- 1School of Environmental Science and Engineering, Shandong University, Qingdao, China
- 2Institute of Eco-Environmental Forensics, Shandong University, Qingdao, China
- 3Formerly at Department of Earth Sciences, University of Western Ontario, London, ON, Canada
- 4Department of Biology, University of Western Ontario, London, ON, Canada
Boreal peatlands are considered sinks for atmospheric mercury (Hg) and are important sources of methylmercury (MeHg) to downstream ecosystems. Climate change-driven increases in average annual temperature in coming decades will be amplified at higher latitudes and will modify many biogeochemical processes in high boreal and subarctic peatlands that are important landscape features in these regions. Changes in water quality are an important issue for Northern ecosystems and fish consumers, and the directionality of changes in mercury levels due to climate warming presents considerable uncertainty. Peatlands are key landscape hotspots for MeHg production, however, the impact of climate warming on Hg cycling in boreal peatlands is not well studied. We use a multi-year field-based warming experiment (2 years passive, 1 year active ground warming) across two boreal peatland types (moss and sedge dominated) to explore the effects of ground warming on inorganic Hg (IHg) release, net MeHg production, and biogeochemical controls on both of these processes including the availability of sulfate (SO42−) and dissolved organic matter (DOM) quality and concentration. There were higher porewater IHg and MeHg concentrations under active ground warming conditions in both peatlands, likely related to both increased microbial metabolism, and changes in biogeochemical conditions that favor Hg methylation. Both SO42− (electron acceptor) and bioaccessible DOM (electron donor) are nutrients for sulfate-reducing bacteria which are dominant Hg methylators in freshwater environments, and increases in SO42− and/or bioaccessible DOM concentrations under warming played an important role in the observed changes in net MeHg production. Warming increased SO42− concentrations in the sedge-dominated but not in the moss-dominated fen likely because of a larger pool of groundwater derived SO42− in the sedge-dominated site. Warming increased DOM concentration in both peatland sites through enhanced decomposition of peat and increased release of root exudates from vascular plants, and the balance of these processes varied by peatland type and degree of warming. Experimentally increased ground temperatures increased microbial metabolism, organic matter turnover, and the availability of IHg all of which resulted in increases in porewater MeHg, indicating that climate-driven ground warming will increase MeHg production in northern peatlands in the future.
1 Introduction
The Earth’s climate is warming due to the increase in atmospheric greenhouse gasses driven mainly by anthropogenic fossil fuel combustion (IPCC, 2018). The IPCC (2018) special report states that the mean global temperature will reach at least 1.5°C above pre-industrial levels between 2030 and 2052 and higher latitudes of the northern hemisphere are experiencing greater rates of change in air temperature with increases of 6°C or greater (Brigham et al., 2009). Northern peatlands are particularly vulnerable to warming due to changes in hydrology (Tarnocai, 2009), primary productivity (McPartland et al., 2020) and community composition (Dieleman et al., 2015; Kolari et al., 2021), and biogeochemical cycles, particularly carbon (Dieleman et al., 2016; Zhang et al., 2020). Much of this is through warming-induced drying of peatlands by increasing evapotranspiration (Tarnocai, 2009; Helbig et al., 2020) which can decrease carbon accumulation (Zhang et al., 2020). Warming is also shown to increase vascular plant abundance and biomass and decrease Sphagnum spp. cover (Buttler et al., 2015; Dieleman et al., 2015), modifying organic matter cycling and carbon storage potential.
Mercury (Hg) is a global pollutant due to its ubiquitous distribution and anthropogenic enrichment (Driscoll et al., 2013). Mining, coal combustion, and cement manufacturing are the largest sources of global Hg emissions and largely responsible for increasing Hg concentrations in the atmosphere many-fold above pre-industrial levels (Streets et al., 2019). Inorganic Hg (IHg) can be converted into the toxic and bioaccumulating form methylmercury (MeHg) in anaerobic environments (e.g., lake sediments and peatlands) by sulfate-reducing bacteria (SRB) (Gilmour et al., 1992), iron-reducing bacteria (FeRB) (Fleming et al., 2006; Kerin et al., 2006), methanogenic archaea (Hamelin et al., 2011), and other syntrophic and acetogenic bacteria (Gilmour et al., 2013) although SRB are primary methylators in most freshwater environments. MeHg is a potent neurotoxin that can affect the nervous, immune, and reproductive systems of vertebrates (see review by Clarkson and Magos, 2006). Owing to its lipophilic and protein-binding properties, MeHg bioaccumulates and biomagnifies in food webs, posing a threat to upper trophic level wildlife and humans (Mergler et al., 2007).
Climate change is expected to substantially change the cycling of Hg, with local to regional scale implications (Driscoll et al., 2013; Krabbenhoft and Sunderland, 2013). These impacts may be direct such as increasing the amount of IHg available for methylation, as well as amplifying the methylation process itself. A warming environment has been directly linked to the release of IHg from degrading permafrost to nearby aquatic ecosystems (Ci et al., 2020; Mu et al., 2020; Schaefer et al., 2020). As a microbial process, it is not surprising that both laboratory (King et al., 1999; St Pierre et al., 2014; Yang et al., 2016) and field studies (Hurley et al., 1998; Hudelson et al., 2020) have found that higher temperatures favor net MeHg production (Canario et al., 2007). Several studies have attributed increased water MeHg concentrations to global warming (MacMillan et al., 2015; Yang et al., 2016).
Boreal peatlands are important “hotspots” of net MeHg production (Branfireun et al., 1999; Mitchell et al., 2008a), and temperature-mediated changes in the metabolism of Hg methylators and Hg availability would be expected in these ecosystems as well.
In addition to these direct effects, climate warming can also indirectly affect Hg methylation through hydrologic fluctuation (Coleman-Wasik et al., 2015), drought-induced wetting and drying cycles can lead to cycling in redox conditions (Helbig et al., 2020), an important factor for net MeHg production (Ullrich et al., 2001). Furthermore, a receding water table driven by climate warming increases the oxidative release of Hg and Hg methylator nutrients, such as sulfate (SO42-) and dissolved organic matter (DOM) (Haitzer et al., 2003). It has also been shown that higher temperatures may also increase the gaseous evasion of S compounds (e.g., hydrogen sulfide and carbonyl sulfides) from boreal peatlands and consequently decrease net MeHg production over time. Predicted changes in peatland plant biomass and community composition under climate warming (Dieleman et al., 2015; Mäkiranta et al., 2018) may also affect Hg methylation, given that plant biomass and community composition controls litter and root characteristics, which influence soil and pore water DOM quantity and bioaccessibility (Mastný et al., 2018).
Peatlands of the circum-boreal region of the northern hemisphere represent a relatively small but important land cover class with respect to carbon storage and the regulation of water quality. Moss-dominated (i.e., Sphagnum) and sedge-dominated (e.g., Carex) fen peatlands are both equally important types of boreal peatlands in Canada, however sedge-dominated more nutrient rich peat-accumulating wetlands are under-represented in the scientific literature relative to moss-dominated systems despite their nearly equal proportion by area in Canada (Wu and Roulet, 2014). Importantly, the potential shift of moss-dominated fens toward a much higher proportion of vascular plant (graminoid) cover due to warmer temperatures and elevated atmospheric CO2 (Dieleman et al., 2015) has significant implications for not only carbon cycling, but other biogeochemical processes that are linked to microbial metabolic processes, such as Hg methylation.
Given the wide ranging implications of future warming on northern peatland biogeochemistry, this study was conducted to assess the impact of experimental ground warming on the net MeHg production in two boreal peatlands: a Sphagnum moss-dominated fen and a Carex sedge-dominated fen peatland. Specifically, this study sought to (1) determine the effect of ground warming on IHg and MeHg concentrations in pore waters, and the net MeHg production potentials of the two fens, (2) characterize the importance of pore water chemistry including SO42− concentrations and DOM concentrations on IHg and MeHg concentrations, and the characteristics that explain among-temperatures differences in pore water IHg and MeHg concentrations, and (3) compare the among-fens differences in concentrations of IHg, MeHg and SO42− and concentrations and characteristics of DOM.
2 Methods
2.1 Study sites
The Biological Response to A Changing Environment (BRACE) experiment was established in 2012 associated with two adjacent peatlands that differ in vegetation composition (moss vs. sedge-dominated), hydrology, and nutrient status (low vs. intermediate) maintained as long-term monitoring sites by the Ontario Ministry of Natural Resources and Forestry–Ontario Forest Research Institute (OMNRF-OFRI). The peatlands are located ∼2 km apart in an 817 ha sub-watershed of the Lake Superior basin near White River, Ontario, Canada (48°21’ N, 84°20’ W). Both peatlands are fen peatlands and share a surrounding catchment of mixed-wood coniferous and deciduous forests. The sedge-dominated fen has lower plant species richness with a high abundance of Carex spp. sedges and a low shrub overstory mainly of sweet gale [Myrica gale L.] with some leatherleaf [Chamaedaphne calyculata L. Moench]. The moss-dominated fen has a near-continuous groundcover of Sphagnum spp. mosses, with a low shrub cover of leatherleaf, Labrador tea [Rhododendron groenlandicum (Oeder) Kron & Judd] and lowbush blueberry [Vaccinium angustifolium Aiton], and a more species rich array of herbs (e.g., Maianthemum trifolium (L.) Sloboda) with a sparse overstory of coniferous trees including tamarack [Larix laricina (Du Roi) K. Koch] and black spruce [Picea mariana (Mill.) Britton, Sterns & Poggenburg]. A full description of the vegetation community can be found in Lyons and Lindo (2020). The sedge-dominated fen is relatively more nutrient-rich with a higher pH (∼5.2) due to groundwater and surface water connections and has a generally higher water table (near soil surface) compared to the moss-dominated fen that has pH ∼4.2 and water table approx. ∼20 cm below the soil surface.
The sedge-dominated fen is 10.2 ha, while the moss-dominated fen is 4.5 ha; weather station data, including air temperature and precipitation, and water table depth is monitored continuously through the OMNRF-OFRI at both sites. Data collected from June to October in 2017, 2018, and 2019 report a mean air temperature of each year was 12.3°C, 8.7°C, and 12.9°C, respectively. The mean precipitation from June to October of each year was 299.05 mm, 277.15 mm, and 219.40 mm for 2017, 2018, and 2019, respectively.
2.2 Field experimental design
Sixteen experimental plots were established at each of the two peatland sites in 2015. At each site plots were established using cylindrical PVC collars (1 m diameter; 40 cm deep) inserted into the peat ∼30 cm. Half the plots were designated as warmed and equipped with six vertical immersion heaters (60 W Watlow FireRod®) installed to a depth of 50 cm at the time of plot establishment while the other half were designated as control plots (ambient temperatures). Plots were arranged in a block design (four plots per block) at each site to account for any small-scale spatial factors (e.g., microtopography, vegetation biomass and composition, moisture variability). Additionally, two reference plots (without PVC collars) were established at each of the two sites for a total of 36 experimental plots (2 sites × 2 treatments (control, warming) × 8 replicates plus 2 reference plots per site = 36 plots); reference plots were located ∼50 m away from the experimental plots in the sedge-dominated fen and ∼25 m away from experimental plots in the moss-dominated fen to ensure minimal disturbance.
In 2016, an integrated groundwater sampling well was permanently installed in each experimental (control and warmed) and reference plots. Sampling wells were custom fabricated from Teflon® (5 cm O.D. 4 cm I.D. 50 cm long; slotted along the entire length), and capped at both ends to prevent contamination. An acid-washed (10% v/v) 6.35 mm (0.25 cm O.D.) Teflon sampling line was installed in each well secured with a Teflon compression fitting in the vented top cap for sampling pore water.
2.2.1 Experimental warming
Experimental ground warming was implemented in stages beginning with passive warming in 2017. Passive warming was accomplished using clear, 1.2 m tall, open-topped chambers (OTCs) that were fitted onto the PVC collars at each of the warmed plots. Teflon sampling lines from central wells passed through the sidewall of the plot collar to allow for water sampling without having to remove the OTCs or otherwise disturb the plot. Passive warming was implemented from June to October in 2017, 2018 and 2019. Passive warming increased the mean surface peat (5 cm) temperatures by 0.38°C and 0.27°C for the moss-dominated fen and sedge-dominated fen, respectively (Lyons et al., 2020).
In June 2019, active ground warming was enabled in addition to passive warming with a target temperature of + 4°C above ambient peat temperatures in the deeper peat (30 cm). Active heating of the peat profile via the immersion heaters was controlled by a custom system designed by Zesta Engineering (Mississauga, ON) using paired thermocouples installed inside and outside of each warming plot. Manual temperature measurements at 25 cm depth were used to validate the + 4°C warming temperature. Active warming was established in June through late September in 2019 at both sites. Active warming increased the soil temperature (25 cm) of warmed plots by + 4.5 (SD ± 0.9)°C and + 3.8 (SD ± 0.8)°C in the moss-dominated fen and sedge-dominated fen, respectively. Two plots at each site had active warming malfunctions during the 2019 experimental season, and data from these warmed plots are not included in the 2019 data analysis of active warming. These plots continued to receive passive warming under the OTCs consistent with previous years.
Manual measurements of soil temperature at 5, 10 and 25 cm soil depth were performed weekly at three random locations in each plot. Surface soil moisture (top 10 cm) was also measured weekly at three different locations for each plot using an HH2 Moisture Meter (Delta-T Devices, Burwell, Cambridge, United Kingdom). We also monitored site-level water table elevation (below peat surface, cm) based on OMNRF-OFRI weather stations established at each site and TD-Diver™ and Baro-Diver® data loggers deployed in four locations across each site that measured water table levels (cm, below peat soil surface) every 15 min.
2.3 Sampling and analysis
Sampling wells were pre-purged using a Geotech® GeoPump (Geotech Ltd. North Aurora, ON, Canada) and acid-washed Masterflex® C-FlexUltra tubing (Cole-Palmer Instrument Co.). Pore waters were collected weekly (2017 and 2019) or bi-weekly (2018) into a pre-acid washed 500 mL PETG (polyethylene terephthalate glycol-modified) bottle (Thermo Scientific™ Nalgene™). A second smaller volume was used for pH measurements (Mettler Toledo Seven2GO™Pro) in the field at the time of water sampling. All pore water samples were kept in a clean cooler and transported each day to a local field laboratory for filtering and preservation. Sample lines were kept capped and clean between sampling times.
Standard ultraclean sampling protocols were used throughout sample collection (US EPA, 1996). Before sample collection, all sampling equipment and sample containers for Hg and DOM analysis were cleaned using Citranox® followed by a MilliQ water rinse and soaked in 10% (v:v) HCl overnight followed by a MilliQ water rinse in the university laboratory. Sample containers for ions analysis were cleaned using Citranox® followed by a MilliQ water rinse. After cleaning, sampling equipment and sample containers for Hg and MeHg sampling were individually double-bagged and shipped to the sampling site. Sample collection was compliant with EPA Method 1669, and is colloquially referred to as the “clean hands–dirty hands technique”. There were two people in a sampling team with one person as “dirty hands” and another as “clean hands”. All operations including preparation of sampling, operation of any machinery, and all other activities that do not directly contact with samples and the inner sample bottles were handled by “dirty hands”. “Clean hands” was responsible for all operations involving contact with sample bottles and the transfer of samples from the sampling equipment to the sample bottles. Clean disposable nitrile gloves were worn all the time to avoid contamination.
All samples were filtered within 6 h with 0.5 μm glass-fiber filters in an enclosed vacuum filter apparatus with Teflon wetted surfaces and transferred to acid-cleaned 250 mL PETG bottles for total Hg (THg) and MeHg analysis, 60 mL HDPE (high-density polyethylene) bottles for ion analysis, and 60 mL amber glass bottles for total DOC and optical characterization analysis. Filtered pore waters for Hg analysis were preserved by acidifying to 0.5% (vol/vol) with OmniTrace® hydrochloric acid. All filtered samples were stored at 4°C in the dark until they were returned to the university analytical lab for analysis.
Blanks of field, equipment and filters, and duplicates of samples were collected on each sampling date. Sample duplicates were performed by randomly choosing one plot and then simultaneously collecting two-pore water samples. Field blanks were performed by directly pouring the deionized water (18.2 MΩ cm) into the 250 mL PETG bottles. Equipment blanks were performed by directly pumping deionized water (18.2 MΩ cm) into the 500 mL PETG bottles using the acid-cleaned Masterflex C-FlexUltra tube. Filter blanks were performed by filtering the deionized water (18.2 MΩ cm) into 250 mL PETG bottles, 60 mL HDPE bottles, and 60 mL amber glass bottles. All field blanks, filter blanks, equipment blanks, and sample duplicates were stored and handled in the same way as field samples. All sampling equipment and sample containers were cleaned carefully before each sampling event, and standard ultraclean sampling protocols were used throughout sample collection (US EPA, 1996).
Pore water samples were analyzed for THg, MeHg, SO42−, and DOM concentrations and several DOM indices. Pore water THg concentrations were analyzed by cold vapor atomic fluorescence spectroscopy (CVAFS, Tekran 2600, Tekran Inc. Canada) using the Environmental Protection Agency (EPA) method 1631 (US EPA 2002). The pore water MeHg concentrations were determined by CVAFS (Tekran 2700, Tekran Inc. Canada) using the methods introduced by Bloom (1989) and Liang et al. (1994). The IHg concentration was calculated as the difference between THg and MeHg concentrations. Pore water SO42− concentrations were analyzed using ion chromatography on a Dionex ICS-1600 (Thermo Scientific™ Dionex™, Canada). Dissolved organic matter was quantified analytically as dissolved organic carbon (DOC); DOC concentrations in pore waters were determined by the persulfate wet oxidation method using the iTOC Aurora 1030 (OI Analytical, College Station, TX, United States).
The DOM in pore waters was characterized using several indices that are indicators of its molecular character and may be used to infer such things as its origin (e.g., microbial vs. detrital). These indices included the specific UV absorbance at 254 nm (SUVA254) and three optical fluorescence excitation-emission matrices (EEMs) indices: the fluorescence index (FI), humification index (HIXEM), and biological index or freshness index (BIX). The SUVA254 is an indicator of DOM aromaticity (aromatic molecules content) (Weishaar et al., 2003); higher SUVA254 values indicate higher DOM aromaticity. The values of SUVA254 were measured and calculated using EPA methods (415.3). The FI also reflects aromaticity and indicates DOM origin source with higher FI values (>1.8) being suggestive of microbially-derived DOM that has lower aromaticity (i.e., originating from processes such as extracellular release and leachate of algae and bacteria), while lower FI values (<1.2) indicate DOM is terrestrially derived (originating from decomposition and leaching of plant and soil organic matter) and has higher aromaticity (Fellman et al., 2010). The HIXEM indicates the humic substance content or the extent of humification that converts simple organic matter derived from plants and animals to more condensed and higher molecular weights organic matter by microbes (Fellman et al., 2010). High HIXEM values (>1.0) indicate high humification of DOM that contains more highly condensed and complex molecules. The BIX denotes the freshness of DOM that is generally produced by microorganisms (Fellman et al., 2010); higher BIX values (>1.0) indicate that DOM is predominantly produced by microbes and is more bioaccessible. Fluorescence and absorbance measurements were made using a Horiba Aqualog® fluorescence spectrofluorometer with a xenon lamp.
2.4 Statistical analysis
All statistical analyses were performed using SPSS statistics software (IBM SPSS Inc. 24.0). The effects of ground warming on surface soil moisture content and concentrations of total (THg), inorganic (IHg), and methyl-mercury (MeHg), and SO42− in porewater alongside percent MeHg, and DOM characteristics (DOC, SUVA254, FI, HIXEM, and BIX) in pore water were analyzed using repeated measures analysis of variance (ANOVA) following examination of the data for parametric test assumptions using Levene’s test and Q-Q plots; block effects were retained in the model only when significant. Separate statistical tests were used for each site as differences in pore water chemistry. Origin 9.3 (Microcal Software Inc. MA) software was used to visualize data. Data are shown as the mean ± standard deviation (SD).
Linear regression or non-parametric regression was used to test the relationships between the concentrations of THg, IHg and DOC. Coefficient of determination (R2) and significance probabilities (p) are presented for linear regression fits, with a level of significance set at p < 0.05.
3 Results
3.1 Effects of ground warming on physical environmental conditions
Water table levels (cm, below peat soil surface) were approximately 10 cm lower in the moss-dominated fen than in the sedge-dominated fen (Figure 1). Mean water table level was generally lowest in July and August of the experimental years, while the relatively high water table levels in June are attributed to snow melt. The higher water table levels in September and October are attributed to late season precipitation events. The mean water table levels during the growing season from June to August in both fens were generally lower in 2019 than in 2017 and 2018 (the moss-dominated fen: −14.1 ± 2.1 cm (2017), −16.5 ± 2.6 cm (2018), and −19.7 ± 4.3 cm (2019); the sedge-dominated fen: −0.2 ± 3.5 cm (2017), −6.3 ± 1.0 cm (2018), and −7.5 ± 3.9 cm (2019)); this coincides with the total annual precipitation across those years which was approximately 598 mm, 554 mm, and 439 mm in 2017, 2018, and 2019, respectively.
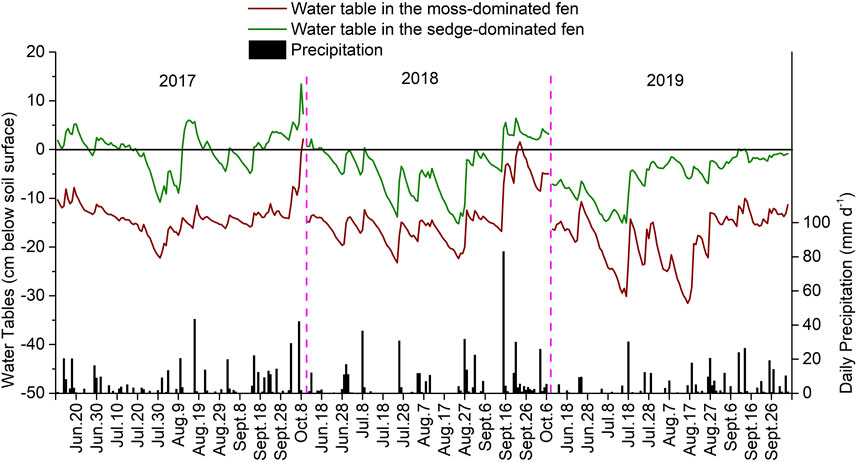
FIGURE 1. Water table levels and daily precipitation in the moss-dominated fen and the sedge-dominated fen over the experiment (2017–2019).
Warming (passive or active) had no significant effect on soil moisture in the top 10 cm of the peat at the moss-dominated fen (F1,6 = 0.133, p = 0.728), however, active warming significantly decreased soil moisture at the sedge-dominated fen (F1,12 = 5.52, p = 0.037), which was driven by a 21% reduction in soil moisture during active warming (Table 1). Active warming also induced periods of drier conditions in the surface peat at the moss-dominated fen (reductions on average 13%), particularly as the season progressed (Table 1). This pattern of later season reductions in soil moisture was also observed in 2017 and 2018 at both sites under passive warming, albeit to a lesser extent.
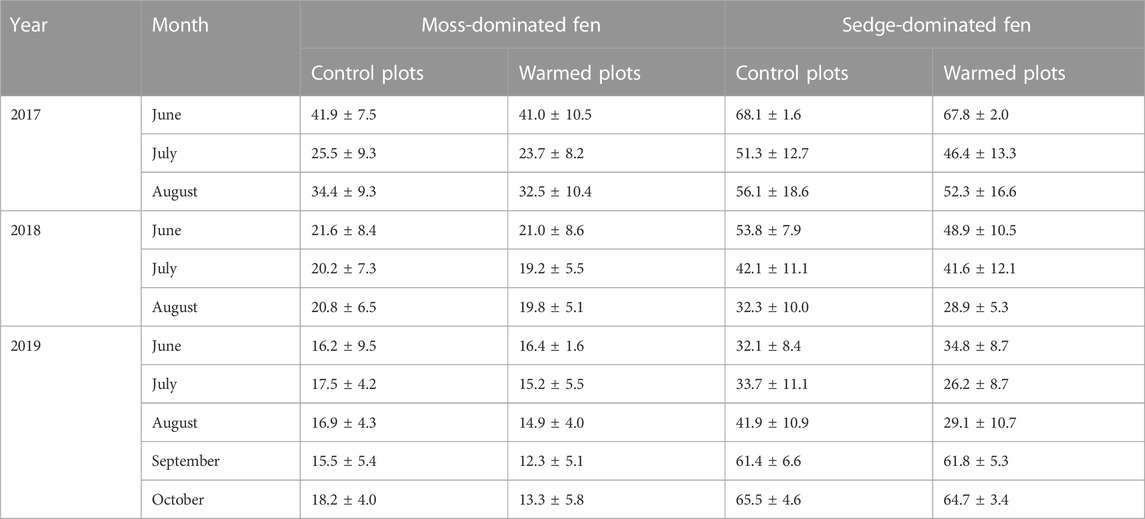
TABLE 1. Average monthly soil moisture (± standard deviation; % v/v) in the control and warmed plots in the moss-dominated fen and the sedge-dominated fen over the experiment.
3.2 Pore water mercury, methylmercury and sulfate concentrations
Total Hg concentrations were non-significantly higher in the warmed plots at both the moss-dominated site (F1,11 = 3.41, p = 0.092) and the sedge-dominated site (F1,12 = 0.049, p = 0.828) when all time points were considered together (Figure 2). In the moss-dominated site, passive warming had no significant effects on THg concentrations (F1,14 = 2.66, p = 0.125), while active warming significantly increased the concentrations of THg concentrations (F1,11 = 5.21, p = 0.043). Total Hg values were ∼50% greater under active warming in 2019 compared to ∼30% and ∼20% greater under passive warming in 2018 and 2017, respectively. There was no effect of passive (F1,14 = 0.36, p = 0.557) or active (F1,12 = 0.33, p = 0.578) warming on THg concentrations in pore waters collected from the sedge-dominated site.
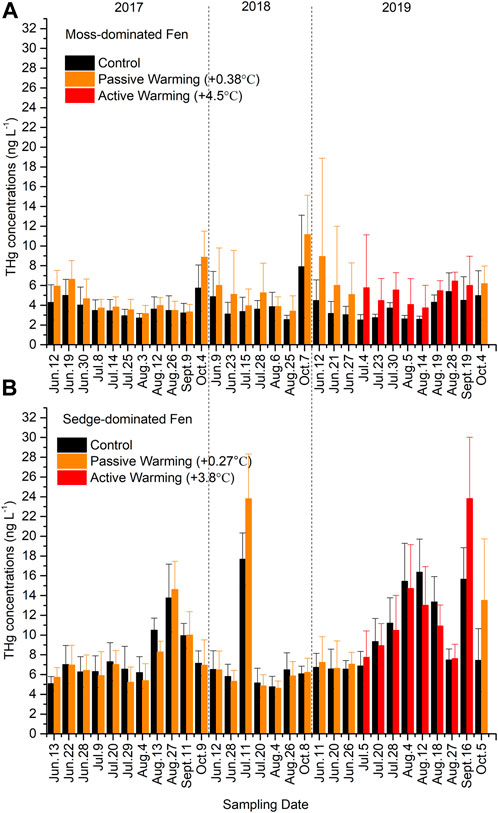
FIGURE 2. Total mercury (THg) concentrations in (A) the moss-dominated fen and (B) the sedge-dominated fen. Colors are different plot treatments: black for the control plots; orange for the passively warmed plots; red for the actively warmed plots. Bars are mean values and whiskers are ± ones tandard deviation. Note differences in y-axis scales.
Inorganic Hg (IHg) largely drove the patterns observed for THg at the moss-dominated site across all years with a trend not statistically different between treatments (F1,11 = 2.59, p = 0.136) (Figure 3A). There were also no significant effects of both passive (F1,14 = 2.44, p = 0.140) and active (F1,11 = 3.18, p = 0.102) warming on IHg concentrations in pore water. In the sedge-dominated site, while there were peaks in IHg that were greater under warming, pore water IHg concentrations in control plots were not significantly different than those in warmed plots overall (F1,12 = 0.10, p = 0.756) (Figure 3B). Both passive warming (F1,14 = 0.44, p = 0.517) and active warming (F1,12 = 0.64, p = 0.440) had no significant effects on IHg concentrations. Indeed under active warming in July and August, pore water IHg concentrations were slightly lower in the actively warmed plots than in the control plots. Although the pattern of IHg was relatively consistent over time between years, an anomalous peak in mid-July 2018 in IHg in both control and passively warmed plots was notable, and inconsistent with the period prior and post measurement.
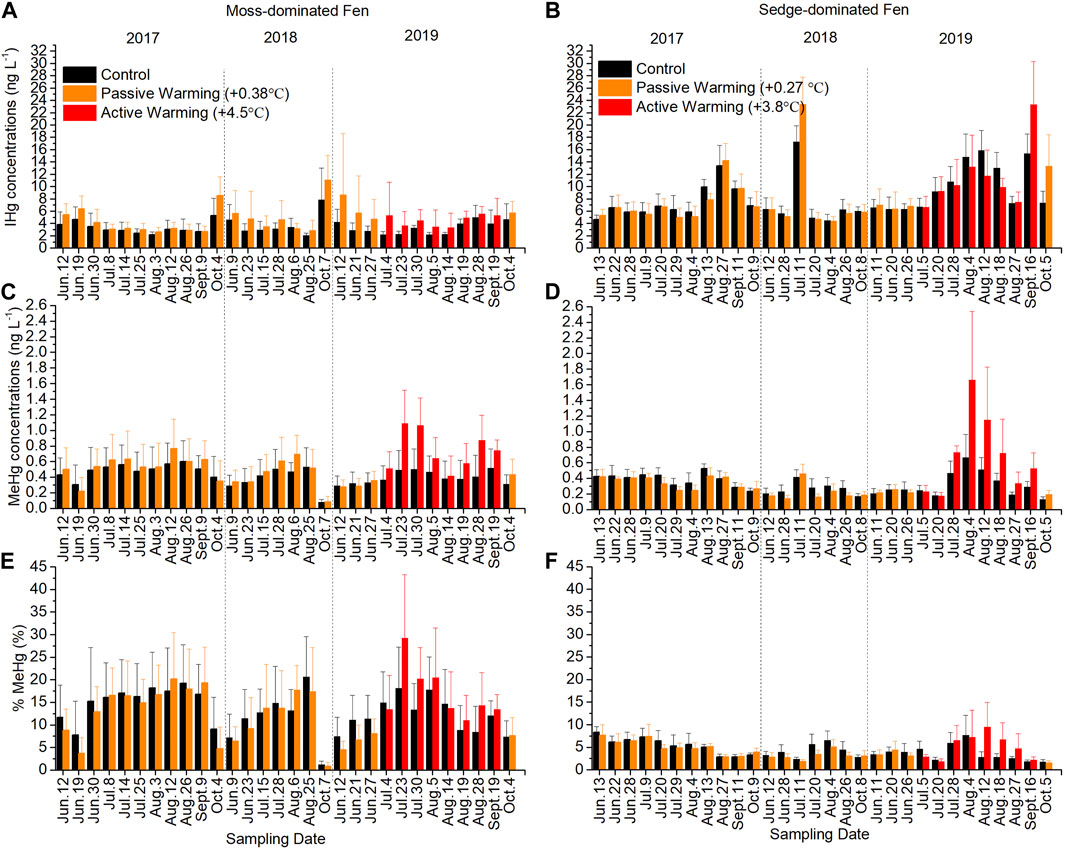
FIGURE 3. Concentrations of inorganic mercury (IHg) and methylmercury (MeHg) and the proportion of total Hg (THg) as MeHg (%MeHg) in pore waters in the control and warmed plots in the moss-dominated fen and the sedge-dominated fen over the experiment. Pore water IHg concentrations in (A) the moss-dominated fen and (B) the sedge-dominated fen; pore water MeHg concentrations in (C) the moss-dominated fen and (D) the sedge-dominated fen; %MeHg changes in pore waters in (E) the moss-dominated fen and (F) the sedge-dominated fen. Colors are different plot treatments: black for the control plots; orange for the passively warmed plots; red for the actively warmed plots. Bars are mean values and whiskers are ± one standard deviation.
There were no statistical difference in MeHg concentrations between treatments at the moss-dominated site (F1,11 = 2.51, p = 0.141) (Figure 3C). Passive warming had no significant effects on MeHg concentrations (F1,14 = 0.28, p = 0.603) but active warming-induced increases in MeHg were evident (F1,11 = 6.09, p = 0.031) where concentrations were on average more than 2× greater than the control plots. In the sedge-dominated site, only active (not passive: F1,13 = 1.63, p = 0.224) warming in 2019 (F1,11 = 9.94, p = 0.009) significantly increased MeHg concentrations, such that the overall effect of warming across all years was statistically non-significant (F1,10 = 3.63, p = 0.086). Increases in MeHg concentrations under active warming were 2–2.5× greater than the control plots in 2019 (Figure 3D). Both the moss-dominated and the sedge-dominated sites showed significant time × warming treatment interactions (moss-dominated: F29,319 = 3.52, p < 0.001; sedge-dominated: F29,290 = 8.69, p < 0.001). The %MeHg showed similar trends as MeHg concentrations with significant time × warming treatment interactions at both sites as %MeHg was lower under the passive warming plots at both sites, but higher under active warming plots compared to control plots (moss-dominated: F29,319 = 1.96, p = 0.003; sedge-dominated: F29,290 = 3.39, p < 0.001) (Figures 3E, F).
Sulfate concentrations were generally low (<0.5 mg/L) but showed strong summer seasonal peaks in concentration in both control and warmed plots (Figure 4). This trend was more pronounced at the sedge-dominated site (Figure 4B) and in 2019 at both sites. During 2017 and 2018 under passive warming and in 2019 under active ground warming, these peaks in pore water SO42− concentrations were greater in the experimentally warmed plots, although peaks were observed at the same sampling periods in the control plots. Ultimately while warming-induced increases in SO42− concentrations at the moss-dominated site were not significant overall (F1,10 = 0.10, p = 0.761), the site showed a significant time × warming treatment interaction (F25,175 = 2.42, p = 0.015). While the sedge-dominated also showed a significant time × warming treatment interaction (F25,175 = 4.83, p < 0.001), there was also an overall effect of warming on SO42− concentrations (F1,7 = 8.78, p = 0.021).
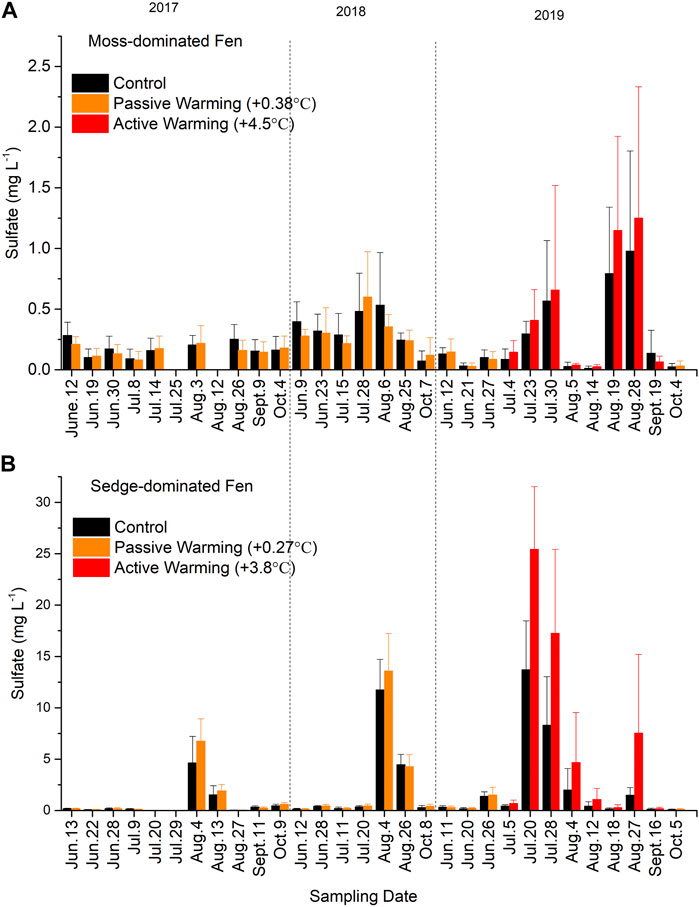
FIGURE 4. Sulfate (SO42−) concentrations in (A) the moss-dominated fen and (B) the sedge-dominated fen. Colors are different plot treatments: black for the control plots; orange for the passively warmed plots; red for the actively warmed plots. Bars are mean values and whiskers are ± one standard deviation. Note differences in y-axis scales.
3.3 Response of dissolved organic matter concentrations and characteristics to ground warming
Superimposed on annual cycles of higher concentrations during the summer months, increase in DOC under warming was small and the overall result was not statistically significant at either site (moss-dominated: F1,10 = 1.59, p = 0.235; sedge-dominated: F1,11 = 1.76, p = 0.211) (Figures 5A, B). Additionally, both passive warming (moss-dominated: F1,13 = 1.14, p = 0.306; sedge-dominated: F1,13 = 0.73, p = 0.408) and active warming (moss-dominated: F1,11 = 3.40, p = 0.092; sedge-dominated: F1,12 = 3.21, p = 0.099) had no significant effects on DOC concentrations at either site. There was a significant time × warming treatment interaction at the sedge-dominated site (F29,319 = 1.69, p < 0.05) but not at the moss-dominated site (F29,290 = 1.21, p = 0.212).
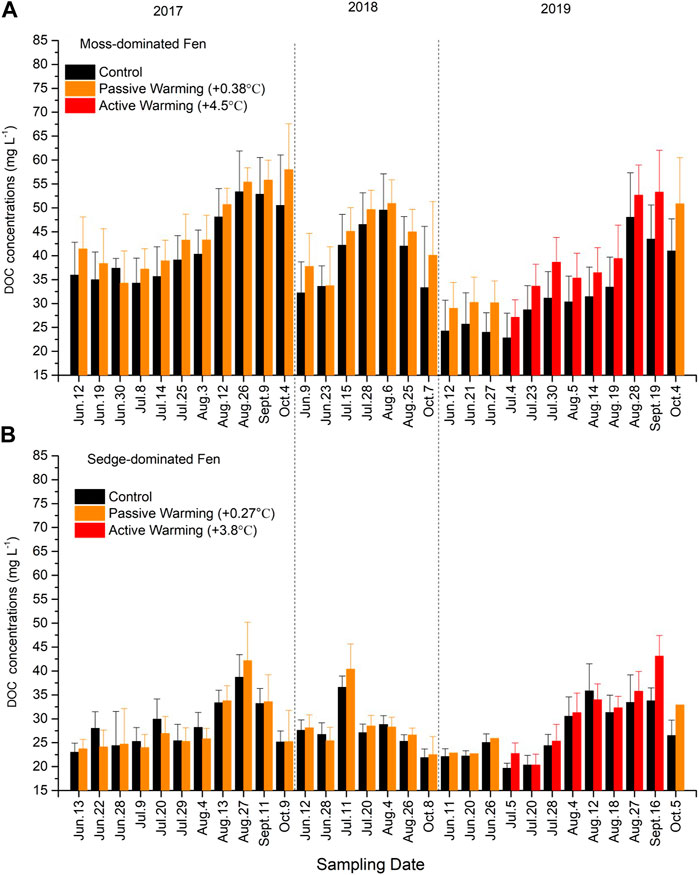
FIGURE 5. Dissolved organic carbon (DOC) concentrations in (A) the moss-dominated fen and (B) the sedge-dominated fen. Colors are different plot treatments: black for the control plots; orange for the passively warmed plots; red for the actively warmed plots. Bars are mean values and whiskers are ± one standard deviation.
Both passive warming (F1,12 = 3.44, p = 0.088) and active warming (F1,11 = 1.48, p = 0.249) had no significant influences on SUVA254 at the moss-dominated site leading to an overall non-significant effect of warming on SUVA254 (F1,9 = 0.14, p = 0.716) (Table 2). SUVA254 was consistently lower under warming at the sedge-dominated site (F1,8 = 3.42, p = 0.102), but only statistically significantly lower under active warming (passive: F1,11 = 0.02, p = 0.901; active: F1,11 = 8.50, p = 0.014). The fluorescence indices FI, HIXEM, and BIX showed no significant difference between warming and control plots at the moss-dominated site. At the sedge-dominated site, FI was higher under warmed conditions (not significant: F1,10 = 3.90, p = 0.076) with the trend largely driven by increases under active warming in 2019 (time × warming treatment interaction: F29,290 = 2.0, p = 0.003). The HIXEM index was not significantly different between warmed and control treatment plots (F1,10 = 1.30, p = 0.276) but was marginally lower during the period of active warming in 2019. The BIX index was significantly higher in the warming treatment plots (F1,10 = 8.04, p = 0.018), which was largely driven by increases during the 2019 active warming (time × warming treatment interaction: F29,290 = 3.60, p < 0.001) (Table 2 presents the summary data for DOM quality measures).
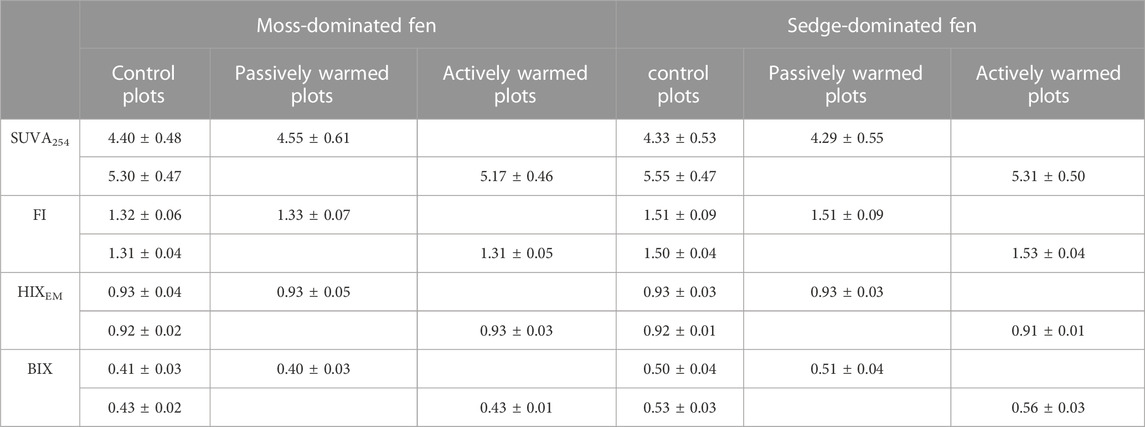
TABLE 2. Measures of specific ultraviolet absorbance at 254 nm (SUVA254, L mg C−1 mL−1) and fluorescence indices (FI, HIXEM, and BIX) in pore waters under the ground warming treatment at moss- and sedge-dominated sites. Passive warming was from June to October in 2017, 2018 and 2019; active warming was from July to September in 2019. Control and Experimental Plot data are paired by year. Values are presented as mean ± standard deviation.
4 Discussion
Given the well-established geochemical and biological controls on Hg cycling and methylation in particular, it is perhaps not surprising that overall, ground warming resulted in increased concentrations of THg and MeHg through decomposition-mediated release from peats into pore waters, and through enhanced net Hg methylation. Higher temperatures increase microbial metabolism (Comeau, 2008), leading in this case to a subsequent increase in decomposition and net Hg methylation. The increased decomposition of soil organic matter (SOM) not only releases more DOM but also releases more Hg, given the strong binding between Hg and the reduced sulfur groups in DOM (Xia et al., 1999). Some of the increases in MeHg concentrations observed indeed may be because MeHg is more readily desorbed from soils than IHg, given that IHg is chemically bound more strongly to the reduced sulfur groups in SOM than MeHg (Skyllberg, 2008; Liem-Nguyen et al., 2017). This study could not differentiate between MeHg in porewaters derived from desorption versus that from active Hg methylation, however there is ample evidence that active methylation played an important role, particularly under the active heating treatment in 2019. Active warming significantly increased THg concentrations in porewaters in the moss-dominated site but not in the sedge-dominated site. An explanation is that active warming plots in the moss-dominated site had a larger increased soil temperature (+4.5°C) than that in the sedge-dominated site (+3.8°C), leading to an enhancement of SOM decomposition and more releases of THg. Additionally, the peat in the sedge-dominated fen will be inherently more decomposable than the more recalcitrant Sphagnum-dominated peat at the moss site.
Measured MeHg concentrations are controlled by both Hg methylation and demethylation (Ullrich et al., 2001), and the measurements made as part of this study cannot determine the relative importance of each of these in regulating net MeHg concentrations in porewaters. Although both may be considered a largely biologically-mediated process in the sub-surface environment, and as such are regulated by temperature as a first-order control, it has been shown that relatively higher temperatures tend to favor the Hg methylation reaction (Canario et al., 2007; St Pierre et al., 2014; Hudelson et al., 2020) while relatively lower temperatures tend to favor MeHg demethylation (Ramlal et al., 1993). Active warming in this study may have warmed soil temperatures into a more optimal range for Hg methylation than demethylation in both fens. The relative fraction of THg that is MeHg (%MeHg) is often used as an indication of net MeHg production in sediment when comparing among sites (Paranjape and Hall, 2017). The overall higher %MeHg in the moss-dominated peatland is consistent with relatively high %MeHg reported in these types of wetlands relative to other methylating environments (Yu et al., 2010). Indeed, %MeHg in the moss-dominated fen is 2–10 times higher than that in the sedge-dominated fen, and is almost certainly due to its high aromatic fraction of DOM and deeply reducing conditions. DOM especially with high aromaticity plays an important role in increasing the mobility and transport of Hg and the bioaccessibility of IHg (Ravichandran, 2004; Hall et al., 2008). Soil interactions with surface water and ground water in the sedge-dominated fen would maintain both the vascular plant community, and a relatively higher redox environment than in the moss-dominated system. The lower water table levels and the deeply reducing conditions in the moss-dominated site can apply more nutrients (such as SO42−) by oxidation under hydrological fluctuation conditions, leading to a significant increase in MeHg production, given that the addition of the more limited SO42−increases net Hg methylation (Branfireun et al., 1999; Mitchell et al., 2008b). Despite active warming increasing both the THg and MeHg concentrations in porewater in the moss-dominated site, the relatively greater increase in MeHg concentrations resulted in a large increase in %MeHg, strongly suggesting active methylation of porewater IHg. Although the sedge-dominated site showed a stronger impact of active warming on absolute porewater MeHg concentrations, the %MeHg remained relatively low because that the relatively high absolute porewater IHg concentrations consistently measured at this site may offset the changes of increased MeHg concentrations. The substantial increase in MeHg concentrations and decreased IHg concentrations in warmed plots relative to controls in the sedge-dominated site could be a reflection of the net conversion of dissolved IHg to MeHg at this site.
Sulfate is an important but limiting nutrient for the dominant Hg methylator, the SRB, in boreal peatlands (Branfireun et al., 1999; Mitchell et al., 2008b). Rising temperature stimulates SRB metabolism with consumption of SO42− (Holmer and Storkholm, 2001), while drier conditions increase the oxidation of reduced sulfur compounds (Mandernack et al., 2000). Warming had no direct significant effects on SO42− concentrations in the moss-dominate site but did in the sedge-dominated site. The sedge-dominated site is relatively more SO42−-rich due to groundwater and surface water connections with a generally higher water table, and this generally higher availability alone may have been responsible for an observable significant effect at this site. The more SO42− limited, and strongly reducing conditions in the moss-dominated fen indeed may have resulted in a much more rapid cycling of available SO42− such that any warming related increase in SO42− availability was not measurable. The significant time × warming treatment interaction in both sites suggested that SO42− concentrations were controlled by both seasonal changes in environmental conditions and warming treatments. The peak summer months generally bring about lower water table levels and lower surface soil moistures due to lower rainfall amounts and higher evapotranspiration rates. Thus, the higher SO42− concentrations in July and August across all years, especially in 2019, were partially attributed to the oxidative release of reduced sulfur compounds from peat soils (Rydin et al., 2013). Although higher SO42− concentrations and more oxic conditions may appear contradictory to the observed concomitant increases in MeHg concentrations, this was likely a function of the sampling approach in the experimental plots. A single fully integrating 50 cm well was used to sample porewater chemistry. During periods of the lower water table and oxidation of reduced sulphur compounds, SO42− becomes available in the upper peat profile and at the oxic-anoxic interface, stimulating Hg methylation in the deeper saturated zone, all of which is sampled together by the integrated closed Teflon well. Enhanced net MeHg production under warming conditions is not just a function of increased microbial metabolism but also increased SO42− availability due to the drying.
Overall microbial metabolism and Hg availability may be also increased by warming-induced changes to DOM quantity and quality. There is no doubt that warming increases microbial metabolism (Comeau, 2008), with a subsequent increase in decomposition. However, there was a non-significant increase in warming on DOC concentrations at both sites. A plausible explanation is that warmer conditions may enhance decomposition rates corresponding with increases in the emissions of CO2 (Dorrepaal et al., 2009; Schuur et al., 2015). At the moss-dominated site, there were no significant differences in mean SUVA254 values and other fluorescence indices between warming plots and control plots, possibly reflecting the contribution of both higher and lower molecular weight (more and less aromatic) compounds from SOM decomposition and microbial biomass or plant root exudates, respectively.
At the sedge-dominated fen, warming significantly decreased SUVA254 values over time and significantly increased BIX index. These trends indicate a larger input of plant-derived lower molecular weight DOM and bacterial biomass. This is entirely consistent with the fact that this fen vegetation community is overwhelmingly dominated by Carex spp. which has been shown to increase in biomass at this site in response to even passive warming in the plots (Lyons et al., 2020). Further, Lyons and Lindo (2020) used phospholipid fatty acid (PLFA) analysis to show that the sedge-dominated fen microbial community was bacterially-dominated, whereas the moss-dominated fen was fungal-dominated. The significant difference in bacterial abundance is a parsimonious explanation for the observed effects on DOM quality indices in the sedge-dominated fen and not the moss-dominated site; over the short duration of the experiment, the much higher “ecosystem metabolism” of the sedge-dominated site allowed for changes to manifest more quickly. The rapid response of the bacteria-dominated microbial community in the sedge-dominated fen coupled with the increased above and belowground biomass of the dominant Carex spp. and the increased delivery of root exudates and relatively labile litter inputs suggests that sedge-dominated systems could be more important sites of Hg methylation in a warmer world. The potential trajectory of moss-dominated fens toward sedge-dominated systems under climate change (Dieleman et al., 2015) raises concerns about northern peatlands more generally becoming more potent sites of Hg methylation because of shifts in plant community composition, in addition to weaker carbon sinks (Wu and Roulet, 2014).
Our results indicate that climate change-induced ground warming will increase MeHg concentrations in the porewaters of northern peatlands, and that the degree of this increase depends in part on peatland type. This increase is driven by not just the expected increase in microbial metabolism which will speed up other biogeochemical cycles in addition to Hg methylation, but also warming-induced increased availability of other reactants required for methylation, including SO42−, labile DOM, and bioavailable IHg. This experiment may represent a transient response to pulse warming treatment, however the size of the pool of available sulfur, OM and IHg in peatlands along with the consistency of the findings here with other work related to warming induced peatland changes strongly suggest that the enhancement of MeHg production in these systems under future warming is both likely to occur, and is likely to be sustained. Ultimately the implications of this increased MeHg production on downstream aquatic systems is a function of MeHg export from these sites of methylation. summer streamflow from northern peatlands will likely decrease as a function of future warming and lower water tables, potentially offsetting the negative impacts of higher rates of MeHg production within the peatland itself.
Data availability statement
The raw data supporting the conclusion of this article will be made available by the authors, without undue reservation.
Author contributions
All authors contributed to the editing of the final manuscript; ZL and BB designed and initiated with BRACE experiment, TS collected and analysed samples, conducted data analysis and interpretation, and wrote the draft manuscript.
Funding
The Funding was provided by the Natural Sciences and Engineering Research Council of Canada (NSERC) Discover Grants program (# 217046-2009 [BB], # 05901–2019 [ZL]), Research Tools and Infrastructure (# 458702-2014 [ZL and BB]), and Strategic Partnership Grants program (# 479026-2015 [BB and ZL]).
Acknowledgments
We thank numerous field crews and technicians from the Ontario Ministry of Natural Resources and Forestry for their help in establishing the experimental system at these two remote peatland sites, including James McLaughlin who was instrumental in overseeing our continued site success. We would also like to thank several undergraduate and graduate students for field support (Bayley Germain, Jing Tian, Caitlyn Lyons, Madelaine Anderson, and Ericka James).
Conflict of interest
The authors declare that the research was conducted in the absence of any commercial or financial relationships that could be construed as a potential conflict of interest.
Publisher’s note
All claims expressed in this article are solely those of the authors and do not necessarily represent those of their affiliated organizations, or those of the publisher, the editors and the reviewers. Any product that may be evaluated in this article, or claim that may be made by its manufacturer, is not guaranteed or endorsed by the publisher.
References
Bloom, N. (1989). Determination of picogram levels of methylmercury by aqueous phase ethylation, followed by cryogenic gas chromatography with cold vapour atomic fluorescence detection. Can. J. Fish. Aquatic Sci. 46, 1131–1140. doi:10.1139/f89-147
Branfireun, B. A., Roulet, N. T., Kelly, C. A., and Rudd, J. W. M. (1999). In situ sulphate stimulation of mercury methylation in a boreal peatland: Toward a link between acid rain and methylmercury contamination in remote environments. Glob. Biogeochem. Cycles 13, 743–750. doi:10.1029/1999gb900033
Brigham, M. E., Wentz, D. A., Aiken, G. R., and Krabbenhoft, D. P. (2009). Mercury cycling in stream ecosystems. 1. water column chemistry and transport. Environ. Sci. Technol. 43, 2720–2725. doi:10.1021/es802694n
Buttler, A., Robroek, B. J. M., Laggoun-Defarge, F., Jassey, V. E. J., Pochelon, C., Bernard, G., et al. (2015). Experimental warming interacts with soil moisture to discriminate plant responses in an ombrotrophic peatland. J. Veg. Sci. 26, 964–974. doi:10.1111/jvs.12296
Canario, J., Branco, V., and Vale, C. (2007). Seasonal variation of monomethylmercury concentrations in surface sediments of the Tagus Estuary (Portugal). Environ. Pollut. 148, 380–383. doi:10.1016/j.envpol.2006.11.023
Ci, Z. J., Peng, F., Xue, X., and Zhang, X. S. (2020). Permafrost thaw dominates mercury emission in Tibetan thermokarst ponds. Environ. Sci. Technol. 54, 5456–5466. doi:10.1021/acs.est.9b06712
Clarkson, T. W., and Magos, L. (2006). The toxicology of mercury and its chemical compounds. Crit. Rev. Toxicol. 36, 609–662. doi:10.1080/10408440600845619
Coleman-Wasik, J., Engstrom, D. R., Mitchell, C. P. J., Mitchell, E. B., Monson, B. A., Balogh, S. J., et al. (2015). The effects of hydrologic fluctuation and sulfate regeneration on mercury cycling in an experimental peatland. J. Geophys. Research-Biogeosciences 120, 1697–1715. doi:10.1002/2015jg002993
Comeau, Y. (2008). “Microbial metabolism,” in Biological wastewater treatment: principles, modelling and design (London: IWA Publishing), 9–32.
Dieleman, C. M., Branfireun, B. A., McLaughlin, J. W., and Lindo, Z. (2015). Climate change drives a shift in peatland ecosystem plant community: Implications for ecosystem function and stability. Glob. Change Biol. 21, 388–395. doi:10.1111/gcb.12643
Dieleman, C. M., Lindo, Z., McLaughlin, J. W., Craig, A. E., and Branfireun, B. A. (2016). Climate change effects on peatland decomposition and porewater dissolved organic carbon biogeochemistry. Biogeochemistry 128, 385–396. doi:10.1007/s10533-016-0214-8
Dorrepaal, E., Toet, S., van Logtestijn, R. S. P., Swart, E., van de Weg, M. J., Callaghan, T. V., et al. (2009). Carbon respiration from subsurface peat accelerated by climate warming in the subarctic. Nature 460, 616–619. doi:10.1038/nature08216
Driscoll, C. T., Mason, R. P., Chan, H. M., Jacob, D. J., and Pirrone, N. (2013). Mercury as a global pollutant: Sources, pathways, and effects. Environ. Sci. Technol. 47, 4967–4983. doi:10.1021/es305071v
Fellman, J. B., Hood, E., and Spencer, R. G. M. (2010). Fluorescence spectroscopy opens new windows into dissolved organic matter dynamics in freshwater ecosystems: A review. Limnol. Oceanogr. 55, 2452–2462. doi:10.4319/lo.2010.55.6.2452
Fleming, E. J., Mack, E. E., Green, P. G., and Nelson, D. C. (2006). Mercury methylation from unexpected sources: Molybdate-inhibited freshwater sediments and an iron-reducing bacterium. Appl. Environ. 72, 457–464. doi:10.1128/AEM.72.1.457-464.2006
Gilmour, C. C., Henry, E. A., and Mitchell, R. (1992). Sulfate stimulation of mercury methylation in freshwater sediments. Environ. Sci. Technol. 26, 2281–2287. doi:10.1021/es00035a029
Gilmour, C. C., Podar, M., Bullock, A. L., Graham, A. M., Brown, S. D., Somenahally, A. C., et al. (2013). Mercury methylation by novel microorganisms from new environments. Environ. Sci. Technol. 47, 11810–11820. doi:10.1021/es403075t
Haitzer, M., Aiken, G. R., and Ryan, J. N. (2003). Binding of mercury (II) to aquatic humic substances: Influence of pH and source of humic substances. Environ. Sci. Technol. 37, 2436–2441. doi:10.1021/es026291o
Hall, B. D., Aiken, G. R., Krabbenhoft, D. P., Marvin-DiPasquale, M., and Swarzenski, C. M. (2008). Wetlands as principal zones of methylmercury production in southern Louisiana and the Gulf of Mexico region. Environ. Pollut. 154, 124–134. doi:10.1016/j.envpol.2007.12.017
Hamelin, S., Amyot, M., Barkay, T., Wang, Y. P., and Planas, D. (2011). Methanogens: Principal methylators of mercury in lake periphyton. Environ. Sci. Technol. 45, 7693–7700. doi:10.1021/es2010072
Helbig, M., Waddington, J. M., Alekseychik, P., Amiro, B. D., Aurela, M., Barr, A. G., et al. (2020). Increasing contribution of peatlands to boreal evapotranspiration in a warming climate. Nat. Clim. Change 10, 555–560. doi:10.1038/s41558-020-0763-7
Holmer, M., and Storkholm, P. (2001). Sulphate reduction and sulphur cycling in lake sediments: A review. Freshw. Biol. 46, 431–451. doi:10.1046/j.1365-2427.2001.00687.x–
Hudelson, K. E., Drevnick, P. E., Wang, F. Y., Armstrong, D., and Fisk, A. T. (2020). Mercury methylation and demethylation potentials in Arctic lake sediments. Chemosphere 248, 126001. doi:10.1016/j.chemosphere.2020.126001
Hurley, J. P., Krabbenhoft, D. P., Cleckner, L. B., Olson, M. L., Aiken, G. R., and Rawlik, P. S. (1998). System controls on the aqueous distribution of mercury in the northern Florida Everglades. Biogeochemistry 40, 293–311. doi:10.1023/a:1005928927272
IPCC (2018). “Summary for policymakers, in ” Global warming of 1.5°C. An IPCC special report on the impacts of global warming of 1.5°C above pre-industrial levels and related global greenhouse gas emission pathways, in the context of strengthening the global response to the threat of climate change, sustainable development, and efforts to eradicate poverty. Editors V. P. Masson-Delmotte, H.-O. Zhai, D. Portner, J. Roberts, P. R. Skea, A. Shuklaet al. (Geneva, Switzerland: World Meteorological Organization), 32
Kerin, E. J., Gilmour, C. C., Roden, E., Suzuki, M. T., Coates, J. D., and Mason, R. P. (2006). Mercury methylation by dissimilatory iron-reducing bacteria. Appl. Environ. Microbiol. 72, 7919–7921. doi:10.1128/aem.01602-06
King, J. K., Saunders, F. M., Lee, R. F., and Jahnke, R. A. (1999). Coupling mercury methylation rates to sulfate reduction rates in marine sediments. Environ. Toxicol. Chem. 18, 1362–1369. doi:10.1002/etc.5620180704
Kolari, T. H. M., Sallinen, A., Wolff, F., Kumpula, T., Tolonen, K., and Tahvanainen, T. (2021). Ongoing fen-bog transition in a Boreal Aapa mire inferred from repeated field sampling, aerial images, and landsat data. Ecosystems 25, 1166–1188. doi:10.1007/s10021-021-00708-7
Krabbenhoft, D. P., and Sunderland, E. M. (2013). Global change and mercury. Science 341, 1457–1458. doi:10.1126/science.1242838
Liang, L., Horvat, M., and Bloom, N. S. (1994). An improved speciation method for mercury by GC/CVAFS after aqueous phase ethylation and room temperature precollection. Talanta 41, 371–379. doi:10.1016/0039-9140(94)80141-x
Liem-Nguyen, V., Skyllberg, U., and Bjorn, E. (2017). Thermodynamic modeling of the solubility and chemical speciation of mercury and methylmercury driven by organic thiols and micromolar sulfide concentrations in boreal wetland soils. Environ. Sci. Technol. 51, 3678–3686. doi:10.1021/acs.est.6b04622
Lyons, C. L., Branfireun, B. A., McLaughlin, J., and Lindo, Z. (2020). Simulated climate warming increases plant community heterogeneity in two types of boreal peatlands in north-central Canada. J. Veg. Sci. 31, 908–919. doi:10.1111/jvs.12912
Lyons, C. L., and Lindo, Z. (2020). Above- and belowground community linkages in boreal peatlands. Plant Ecol. 221, 615–632. doi:10.1007/s11258-020-01037-w
MacMillan, G. A., Girard, C., Chetelat, J., Laurion, I., and Amyot, M. (2015). High methylmercury in Arctic and Subarctic ponds is related to nutrient levels in the warming eastern Canadian Arctic. Environ. Sci. Technol. 49, 7743–7753. doi:10.1021/acs.est.5b00763
Mäkiranta, P., Laiho, R., Mehtätalo, L., Straková, P., Sormunen, J., Minkkinen, K., et al. 2018. Responses of phenology and biomass production of boreal fens to climate warming under different water-table level regimes. Glob. Change Biol., 24: 944–956. doi:10.1111/gcb.13934
Mandernack, K. W., Lynch, L., Krouse, H. R., and Morgan, M. D., (2000). Sulfur cycling in wetland peat of the New Jersey Pinelands and its effect on stream water chemistry. Geochimica Cosmochimica Acta, 64, 3949–3964.
Mastný, J., Kastovska, E., Barta, J., Chronakova, A., Borovec, J., Santruckova, H., et al. (2018). Quality of DOC produced during litter decomposition of peatland plant dominants. Soil Biol. Biochem., 121, 221–230. doi:10.1016/j.soilbio.2018.03.018
McPartland, M. Y., Montgomery, R. A., Hanson, P. J., Phillips, J. R., Kolka, R., and Palik, B. (2020). Vascular plant species response to warming and elevated carbon dioxide in a boreal peatland. Environ. Res. Lett. 15, 124066. doi:10.1088/1748-9326/abc4fb
Mergler, D., Anderson, H. A., Chan, L. H. M., Mahaffey, K. R., Murray, M., Sakamoto, M., et al. (2007). Methylmercury exposure and health effects in humans: A worldwide concern. Ambio 36, 3–11. doi:10.1579/0044-7447(2007)36[3:meahei]2.0.co;2
Mitchell, C. P. J., Branfireun, B. A., and Kolka, R. K. (2008b). Assessing sulfate and carbon controls on net methylmercury production in peatlands: An in situ mesocosm approach. Appl. Geochem. 23, 503–518. doi:10.1016/j.apgeochem.2007.12.020
Mitchell, C. P. J., Branfireun, B. A., and Kolka, R. K. (2008a). Total mercury and methylmercury dynamics in upland-peatland watersheds during snowmelt. Biogeochemistry 90, 225–241. doi:10.1007/s10533-008-9246-z
Mu, C. C., Schuster, P. F., Abbott, B. W., Kang, S. C., Guo, J. M., Sun, S. W., et al. (2020). Permafrost degradation enhances the risk of mercury release on Qinghai-Tibetan Plateau. Sci. Total Environ. 708. doi:10.1016/j.scitotenv.2019.135127
Paranjape, A. R., and Hall, B. D. (2017). Recent advances in the study of mercury methylation in aquatic systems. Facets 2, 85–119. doi:10.1139/facets-2016-0027
Ramlal, P. S., Kelly, C. A., Rudd, J. W. M., and Furutani, A. (1993). Sites of methyl mercury production in remote Canadian shield. Can. J. Fish. Aquatic Sci. 50, 972–979. doi:10.1139/f93-112
Ravichandran, M. (2004). Interactions between mercury and dissolved organic matter: A review. Chemosphere 55, 319–331. doi:10.1016/j.chemosphere.2003.11.011
Rydin, H., Jeglum, J. K., and Bennett, K. D. (2013). The biology of peatlands. Oxford, United Kingdom: Oxford University Press.
Schaefer, K., Elshorbany, Y., Jafarov, E., Schuster, P. F., Striegl, R. G., Wickland, K. P., et al. (2020). Potential impacts of mercury released from thawing permafrost. Nat. Commun. 11, 4650. doi:10.1038/s41467-020-18398-5
Schuur, E. A. G., McGuire, A. D., Schadel, C., Grosse, G., Harden, J. W., Hayes, D. J., et al. (2015). Climate change and the permafrost carbon feedback. Nature 520, 171–179. doi:10.1038/nature14338
Skyllberg, U., (2008). Competition among thiols and inorganic sulfides and polysulfides for Hg and MeHg in wetland soils and sediments under suboxic conditions: Illumination of controversies and implications for MeHg net production. J. Geophys. Research:Biogeosciences, 113, G00C03. doi:10.1029/2008JG000745
St Pierre, K. A., Chetelat, J., Yumvihoze, E., and Poulain, A. J. (2014). Temperature and the sulfur cycle control monomethylmercury cycling in high Arctic coastal marine sediments from Allen Bay, Nunavut, Canada. Environ. Sci. Technol. 48, 2680–2687. doi:10.1021/es405253g
Streets, D. G., Horowitz, H. M., Lu, Z. F., Levin, L., Thackray, C. P., and Sunderland, E. M. (2019). Global and regional trends in mercury emissions and concentrations. Atmos. Environ. 201, 417–427. doi:10.1016/j.atmosenv.2018.12.031
Tarnocai, C. (2009). The impact of climate change on Canadian peatlands. Can. Water Resour. J. 34, 453–466. doi:10.4296/cwrj3404453
Ullrich, S. M., Tanton, T. W., and Abdrashitova, S. A. (2001). Mercury in the aquatic environment: A review of factors affecting methylation. Crit. Rev. Environ. Sci. Technol. 31, 241–293. doi:10.1080/20016491089226
US EPA (1996). “Sampling ambient water for trace metals at EPA water quality criteria levels, ” in U.S. environmental protection agency office of water engineering and analysis division (4303) (Washington, D. C.) 20460.
Weishaar, J. L., Aiken, G. R., Bergamaschi, B. A., Fram, M. S., Fujii, R., and Mopper, K. (2003). Evaluation of specific ultraviolet absorbance as an indicator of the chemical composition and reactivity of dissolved organic carbon. Environ. Sci. Technol. 37, 4702–4708. doi:10.1021/es030360x
Wu, J. H., and Roulet, N. T. (2014). Climate change reduces the capacity of northern peatlands to absorb the atmospheric carbon dioxide: The different responses of bogs and fens. Glob. Biogeochem. Cycles 28, 1005–1024. doi:10.1002/2014gb004845
Xia, K., Skyllberg, U. L., Bleam, W. F., Bloom, P. R., Nater, E. A., and Helmke, P. A. (1999). X-ray absorption spectroscopic evidence for the complexation of Hg (II) by reduced sulfur in soil humic substances. Environ. Sci. Technol. 33, 257–261. doi:10.1021/es980433q
Yang, Z. M., Fang, W., Lu, X., Sheng, G. P., Graham, D. E., Liang, L. Y., et al. (2016). Warming increases methylmercury production in an Arctic soil. Environ. Pollut. 214, 504–509. doi:10.1016/j.envpol.2016.04.069
Yu, R. Q., Adatto, I., Montesdeoca, M. R., Driscoll, C. T., Hines, M. E., and Barkay, T. (2010). Mercury methylation in Sphagnum moss mats and its association with sulfate-reducing bacteria in an acidic Adirondack forest lake wetland. FEMS Microbiol. Ecol. 74, 655–668. doi:10.1111/j.1574-6941.2010.00978.x
Keywords: mercury, methylmercury, climate change, wetland, sulfate, dissolved organic matter
Citation: Sun T, Lindo Z and Branfireun BA (2023) Ground warming releases inorganic mercury and increases net methylmercury production in two boreal peatland types. Front. Environ. Sci. 11:1100443. doi: 10.3389/fenvs.2023.1100443
Received: 16 November 2022; Accepted: 06 March 2023;
Published: 14 April 2023.
Edited by:
Andrea G. Bravo, Institute of Marine Sciences (CSIC), SpainReviewed by:
Bo Meng, Institute of Geochemistry (CAS), ChinaAmanda J. Barker, Cold Regions Research and Engineering Laboratory, United States
Douglas Burns, United States Geological Survey (USGS), United States
Copyright © 2023 Sun, Lindo and Branfireun. This is an open-access article distributed under the terms of the Creative Commons Attribution License (CC BY). The use, distribution or reproduction in other forums is permitted, provided the original author(s) and the copyright owner(s) are credited and that the original publication in this journal is cited, in accordance with accepted academic practice. No use, distribution or reproduction is permitted which does not comply with these terms.
*Correspondence: Brian A. Branfireun, bbranfir@uwo.ca