficient olive production sector (Wheeler and Von Braun, 2013; Fraga et al., 2020). Given the key role of the olive sector in the Mediterranean and global food security, sustainable water management practices are urgently required to maintain olive productivity in this era of global changes (Gucci et al., 2019).
Traditional low-density olive farms are the prevailing production systems in Morocco, as in many other Mediterranean regions, with a density range of 50–160 trees/ha. These farms are typically located in arid and semi-arid agricultural lands under rainfed conditions and could be seen as a strategy to cope with low water availability and poor soils (Lorite et al., 2018). However, with the introduction of new management techniques and olive varieties, high-density (350 − 700 trees/ha) and super-density (1,200 − 2,500 trees/ha) olive orchards, which are usually irrigated, have emerged recently (Fernández-Escobar et al., 2013). The sustainability of olive productivity in arid lands, including the Mediterranean region and Morocco, is challenged by the impact of climate change on olive orchards (Lorite et al., 2018). Despite olive trees being regarded as drought-tolerant, water deficit during sensitive periods such as flowering, fruit set, and oil accumulation can cause significant economic losses in olive productivity Moriana et al. (2003); Díaz-Espejo et al. (2018). Thus, optimizing irrigation in olive orchards during sensitive periods can mitigate the impacts of water deficit, hence maintaining profitable olive productivity under arid and semi-arid conditions (Fereres and Soriano, 2007; Fernandes et al., 2018). Numerous studies have explored the impact of restricted irrigation on various olive cultivars, which has generally resulted in water savings but accompanied by yield reductions (Lavee et al., 2007; Servili et al., 2007; Gómez-del Campo, 2013; Caruso et al., 2017; Gucci et al., 2019; Serman et al., 2021). However, adequate deficit irrigation strategies have been shown to maintain profitable olive productivity (Lavee et al., 2007; Gucci et al., 2019). To this end, different deficit irrigation (DI) approaches have been developed to match commercial objectives in the olive sector, including sustained deficit irrigation (SDI) and regulated deficit irrigation (RDI) (Moriana et al., 2003; Iniesta et al., 2009; Ramos and Santos, 2009). SDI involves providing a regular volume of water that is less than the crop evapotranspirative (ETc) demand during the entire irrigation season, while RDI consists of replacing the ETc demand at specific phenological stages that are sensitive to water stress and applying much lower irrigation levels than ETc demand where the stress has no significant impact on yields (Moriana et al., 2003; Fereres et al., 2012). Consequently, RDI is a crucial consideration in arid regions, where water scarcity necessitates the adoption of sustainable water management practices. Previous research (Fernández-Escobar et al., 2013; Gómez-del Campo, 2013; Gucci et al., 2019) has demonstrated that applying moderate RDI to olive trees, especially from fruit drop to the onset of the oil synthesis stage, achieves a balance between water savings, yield, and oil quality. However, implementing RDI strategies during highly sensitive periods can significantly impact the growth and productivity of olive trees (Gucci et al., 2019) and subsequently influence oil quality (García et al., 2020).
The main objective of this study is to identify the most appropriate water irrigation strategy for a new and local grown variety of olives orchards in Morocco, called “Menara”. To achieve this goal, two specific objectives were targeted: i) to evaluate the effect of sustained deficit irrigation and regulated deficit irrigation on the agronomical and productive parameters of the Menara cultivar and ii) to determine the most suitable RDI strategy depending on different phenological stages.
An irrigation experiment was conducted in an olive orchard over 2 years, 2021 and 2022, to compare the effect of RDI and SDI at different phenological stages with fully irrigated (FI) trees. The study was carried out on 12-year-old trees of the Menara cultivar planted in 2011 at the research station of Saâda (31°37′33.6″ N, 8°08′45.6″ W, and 411 m a.s.l.) managed by the National Institute of Agronomic Research of Marrakech (Morocco). The trees are planted in a squared spaced scheme of 8 m of side length, under drip irrigation, with a density of 156 trees/ha, as shown in Figure 1. The experiment included seven different treatments replicated for two successive years on 18 trees per treatment. The soil at the experimental site had a clayey texture, pH of 7.82, and organic matter content of 2.33%. No fertilizers or pesticides were used, and weeding was performed manually.
FIGURE 1. Aerial view of the research station, with the trees subjected to the experiment highlighted in green. The black square on the right represents the irrigation basin, which has a side length of 30 m.
The climate at the study site is Mediterranean, characterized by an arid or semi-arid climate with hot and dry summers, low rainfall, and spatiotemporal irregularity of precipitation (Er-Raki et al., 2010; Sebbar et al., 2011). These factors, along with climate change effects and water shortage, significantly limit the crop yield. According to the Köppen climate classification, the climate subtype at the study site is either “BSh” (hot semi-arid) or “BWh” (hot arid). Most of the annual rainfall occurs between late November and March, with very little precipitation during the summer period, as shown in the climatograph depicted in Figure 2.
FIGURE 2. Climatograph of the study site, showing monthly climatic data from 1990 to 2019. The red line represents temperature, and the blue bars represent monthly precipitation. The data source is cited by Zepner et al. (2020).
The cumulative reference evapotranspiration (ETo), which represents the climatic demand, between 1st January and 10th October, was 1295.7 and 1113.7 mm in 2021 and 2022, respectively. However, the cumulative precipitation (P) during the same period was only 112.6 and 75 mm in 2021 and 2022, respectively. This confirms that the second year (2022) of the experiment was affected by drought.
The irrigation requirements were calculated daily using the standard FAO56 formula for crop evapotranspiration (ETc = ETo ⋅ Kc ⋅ Kr), where the daily reference evapotranspiration ETo was estimated using the Penman–Monteith equation (Allen et al., 1998, 2006). Daily ETo values (Figure 3) were calculated using daily climatic data measured by a standard meteorological station (model iMETOS, Pessl instruments) installed on the experimental site. The crop coefficient Kc was estimated to be 0.65 in April and October; 0.6 in May and June; 0.55 from July to September; and 0.7 in November and December (Orgaz et al., 2007). The coefficient reduction Kr, which is related to the degree of orchard floor plant cover and applied to canopies that covered less than 50% of the ground, was calculated as Kr = 2C/100, where C is the percentage of canopy cover.
FIGURE 3. Daily meteorological data from the study site during the experiment. The records start on 4 January 2021 and end on 5 October 2022.
The control treatment, T0, involved full irrigation to meet the crop evapotranspiration (ETc), demand throughout the irrigation season, which was divided into the sensitive period (SP) and normal period (NP). The first SP extended from flower differentiation to the beginning of pit hardening, the NP covered the phase of pit hardening, and the second SP spanned from oil accumulation to harvest.
The study included four RDI treatments, which consisted of the following four water regimes: T1 (SP 100—NP 70% ETc); T2 (SP 100—NP 60% ETc); T3 (SP 80—NP 70% ETc); and T4 (SP 80—NP 60% ETc). Trees under T1 and T2 were fully irrigated during the SP, while they received 70% and 60% of ETc during the NP, respectively. Trees under T3 and T4 received 80% of ETc during SP and 70% and 60% of ETc during the NP.
Two SDI treatments were also applied, which kept the plants irrigated throughout the season with a fixed percentage of the ETc. The first regime, T5, maintained irrigation at 70% of the ETc, while the second regime T6 maintained irrigation at 60% of the ETc.
Table 1 summarizes the total amount of water delivered to the Menara trees for each treatment. Irrigation amounts were adjusted based on weather conditions and effective water deficit.
TABLE 1. Summary of different irrigation treatments and total water delivered in 2021 and 2022. The total water delivered may vary annually based on ETc and phenological phases, as summarized in Table 2, and water deficits. The percentage of the total applied water relative to the control treatment for each year is shown in parentheses.
Phenological development, including mean budburst, full flowering, pit hardening, and maturity, was recorded in both years following the method described by Sanz-Cortés et al. (2002). The periods are given in Table 2.
TABLE 2. Periods of different phenological phases characterizing the sensitive period (SP) and normal period (NP) in 2021 and 2022.
To characterize the effect of different treatments on tree growth and development, vegetative growth was measured on six representative trees per treatment at weekly intervals during the vegetative growth season, from bud break until the beginning of July. Four current-year shoots per tree were selected for measurement at 1.5 m above the ground below the insertion of the first-year branch. Hence, productive-related parameters were monitored for each of the six selected trees per treatment, and 1-year old shoots were selected and marked. The following measurements were undertaken: shoot length (SL, cm), the number of buds (BUN, buds per shoot), buds initiated (BI, inflorescences per buds), flowers per inflorescence at the white button stage just before flowering (FPI), and the number of fruits per shoot (FNS). The flowering rate (FR) was expressed as the percentage of buds developed into inflorescences. Additionally, fruit yield (FY, kg/tree), fruit weight (FW, g), fruit volume (FV, mL), and oil content (%) were measured for each treatment. FV was measured using the water displacement method, which consists of measuring the difference in the water volume in a graduated cylinder filled with water before and after fruit immersion. Oil content was measured using a laboratory scale system within 24 h of harvesting. Fruits were crushed using a hammer mill, and the resulting olive paste was homogenized and dried at 70°C; then, using a Soxhlet apparatus and 30°C hexane, the oil content was measured (Donaire et al., 1977).
Statistical differences among irrigation treatments for each season were estimated using ANOVA tests for all evaluated parameters. Tukey’s test was used to identify significant differences between the means (p < 0.05). Descriptive statistics, such as the mean, standard deviation, and standard error, were calculated to explore variation in the measured variables obtained from different treatments. The coefficient of variation was also calculated to determine the amplitude of variation of the measured variables under different treatments, which is expressed as 100 ⋅ σ/|μ|. To test for statistical differences between the treatments and measured variables, ANOVA was used, followed by Tukey HSD post hoc tests for inter-treatment comparisons with a significance level of p = 0.05.
To test for differences between the studied years, we conducted a t-test between the years and for each variable. Additionally, a variance decomposition analysis was conducted (Kassout et al., 2019) using R software to explore the four organizational scales within the experiment: 1) “years” (variation between different years); 2) “treatments” (variation between different irrigation treatments); 3) “inter-trees” (variation between different trees within the same treatment); and 4) “intra-trees” (variation between different parts of the same tree). The “lme” function in the “nlme” package (Pinheiro et al., 2018) was used to fit a general linear model using the restricted maximum likelihood (RMEL) method across the studied levels. Then, the “varcomp” function in the “ape” package (Paradis et al., 2004) was used to extract the variance expressed at each level. This approach was used to assess the variability for growth and phenological variables, which were measured at the tree level with multiple repetitions.
Table 3 summarizes the results showing that RDI, SDI, and the volume of applied water had a significant impact on vegetative growth. Differences in growth rates were observed during the vegetative growth season, which occurred from March to July. The shoot length of trees from T0 was approximately 9.3 cm in 2021 and 8.9 cm in 2022. ANOVA, assuming α = 0.05, demonstrated significant differences in the average vegetative growth between irrigation treatments in both years with p < 0.001. The most significant reduction in vegetative growth was observed for T6, where the final length of the shoots decreased by approximately 22% in 2021 and by 40% in 2022 compared to T0 and T1.
TABLE 3. Vegetative growth at the end of the growing period (7 July 2021 and 15 July 2022) (average and standard error (S.E.)) and its percentage of variation for each treatment compared to T0. The obtained results are statistically significant with p < 0.001. The measurements were taken on current-year shoots. Here, in Figure 4, and in Tables 5–8, the same letters indicate no significant differences between irrigation treatments within the group marked by the same letter, according to Tukey’s test.
The results showed that deficit irrigation had a significant effect on both the phenological and growth-related traits across all treatments. Significant differences were observed among the Menara trees for all studied parameters except for SL and BUN in the first year (2021), as shown in Figure 4. In 2022, the ANOVA test indicated significant differences in the studied parameters among treatments with p < 0.001. SL was higher in 2022 than in 2021 for both the control and RDI treatments, and this increase was attributed to the bud number per shoot. Overall, a significant difference in fruit number per shoot between irrigation treatments was observed in 2022. The fruits per shoot in FI and RDI treatments were significantly higher than in SDI treatments. Moreover, according to the findings given in Figure 4, in 2022, flowering parameters, notably, BUN, FPI, and FR, were found to be lower in trees under SDI than those subjected to RDI and FI.
FIGURE 4. Plots of the average values of the investigated parameters, with error bars representing the standard deviation. In 2022, the ANOVA test indicated highly significant differences in the studied parameters among treatments with p <0.001. The same letters indicate the absence of significant differences between irrigation treatments within the group marked by the same letter, according to Tukey’s test. Fully irrigated (T0) and SDI (T5 and T6) treatments are represented with different bar textures.
Generally, the percentage variance was high at the intra-tree level for all variables, ranging from 39.54% to 65.81% (Table 4). Shoot length explained the highest proportion of variance between years (25.11%); meanwhile, the flowering rate expressed the lowest value (1.42%). In addition, flowers per inflorescence (FPI) exhibited an important proportion of variance at the inter-tree level (25.13%), between years (18.42%), and at the treatment level (9.89%). In addition, the flowering rate (FR) and fruit number per shoot (FNS) explained an important amount of variance at the inter-tree level, with 15.97% and 17.30%, respectively.
TABLE 4. Estimated percentage of variance across the studied hierarchical levels included in the experimental design of the study for growth and phenological parameters. Maximum values are in bold.
Table 5 shows that the applied irrigation treatments significantly influenced the fruit yield in terms of Kg/tree. In 2021, the parameter decreased significantly in the range of 10%–25%, but it later increased significantly for T1 and T2 and decreased significantly for T5 and T6 in 2022. In 2022, it is worth mentioning that fruit yield was not significantly affected for T3 and T5 treatments, despite implementing water saving by 20% during the SP, 30% during the NP in T3, and 30% throughout the growing season in T5 compared to FI treatment in 2021. The ANOVA test, assuming that α = 0.05, showed a significant variation in the average fruit yield between irrigation treatments in 2021 with p < 0.01 and an extremely significant difference in 2022 with p < 0.001. This difference can be attributed to the fact that 2022 was drier than 2021.
TABLE 5. Fruit yield (average and standard error (S.E.)) and its percentage of variation for each treatment compared to T0. The ANOVA test indicated a significant difference among treatments with p < 0.01 in 2021 and p < 0.001 in 2022.
The fruit volume was significantly affected by the RDI and SDI treatments. ANOVA, assuming that α = 0.05, showed an extremely significant variation in the average fruit volume between irrigation treatments in both years with p < 0.001, as shown in Table 6. It is interesting to note that for T1, although the yield per tree increased, the average fruit volume decreased.
TABLE 6. Fruit volume (average and standard error (S.E.)) and its percentage of variation for each treatment compared to T0. The obtained results are statistically significant with p < 0.001.
Fruit weight was significantly influenced by irrigation treatments. ANOVA showed significant differences in the average fruit weight between irrigation treatments in both years with p < 0.001, as given in Table 7. Compared to T0, the fruit weight for almost all the other treatments decreased by 15%–25%, with the exception of T6 in 2022 where it decreased by an exceptional 45%.
TABLE 7. Fruit weight (average and standard error (S.E.)) of 100 drupes and its percentage of variation for each treatment compared to T0. The obtained results are statistically significant with p < 0.001.
The fruit oil content, measured as the percentage of dry weight, was significantly affected by water deficit. ANOVA, assuming that α = 0.05, showed an extremely significant variation of the average fruit oil content between irrigation treatments in 2021 with p < 0.001. Compared to T0, the oil content decreased by 5% for T5, 10% for T1 and T4, and 20% for T2 and T3, but remained unchanged for T6. In contrast to 2021, in 2022, a significant increase in the oil content was observed for fruits from trees subjected to SDI treatment, as well as for T3 and T4 subjected to RDI treatment. The detailed results are given in Table 8.
TABLE 8. Oil content over dry weight expressed as a percentage (average and standard error (S.E.)) and its percentage of variation for each treatment compared to T0. The obtained results are statistically significant with p < 0.001.
To evaluate water productivity (WP) in terms of fruit yield, measured in kg/mm, with respect to T0, fruit yield (FY) for each treatment was divided by the percentage of the total water delivered with respect to T0 (Table 1). The results obtained are shown in Table 9, which indicate that the applied irrigation treatments significantly influenced WP. The SDI strategy showed the highest water productivity during the first season (2021), with significant water savings, whereas during the second season of the experiment (2022), this strategy reduced water productivity, which might be due to the prolonged effects of stress in perennial crops. Among the RDI treatments, T3 showed the highest water productivity, with an average increase of 17% over the 2 years of the experiment compared to T0.
TABLE 9. Variation of water productivity in terms of fruit yield compared to T0. “FY % T0” indicates the percentage variation of fruit yield with respect to T0. “Total water % T0” indicates the percentage of total water delivered according to T0, and “% Var. WP” indicates the percentage variation of water productivity.
The results obtained from the experiment showed that different irrigation treatments significantly affect all the studied traits of the Menara olive cultivar, leading to an important variability at the intra- and inter-tree levels, and thus, across years (Table 4). Generally, the Menara cultivar responds quickly to RDI and SDI treatments in both years of the experiment. The response of the cultivar to treatment is characterized by a reduction in the fruit yield of around 20% and a reduction in the fruit oil content of 5% and 10% in the first year, followed by an increase in value up to 15% in the second year. In contrast, other varieties evaluated over years in the RDI treatment showed no significant difference in fruit yield at the end of the third year (Pierantozzi et al., 2020).
Water deficit is a critical stressor that affects plant growth and development. It is also the most significant factor that limits olive yield (Brito et al., 2019). Our findings suggest that the Menara tree’s response to RDI and SDI treatments was influenced by both the effect of treatments and varying seasons. The growth rate of Menara trees varied among treatments, with vegetative growth responding differently to applied water deficits. Analysis of the experimental years showed that deficit irrigation treatments significantly reduced shoot growth. Vegetative shoot growth started toward the end of winter and was significantly reduced by deficit irrigation (Pierantozzi et al., 2020). Water availability is a crucial limiting factor for trees, with significant consequences on ecophysiological processes, and the growth potential is substantially affected by water stress in trees, such as in the case of the olive tree (Pierantozzi et al., 2013; Steppe et al., 2015; Kassout et al., 2021). Therefore, shoot growth in Menara trees was significantly lower under DI treatment compared to FI trees. Furthermore, the growth capacity of trees subjected to RDI treatment was higher than those subjected to SDI treatment, indicating that the RDI strategy might be a more suitable irrigation method for promoting shoot growth in trees (Table 3). Correa-Tedesco et al. (2010) showed that fully irrigated trees subjected to a warm climate exhibited significant shoot growth. In the case of the studied Menara trees, analysis results of Student’s t-test showed that after two consecutive years, vegetative growth was significantly affected by the enduring effects of treatments T3 and T4 under RDI and T6 under SDI, where olive Menara trees may not have enough water to support optimal vegetative growth (Supplementary Table S1). Trentacoste et al. (2019) observed a significant reduction in vegetative growth in the “Arbosana” olive cultivar subjected to different RDI treatments over three consecutive seasons compared to those with full irrigation. Nevertheless, these trees still yielded significant water savings and a substantial increase in fruit yield. Moreover, reduced vegetative growth can be beneficial in super-intensive olive orchards as it can improve canopy and fruit illumination (Gomez-del Campo et al., 2017; Trentacoste et al., 2019).
Our analysis of phenological variability at different hierarchical levels revealed significant intra-tree variability, ranging from 40% for FPI to 66% for FR (Table 4). This high variability between trees is not negligible and has been observed previously in leaf- (Kassout et al., 2019) and wood-related traits (Kassout et al., 2021) in wild olive trees in Morocco. This variability was attributed to differences in water availability at multiple scales, from regional to local. The substantial variability observed between the study years (Table 4) suggests that environmental conditions may also play a significant role in shaping the variability of the studied traits (Siefert et al., 2015). Therefore, the observed variability in phenological traits at different hierarchical levels could be attributed to the complex interactions between genetic and environmental factors (Kassout et al., 2021).
The influence of irrigation treatments included in our study on “Menara” tree fruit yield, fruit weight, and volume, as well as fruit oil content, has been well documented. Previous studies have reported that irrigated olive trees produce higher yields and better fruit characteristics than non-irrigated ones (Moriana et al., 2003; Lavee et al., 2007). In our research, Menara trees under RDI treatment exhibited a higher yield in 2022 than in 2021, while those under SDI treatment had a lower yield in 2022 than in 2021. The SDI treatments resulted in significant water savings, with the trees receiving only 70% and 60% of the water supplied to FI throughout the growing season, causing a decline in fruit yield by more than 50% in 2022. Correa-Tedesco et al. (2010) found that applying 66% ETc over the growing season in a table olive cultivar Manzanilla fina led to mild water stress, which reduced fruit yield by 25% compared to well-watered trees. Fruit volume was significantly influenced by the irrigation treatments, with significant differences observed in both years. The fruit volume was directly related to the water status of the trees, with less water supplied leading to smaller olive fruit. For table olive cultivars, fruit size and yield are critical parameters (Alcaras et al., 2021). The individual fruit volume in trees under the T1 irrigation treatment was smaller in 2022 due to the high fruit load during that season. Grappadelli and Lakso (2004) reported that fruit size was influenced and regulated by water availability and crop load. Crop load can significantly decrease fruit size in well-irrigated or deficit-irrigated trees (Gucci et al., 2007). The fruit volume of Menara trees was also significantly influenced by the year, with p < 0.0001 in T1, T2, and T3 for the RDI strategy. Additionally, FV decreased significantly with p < 0.01 in T6 treatment with the poorest water status during the irrigation season (Supplementary Table S8). Significant differences were also found in fruit weight according to the applied DI treatments (Table 7). The year was a significant factor (p < 0.0001) influencing fruit weight (Supplementary Table S9) for T6 treatment. The volume of water applied significantly affected fruit oil content. The relationship between oil content and irrigation regimes was weak during the first year of the experiment (2021). However, in 2022, oil content increased significantly with decreasing irrigation water. Therefore, fruit oil content is assumed to be lower under irrigation due to competition between oil and water in cellular spaces because of their opposite polarity (Mazliak, 1968). The SDI, T3, and T4 irrigation regimes did not alter the oil accumulation process, confirming results from previous investigations (Gucci et al., 2007; Trentacoste et al., 2018, 2019). Furthermore, previous studies (Dag et al., 2009; Ben-Gal et al., 2021) have shown that increasing water stress could enhance oil quality. Therefore, the observed increase in oil content under water stress in olive cultivars is well documented (Gomez-del Campo, 2010; Ramos and Santos, 2010; Trentacoste et al., 2019). In fact, full irrigation was not required to achieve maximum fruit oil content in the “Menara” cultivar.
The studied parameters were significantly affected by the availability of water during sensitive stages. The Menara cultivar showed differences between RDI and SDI treatments regarding the intensity and timing of water deficit, particularly during sensitive phenological stages. Table 3 shows that Menara trees under SDI treatment exhibited less shoot growth capacity than trees under RDI treatment. Our observations indicate that trees subjected to RDI treatment suffered less from water stress during sensitive phenological stages (100% or 80% of estimated ETc) in the spring–early summer period when growth performance is more critical. This trend can be explained by the stimulation of shoot growth under RDI treatment, which is an evident response caused by the increase in transpiration (Mahhou et al., 2005). Several reversible mechanisms, including an increase in cell division speed (Granier et al., 2000), elasticity of cellular wall, facilitated cell growth (Cosgrove, 2005), and increased cell turgor (Bouchabke et al., 2006), contribute to its occurrence. Providing full or elevated levels of water availability (100% or 75% of estimated ETc) during the spring months, according to Pierantozzi et al. (2013), can protect photosynthetic pigments from oxidative degradation and significantly improve CO2 assimilation rates. The tree growth and development and, consequently, the physiological functioning appeared to be maintained through these sensitive periods (Hernandez-Santana et al., 2017). However, previous studies have shown that water deficit during spring can reduce shoot growth (Palese et al., 2010; Hueso et al., 2021). Important physiological events determine shoot growth and various flowering parameters during spring, such as flower differentiation, flowering, and fruit number. During the second year of the experiment, non-significant differences were observed in shoot length and bud number as a result of reduced water application due to the RDI strategy. Rapoport et al. (2012) found that water deficit during inflorescence development led to a reduction in various flowering traits. In our study, we observed that water deprivation during sensitive periods through the SDI strategy resulted in reduced phenological traits and slowed down shoot growth. Hueso et al. (2021) reported significantly lower numbers of flower buds in rainfed and 40% ETc of “Arbequina” trees than those in fully irrigated trees. However, we observed only a slight decrease when comparing RDI and FI treatments, which may be due to the set of flowering parameters before the reduction in irrigation during the normal period (Rapoport et al., 2012). Conversely, Menara trees under SDI treatment experienced water stress, negatively affecting their flowering-related traits, even before the flower differentiation period, confirming the effect of RDI and irrigation scheduling on the studied parameters. Drought could highly impair fruit set and subsequent lower yield due to the increased presence of imperfect flowers in olive trees. In contrast, adequate irrigation during flower initiation and induction improved perfect flowers and, consequently, fruit yield in olive trees (Tadayon and Hosseini, 2023). Applying irrigation during early spring can prevent severe physiological disturbances such as low floral development (Cuevas et al., 1997; Crous, 2009) and significantly enhance fruit growth kinetics even during the stone-hardening period, where fruit growth is usually slowed (Manrique et al., 1997). These findings suggest that trees subjected to RDI treatment were more resilient to water stress than those under SDI treatment. Furthermore, trees can better tolerate deficit irrigation during periods when productivity-determining processes are less sensitive to water stress (Gucci et al., 2019). Trentacoste et al. (2019) reported that fruit number was reduced by water deficit when applying deficit irrigation from spring to early summer. According to Pierantozzi et al. (2013), trees that did not receive any irrigation experienced a delay in flowering, and this delay, coupled with a decrease in flowering intensity, resulted in lower oil yield in the moderately and severely water-stressed treatments compared to the well-irrigated trees. Reduced soil moisture during inflorescence formation and flower development resulted in fewer flowers per inflorescence, as reported by Hartmann and Panetsos (1961).
The results of Student’s t-test analysis for shoot length and fruit yield revealed significant differences across the years for all RDI treatments and T5 treatments in the SDI treatment, as presented in Supplementary Tables S2, S7. In 2022, fruit yield significantly increased for the RDI treatments but decreased significantly for T5, indicating that olive production decreased significantly under water stress during the sensitive period. Furthermore, across seasons, all RDI regimes had a significant effect on bud number, as presented in Supplementary Table S3. Similar findings were reported by Moriana et al. (2003), who showed that RDI treatment increased the yield compared to SDI, where the latter showed a significant reduction in agronomical parameters. Likewise, moderate RDI was found to increase the fruit yield in high-density orchards (Rosecrance et al., 2015) and provide similar or greater production than full irrigation with less water usage, resulting in significant water savings (Fernández-Escobar et al., 2013). However, negative effects of RDI have been observed in other fruit trees after two to three consecutive seasons of deficit irrigation, where negative carryover effects on the yield of peach trees were observed after 3 consecutive years of RDI treatment (Girona et al., 2003). The negative effects were due to a reduction in floral density and fruit set, resulting from a decreased accumulation of reserves in RDI trees. Hence, optimizing irrigation is crucial for maximizing crop productivity while minimizing water usage. In 2022, reducing irrigation volumes by 30% during the normal period resulted in fruit weight similar to control trees, while deficit treatments at 60% of crop evapotranspiration during the NP significantly decreased the fruit weight compared to T0. This result could be due to differences in water stress intensity, which was slight in T1. Lavee et al. (2007) suggested that irrigation influenced fruit characteristics and recommended supplying all the water volume after pit hardening. Additionally, Lodolini et al. (2011) reported that different olive fruit tissues and components respond differently to water stress, depending on their different sensitivities in the phases in which deficit irrigation is applied and their capacity to recover once the olive tree is fully irrigated. Water stress during the first phase of fruit growth produced smaller pits that could lead to higher pulp-to-pit ratios but could also reduce fruit size and number (Lavee and Nir, 1986; Rapoport et al., 2004). However, the endocarp size of the stressed plants was similar to the control plants once the stress was relieved, as reported by these authors. Under more prolonged stress, the endocarp size remained smaller. Therefore, the problems in deficit irrigation of perennial crops may be the prolonged effects of stress that last longer than the current season and often become detrimental in the following years (Gucci et al., 2019).
An irrigation strategy should aim to balance high crop production with high water productivity. For olive oil producers practicing deficit irrigation, measuring crop water productivity (WP), which is the fruit yield per unit of water consumed, is a useful indicator for assessing the impact of irrigation scheduling protocols. Table 9 shows that the T3 and T4 treatments were the most suitable strategies for maximizing water productivity in both studied years. However, WP was reduced with the SDI strategy in 2022. It is important to note that attempting to maximize WP through SDI, in which trees received only 70% and 60% of the water supplied to fully irrigated trees throughout the growing season, resulted in a significant reduction in crop yield. Therefore, although improving water use efficiency is critical for producers, it should not be at the expense of yield as it is the primary determinant of producers’ income (Gucci et al., 2019). Additionally, Trentacoste et al. (2019) reported higher productivity in some deficit treatments in the spring.
In conclusion, this study investigated the effects of two different irrigation strategies (RDI and SDI) on the growth and yield of Menara olive trees over 2 years. The results indicated that RDI treatment was a viable strategy for improving the acclimation of trees to water stress, as evidenced by the better performance of trees under RDI treatment in terms of vegetative growth, bud number, and fruit yield. However, prolonged exposure to deficit irrigation can have a cumulative negative effect on crop performance, as observed in T6 under SDI treatment. These findings highlight the importance of the careful management of irrigation strategies and consideration of long-term effects on crop performance. Moreover, these findings can provide valuable insights for farmers and policymakers in implementing effective irrigation management practices for sustainable crop production. Future research should aim to optimize irrigation strategies to improve both short-term and long-term crop performance while also taking into account environmental and economic sustainability. Hence, further research is required to better understand the ecophysiological mechanisms underlying the response of olive trees to deficit irrigation and to identify the optimal irrigation strategies for maximizing crop yield and quality while minimizing water use.
The limitations of this study are primarily related to the short observation period, which does not provide a complete understanding of the long-term adaptation and productivity of the plant beyond 2 years. Extending the study period to observe the adaptation of the plant to the irrigation regime is a natural progression of this research. Although there are studies on different cultivars, they are limited to 2 or 3 years of observation. Therefore, it is essential to conduct long-term studies to fully comprehend the effects of deficit irrigation on the agronomic productivity and adaptation of olive cultivars.
The data supporting the conclusion of this article will be made available by the authors under reasonable request.
KI, SE, SO, LM, AB, and RH contributed to the conception and design of the study. KI, AB, LS, and RH collected the data and organized the database. KI, JK, and VB performed the statistical analysis. KI, JK, VB, and RH wrote the first draft of the manuscript. KI, AB, SE, RH, JK, and VB wrote sections of the manuscript. All authors contributed to the article and approved the submitted version.
This is a short text to acknowledge the contributions of the Institut National de la Recherche Agronomique (INRA) who provided the physical space, plants and technical staff for the construction of the study. The authors thank Sarah Hadden for the English improvements on a previous version of the manuscript.
The authors declare that the research was conducted in the absence of any commercial or financial relationships that could be construed as a potential conflict of interest.
All claims expressed in this article are solely those of the authors and do not necessarily represent those of their affiliated organizations, or those of the publisher, the editors, and the reviewers. Any product that may be evaluated in this article, or claim that may be made by its manufacturer, is not guaranteed or endorsed by the publisher.
The Supplementary Material for this article can be found online at: https://www.frontiersin.org/articles/10.3389/fenvs.2023.1100552/full#supplementary-material
Alcaras, L. M. A., Rousseaux, M. C., and Searles, P. S. (2021). Yield and water productivity responses of olive trees (cv. Manzanilla) to post-harvest deficit irrigation in a non-Mediterranean climate. Agric. Water Manag. 245, 106562. doi:10.1016/j.agwat.2020.106562
Allen, R. G., Pereira, L. S., Raes, D., and Smith, M. (1998). Crop evapotranspiration: Guidelines for computing crop water requirements. Rome, Italy: FAO Irrigation and Drainage. Paper 56, 17–28.Fao penman–monteith equation.
Allen, R. G., Pruitt, W. O., Wright, J. L., Howell, T. A., Ventura, F., Snyder, R., et al. (2006). A recommendation on standardized surface resistance for hourly calculation of reference ETo by the FAO56 Penman-Monteith method. Agric. water Manag. 81, 1–22. doi:10.1016/j.agwat.2005.03.007
Ater, M., Barbara, H., and Kassout, J. (2016). “Importance des variétés locales, de l’oléastre et des pratiques traditionnelles de l’oléiculture dans la région de Chefchaouen (Nord du Maroc),” in L ‘oléiculture au maroc de la préhistoire à nos jours: Pratiques, diversité, adaptation, usages, commerce et politiques (Montpellier (France): CIHEAM), 110–121.
Bartolini, G., and Cerreti, S. (2008). Olive germplasm (Olea europaea L), cultivars, synonyms, cultivation area, collections, descriptors. Available at: http://www.oleadb.it
Ben-Gal, A., Ron, Y., Yermiyahu, U., Zipori, I., Naoum, S., and Dag, A. (2021). Evaluation of regulated deficit irrigation strategies for oil olives: A case study for two modern Israeli cultivars. Agric. Water Manag. 245, 106577. doi:10.1016/j.agwat.2020.106577
Besnard, G., Terral, J.-F., and Cornille, A. (2018). On the origins and domestication of the olive: A review and perspectives. Ann. Bot. 121, 385–403. doi:10.1093/aob/mcx145
Bouchabke, O., Tardieu, F., and Simonneau, T. (2006). Leaf growth and turgor in growing cells of maize (zea mays l) respond to evaporative demand under moderate irrigation but not in water-saturated soil. Plant, Cell & Environ. 29, 1138–1148. doi:10.1111/j.1365-3040.2005.01494.x
Brito, C., Dinis, L.-T., Moutinho-Pereira, J., and Correia, C. M. (2019). Drought stress effects and olive tree acclimation under a changing climate. Plants 8, 232. doi:10.3390/plants8070232
Caruso, G., Gucci, R., Sifola, M. I., Selvaggini, R., Urbani, S., Esposto, S., et al. (2017). Irrigation and fruit canopy position modify oil quality of olive trees (cv. Frantoio). J. Sci. Food Agric. 97, 3530–3539. doi:10.1002/jsfa.8207
Correa-Tedesco, G., Rousseaux, M. C., and Searles, P. S. (2010). Plant growth and yield responses in olive (Olea europaea) to different irrigation levels in an arid region of Argentina. Agric. Water Manag. 97, 1829–1837. doi:10.1016/j.agwat.2010.06.020
Cosgrove, D. J. (2005). Growth of the plant cell wall. Nat. Rev. Mol. Cell Biol. 6, 850–861. doi:10.1038/nrm1746
Cramer, W., Guiot, J., Fader, M., Garrabou, J., Gattuso, J.-P., Iglesias, A., et al. (2018). Climate change and interconnected risks to sustainable development in the Mediterranean. Nat. Clim. Change 8, 972–980. doi:10.1038/s41558-018-0299-2
Cuevas, J., Pinney, K., and Polito, V. (1997). Flower differentiation, pistil development and pistil abortion in olive (olea europaea l’manzanillo’). III Int. Symposium Olive Grow. 474, 293–296. doi:10.17660/actahortic.1999.474.59
Dag, A., Bustan, A., Avni, A., Lavee, S., and Riov, J. (2009). Fruit thinning using NAA shows potential for reducing biennial bearing of ‘Barnea’ and ‘Picual’ oil olive trees. Crop Pasture Sci. 60, 1124–1130. doi:10.1071/cp09090
Díaz-Espejo, A., Fernandez, J. E., Torres-Ruiz, J. M., Rodriguez-Dominguez, C. M., Perez-Martin, A., and Hernandez-Santana, V. (2018). “The olive tree under water stress: Fitting the pieces of response mechanisms in the crop performance puzzle,” in Water scarcity and sustainable agriculture in semiarid environment (Amsterdam, Netherlands: Elsevier), 439–479.
Dichio, B., Xiloyannis, C., Sofo, A., and Montanaro, G. (2006). Osmotic regulation in leaves and roots of olive trees during a water deficit and rewatering. Tree Physiol. 26, 179–185. doi:10.1093/treephys/26.2.179
Donaire, J. P., Sanchez Raya, A. J., Lopez Gorge, J., and Recalde, L. (1977). Études physiologiques et biochimiques de l’olive: I. Variations de la concentration de divers métabolites pendant son cycle évolutif. Agrochimica 21, 311–321.
El Bakkali, A., Haouane, H., Hadiddou, A., Oukabli, A., Santoni, S., Udupa, S. M., et al. (2013). Genetic diversity of on-farm selected olive trees in Moroccan traditional olive orchards. Plant Genet. Resour. 11, 97–105. doi:10.1017/s1479262112000445
Er-Raki, S., Chehbouni, A., Khabba, S., Simonneaux, V., Jarlan, L., Ouldbba, A., et al. (2010). Assessment of reference evapotranspiration methods in semi-arid regions: Can weather forecast data be used as alternate of ground meteorological parameters? J. Arid Environ. 74, 1587–1596. doi:10.1016/j.jaridenv.2010.07.002
Food and Agriculture Organization of the United Nations (2019). FAOSTAT statistical database. https://www.fao.org/faostat/fr/#home
Fereres, E., Goldhamer, D., and Sadras, V. (2012). Yield response to water of fruit trees and vines: Guidelines. Rome, Italy: FAO Irrigation and Drainage Paper, 246–497.
Fereres, E., and Soriano, M. A. (2007). Deficit irrigation for reducing agricultural water use. J. Exp. Bot. 58, 147–159. doi:10.1093/jxb/erl165
Fernandes, R. D. M., Cuevas, M. V., Diaz-Espejo, A., and Hernandez-Santana, V. (2018). Effects of water stress on fruit growth and water relations between fruits and leaves in a hedgerow olive orchard. Agric. water Manag. 210, 32–40. doi:10.1016/j.agwat.2018.07.028
Fernandez, J.-E. (2014). Understanding olive adaptation to abiotic stresses as a tool to increase crop performance. Environ. Exp. Bot. 103, 158–179. doi:10.1016/j.envexpbot.2013.12.003
Fernández-Escobar, R., De la Rosa, R., Leon, L., Gomez, J., Testi, L., Orgaz, F., et al. (2013). Evolution and sustainability of the olive production systems. Options Mediterr. 106, 11–42.
Fraga, H., Pinto, J. G., Viola, F., and Santos, J. A. (2020). Climate change projections for olive yields in the Mediterranean basin. Int. J. Climatol. 40, 769–781. doi:10.1002/joc.6237
García, J. M., Hueso, A., and Gómez-del- Campo, M. (2020). Deficit irrigation during the oil synthesis period affects olive oil quality in high-density orchards (cv. Arbequina). Agric. Water Manag. 230, 105858. doi:10.1016/j.agwat.2019.105858
Girona, J., Mata, M., Arbonès, A., Alegre, S., Rufat, J., and Marsal, J. (2003). Peach tree response to single and combined regulated deficit irrigation regimes under shallow soils. J. Am. Soc. Hortic. Sci. 128, 432–440. doi:10.21273/jashs.128.3.0432
Gomez-del Campo, M., Connor, D. J., and Trentacoste, E. R. (2017). Long-term effect of intra-row spacing on growth and productivity of super-high density hedgerow olive orchards (cv. Arbequina). Front. Plant Sci. 8, 1790. doi:10.3389/fpls.2017.01790
Gomez-del Campo, M. (2010). Physiological and growth responses to irrigation of a newly established hedgerow olive orchard. HortScience 45, 809–814. doi:10.21273/hortsci.45.5.809
Gómez-del Campo, M. (2013). Summer deficit-irrigation strategies in a hedgerow olive orchard cv. ’Arbequina’: Effect on fruit characteristics and yield. Irrigation Sci. 31, 259–269. doi:10.1007/s00271-011-0299-8
Granier, C., Inzé, D., and Tardieu, F. (2000). Spatial distribution of cell division rate can be deduced from that of p34cdc2 kinase activity in maize leaves grown at contrasting temperatures and soil water conditions. Plant Physiol. 124, 1393–1402. doi:10.1104/pp.124.3.1393
Grappadelli, L. C., and Lakso, A. N. (2004). Is maximizing orchard light interception always the best choice? Rootstocks Environ. Physiology Orchard Syst. 732, 507–518. VIII International Symposium on Canopy.
Gucci, R., Caruso, G., Gennai, C., Esposto, S., Urbani, S., and Servili, M. (2019). Fruit growth, yield and oil quality changes induced by deficit irrigation at different stages of olive fruit development. Agric. Water Manag. 212, 88–98. doi:10.1016/j.agwat.2018.08.022
Gucci, R., Lodolini, E., and Rapoport, H. F. (2007). Productivity of olive trees with different water status and crop load. J. Hortic. Sci. Biotechnol. 82, 648–656. doi:10.1080/14620316.2007.11512286
Hartmann, H. T., and Panetsos, C. (1961). Effect of soil moisture deficiency during floral development on fruitfulness in the olive. Proc. Amer. Soc. Hort. Sci. 78, 209–217.
Hernandez-Santana, V., Fernández, J., Cuevas, M., Perez-Martin, A., and Diaz-Espejo, A. (2017). Photosynthetic limitations by water deficit: Effect on fruit and olive oil yield, leaf area and trunk diameter and its potential use to control vegetative growth of super-high density olive orchards. Agric. Water Manag. 184, 9–18. doi:10.1016/j.agwat.2016.12.016
Hueso, A., Camacho, G., and Gomez-del Campo, M. (2021). Spring deficit irrigation promotes significant reduction on vegetative growth, flowering, fruit growth and production in hedgerow olive orchards (cv. Arbequina). Agric. Water Manag. 248, 106695. doi:10.1016/j.agwat.2020.106695
Iniesta, F., Testi, L., Orgaz, F., and Villalobos, F. J. (2009). The effects of regulated and continuous deficit irrigation on the water use, growth and yield of olive trees. Eur. J. Agron. 30, 258–265. doi:10.1016/j.eja.2008.12.004
Kassout, J., Ater, M., Ivorra, S., Barbara, H., Limier, B., Ros, J., et al. (2021). Resisting aridification: Adaptation of sap conduction performance in Moroccan wild olive subspecies distributed over an aridity gradient. Front. plant Sci. 12, 663721. doi:10.3389/fpls.2021.663721
Kassout, J., Terral, J.-F., El Ouahrani, A., Houssni, M., Ivorra, S., Kadaoui, K., et al. (2022). “Species Distribution Based-Modelling Under Climate Change: The Case of Two Native Wild Olea europaea Subspecies in Morocco, O. e. subsp. europaea var. sylvestris and O. e. subsp. maroccana,” in Climate change in the mediterranean and middle eastern region (Berlin, Germany: Springer), 21–43.
Kassout, J., Terral, J.-F., Hodgson, J. G., and Ater, M. (2019). Trait-based plant ecology a flawed tool in climate studies? The leaf traits of wild olive that pattern with climate are not those routinely measured. PloS one 14, e0219908. doi:10.1371/journal.pone.0219908
Khadari, B., Charafi, J., Moukhli, A., and Ater, M. (2008). Substantial genetic diversity in cultivated Moroccan olive despite a single major cultivar: A paradoxical situation evidenced by the use of SSR loci. Tree Genet. Genomes 4, 213–221. doi:10.1007/s11295-007-0102-4
Khadari, B., El Bakkali, A., Essalouh, L., Tollon, C., Pinatel, C., and Besnard, G. (2019). Cultivated olive diversification at local and regional scales: Evidence from the genetic characterization of French genetic resources. Front. plant Sci. 10, 1593. doi:10.3389/fpls.2019.01593
Lavee, S., Hanoch, E., Wodner, M., and Abramowitch, H. (2007). The effect of predetermined deficit irrigation on the performance of cv. Muhasan olives (Olea europaea L) in the eastern coastal plain of Israel. Sci. Hortic. 112, 156–163. doi:10.1016/j.scienta.2006.12.017
Lavee, S., and Nir, G. (1986). “Grape,” in Crc handbook of fruit set and development (Italy: sp monselise), 167–191.
Lodolini, E. M., Gucci, R., and Rapoport, H. F. (2011). Interaction of crop load and water status on growth of olive fruit tissues and mesocarp cells. Acta Hortic. 2011, 89–93. doi:10.17660/actahortic.2011.924.10
Lorite, I., Gabaldón-Leal, C., Ruiz-Ramos, M., Belaj, A., De La Rosa, R., León, L., et al. (2018). Evaluation of olive response and adaptation strategies to climate change under semi-arid conditions. Agric. Water Manag. 204, 247–261. doi:10.1016/j.agwat.2018.04.008
Mahhou, A., DeJong, T. M., Cao, T., and Shackel, K. S. (2005). Water stress and crop load effects on vegetative and fruit growth of ‘elegant lady’ peach [prunus persica (l) batch] trees. Fruits 60, 55–68. doi:10.1051/fruits:2005013
Manrique, T., Rapoport, H. F., Castro, J., and Pastor, M. (1997). Mesocarp cell division and expansion in the growth of olive fruits. III Int. Symposium Olive Grow. 474, 301–304. doi:10.17660/actahortic.1999.474.61
Medail, F., Quezel, P., Besnard, G., and Khadari, B. (2001). Systematics, ecology and phylogeographic significance of Olea europaea L. ssp. maroccana (Greuter & Burdet) P. Vargas et al., a relictual olive tree in south-west Morocco. Botanical J. Linn. Soc. 137, 249–266. doi:10.1006/bojl.2001.0477
Moriana, A., Orgaz, F., Pastor, M., and Fereres, E. (2003). Yield responses of a mature olive orchard to water deficits. J. Am. Soc. Hortic. Sci. 128, 425–431. doi:10.21273/jashs.128.3.0425
Orgaz, F., Villalobos, F. J., Testi, L., and Fereres, E. (2007). A model of daily mean canopy conductance for calculating transpiration of olive canopies. Funct. Plant Biol. 34, 178–188. doi:10.1071/fp06306
Palese, A. M., Nuzzo, V., Favati, F., Pietrafesa, A., Celano, G., and Xiloyannis, C. (2010). Effects of water deficit on the vegetative response, yield and oil quality of olive trees (Olea europaea L., cv Coratina) grown under intensive cultivation. Sci. Hortic. 125, 222–229. doi:10.1016/j.scienta.2010.03.025
Paradis, E., Claude, J., and Strimmer, K. (2004). Ape: Analyses of phylogenetics and evolution in R language. Bioinformatics 20, 289–290. doi:10.1093/bioinformatics/btg412
Pierantozzi, P., Torres, M., Bodoira, R., and Maestri, D. (2013). Water relations, biochemical–physiological and yield responses of olive trees (Olea europaea L. cvs. Arbequina and Manzanilla) under drought stress during the pre-flowering and flowering period. Agric. Water Manag. 125, 13–25. doi:10.1016/j.agwat.2013.04.003
Pierantozzi, P., Torres, M., Tivani, M., Contreras, C., Gentili, L., Parera, C., et al. (2020). Spring deficit irrigation in olive (cv. Genovesa) growing under arid continental climate: Effects on vegetative growth and productive parameters. Agric. Water Manag. 238, 106212. doi:10.1016/j.agwat.2020.106212
Pinheiro, J., Bates, D., DebRoy, S., Sarkar, D., and Team, R. C. (2018). nlme: linear and nonlinear mixed effects models. R package version 3.1-137. Vienna, Austria: R Foundation.
Rallo, L., Caruso, T., Díez, C. M., and Campisi, G. (2016). “Olive growing in a time of change: From empiricism to genomics,” in The olive tree genome (Berlin, Germany: Springer), 55–64.
Ramos, A. F., and Santos, F. L. (2009). Water use, transpiration, and crop coefficients for olives (cv. Cordovil), grown in orchards in Southern Portugal. Biosyst. Eng. 102, 321–333. doi:10.1016/j.biosystemseng.2008.12.006
Ramos, A. F., and Santos, F. L. (2010). Yield and olive oil characteristics of a low-density orchard (cv. Cordovil) subjected to different irrigation regimes. Agric. Water Manag. 97, 363–373. doi:10.1016/j.agwat.2009.10.008
Rapoport, H. F., Costagli, G., and Gucci, R. (2004). The effect of water deficit during early fruit development on olive fruit morphogenesis. J. Am. Soc. Hortic. Sci. 129, 121–127. doi:10.21273/jashs.129.1.0121
Rapoport, H. F., Hammami, S. B., Martins, P., Pérez-Priego, O., and Orgaz, F. (2012). Influence of water deficits at different times during olive tree inflorescence and flower development. Environ. Exp. Bot. 77, 227–233. doi:10.1016/j.envexpbot.2011.11.021
Rosecrance, R. C., Krueger, W. H., Milliron, L., Bloese, J., Garcia, C., and Mori, B. (2015). Moderate regulated deficit irrigation can increase olive oil yields and decrease tree growth in super high density ‘Arbequina’ olive orchards. Sci. Hortic. 190, 75–82. doi:10.1016/j.scienta.2015.03.045
Sanz-Cortés, F., Martinez-Calvo, J., Badenes, M. L., Bleiholder, H., Hack, H., Llácer, G., et al. (2002). Phenological growth stages of olive trees (Olea europaea). Ann. Appl. Biol. 140, 151–157. doi:10.1111/j.1744-7348.2002.tb00167.x
Sebbar, A., Badri, W., Fougrach, H., Hsaine, M., and Saloui, A. (2011). Étude de la variabilité du régime pluviométrique au maroc septentrional (1935-2004). Sci. changements planétaires/Sécheresse 22, 139–148.
Serman, F. V., Orgaz, F., Starobinsky, G., Capraro, F., and Fereres, E. (2021). Water productivity and net profit of high-density olive orchards in San Juan, Argentina. Agric. Water Manag. 252, 106878. doi:10.1016/j.agwat.2021.106878
Servili, M., Esposto, S., Lodolini, E., Selvaggini, R., Taticchi, A., Urbani, S., et al. (2007). Irrigation effects on quality, phenolic composition, and selected volatiles of virgin olive oils cv. Leccino. J. Agric. Food Chem. 55, 6609–6618. doi:10.1021/jf070599n
Siefert, A., Violle, C., Chalmandrier, L., Albert, C. H., Taudiere, A., Fajardo, A., et al. (2015). A global meta-analysis of the relative extent of intraspecific trait variation in plant communities. Ecol. Lett. 18, 1406–1419. doi:10.1111/ele.12508
Steppe, K., Sterck, F., and Deslauriers, A. (2015). Diel growth dynamics in tree stems: Linking anatomy and ecophysiology. Trends plant Sci. 20, 335–343. doi:10.1016/j.tplants.2015.03.015
Tadayon, M. S., and Hosseini, S. M. (2023). Effect of irrigation regimes and foliar nutrition on flower development and water productivity of olive (olea europaea l cv ‘shengeh’). J. Plant Growth Regul. 42, 735–747. doi:10.1007/s00344-022-10580-x
Torres-Ruiz, J. M., Diaz-Espejo, A., Perez-Martin, A., and Hernandez-Santana, V. (2015). Role of hydraulic and chemical signals in leaves, stems and roots in the stomatal behaviour of olive trees under water stress and recovery conditions. Tree Physiol. 35, 415–424. doi:10.1093/treephys/tpu055
Trentacoste, E. R., Calderón, F. J., Contreras-Zanessi, O., Galarza, W., Banco, A. P., and Puertas, C. M. (2019). Effect of regulated deficit irrigation during the vegetative growth period on shoot elongation and oil yield components in olive hedgerows (cv. Arbosana) pruned annually on alternate sides in San Juan, Argentina. Irrigation Sci. 37, 533–546. doi:10.1007/s00271-019-00632-8
Trentacoste, E. R., Calderón, F. J., Puertas, C. M., Banco, A. P., Contreras-Zanessi, O., Galarza, W., et al. (2018). Vegetative structure and distribution of oil yield components and fruit characteristics within olive hedgerows (cv. Arbosana) mechanically pruned annually on alternating sides in San Juan, Argentina. Sci. Hortic. 240, 425–429. doi:10.1016/j.scienta.2018.06.045
Wheeler, T., and Von Braun, J. (2013). Climate change impacts on global food security. Science 341, 508–513. doi:10.1126/science.1239402
Keywords: regulated deficit irrigation, sustained deficit irrigation, olive production, water saving, water productivity
Citation: Ibba K, Kassout J, Boselli V, Er-Raki S, Oulbi S, Mansouri LE, Bouizgaren A, Sikaoui L and Hadria R (2023) Assessing the impact of deficit irrigation strategies on agronomic and productive parameters of Menara olive cultivar: implications for operational water management. Front. Environ. Sci. 11:1100552. doi: 10.3389/fenvs.2023.1100552
Received: 16 November 2022; Accepted: 15 May 2023;
Published: 19 June 2023.
Edited by:
Giulio Castelli, University of Florence, ItalyCopyright © 2023 Ibba, Kassout, Boselli, Er-Raki, Oulbi, Mansouri, Bouizgaren, Sikaoui and Hadria. This is an open-access article distributed under the terms of the Creative Commons Attribution License (CC BY). The use, distribution or reproduction in other forums is permitted, provided the original author(s) and the copyright owner(s) are credited and that the original publication in this journal is cited, in accordance with accepted academic practice. No use, distribution or reproduction is permitted which does not comply with these terms.
*Correspondence: Khaoula Ibba, a2hhb3VsYWliYmExOTk2QGdtYWlsLmNvbQ==
Disclaimer: All claims expressed in this article are solely those of the authors and do not necessarily represent those of their affiliated organizations, or those of the publisher, the editors and the reviewers. Any product that may be evaluated in this article or claim that may be made by its manufacturer is not guaranteed or endorsed by the publisher.
Research integrity at Frontiers
Learn more about the work of our research integrity team to safeguard the quality of each article we publish.