- 1Department of Chemistry, College of Science, Southern University of Science and Technology, Shenzhen, China
- 2School of Environment and Energy, Shenzhen Graduate School of Peking University, Shenzhen, China
- 3School of Innovation and Entrepreneurship, Southern University of Science and Technology, Shenzhen, China
Highly efficient, economic feasible and environmentally friendly adsorbents have been a research hotspot for Cd(Ⅱ) sequestration. In this study, various nonliving marine diatom biomass were prepared for Cd(Ⅱ) removal. The Cd(Ⅱ) adsorption properties of the diatom biomass was unveiled by adsorption kinetic, isotherm and thermodynamic analysis, and the adsorption mechanism was revealed using spectrometric identification methods. Results suggested that nonliving marine diatom biomass (Chaetoceros, Nitzschia, and Thalassiosira sp.) could efficiently remove Cd(Ⅱ) from aqueous solution, with nonliving Nitzschia possessing the highest adsorption capacity. The adsorption capacity of nonliving Nitzschia was 289–430 mg g−1 (288–308 K) in the absence of ionic competition and under low turbidity conditions, which was much higher than that of most of adsorbents of great concern. The kinetic, isotherm and thermodynamic analysis suggested the adsorption of Cd(Ⅱ) was a spontaneous, endothermic and chemisorption involved process. Moreover, inorganic salts and turbidity showed negative effects on Cd(Ⅱ) adsorption, whereas humic acid showed a positive effect. Adsorption mechanism analysis demonstrated that amine and pyrrolic nitrogen groups were responsible for Cd(Ⅱ) adsorption. It is also worth noting that organics leaching during adsorption would also affect chemical bonds distribution on the biomass, which should be taken into account when elucidating the heavy metal adsorption mechanism of bio-adsorbents.
1 Introduction
Cadmium, one of the toxic and commonly used heavy metals, is released into the environment through a number of anthropogenic activities, including the manufacturing of batteries, pigments, screens, and the combustion of fossil fuels. (Ozer and Pirinççi, 2006). Cadmium ions are not only present in aquatic solutions but can also get concentrated through food trains (e.g., rice). Drinking water or eating food containing cadmium can cause a series of health problems (Dinesh and Singh, 2002).
Due to the toxic implications of cadmium ions, the removal of cadmium from aqueous environments is a focus of many ongoing research studies. Among the techniques, adsorption has been proven to be one of the most efficient, popular, and cost-effective methods for cadmium ion removal from an aqueous solution (Burakov et al., 2018). With increasing emphasis on sustainable development, economically feasible and environmentally friendly adsorbents have become a research hotspot (Zhou et al., 2018). Bio-adsorbents have been widely reported as efficient and eco-friendly adsorbents for heavy-metal sequestration, which includes algae, bacteria, fungi, natural organic matters, and agroforestry wastes (Bilal et al., 2013; He and Chen, 2014; Zeraatkar et al., 2016). Among these adsorbents, algae biomass is a great alternative bio-adsorbent for heavy metal removal because algae can use carbon dioxide as a carbon source via photosynthesis, which makes it more eco-friendly and sustainable than other microorganism adsorbents (Shang et al., 2015).
The algae species used for cadmium adsorption mainly include living and non-living green, red, and brown algae (Sheng et al., 2004; He and Chen, 2014; Suresh et al., 2015). There is more inclination to use marine algae than freshwater algae to treat heavy-metal wastewater because freshwater shortage has become a worldwide issue. Marine macroalgae, including green algae, red algae, and brown algae, were widely reported as effective adsorbents for Cd(Ⅱ) sequestration from aqueous solution (Davis et al., 2003; Ibrahim, 2011; He and Chen, 2014). However, very few studies concerning heavy-metal removal by marine microalgae, which are very widespread in the ocean, were conducted. Diatom, which is a common microalgae species in the ocean and accounts for over 40% of marine primary production, contributes considerable biomass to the marine ecosystem (Armbrust, 2009; Ma et al., 2018). The cell wall of a diatom mainly consists of C, O, N, Si, S, and P elements and possesses abundant chemical bonds, including -OH, -COOH, -COOM, -NH2, -R2NH, -SO-, -PO-, -HC=O, R2C=O, -NO-, -CH3, and -CH2- (Ma et al., 2018). Moreover, a silicon skeleton with an abundant microporous structure consisting of the cell wall of a diatom would make the diatom provide more adsorption sites than other algae species. From these aspects, it is likely that marine diatoms possess great potential to be an efficient and economic bio-adsorbent for heavy-metal ion removal. At present, only a few studies focus on the physiological and biochemical responses of freshwater diatom to metal ions, which reveals that some living diatom species can uptake and tolerate some heavy metal ions (Morin et al., 2008; Guo et al., 2010; Ding et al., 2012; Ma et al., 2018).
Therefore, in the present study, the potentials of three non-living marine diatom species (Chaetoceros sp., Nitzschia sp., and Thalassiosira sp.) have been examined for sequestration of Cd(II) from aqueous solution. The adsorption process was analyzed using various kinetic, isothermal, and thermodynamic models. Meanwhile, influences of environmental substances, including inorganics, turbidity, and humic acids, on the adsorption were also investigated. Moreover, the mechanisms of Cd(II) adsorption of dry inactivated biomass of marine diatom were fully studied. This is among the first studies to unveil the potential of various kinds of non-living marine diatom biomass species to adsorb Cd(II) from an aqueous solution.
2 Materials and methods
2.1 Preparation of bio-adsorbent
The three pure marine diatom strains, which belong to Chaetoceros sp., Nitzschia sp., and Thalassiosira sp., respectively, were isolated from Dapeng Bay of Shenzhen City, China. The cultivations of pure marine diatom were carried out in f/2 media (Guillard et al., 1975) under aeration conditions and harvested at the end of the stationary phase. The harvested diatom solution was centrifuged at 4,000 r/min for 10 min (Hettich UNIVERSAL 5810R, Germany). The collected biomass was re-suspended in deionized water and centrifuged under the same condition three times to remove the impurities in the culture. Then, the diatom biomass was freeze-dried in a refrigerated compressed air dryer (Labconco FreeZone 2.5, United States). The obtained dry biomass samples were maintained in a drying basin for the preparation experiments.
2.2 Batch Cd(Ⅱ) adsorption experiments
Batch adsorption experiments were carried out in plastic centrifuge tubes containing 50 mL metal ion solution with known initial concentrations varying from 10 to 700 mg/L. The adsorbent dose was set to 1 g/L, and the pH of the metal ion solution was 6.4. The tubes were agitated in a shaker at 100 rpm and subjected to a temperature of 25°C for 24 h to ensure the adsorption reached equilibrium. The sample was taken from each tube at various intervals (5, 10, 15, 20, 30, 60, 120, 360, 720, and 1440 min). The samples were then centrifuged at 4,000 rpm for 10 min, and the supernatants were filtered (0.22 μm). The resulting solution was diluted with distilled water and then used for heavy-metal concentration measurement (ICP, Shimadzu ICPE-9000, Japan), and each measurement has three parallel samples in order to reduce the random errors. All experiments were performed twice. In order to investigate the influences of co-existing substances in natural water on Cd(II) adsorption by the biomass, the adsorption assays under different levels of common cations in water (Na+, Ca2+, and Mg2+), turbidity, and humic acid were also conducted, respectively.
2.3 Characterization of bio-adsorbent
The surface morphology of adsorbents was examined using a scanning electron microscope equipped with an energy-dispersive X-ray analyzer (Hitachi, S4800, Japan). The surface chemical groups of the adsorbents were identified using Fourier-transform infrared (FTIR) spectroscopy (KBr pellets; IR Prestige-21 FTIR spectrometer, Shimadzu, Japan) in the range 400–4,000 cm−1. The Brunauer–Emmett–Teller (BET) surface areas and pore size distributions of the adsorbents were determined using a TriStar II 3020 series surface area and porosity analyzer (Micromeritics Instrument Corporation, United States). The chemical states of atoms on the surface of the adsorbents before and after Cd(II) adsorption were analyzed using X-ray photoelectron spectroscopy (XPS; PH 1800, Japan).
2.4 Analysis
2.4.1 Adsorption amount of Cd(II)
The adsorption amount of Cd(II) per unit mass of the diatom biomass at equilibrium (qe, mg g−1) and the adsorption amount of Cd(II) per unit mass of adsorbent at time t (qt, mg g−1) were determined as follows:
where C0 (mg L−1), Ct (mg L−1), and Ce (mg L−1) are the heavy-metal concentrations at time zero, time t, and at equilibrium, respectively; V is the volume of the solution (L); and W is the mass of dry adsorbent used (g).
2.4.2 Kinetic study
Pseudo-second-order model is widely used for bio-adsorbent to predict the adsorption kinetics. The non-linear form of the pseudo-second-order equation is given by
where qe and qt are the amounts of solute adsorbed (mg g−1) at equilibrium and at any time t (min), respectively. k2 is the rate constant for pseudo-second-order reaction (g mg−1 min−1). The straight line plots of 1/qt versus 1/t are used to obtain the constants for the pseudo-second-order reaction.
2.4.3 Adsorption isotherms
The most common adsorption isotherm models, Langmuir and Freundlich, were applied to predict the equilibrium parameters of the Cd(II) adsorption process on the diatom biomass.
The non-linear form of the Langmuir isotherm model can be expressed as
where qe is the amount of Cd(II) adsorbed at equilibrium (mg g−1), qm is the maximum adsorption capacity (mg g−1), Ce is the equilibrium concentration of metal ions in solution (mg L−1), and KL is the Langmuir constant that is related to the free energy of adsorption (L mg−1).
The non-linear form of the Freundlich isotherm model is given as
where qe is the amount of Cd(II) adsorbed at equilibrium (mg g−1), Ce is the equilibrium concentration of metal ions in solution (mg L−1), KF is the Freundlich constant (mg L−1) that is related to the adsorption capacity of the sorbent, and n is the Freundlich constant related to the adsorption intensity (dimensionless) of adsorbent.
2.4.4 Adsorption thermodynamics
The sorption isotherms of Cd(II) on diatom biomass at 288 K, 298 K, and 308 K were obtained to determine the thermodynamic parameters. The standard Gibbs free energy change (△G), standard enthalpy change (△H), and standard entropy change (△S) were determined from KL and T by the following equations:
where R is the universal gas constant (8.314 × 10−3 kJ mol−1 K−1), and T is the absolute temperature (K). K0 is the thermodynamic equilibrium constant. Values of K0 are obtained by plotting ln (qe/Ce) versus qe. The intercept of the fitting line with the vertical axis gives the values of K0 (Niwas et al., 2000). △H was obtained from the slope of the linear plot of lnKL against 1/T, and △S was obtained from Eq. 8.
3 Results and discussion
3.1 Characteristics of non-living marine diatom biomass
3.1.1 Cell morphology and composition of the biomass
The cell morphology of Chaetoceros sp., Nitzschia sp., and Thalassiosira sp. strains were measured using a fluorescent microscope. It can be seen from Figure 1 that the cell of Chaetoceros sp. was almost round or cylinder-shaped with several strip-shaped matters attached to the cell. In comparison, the cells of Nitzschia sp. and Thalassiosira sp. were rhombus- and cylinder-shaped, respectively.
As shown in Figure 2A, both the non-living biomass of Chaetoceros sp. and Thalassiosira sp. consisted of C, O, N, Si, Na, Cl, and S, with a little difference in the atomic ratio of these elements. In comparison, the non-living biomass of Nitzschia sp. contained Ca and Mg elements except for C, O, N, Si, Na, Cl, and S, which suggested that the chemical composition on the surface of Nitzschia sp. was significantly different from that of Chaetoceros sp. and Thalassiosira sp.
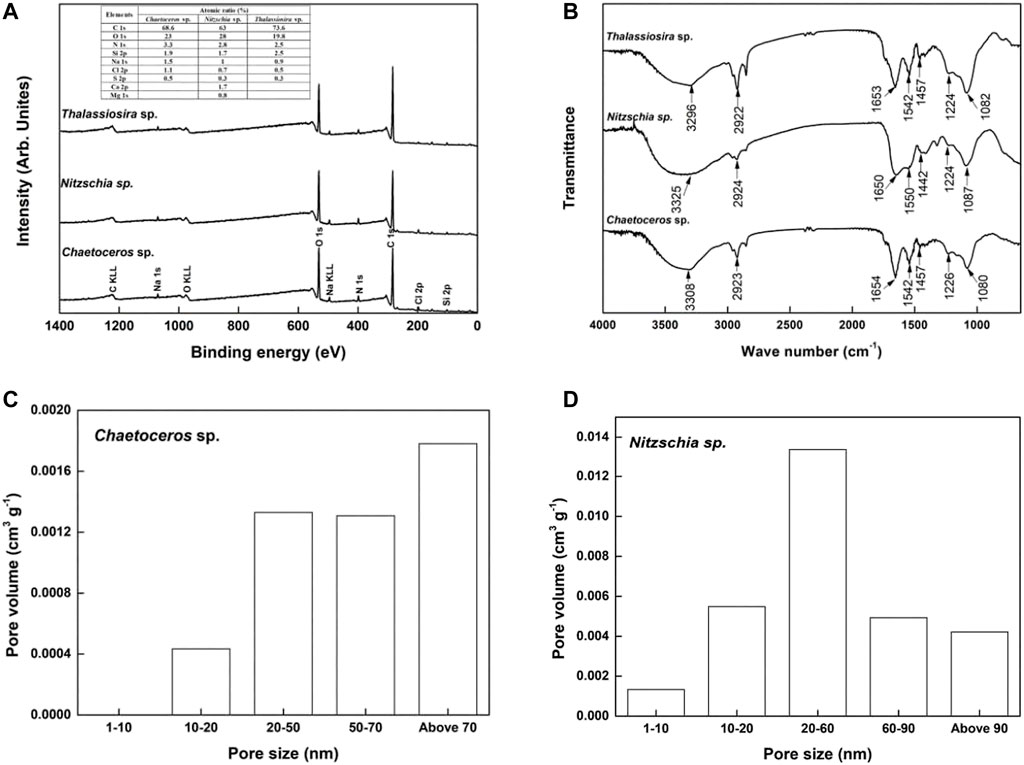
FIGURE 2. Properties of non-living diatom biomass. (A) XPS spectrum, (B) FTIR spectrum, and (C–D) Pore size distribution.
3.1.2 Functional groups of the biomass
FTIR spectra of the non-living diatom biomass were visualized to obtain information about the chemical bonds presented on the surface of the biomass. The chemical bond information corresponding to each FTIR peak was obtained mainly according to published references (Zhu, 2005; Robert et al., 2007). As shown in Figure 2B, the shapes of FTIR spectra for non-living Chaetoceros sp. and Nitzschia sp., and Thalassiosira sp. biomass were similar, which implies that these three diatom biomasses possess similar chemical groups on the surface. A broad and strong peak (3,308 cm−1 for Chaetoceros sp., 3,325 cm−1 for Nitzschia sp., and 3,296 cm−1 for Thalassiosira sp.) assigned to the overlapping of –OH and –NH stretching bands of polymeric compounds. The peaks at around 2,923 cm−1 represented the stretching vibration of –CH3. The peaks at around 1,653 cm−1 were due to the overlapping of C=O stretching vibration and C=C bonds, respectively. The peaks at around 1,550 and 1,450 cm−1 could be assigned to the stretching vibration of N–H and variable angle vibration of–CH2–, respectively. The peak at around 1,080 cm−1 represented the overlapping of C–O vibration in the alcoholic hydroxyl group and Si–O–Si stretching vibration. These results indicated that the non-living biomass of Chaetoceros sp., Nitzschia sp., and Thalassiosira sp. contained diverse chemical groups, such as carbonyl, hydroxyl, and amine groups, on its surface, which were potential binding sites for the heavy-metal ion (González et al., 2011; He and Chen, 2014).
3.1.3 Surface area and porous distribution of the biomass
The BET surface area of non-living Chaetoceros and Nitzschia were 0.7 and 4.6 m2 g−1, respectively, while the BET surface area of non-living Thalassiosira was not detected under the same weight condition as the other two species. This result suggested that Nitzschia possesses the highest surface area, followed by Chaetoceros, and Thalassiosira possesses the lowest. The cumulative pore volume of non-living Chaetoceros and Nitzschia were 0.0048 and 0.0293 cm3 g−1, respectively. Moreover, it is clear from Figure 2D that mesopores took up the majority of pore volume on the surface of non-living Nitzschia, followed by macropores, and micropores took up the least. In comparison, the surface of non-living Chaetoceros was occupied by macropores, followed by mesopores, with no micropores on it (Figure 2C). These results indicated that non-living Nitzschia biomass possesses a more abundant pores structure than the other two diatom species.
3.2 Adsorption behavior of Cd(II) on non-living marine diatom biomass
3.2.1 Adsorption kinetics
As shown in Figure 3 and Table 1, the results show that Cd(II) adsorption by all the diatom biomass fit the pseudo-second-order model very well, with a correlation coefficient R2 > 0.88. For initial Cd(II) concentration of 10 and 120 mg L−1, the equilibrium adsorption amount of Cd(II) by Chaetoceros sp., Nitzschia sp., and Thalassiosira sp. were calculated to be 6.14, 6.14, and 5.53 mg g−1 and 111, 106, and 103 mg g−1, respectively, which were in good agreement with the experimental capacities of 6.25, 6.15, and 5.69 mg g−1 and 113, 114, and 107 mg g−1, respectively. The suitability of the pseudo-second-order model indicates that chemical interaction is the rate-controlling step in the whole adsorption process, and the adsorption behavior involves valence forces through sharing of electrons between metal ions and the diatom biomass. Furthermore, the tendency of the adsorption rate constant (k2) was calculated according to the model as follows: Nitzschia sp. > Chaetoceros sp. > Thalassiosira sp., suggesting that Cd(II) was adsorbed fastest by non-living Nitzschia, followed by non-living Chaetoceros, and the slowest by non-living Thalassiosira.
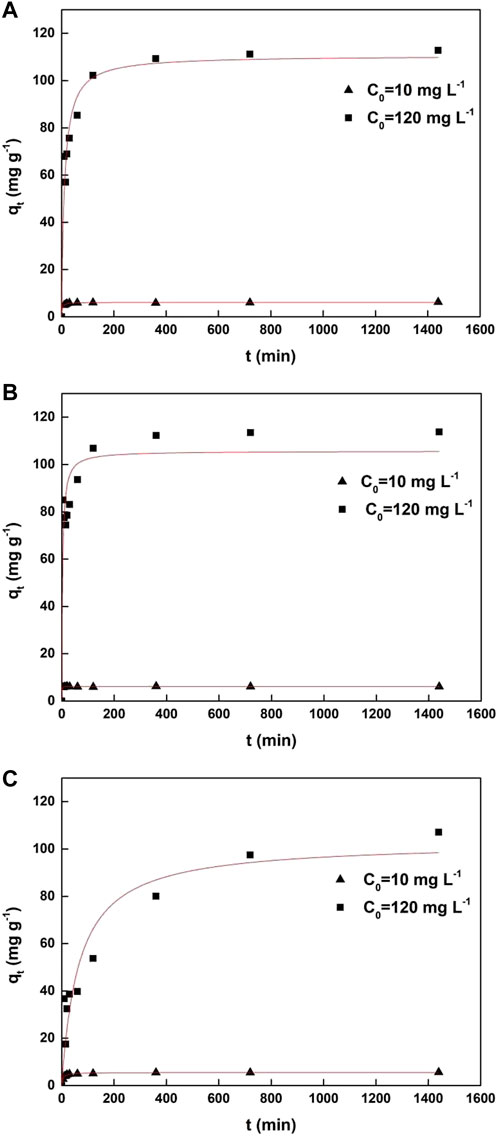
FIGURE 3. Pseudo-second-order kinetic models of non-linear fitting for Cd(II) adsorption by non-living marine diatom biomass. (A) Chaetoceros sp., (B) Nitzschia sp., and (C) Thalassiosira sp.
3.2.2 Adsorption isotherms
The Cd(II) equilibrium adsorption data were fit to the non-linear Langmuir and Freundlich adsorption isotherm models (Figure 4). The calculated parameters corresponding to the two models were summarized in Table 2. For all temperature conditions (T = 288, 298, and 308 K), based on the values of correlation coefficient (R2), the adsorption data of Cd(II) by three diatom biomass fit both the Langmuir and Freundlich models well. Furthermore, for every non-living diatom biomass, the Langmuir constant (KL) generally increased with temperature, which indicates that the adsorption of Cd(II) by the diatom biomass was an endothermic reaction process and higher temperature within some range favors the adsorption. The generally higher values of Freundlich constants (KF and n) under higher temperatures also indicated that the adsorption processes of the three diatom biomass can be promoted by temperature.
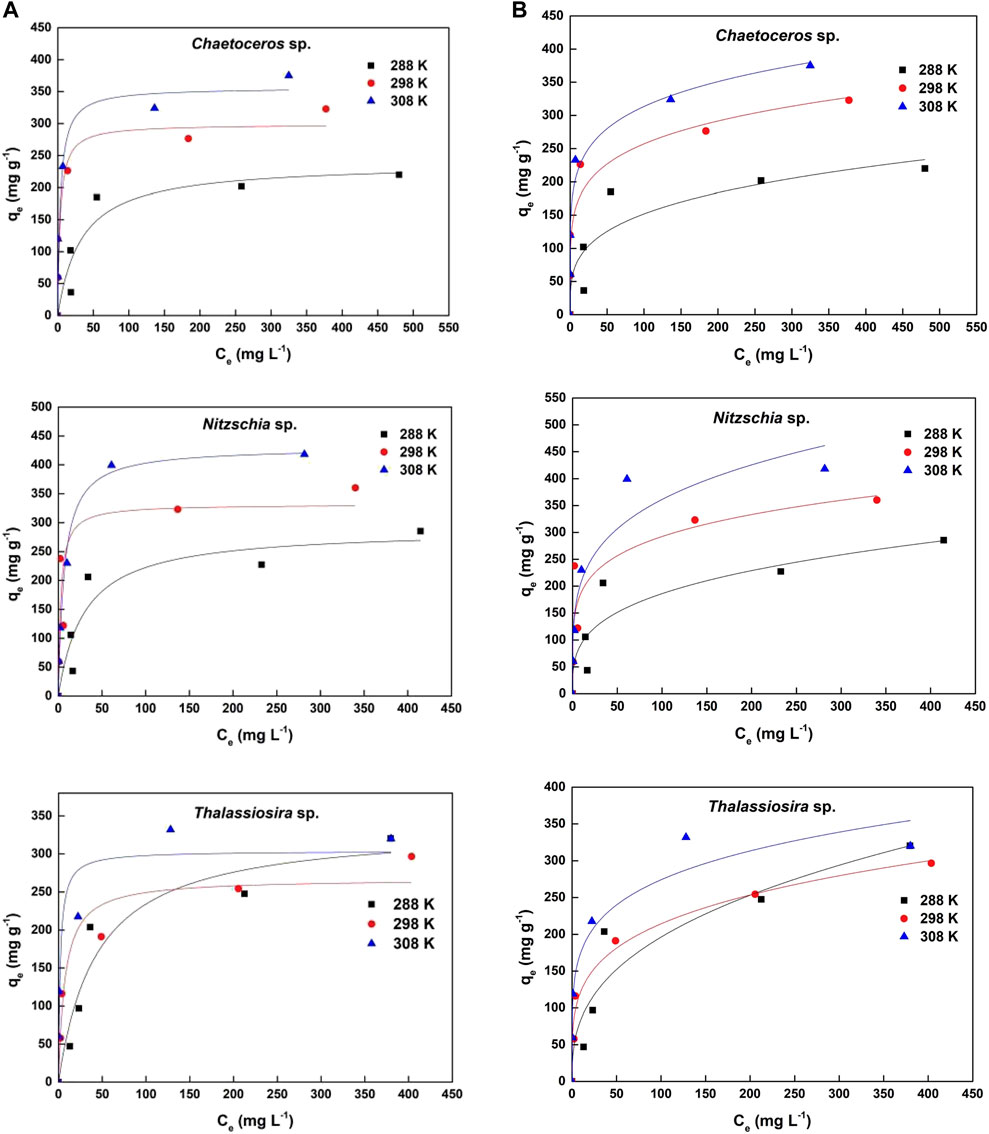
FIGURE 4. (A) Langmuir isotherm and (B) Freundlich isotherm non-linear fittings for Cd(II) adsorption by three kinds of non-living marine diatom biomass.
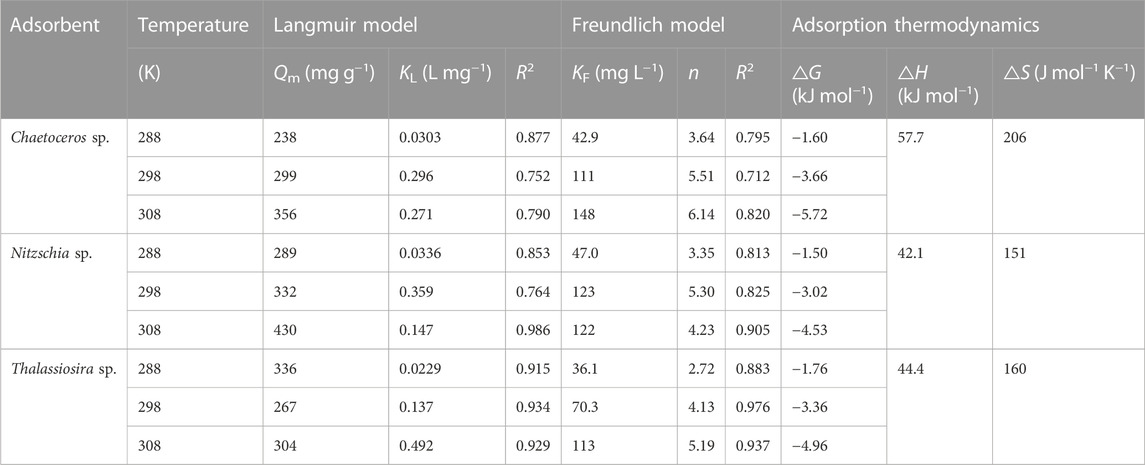
TABLE 2. Non-linear fit parameters of Langmuir and Freundlich models and thermodynamic parameters for Cd(II) adsorption onto various non-living marine diatom biomass.
As shown in Table 2, for the temperature ranging from 288 K to 308 K, the theoretical qmax of Chaetoceros sp., Nitzschia sp., and Thalassiosira sp. were 238–356 mg g−1, 289–430 mg g−1, and 267–336 mg g−1, respectively, which indicates that Nitzschia sp. possesses better Cd(II) adsorption capacity than Chaetoceros sp. and Thalassiosira sp. The higher BET surface of non-living Nitzschia was probably one of the reasons for its higher adsorption capacity compared with the other two algae species. The highly efficient and newest adsorbents reported elsewhere were selected to compare with the non-living diatom biomass in the present study. As shown in Table 3, in the absence of competitive cations and under low turbidity conditions, the non-living marine diatom biomass in the present study possess much higher Cd(II) adsorption capacity than all the other reported algae species, and also most of the other kinds of bio-adsorbents. In comparison with other adsorbents except for bio-adsorbents, the dry marine diatom biomass still shows better Cd(II) adsorption capacity. Although some reported adsorbents, such as some clay-based materials, nanoscale particles, modified bio-adsorbents, and polymeric substances, showed higher Cd(II) adsorption capacity than the diatom biomass, there is still a long way to go to use these adsorbents in heavy-metal wastewater treatment in future because of complicated modification/preparation processes applied for those adsorbents (Uddin, 2017; Chen et al., 2018). In addition, nano-materials always face difficult separation and potential biological safety risk problems, which make it hard to apply in wastewater treatment. In comparison, marine diatom biomass was not only highly efficient in adsorbing cadmium ions from an aqueous solution, but it is also easy to obtain and eco-friendly. From these aspects, the dry marine diatom biomass would be a competitive adsorbent used for cadmium wastewater in the future.
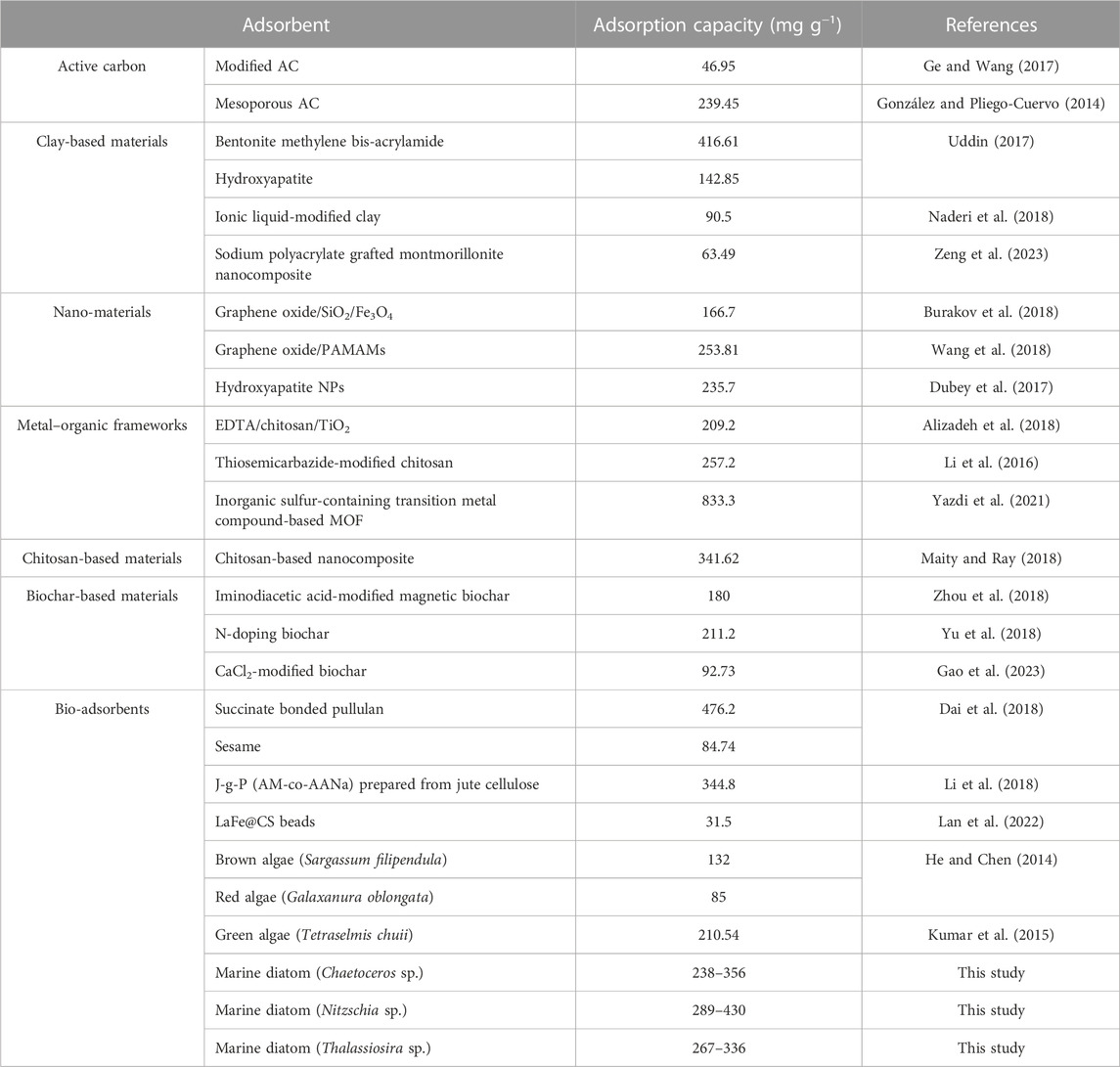
TABLE 3. Comparisons of Cd adsorption capacities between non-living marine diatom biomass and highly efficient adsorbents reported elsewhere.
3.2.3 Adsorption thermodynamics
The thermodynamics parameters, the enthalpy change (△H) and entropy (△S), were calculated from the linear plot of ln K0 versus 1/T and are given in Table 2. The values of △H were positive for all adsorption cases, which indicates that the adsorption process was endothermic and Cd(II) ions were adsorbed more efficiently on the diatom biomass under higher temperatures. The value of △H can be used to differentiate chemisorption and physisorption. It was reported by Wang et al. (2012) and Sun et al. (2015) that the adsorption process was mainly physisorptive when △H < 20 kJ mol−1 and was mainly chemisorptive when 80 <△H < 400 kJ mol−1 or 40 <△H < 120 kJ mol−1. Therefore, it can be inferred from the △H values for the three diatom biomass that the Cd(II) adsorption processes of non-living Chaetoceros sp., Nitzschia sp., and Thalassiosira sp. were dominated by chemisorption.
The positive value of △S indicates that there is an increase in the randomness in the system solid/solution interface during the adsorption process (Xie et al., 2011). The positive entropy change was possibly stemming from the changes taking place in the structure of the adsorbent and dispersing of water molecules from Cd(II) ions surrounded by water molecules during the adsorption process (Ozer and Pirinççi, 2006). Negative values of △G for three non-living diatom biomass indicated the spontaneous nature of the reaction between Cd(II) and the biomass. The values of △G became more negative with temperature, which meant that the adsorption process was more favorable at high temperatures.
3.3 Influences of co-existing substances in natural water on adsorption capacity of Cd(II) by non-living diatom biomass
3.3.1 Inorganic salts
Investigation of the influences of Na+, Ca2+, and Mg2+ on Cd(II) removal is of great importance due to their general existence in natural water and wastewater. As shown in Figure 5A, the amount of Cd(II) adsorbed by non-living Nitzschia decreased sharply from 108.1 mg g−1 to below 30 mg g−1 when Na+, Ca2+, and Mg2+ at 0.05 mol L−1 were, respectively, added into the adsorption system. As the concentration of Na+, Ca2+, and Mg2+ increases to 0.05–0.2 mol L−1, the amount of Cd(II) adsorbed by non-living Nitzschia decreased by 68.7%–88.95%, 83.3%–95.3%, and 75.1%–92.2%, respectively. According to the significance analysis results by SPSS software, the decreased extent of the amount of Cd(II) adsorbed by the biomass shows no significant correlation to the type of foreign ions (p > 0.05), suggesting similar influences of Na+, Ca2+, and Mg2+ in the range from 0.05 to 0.2 mol L−1 on the amount of Cd(II) adsorbed by non-living Nitzschia. The effects of foreign ions (Na+, Ca2+, and Mg2+) on the adsorption of cadmium ions are mainly ascribed to 1) foreign ions competing with Cd(II) for the limited reactive sites on the surface of non-living Nitzschia, which reduces the adsorption amount of the adsorbates; 2) ionic strength of Na+, Ca2+, and Mg2+ impacting non-living Nitzschia particles aggregation by influencing electrostatic interactions; and 3) ionic strength affecting the activity coefficient of Cd(II) and thus limiting the cadmium ions transfer from the solution to the surface of adsorbent (Peng et al., 2017). Another explanation for the negative effects of Ca2+ and Mg2+ on the adsorption capacity of adsorbents was that both Ca2+ and Mg2+ in high concentration (>400 mg L−1) can form hydrates with water “Ca2+/Mg2+-(H2O)n”, and these complexes could cover the surface of the adsorbents, making it less available to adsorbates, and resulting in a decreased number of heavy-metal ions adsorbed (Cheng et al., 2014). It is also noted from the results that the decreased extent of the amount of Cd(II) adsorbed by non-living Nitzschia shows no significant correlation to the concentration of foreign ions from 0.05 to 0.2 mol L−1 (p > 0.05). This would be explained by the possibility that the minimum concentration of the foreign ions (0.05 mol L−1) was high enough to make the adsorption capacity decrease to the lowest level.
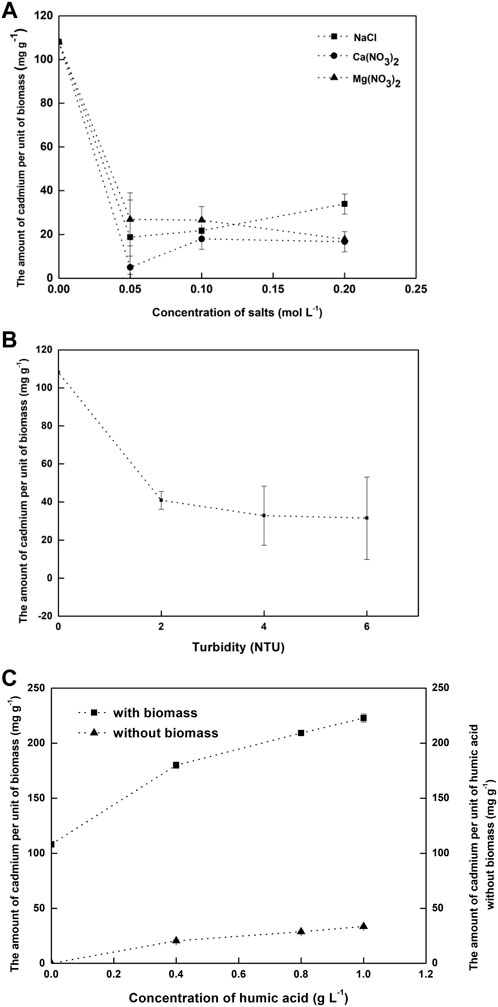
FIGURE 5. Influences of (A) salts, (B) turbidity, and (C) humic acid on the adsorption amount of Cd(II) by non-living Nitzschia.
Although Ca2+ and Mg2+ showed negative effects on the amount of Cd(II) adsorbed by the diatom biomass, the concentration of Ca2+ and Mg2+ is detected in the range of 10–80 mg L−1 (Ca2+: 0.00025–0.0020 mol L−1, Mg2+: 0.0004–0.0033 mol L−1) in the surface freshwater (Cheng et al., 2014), which was far lower than the concentration set in this study. Therefore, it is likely that the adsorption of Cd(II) would be far less affected than the extent shown in the present study when using non-living Nitzschia for Cd(II) treatment in surface freshwater.
3.3.2 Turbidity
Figure 5B shows the effect of turbidity on the amount of Cd(II) adsorbed by non-living Nitzschia. The amount of Cd(II) per unit mass of non-living Nitzschia sharply decreased by 62.2% when the turbidity increased to 2 NTU and tended to be stable afterward. The suspended particles might have blocked the micro-pores on the surface of non-living Nitzschia and occupied the active sites for Cd(II) adsorption, therefore resulting in the decreased adsorption amount of Cd(II).
3.3.3 Humic acid
Humic acid is a widespread organic macromolecule in the natural environment. It is usually applied in agricultural feed, the petrochemical industry, battery production, etc. It has been reported that humic acid can combine with cations through ionic bonding force or coordination interactions (Kinniburgh et al., 1999). As shown in Figure 5C, the amount of Cd(II) adsorbed by non-living Nitzschia increased evidently with the concentration of additive humic acids. A similar result was reported by Zeng et al. that the adsorption capacity of Cd(II) by the bentonite could be enhanced by humic acids (Zeng, 2008). Meanwhile, it can be seen from Figure 5C that humic acid was capable of adsorbing Cd(II) to some extent (around 25 mg g−1) in the absence of an adsorbent, which was similar to the results reported elsewhere. However, the significantly increased amount of Cd(II) adsorbed by non-living Nitzschia when humic acid was introduced cannot be fully explained by the small adsorption amount of Cd(II) by humic acid itself. As the surface of non-living Nitzschia contains various cations, such as Na+, Ca2+, Mg2+, and Cu2+, it can be inferred that the interactions between humic acid and those cations probably simultaneously occurred during the Cd(II) adsorption process. Therefore, the positive effect of humic acid for Cd(II) adsorption by non-living Nitzschia might be ascribed to 1) humic acid combining with some other cations (e.g., Na+, Ca2+, Mg2+, etc.) on the surface of non-living Nitzschia or in the solution, which makes more active sites available for Cd(II) adsorption. 2) Cd(II) was directly complexed by humic acid to form organic bonds with cadmium; 3) humic acid was first adsorbed onto the surface of non-living Nitzschia, and then complexation or ion exchange of Cd(II) with abundant functional groups of humic acids (amines, carboxylic acid, and carbonyl) took place.
3.4 Adsorption mechanism
Non-living Nitzschia, which possessed the highest Cd(II) adsorption capacity among the three diatom species, was used for adsorption mechanism analysis. Organics leaching was a common phenomenon for non-living bio-adsorbents during the adsorption process (Chen and Yang, 2005; Yang et al., 2011), which would possibly affect the distribution of chemical bonds on the bio-adsorbents. Therefore, in order to reveal the influence of organics leaching on the chemical bonds on the surface of non-living Nitzschia, FTIR and XPS analyses for distilled water-washed Nitzschia under the same conditions as the adsorption process were also conducted.
3.4.1 SEM
The surface morphologies of non-living Nitzschia biomass before and after Cd(II) adsorption were examined by SEM (Figure 6A). The non-living Nitzschia biomass shows an unconsolidated and flocky surface texture. After Cd(II) adsorption, the loose surface becomes caked and lumpish, which might be due to the complexation of cadmium ions with the functional chemical groups on the surface of non-living Nitzschia.
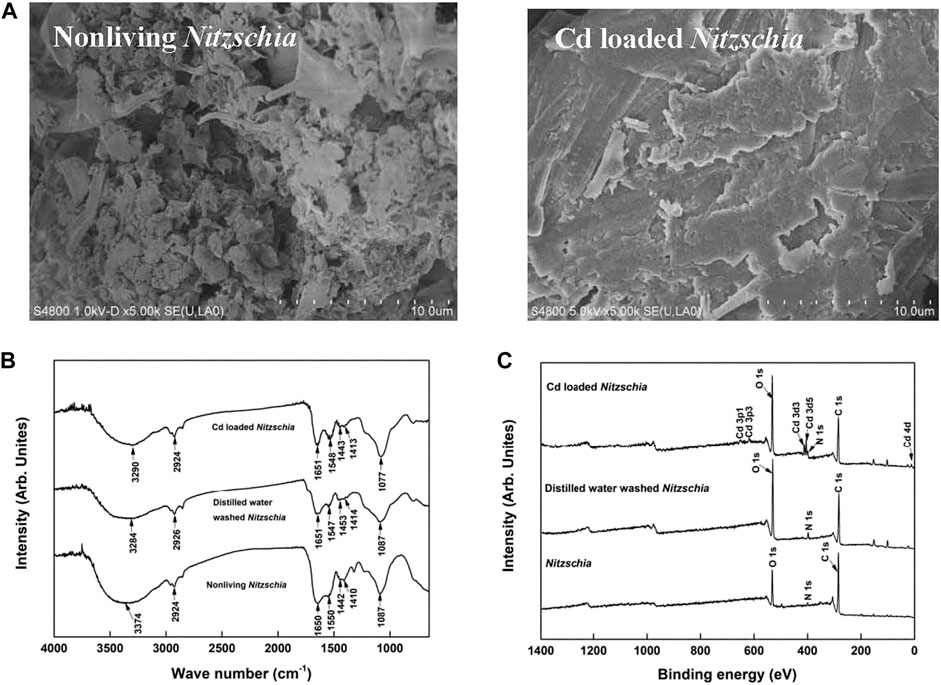
FIGURE 6. Characteristics of non-living, distilled water-washed, and Cd-loaded Nitzschia biomass. (A) SEM images, (B) FTIR spectra, and (C) XPS spectra.
3.4.2 FTIR
As shown in Figure 6B, after being washed with distilled water, the overall FTIR spectrum of Nitzschia biomass was similar to raw non-living Nitzschia, with an evident shift of the peak assigned to the overlapping of –OH and –NH stretching bands of polymeric compounds. The peak representing the overlap of C=C/C=O became a little narrow. These results suggested that the distribution of chemical groups such as –OH, –NH, C=C/C=O could also be affected by water washing, which was hardly mentioned in most of the reported studies (Yang et al., 2011; Zheng et al., 2011). In comparison, after Cd adsorption, an evident shift of the peak assigned to the overlapping of –OH and –NH stretching bands of polymeric compounds was observed, which was similar to the biomass sample being washed by distilled water. In contrast, the intensity of the peak assigned to –NH (1548 cm−1) became much stronger after Cd adsorption. This result implied that –NH bonds might be involved in the interaction with Cd(II) on the surface of the non-living Nitzschia.
3.4.3 XPS analysis
A comparison of XPS spectra survey scans among non-living Nitzschia, distilled water-washed Nitzschia, and Cd(II)-loaded Nitzschia is illustrated in Figure 6C. It can clearly be seen from the figure that the peaks representing the Cd chemical state occurred only in the XPS spectra of Cd(II)-loaded Nitzschia, which demonstrated that Cd(II) was adsorbed onto the surface of non-living Nitzschia. Additionally, it is also noted that the atomic ratio of C 1s on the surface of the non-living Nitzschia decreased from 76.5% to 56.5% after being washed by distilled water, indicating that the organic leaching happened when non-living Nitzschia mixed with water. A similar decrease of C 1s in Cd-loaded Nitzschia was also observed. In order to further understand the functional bonds responsible for Cd ion adsorption, binding energy (BE) profiles of carbon (C 1s), oxygen (O 1s), and nitrogen (N 1s) atoms on non-living Nitzschia, distilled water-washed, and Cd(II)-loaded Nitzschia are shown, respectively, in Figure 7, and the ratio of various C 1s, O 1s, and N 1s bonds which were calculated by peak area is shown in Table 4.
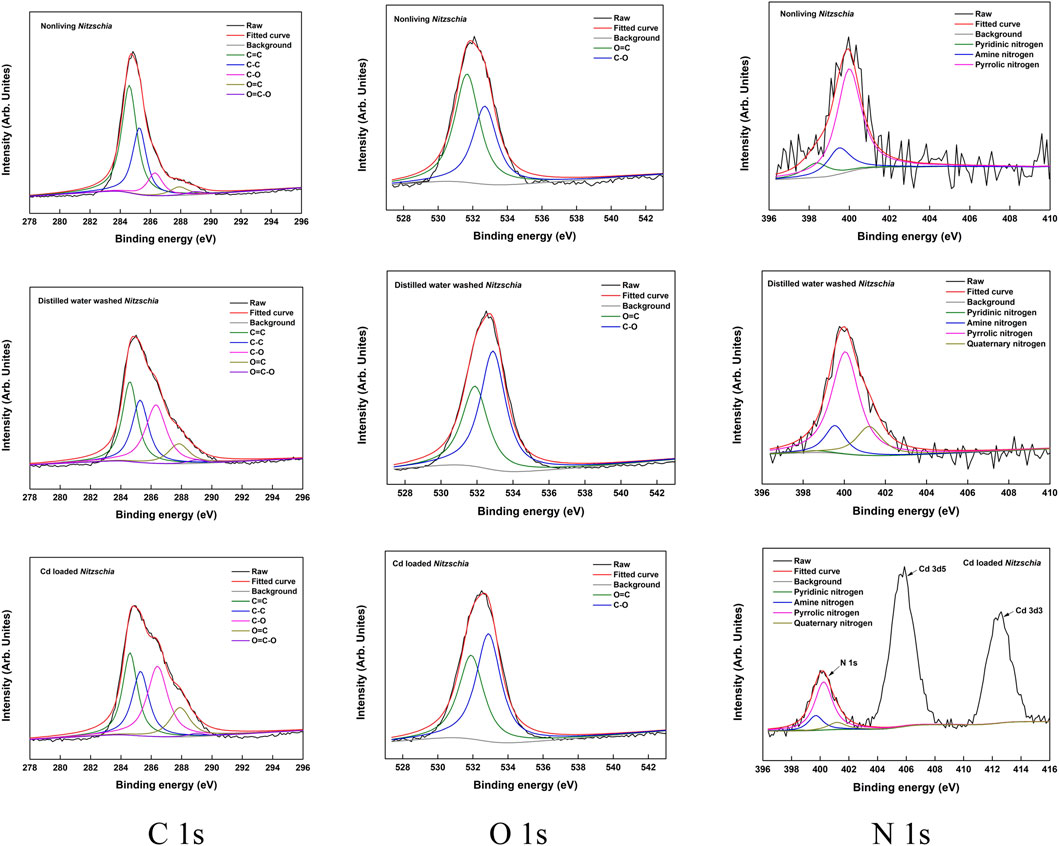
FIGURE 7. XPS spectra of C 1s, O 1s, and N 1s of non-living Nitzschia, distilled water-washed Nitzschia, and Cd-loaded Nitzschia.
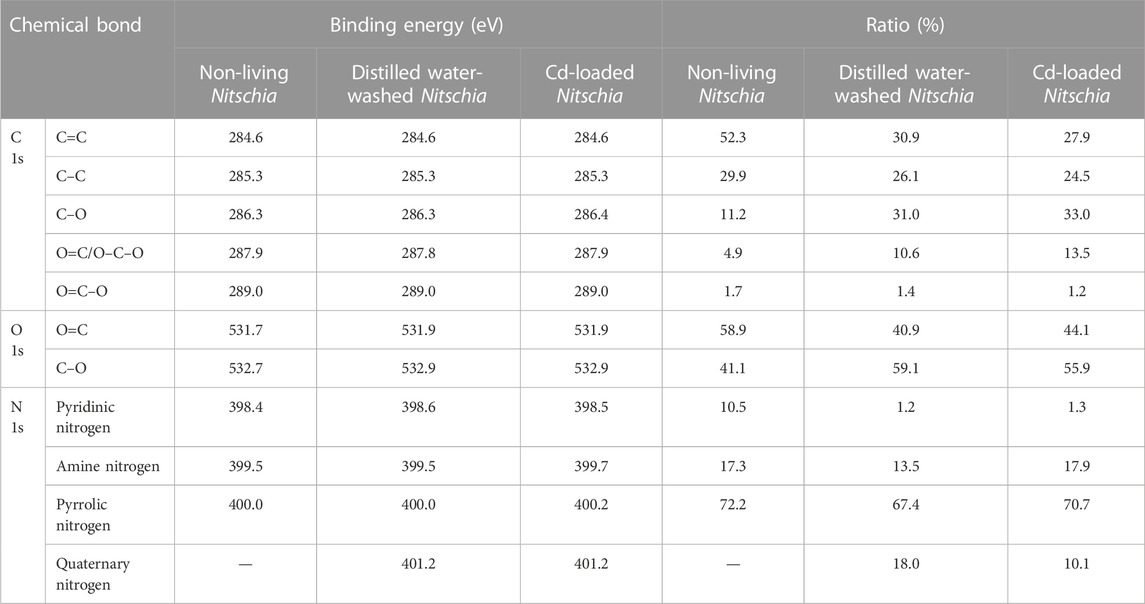
TABLE 4. Summary of binding energy and area ratios of C 1s, O 1s, and N 1s on non-living, distilled water-washed, and Cd-loaded Nitschia biomass.
The C 1s spectra of the non-living Nitzschia comprised five peaks with differentiated BE values (284. 6, 285.3, 286.3, 287.9, and 289 eV) via deconvolution. These peaks can be assigned to C=C, C–C, C–O, O=C, and O=C–O bonds, respectively (Beamson and Briggs, 1992; Yang et al., 2011; Deng et al., 2017). After Cd(II) adsorption, the ratio of C=C and C–C decreased, whereas the ratio of C–O and O=C increased, with no notable shifts of binding energy for the five carbon bonds. Meanwhile, after being mixed with distilled water, the changing trend of the carbon bonds was found to be very similar to the diatom sample after Cd(II) adsorption. Consequently, taking organics leaching into account, the decreases of C–C and C=C bonds after adsorption were due to organics leaching. In comparison, the decrease of C–C/C=C was also found for some bio-adsorbents after heavy-metal adsorption (Yang et al., 2011; Zheng et al., 2011). However, the decrease in the C–C/C=C ratio was attributed to partial breakage of the C–C/C=C bonds oxidized by heavy-metal ions (Zheng et al., 2011) or confirming the functional carbon bonds directly based on the change of C 1s after adsorption (Yang et al., 2011), without unveiling the effects of organics leaching on the chemical bonds of the adsorbent surface.
The O 1s spectra of the non-living comprised two peaks with differentiated BE values via deconvolution, which were assigned to O=C and C–O, respectively, with a higher ratio of O=C and a lower ratio of C–O bonds. After being washed with distilled water, the ratio of O=C was found to decrease by 18%, whereas the ratio of C–O increased correspondingly by 18%. Moreover, the binding energy (BE) value of O=C and C–O shifted to a higher level by 0.2 eV. This result suggested that organics leaching could also affect the ratio of oxygen bonds and induce BE shifts of oxygen bonds on Nitzschia. In previous studies, the complexation between O=C and heavy metal ions was usually confirmed by the results that O=C bonds on the bio-adsorbent decreased and C–O bonds increased after heavy-metal adsorption, in which the formation of O-heavy-metal bonds was not observed, and the effect of organics leaching on oxygen bonds was hardly mentioned (Chen and Yang, 2006; Yang et al., 2011; Li et al., 2012). Based on the present results, the involvement of O=C in heavy-metal complexation became ambiguous in those previous studies because organics leaching could also lead to the decrease of O=C and increase of C–O bonds. After Cd(II) adsorption, a decrease of O=C and an increase of C–O bonds were found compared with raw non-living Nitzschia with no new peak representing metallic oxygen observed, which was similar to the result of distilled-water-washed Nitzschia. Moreover, the binding energy (BE) value of O=C and C–O also shifted to a higher level by 0.2 eV compared with raw non-living Nitzschia. Therefore, it can be inferred that the change of oxygen bond distribution on Nitzschia after Cd(II) adsorption was due to organic leaching rather than the complexation of C=O with Cd(II).
The N 1s spectra of the non-living Nitzschia comprised three peaks with differentiated BE values via deconvolution, which can be assigned to pyridinic nitrogen, amine nitrogen, and pyrrolic nitrogen, respectively (Wang et al., 2017; Ji et al., 2018). After being washed with distilled water, the quantities of pyridinic nitrogen, amine nitrogen, and pyrrolic nitrogen decreased by 9.3, 3.8, and 4.8, respectively, and a new peak representing quaternary nitrogen of 18.0% occurred. This result indicated that some of the pyridinic nitrogen, amine nitrogen, and pyrrolic nitrogen were protonated in distilled water. In comparison, after Cd(II) adsorption, the ratio of pyridinic nitrogen decreased by 9.2%, which was similar to the sample after being washed with distilled water. However, different from the sample after being washed with distilled water, the ratio of amine and pyrrolic nitrogen groups after Cd(II) adsorption showed no notable change compared with raw non-living Nitzschia. These results implied that Cd(II) ions interacted with both amine and pyrrolic nitrogen during adsorption, which inhibited the protonation of these two nitrogen bonds. Moreover, the binding energy of amine nitrogen and pyrrolic nitrogen increased from 399.5 and 400.0 to 399.7 and 400.2, respectively, after Cd(II) adsorption (Table 4), which further confirmed the coordination of amine nitrogen and pyrrolic nitrogen with cadmium ions. The increased BE value of the nitrogen atom was because the nitrogen atom donates electrons to cadmium ions, and thus, the electron density around the nitrogen atom decreases (Yang et al., 2011). The greater affinities of amine and pyrrolic nitrogen to Cd ions than pyridinic nitrogen might be explained by the hard and soft acids and bases theory (HSAB) that the soft acid (Cd2+) interacts preferentially with the soft base (pyrrolic nitrogen and amine) than the hard base (pyridinic nitrogen) and the soft acid interacts with the soft base, usually via coordination. Similarly, Ji et al. reported a higher affinity of pyrrolic nitrogen to Pb2+ than pyridinic nitrogen (Ji et al., 2018).
Conclusion
Non-living marine diatom biomass (Chaetoceros, Nitzschia, and Thalassiosira sp.) could efficiently remove Cd(II) from aqueous solution in the absence of ionic competition and under low turbidity conditions, with non-living Nitzschia possessing the highest adsorption capacity. The adsorption process was a spontaneous, endothermic, and chemisorption-involved process. The adsorption of Cd(Ⅱ) was inhibited by inorganic salts and turbidity but was promoted by humic acid. The adsorption mechanism analysis demonstrated that amine and pyrrolic nitrogen showed good affinities to Cd(Ⅱ), whereas pyridinic nitrogen did not. The results of the present study indicated that diatom biomass was a great alternative for Cd(II) sequestration from an aqueous solution. Further studies, such as immobilization and recycling, should be conducted to promote its application in real heavy-metal wastewater treatment.
Data availability statement
The original contributions presented in the study are included in the article/Supplementary Material; further inquiries can be directed to the corresponding author.
Author contributions
XW contributed to the conception and design of the study, conducted part of experiments, analyzed the data, and wrote the manuscript. JW and SZ conducted parts of the experiments. All authors contributed to manuscript revision, read, and approved the submitted version.
Funding
This work was supported by the Key R&D Program of Shandong Province, China (2022SFGC0304), Guangdong Innovative and Entrepreneurial Research Team Program (no. 2016ZT06N532) with Shenzhen Government Related Supporting Fund (no. KYTDPT20181011104002), and the SUSTech-RIGEL Joint Ecological Laboratory (Y01422304).
Conflict of interest
The authors declare that the research was conducted in the absence of any commercial or financial relationships that could be construed as a potential conflict of interest.
Publisher’s note
All claims expressed in this article are solely those of the authors and do not necessarily represent those of their affiliated organizations, or those of the publisher, the editors, and the reviewers. Any product that may be evaluated in this article, or claim that may be made by its manufacturer, is not guaranteed or endorsed by the publisher.
References
Alizadeh, B., Delnavaz, M., and Shakeri, A. (2018). Removal of Cd(ӀӀ) and phenol using novel cross-linked magnetic EDTA/chitosan/TiO2 nanocomposite. Carbohyd. Polym. 181, 675–683. doi:10.1016/j.carbpol.2017.11.095
Armbrust, E. V. (2009). The life of diatoms in the world’s oceans. Nature 459 (7244), 185–192. doi:10.1038/nature08057
Beamson, G., and Briggs, D. (1992). High resolution XPS of organic polymers - the scienta ESCA 300 database. Chichester, UK: John Wiley & Sons.
Bilal, M., Shah, J. A., Ashfaq, T., Gardazi, S. M. H., Tahir, A. A., Pervez, A., et al. (2013). Waste biomass adsorbents for copper removal from industrial wastewater—a review. J. Hazard Mat. 263, 322–333. doi:10.1016/j.jhazmat.2013.07.071
Burakov, A. E., Galunin, E. V., Burakova, I. V., Kucherova, A. E., Agarwal, S., Tkachev, A. G., et al. (2018). Adsorption of heavy metals on conventional and nanostructured materials for wastewater treatment purposes: A review. Ecotox. Environ. Safe. 148, 702–712. doi:10.1016/j.ecoenv.2017.11.034
Chen, H., Luo, J., Wang, X., Liang, X., Zhao, Y., Yang, C., et al. (2018). Synthesis of Al2O3/carbon composites from wastewater as superior adsorbents for Pb(II) and Cd(II) removal. Micropor. Mesopor. Mat. 255, 69–75. doi:10.1016/j.micromeso.2017.07.023
Chen, J. P., and Yang, L. (2005). Chemical modification of Sargassum sp. for prevention of organic leaching and enhancement of uptake during metal biosorption. Ind. Eng. Chem. Res. 44, 9931–9942. doi:10.1021/ie050678t
Chen, J. P., and Yang, L. (2006). Study of a heavy metal biosorption onto raw and chemically modified Sargassum sp. via spectroscopic and modeling analysis. Langmuir 22 (21), 8906–8914. doi:10.1021/la060770+
Cheng, C., Wang, J., Yang, X., and Philippe, C. (2014). Adsorption of Ni(II) and Cd(II) from water by novel chelating sponge and the effect of alkali-earth metal ions on the adsorption. J. Hazard Mat. 264 (2), 332–341. doi:10.1016/j.jhazmat.2013.11.028
Dai, Y. J., Sun, Q. Y., Wang, W. S., Lu, L., Liu, M., Li, J., et al. (2018). Utilizations of agricultural waste as adsorbent for the removal of contaminants: A review. Chemosphere 211, 235–253. doi:10.1016/j.chemosphere.2018.06.179
Davis, T. A., Volesky, B., and Mucci, A. (2003). A review of the biochemistry of heavy metal biosorption by Brown algae. Water Res. 37 (18), 4311–4330. doi:10.1016/s0043-1354(03)00293-8
Deng, J., Liu, Y., Liu, S., Zeng, G., Tan, X., Huang, B., et al. (2017). Competitive adsorption of Pb(II), Cd(II) and Cu(II) onto chitosan-pyromellitic dianhydride modified biochar. J. Colloid Interf. Sci. 506, 355–364. doi:10.1016/j.jcis.2017.07.069
Dinesh, M., and Singh, K. P. (2002). Single and multi-component adsorption of cadmium and zinc using activated carbon derived from bagasse-an agricultural waste. Water Res. 36, 2304–2318. doi:10.1016/s0043-1354(01)00447-x
Ding, T. D., Ni, W. M., and Zhang, J. Y. (2012). Research advances in heavy metals pollution ecology of diatom. Chin. J. Appl. Ecol. 23 (3), 857–866. doi:10.13287/j.1001-9332.2012.0117
Dubey, S., Banerjee, S., Upadhyay, S. N., and Sharma, Y. C. (2017). Application of common nano-materials for removal of selected metallic species from water and wastewaters: A critical review. J.Mol. Liq. 240, 656–677. doi:10.1016/j.molliq.2017.05.107
Gao, Z. Y., Shan, D. X., He, J. H., Huang, T., Mao, Y., Tan, H., et al. (2023). Effects and mechanism on cadmium adsorption removal by CaCl2-modified biochar from selenium-rich straw. Bioresour. Technol. 370, 128563. doi:10.1016/j.biortech.2022.128563
Ge, H., and Wang, J. (2017). Ear-like poly (acrylic acid)-activated carbon nanocomposite: A highly efficient adsorbent for removal of Cd(II) from aqueous solutions. Chemosphere 169, 443–449. doi:10.1016/j.chemosphere.2016.11.069
González, F., Romera, E., Ballester, A., Blazquez, M. L., Munoz, J. A., and Garcia-Balboa, C. (2011). “Algal biosorption and biosorbents[M],” in Microbial biosorption of metals (Springer Netherlands), 159–178.
González, P. G., and Pliego-Cuervo, Y. B. (2014). Adsorption of Cd(II), Hg(II) and Zn(II) from aqueous solution using mesoporous activated carbon produced from Bambusa vulgaris striata. Chem. Eng. Res. Des. 92 (11), 2715–2724. doi:10.1016/j.cherd.2014.02.013
Guillard, R. R. L. (1975). “Culture of phytoplankton for feeding marine invertebrates,” in Culture of marine invertebrate animals. Editors W. L. Smith, and M. H. Chanley (New York, USA: Plenum Press), 26–60.
Guo, J., Annett, A. L., Taylor, R. L., Lapi, S., Ruth, T. J., and Maldonado, M. T. (2010). Copper-uptake kinetics of coastal and oceanic diatoms. J. Phycol. 46 (6), 1218–1228. doi:10.1111/j.1529-8817.2010.00911.x
He, J., and Chen, J. P. (2014). A comprehensive review on biosorption of heavy metals by algal biomass: Materials, performances, chemistry, and modeling simulation tools. Bioresour. Technol. 160 (6), 67–78. doi:10.1016/j.biortech.2014.01.068
Ibrahim, W. M. (2011). Biosorption of heavy metal ions from aqueous solution by red macroalgae. J. Hazard Mat. 192 (3), 1827–1835. doi:10.1016/j.jhazmat.2011.07.019
Ji, Q., Hu, C., Liu, H., and Qu, J. (2018). Development of nitrogen-doped carbon for selective metal ion capture. Chem. Eng. J. 350, 608–615. doi:10.1016/j.cej.2018.06.018
Kinniburgh, D. G., ‚van Riemsdijk, W. H., ‚Koopal, L. K., ‚et, al., Benedetti, M. F., and Avena, M. J. (1999). Ion binding to natural organic matter: competition‚heterogeneity‚stoichiometry and thermodynamic consistency. Colloid. Surf. A 151, 147–166. doi:10.1016/s0927-7757(98)00637-2
Kumar, K. S., Dahms, H. U., Eunji, W., Lee, J. S., and Shin, K. H. (2015). Microalgae - a promising tool for heavy metal remediation. Ecotoxicol. Environ. Saf. 113, 329–352. doi:10.1016/j.ecoenv.2014.12.019
Lan, Z. C., Lin, Y., and Yang, C. P. (2022). Lanthanum-iron incorporated chitosan beads for adsorption of phosphate and cadmium from aqueous solutions. Chem. Eng. J. 448, 137519. doi:10.1016/j.cej.2022.137519
Li, Q., Chai, L., and Qin, W. (2012). Cadmium(II) adsorption on esterified spent grain: Equilibrium modeling and possible mechanisms. Chem. Eng. J. 197 (29), 173–180. doi:10.1016/j.cej.2012.04.102
Li, M., Zhang, Z., Li, R., Wang, J. J., and Ali, A. (2016). Removal of Pb(II) and Cd(II) ions from aqueous solution by thiosemicarbazide modified chitosan. Int. J. Biol. Macromol. 86, 876–884. doi:10.1016/j.ijbiomac.2016.02.027
Li, C., Zhang, M., Zhong, H., He, H., Feng, Y., and Yin, X. (2018). Synthesis of a bioadsorbent from jute cellulose, and application for aqueous Cd(II) removal. Carbohyd. Polym. 189, 152–161. doi:10.1016/j.carbpol.2018.01.094
Ma, J., Zhou, B., Duan, D., Wei, Y., and Pan, K. (2018). Silicon limitation reduced the adsorption of cadmium in marine diatoms. Aquat. Toxicol. 202 (1), 136–144. doi:10.1016/j.aquatox.2018.07.011
Maity, J., and Ray, S. K. (2018). Chitosan based nanocomposite adsorbent-Synthesis, characterization and application for adsorption of binary mixtures of Pb(II) and Cd(II) from water. Carbohyd. Polym. 182, 159–171. doi:10.1016/j.carbpol.2017.10.086
Morin, S., Duong, T. O., Feurtet-Mazel, A., and Coste, M. (2008). Cadmium toxicity and bioaccumulation in freshwater biofilms. Arch. Environ. Con. Tox. 54 (2), 173–186. doi:10.1007/s00244-007-9022-4
Naderi, A., Delavar, M. A., Ghorbani, Y., Kaboudin, B., and Hosseini, M. (2018). Modification of nano-clays with ionic liquids for the removal of Cd(II) ion from aqueous phase. Appl. Clay Sci. 158, 236–245. doi:10.1016/j.clay.2018.03.037
Niwas, R., Gupta, U., Khan, A. A., and Varshney, K. (2000). The adsorption of phosphamidon on the surface of styrene supported zirconium (IV) tungstophosphate: A thermodynamic study. Colloid. Surf. A 164, 115–119. doi:10.1016/s0927-7757(99)00247-2
Ozer, A., and Pirinççi, H. B. (2006). The adsorption of Cd(II) ions on sulphuric acid-treated wheat bran. J. Hazard Mat. 137 (2), 849–855. doi:10.1016/j.jhazmat.2006.03.009
Peng, W., Li, H., Liu, Y., and Song, S. (2017). A review on heavy metal ions adsorption from water by graphene oxide and its composites. J. Mol. Liq. 230, 496–504. doi:10.1016/j.molliq.2017.01.064
Robert, M. S., Francis, X. W., and David, J. K. (2007). Spectrometric identification of organic compounds. Shanghai: East china university of science and technology press.
Shang, Y., Yu, X., and Romero-González, M. E. (2015). Screening of algae material as a filter for heavy metals in drinking water. Algal Res. 12, 258–261. doi:10.1016/j.algal.2015.09.003
Sheng, P. X., Ting, Y. P., Chen, J. P., and Hong, L. (2004). Sorption of lead, copper, cadmium, zinc, and nickel by marine algal biomass: Characterization of biosorptive capacity and investigation of mechanisms. J. Colloid Interf. Sci. 275 (1), 131–141. doi:10.1016/j.jcis.2004.01.036
Sun, W., Jiang, B., Wang, F., and Xu, N. (2015). Effect of carbon nanotubes on Cd(II) adsorption by sediments. Chem. Eng. J. 264, 645–653. doi:10.1016/j.cej.2014.11.137
Suresh, K. K., Dahms, H., Won, E., Lee, J. S., and Shin, K. H. (2015). Microalgae - a promising tool for heavy metal remediation. Ecotox. Environ. Safe. 113, 329–352. doi:10.1016/j.ecoenv.2014.12.019
Uddin, M. K. (2017). A review on the adsorption of heavy metals by clay minerals, with special focus on the past decade. Chem. Eng. J. 308, 438–462. doi:10.1016/j.cej.2016.09.029
Wang, B. Z., Zhang, D., Shi, Z. K., and Wu, T. (2012). Thermodynamics analysis of cesium adsorption on Na2Ti2O3SiO4•2H2O. J. Isot. 25 (1), 42–46. doi:10.7538/tws.2012.25.01.0042
Wang, Q., Liu, Q., Wang, Z. C., Liu, H. P., Bai, J. R., and Ye, J. B. (2017). Characterization of organic nitrogen and sulfur in the oil shale kerogens. Fuel Process. Technol. 160, 170–177. doi:10.1016/j.fuproc.2017.02.031
Wang, S., Xin, L., Liu, Y., Zhang, C., Tan, X., Zeng, G., et al. (2018). Nitrogen-containing amino compounds functionalized graphene oxide: Synthesis, characterization and application for the removal of pollutants from wastewater: A review. J. Hazard Mat. 342, 177–191. doi:10.1016/j.jhazmat.2017.06.071
Xie, G., Shang, X., Liu, R., Hu, J., and Liao, S. (2011). Synthesis and characterization of a novel amino modified starch and its adsorption properties for Cd(II) ions from aqueous solution. Carbohyd. Polym. 84 (1), 430–438. doi:10.1016/j.carbpol.2010.12.003
Yang, F., Liu, H., Qu, J., and Paul Chen, J. (2011). Preparation and characterization of chitosan encapsulated Sargassum sp. biosorbent for nickel ions sorption. Bioresour. Technol. 102 (3), 2821–2828. doi:10.1016/j.biortech.2010.10.038
Yazdi, M. N., Dadfarnia, S., and Shabani, A. M. H. (2021). Synthesis of stable S-functionalized metal-organic framework using MoS42- and its application for selective and efficient removal of toxic heavy metal ions in wastewater treatment. J. Environ. Chem. Eng. 9, 104696. doi:10.1016/j.jece.2020.104696
Yu, W., Lian, F., Cui, G., and Liu, Z. (2018). N-doping effectively enhances the adsorption capacity of biochar for heavy metal ions from aqueous solution. Chemosphere 193, 8–16. doi:10.1016/j.chemosphere.2017.10.134
Zeng, J. P. (2008). Study of adsorption and desorption behavior of Cd on bentonite. Doctoral dissertation. Chengdu: Cheng Du University of Technology.
Zeng, Z. L., Yu, C., Liao, R. P., Cai, X., Chen, Z. h., Yu, Z., et al. (2023). Preparation and characterization of sodium polyacrylate grafted montmorillonite nanocomposite for the adsorption of cadmium ions form aqueous solution. Colloid Surf. A 656, 130389. doi:10.1016/j.colsurfa.2022.130389
Zeraatkar, A. K., Ahmadzadeh, H., Talebi, A. F., Moheimani, N. R., and McHenry, M. P. (2016). Potential use of algae for heavy metal bioremediation, a critical review. J. Environ. Manage. 181, 817–831. doi:10.1016/j.jenvman.2016.06.059
Zheng, Y. M., Liu, T., Jiang, J., Yang, L., Fan, Y., Wee, A. T., et al. (2011). Characterization of hexavalent chromium interaction with Sargassum by X-ray absorption fine structure spectroscopy, X-ray photoelectron spectroscopy, and quantum chemistry calculation. J. Colloid Interf. Sci. 356 (2), 741–748. doi:10.1016/j.jcis.2010.12.070
Zhou, X., Zhou, J., Liu, Y., Guo, J., Ren, J., and Zhou, F. (2018). Preparation of iminodiacetic acid-modified magnetic biochar by carbonization, magnetization and functional modification for Cd(II) removal in water. Fuel 233, 469–479. doi:10.1016/j.fuel.2018.06.075
Keywords: heavy metal, cadmium ions, adsorption, microalgae, marine diatom
Citation: Wang X, Wang J, Zhang S and Li J (2023) Highly effective sequestration of Cd(Ⅱ) from aqueous solution using marine diatom biomass: Adsorption performances and mechanism. Front. Environ. Sci. 11:1085277. doi: 10.3389/fenvs.2023.1085277
Received: 31 October 2022; Accepted: 06 February 2023;
Published: 22 February 2023.
Edited by:
Yingxin Zhao, Tianjin University, ChinaReviewed by:
Cláudia Batista Lopes, University of Aveiro, PortugalRongjun Bian, Nanjing Agricultural University, China
Copyright © 2023 Wang, Wang, Zhang and Li. This is an open-access article distributed under the terms of the Creative Commons Attribution License (CC BY). The use, distribution or reproduction in other forums is permitted, provided the original author(s) and the copyright owner(s) are credited and that the original publication in this journal is cited, in accordance with accepted academic practice. No use, distribution or reproduction is permitted which does not comply with these terms.
*Correspondence: Xin Wang, d2FuZ3gzQHN1c3RlY2guZWR1LmNu