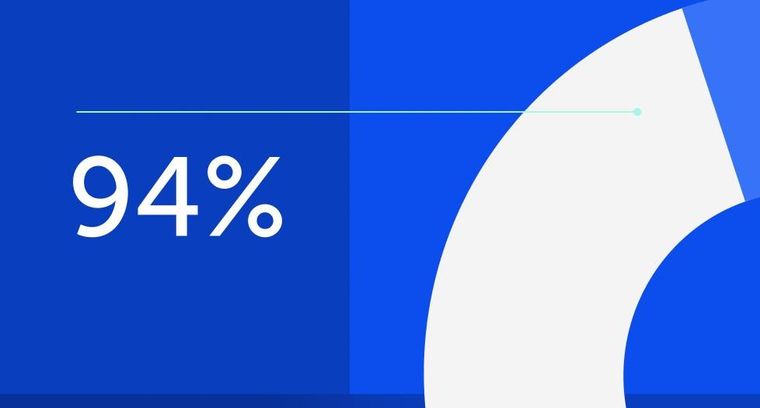
94% of researchers rate our articles as excellent or good
Learn more about the work of our research integrity team to safeguard the quality of each article we publish.
Find out more
ORIGINAL RESEARCH article
Front. Environ. Sci., 27 February 2023
Sec. Interdisciplinary Climate Studies
Volume 11 - 2023 | https://doi.org/10.3389/fenvs.2023.1082930
This article is part of the Research TopicFood-Energy-Water Systems: Achieving Climate Resilience and Sustainable Development in the 21st CenturyView all 21 articles
Incorporating the interdependencies between water, energy and food (WEF) within an integrated approach of planning and management could help nations worldwide to address sustainability concerns. This is a topic of great importance for the Middle East and North Africa (MENA) region, where water is a very limited resource. In this study, we develop an analytical framework to analyze the water-energy-food nexus in the MENA region to inform the formulation of integrated strategies for water, energy and food activities. Our approach is based on an integrated assessment model for the MENA region, which explicitly represents WEF sectors within an economic framework, in tandem with a set of relevant scenarios addressing three key dimensions (socioeconomics, climate and water-management). Using this framework, our study analyzes the current and projected status of water resources in the region, and the potential implications for the agriculture and electricity sectors. Our scenarios demonstrate that water scarcity worsens by the end of the 21st century in most MENA countries, mostly due to growing demands. The impacts of growing scarcity on agriculture are significant, with production projected to drop by 60 percent by 2050 in some countries. On the other hand, and to a lesser extent, water-saving technologies and fuel-switching in the power sector play a key role in mitigating the effects of water scarcity on electricity generation in some parts of the MENA region. Our analysis then underscores the need to reduce the dependence of MENA’s agricultural and energy sectors on water, and transition to renewable energies to reduce water scarcity.
The interdependency between water, energy and food (WEF) is growing in importance as demand for each of these vital resources increases. Several regions of the world are already experiencing WEF security challenges [e.g., South Africa (du Plessis 2017); Australia (Radcliffe, 2018); United States and China (Zhuang et al., 2021)], which adversely affect sustainable economic growth (Bazilian et al., 2011; Khan et al., 2021). In addition, there is already evidence of the effects of climate change on the availability and demand for water, energy and food. At the same time, scarcity in either water, energy or food is caused not only by physical factors, but there are also social, political and economic issues such as demographic growth, lack of institutions, poor governance, among others that affect the allocation, availability, and use of these resources (D’Odorico et al., 2018).
In the Middle East and North Africa (MENA) region, this nexus between water, energy and food is particularly important for the region’s sustainability and continued growth. Countries in this region are arid to semiarid, with many areas already facing water stress and a highly variable precipitation rate due to their geographic and climatic conditions. Although to date governments in the region have been at large successful in satisfying the needs of the population through ambitious dam building and groundwater provision systems, reduction in leakages, conservation, desalination, reuse and water transfers, per capita supply is declining due to growing population, increased urbanization, extended irrigated agriculture and highly water intensive crops together with the development of the industrial and the tourism sectors (Verner, 2012). This decline in per capita supply has increasingly pushed some countries in the region to think of ambitious desalination plans to supply water to coastal cities and agricultural areas, and to explore the possibility of large transfers of water from less arid parts of the region. Some examples of such projects are: the Peace Water Pipeline and the Manavgat River Project which explored the feasibility to move water from Turkey to its southern neighbors; the Ras Al Khair Desalination Plant is able to move water from the Persian Gulf to Riyadh City; and the Great Man-Made River is a network of pipes that supplies water to the Sahara in Libya, from the Nubian Sandstone Aquifer System. These options (desalination, reuse and water transfers) require relatively larger amounts of energy, mainly electricity, and significant capital investment (Caldera et al., 2018; Parkinson et al., 2019).
Despite the deficiencies in the observational records, historical climate data on rainfall amounts in the MENA region show a negative trend at national and regional scales (Hijioka et al., 2014; Shaw et al., 2022; Trisos et al., 2022). Average annual runoff and water availability are expected to decrease in the future due to climate change impacts, while temperatures, heat extremes and evapotranspiration rates are expected to increase (Ozturk et al., 2015; Lelieveld et al., 2016; Waha et al., 2017; Ajjur and Al-Ghamdi 2021; Paltán et al., 2021). High evapotranspiration and soil infiltration rates in the region reduce soil moisture and consequently increase irrigation requirements that typically surpass 80 percent of total water withdrawals in most countries (Verner, 2012). The agriculture sector in countries of the MENA region consumes a large share of water. Moving forward, water allocation to agriculture will likely face increased competition from high value uses in the industrial and urban sectors. The competition will differ across regions given that the economic returns of water used for irrigation for different crops differ significantly among Arab countries (Supplementary Figure S1). Managing water in the region will benefit from including agriculture within a nexus strategy that involves all sectors. This approach will be particularly important given that the agriculture sector is the largest employer in many countries of the region and contributes significantly, yet decreasingly, to meeting food requirements (Verner, 2012). For instance, countries can optimize their return on water by choosing different crop mixes, which will lead to different returns on the agricultural water used. Also worth considering is the fact that the cost of producing crops will continue to rise in significant parts of the region, as fossil (non-renewable) groundwater resources are depleted, and groundwater levels sink. Currently, wells require deep drilling, and the cost per cubic meter is increasing.
On the energy side, the region heavily relies on fossil fuels to generate electricity and, in some countries, to generate non-conventional water supplies (Siddiqi and Anadon, 2011; Huttner, 2013; Khatib, 2014). The dependency on fossil fuels is frequently complemented with energy imports, implying that the energy sector could face serious challenges in the near future (Al-Badi and AlMubarak, 2019). To address this issue, some countries in the region have launched renewable energy development programs to diversify their energy sources and achieve energy security objectives and environmental sustainability. Also, several countries have announced their ambition to achieve carbon neutrality by mid-century calling for rapid energy transition including carbon removal technologies (Climate Action Tracker, 2022). While the large-scale implementation of technologies such as nuclear, concentrated solar power, and fossil-based with carbon removal technologies like carbon capture and storage can have a positive impact on greenhouse gas emissions and on energy security, they could negatively impact water resources availability if such resources are not considered in the planning stages (Liu et al., 2015). Therefore, since water is needed for electricity generation, energy policy choices that focus on more water-efficient electricity generation technologies (e.g., solar photovoltaic and wind power) can have a positive impact on the water resources of a country and impact the development of the region. Managing the WEF nexus in the region while satisfying the future water needs of all sectors has become a strategic challenge for the MENA region (Hoff et al., 2019). In some parts of the region, the combined effects of population growth, increasing hydrological variability and climate change may result in increased reliance on energy-intensive water supply options. At the same time, agriculture is expected to continue to pose major pressures on the region’s diminishing water supplies. The WEF nexus poses not only challenges for sustainability in the MENA region, but also for the region’s food, energy and water security, and improving its social, economic and political stability.
The objective of this study is to develop and illustrate an analytical framework—based on the Global Change Analysis Model (GCAM; Wise et al., 2009; Calvin et al., 2019)—that can be used to help assess long-term integrated (nexus) scenarios for water, energy and food activities in the MENA region. Within this analytical framework, the remainder of the manuscript addresses three main research questions: 1) What are the physical impacts of climate change on water scarcity in the MENA region? 2) What are the impacts of socioeconomic pressures on water scarcity in the MENA region? and 3) What are impacts of both in tandem on water resources and consequently on agricultural and energy productions (i.e., the water-energy-food nexus) in the MENA region?
We first employ multiple general circulation models (GCMs) in a “reference climate change” scenario to assess the level of uncertainty propagating from climate models on water scarcity through the MENA region. Three climate models that roughly span the range of uncertainty (“wet,” “dry,” and “normal”) are selected. This allows a comparison between the uncertainties surrounding climate models and their impacts. Then we provide an assessment of different socioeconomic development pathways on water security throughout the MENA region. To do this, we use the socioeconomic development scenarios from the Shared Socioeconomic Pathways (SSPs; O’Neill et al., 2015). This scenario analysis shows how socioeconomic development trajectories might affect water demands and consequently water scarcity in different countries of the region. This helps to assess the relative effects of global socioeconomics and technological trends as compared to the effects of climate change. To address the third question, we explore the implications for the water-energy-food nexus of limited water supplies in the context of varying climatological conditions and varying socioeconomic conditions. The analysis quantifies water supply and demand changes throughout the region, shedding light on infrastructure needs, costs of policies and associated investment needs. The adopted modeling approach also allows for different technologies to compete endogenously (e.g., cooling technologies in the power sector), thus, implicitly accounting for the value of technologies in meeting future water, energy, and food demands in the MENA region.
The flowchart diagram shown in Figure 1 provides a schematic of the overall methodology to address the three specific research questions (Analysis), the associated scenarios (Scenarios), the underlying core modeling capability (Model), and metrics used to address the questions (Metrics). In this section, we first introduce the core modeling capability, followed by the analytical methods to establish the key metrics, and then by the various scenarios employed in this study.
Integrated Assessment Models (IAMs) provide a general modeling framework for exploring the relationships between water, climate, land and energy (nexus) through an interwoven understanding of the physical, economic and institutional constraints of water resources issues and consideration of climate-related impacts on management and decision-making process in water supply, energy generation and food production.
The Global Change Analysis Model (GCAM) is an IAM for exploring consequences and responses to global change. Climate change is a global issue that impacts all regions of the world and all sectors of the global economy. Thus, any responses to the threat of climate change, such as policies or international agreements to limit greenhouse gas emissions, can have wide ranging consequences throughout the energy system as well as on water resources, food production, land use and land cover. IAMs endeavor to represent all world regions and all sectors of the economy in an economic framework in order to explore interactions between sectors and understand the potential ramifications of climate change mitigation actions.
GCAM has been built based on global and detailed datasets for over 30 years and is extensively used to explore climate change mitigation and adaptation policies (Clarke, 2014; Kyle et al., 2014; Turner et al., 2019a; Feijoo et al., 2020). A key advantage of GCAM over some other IAMs is that it is a Representative Concentration Pathway (RCP)-class model. This means it can be used to simulate scenarios, policies, and emission targets from various sources including the Intergovernmental Panel on Climate Change (IPCC).
GCAM solves for partial market equilibrium of water, energy and food at discrete time steps. It represents the economy, energy sector, land use and water resources linked to climate models. In this framework, GCAM represents the global economy by disaggregating the world into a number of geopolitical energy-economy regions (32 in the standard version). Along the socioeconomics system, population and labor productivity growth assumptions set the scale of regional economic activity, which in turn drives demands across all sectors. GCAM solves each period sequentially by iteratively searching for the equilibrium prices that ensure that supplies and demands are equal in all existing markets (energy, agriculture, land, GHG emissions). GCAM can be used to explore the effects of climate adaptation and mitigation policies, including carbon taxes, carbon trading, regulations, deployment of energy technologies and spatial representations of food production, particularly agriculture. In the energy system, the model employs numerous technology options to produce, transform, and provide energy services as described in its online documentation (JGCRI, 2022). The supplies of agricultural and forest products are determined in GCAM’s agriculture and land use model (Calvin et al., 2019). For agriculture and land-use, each geopolitical region in the model can be disaggregated into up to 18 agro-ecological zones (AEZs) such that within each of these subregions land is categorized into twelve types based on cover and use (e.g., forestlands, shrublands, grasslands, croplands, etc.). Land allocation decisions within any geopolitical region depends on the relative profitability of all possible land uses within each land-use region (Kyle et al., 2014). Land used for any purpose competes economically with croplands, commercial forests, pastures, and all lands not involved in commodity production, except for tundra, deserts, and urban lands (assumed constant over time). The profitability of any land used for commercial production is derived from the price (value) of the commodity produced, the costs of production, and the yields (Kyle et al., 2014). GCAM models the production of twelve crop categories based on exogenously specified yields that are crop-specific and the amount of land allocated to that particular use.
Using a run period extending from 1990—2,100 at 5-year intervals, GCAM has been used to explore the potential role of emerging energy supply technologies and the greenhouse gas consequences of specific policy measures or energy technology adoption. Outputs of GCAM include projections of future energy supply and demand and the resulting greenhouse gas emissions, radiative forcing and climate effects of 16 greenhouse gases, aerosols and short-lived species, contingent on assumptions about future population, economy, technology, and climate mitigation policy. On the water side, GCAM represents demands in six major water use sectors—irrigation, municipal uses, primary resource extraction (energy/mining), livestock production, electricity generation and manufacturing. Furthermore, GCAM water supplies consider three main sources—renewable water, non-renewable groundwater, and desalinated water. Supplementary Notes S1–S4 provide details about the water sector representation in GCAM (demands, supplies and water allocation across users).
GCAM is a publicly available, open-source modeling tool, developed and maintained by the Pacific Northwest National Laboratory, part of the US Department of Energy. Further details about GCAM can also be found on its online documentation (JGCRI, 2022).
A primary portion of this work was the development of a version of GCAM with regional detail for the MENA region (hereinafter referred to as GCAM-MENA). GCAM-MENA was developed specifically to analyze water-energy-food nexus issues in the MENA region at the country level of spatial resolution. For this purpose, the 2 geopolitical regions in the existing GCAM model covering the MENA region (North Africa and Middle East) were further divided into 15 geopolitical regions (Figure 2). Thus, GCAM-MENA has a total of 45 geopolitical energy-economy regions (i.e., 15 capturing the new MENA regions, and 30 for the rest of the world).
FIGURE 2. Telescoping approach implemented in the GCAM-MENA model (i.e., the MENA region is broken into 15 regions as shown in bottom panel; top panel shows the default 32 regions in GCAM).
This effort of breaking out the MENA into 15 unique geopolitical regions in GCAM required a substantial data compilation and rearrangement effort to ensure the ability of the model to balance demands and supplies for all sectors over the calibration years at the country level. For example, several datasets used in GCAM were missing entries for some of the MENA countries (e.g., Bahrain). And many MENA countries had missing values in these input datasets especially over historical years. To overcome these issues, we had to aggregate some of these countries together and carefully estimate some of the missing energy and land entries to ensure that markets for all tracked commodities are solved in all historical time periods for all 15 MENA regions. Countries/regions that were aggregated to form single geopolitical regions in the model are: Saudi Arabia and Bahrain (henceforth: Arabian Peninsula), Israel and Palestine (henceforth: Israel-Palestinian Territory), and Morocco and Western Sahara (henceforth: Morocco-Western-Sahara).
Using a physics-based methodology to estimate groundwater resources and extraction costs detailed in Turner et al., 2019a, Turner et al., 2019b and Niazi et al., 2022, three groundwater resource curves for each of the 15 MENA regions were constructed (Supplementary Figures S2, S3). These resource curves follow the three groundwater availability scenarios in Turner et al., 2019a, so that the resource curves represent the amount of groundwater resources that can be economically exploited without exceeding the specified maximum threshold amounts of groundwater. These resource curves also account for environmental flow requirements, which are deducted from the total renewable water resources calculation. The environmental flow requirements account for in-stream water demands for uses such as ecosystem services, navigation, and recreation. In this study, this amount of water is estimated as 10% of the long-term mean monthly natural streamflow following the work of Voisin et al. (2013).
In the groundwater resource curves shown in Supplementary Figure S3, the cost is only reflecting the cost of electricity required to pump water from the ground plus groundwater well drilling and installation, pumping, and other maintenance costs. There are other costs (the cost of water treatment, and transport) that are not considered in the cost computation of the groundwater resource curves employed in this work.
A key analytical element of this research is the development of water scarcity measures. For this purpose, a water scarcity index (WSI) can be calculated at the country scale using Eq. 1.
The denominator of Eq. 1, i.e., the total water available in each MENA country, is calculated as the sum of runoff and inflow. Runoff is the available surface runoff and renewable groundwater resources, which were internally generated within the country and computed using the global hydrologic model described in Supplementary Note S2. The cross-boundary river inflows were also estimated and then bias-corrected to the inflow estimates from the FAO’s Aquastat database. A modified version of the WSI can also be calculated by including non-conventional water sources such as desalination in the denominator of Eq. 1.
The effects of climate change, mitigation activities, and adaptation strategies all have the potential to impact multiple aspects of the economy in both direct and indirect ways. Although some regions may experience positive impacts for some economic sectors, the distribution of impacts will vary across countries and the economic burden will not be evenly distributed. Adaptation may lessen negative impacts in some sectors, but in some cases, these strategies may have unintended consequences on other parts of the economy. Finally, economic impacts can have a long-term, cumulative nature, so that seemingly short-term events may have longer-lasting impacts on economic growth. For these reasons, the economic impacts of climate change can be most effectively analyzed with an integrated modeling approach. In this section, we describe how GCAM can be used to estimate the economic effects of climate change and adaptation strategies, using an example of a water-constrained agricultural sector and the use of different irrigation technologies to adapt.
There are multiple time scales on which physical and economic impacts and damages may occur. Agricultural production and revenues may be affected by severe weather over the course of one or several years, while in the longer term, changing climatic conditions may cause shifts in productive growing regions. The effects of these impacts will differ both in economic value, broader macroeconomic consequences, and effective adaptation options. Therefore, multiple methods of analysis are required including Integrated Assessment modeling to analyze intersectoral changes, finer resolution sectoral models (e.g., hydrological or agricultural), and post modeling economic valuation. Below we present a general methodology that can be used to conduct research on the broader economic costs and benefits of different adaptation methods, using irrigation technologies as an example. Analysis can focus on either (or both) the long-term effects of changing precipitation patterns and short-term, extreme drought events.
Climate change will affect the agricultural sector directly, through changing temperature and precipitation patterns and more frequent extreme weather events. These changes may result in improved yields in some regions and lower yields in others (Rosenzweig et al., 2014). In our example, we focus on water-constrained agricultural production, where yields are lower due to insufficient soil moisture; a shift in the Supply curve from S0 to S1. In this scenario, total yields decrease and prices increase as illustrated in Figure 3A. P and Q are the price per unit and the total production of a specific agricultural commodity, respectively. S is supply, and D is demand. The subscript “0” reflects an initial state whereas subscript “1” means a change to a water-constrained state.
FIGURE 3. Framework to estimate the economic impacts on agricultural sector (A) food demand-price relationship and the effects of decreasing yields, (B) the effect of price increase on food consumption.
Due to the inelastic nature of food demand, price increases may more than offset production losses for producers, because
The total economic effect of these changes is more complicated and can be ambiguous when multiple aspects of economic welfare are considered. Even when agricultural producers and net exports increase, all groups will face higher food prices. Figure 3B shows the effect on consumption when the price of good 1 (X1) increases leading to shift in the demand curve to the left. Within this context, total consumption typically decreases. However, diverse consumption patterns and relative yield impacts may result in very different patterns of impacts across countries. Because agricultural products are generally globally traded goods, modeling the impacts of climate change on a specific region requires a global assessment.
Through this approach, GCAM can be used to estimate the impacts of water scarcity on the net value of agricultural commodities (recall that GCAM tracks twelve crop commodity classes and GCAM’s approach to estimate agricultural production is provided in Section 2.1):
Impacts are only one part of the story. When farmers face long-term changes in weather patterns, they will change their behavior to adapt to the new circumstances. Adaptation to water scarcity may occur through multiple channels, such as planting different crops or more drought-resistant varieties and increasing use of irrigation. The adaptive responses will tend to reduce the negative impacts of water scarcity, but they also have the potential to affect the wider economy through interactions with energy, manufacturing, or other economic sectors. The net impact on an economy of any given adaptation response cannot be known without modeling the global system.
Understanding the potential impacts forms a basis on which to model the costs and benefits of various adaptation strategies. For example, increased investment in irrigation may help to reduce the negative impact on yields, but will also increase production costs, which will depend greatly on the supply of water and demand in other sectors (e.g., water for cooling thermoelectric power plants). The costs and benefits of the same irrigation technology varies among countries.
In a scenario where water is a constraining factor, the relative costs and benefits in terms of production, prices, and net trade flows can be modeled in GCAM. For instance, the capital investment and operating costs of sprinkle, drip, flood, and micro-irrigation can be used to estimate production costs under these technologies and their impact on macroeconomic metrics. Analysis can also be conducted on the costs of different water supply options, such as using non-renewable groundwater or desalinization plants (Hussain & Bhattarai, 2004). As a starting point for this analysis, we assume a given unit cost of (e.g., $1.0/m3) for desalination plants with a lifetime of 30 years following the work of Parkinson et al. (2016). The work also relies on the previous work of Immerzeel et al. (2011) and Droogers et al. (2012).
This analysis is built on exploration of scenarios. As discussed earlier, the scenarios in this study cover three key dimensions: 1) climate impacts, 2) socioeconomics pathways, and 3) limitations on water supplies. These three areas are discussed in the remainder of this section.
Representative Concentration Pathways (RCPs) are four greenhouse gas concentration (not emissions) trajectories adopted by the IPCC for its fifth Assessment Report (AR5) in 2014 (Moss et al., 2008 These pathways are used for climate modeling and research. They describe four possible climate futures, all of which are considered possible depending on how much greenhouse gases are emitted in the years to come. The four RCPs: RCP2.6, RCP4.5, RCP6.0, and RCP8.5, are named after a possible range of radiative forcing values in the year 2,100 relative to pre-industrial values (increases of +2.6, +4.5, +6.0, and +8.5 W/m2, respectively) (Weyant et al., 2009).
The RCPs are consistent with a wide range of possible changes in future anthropogenic GHG emissions RCP2.6 assumes that global annual GHG emissions (measured in CO2-equivalents) peak between 2010–2020, with emissions declining substantially thereafter Emissions in RCP4.5 peak around 2040, then decline In RCP6.0, emissions peak around 2080, then decline In RCP8.5, emissions continue to rise throughout the 21st century.
For the purposes of this study, a “no climate policy” reference scenario (RCP6.0) has been implemented in GCAM to reflect “reference” or baseline efforts towards climate mitigation. Moreover, three different GCMs were selected to represent relatively wet, average and dry conditions in the region in an effort to provide a robust envelope of impacts of climate change on water resources (See Supplementary Figure S4). The three selected GCMs for this analysis are: The Community Climate System Model (CCSM) (Gent et al., 2011), the Goddard Institute for Space Studies (GISS) (Schmidt et al., 2014), and the First Institute of Oceanography Earth System Model (FIO-ESM; Qiao et al., 2013; Bao et al., 2020).
Long-term scenarios play an important role in research on global environmental change. The climate change research community has developed a set of scenarios integrating future changes in climate and society to investigate climate impacts as well as options for mitigation and adaptation. One component of these scenarios is a set of alternative futures of societal development known as the Shared Socioeconomic Pathways (SSPs). The conceptual framework for the design and use of the SSPs calls for the development of global pathways describing the future evolution of key aspects of society, which would together imply a range of challenges for mitigating and adapting to climate change.
O’Neill et al. (2015) defines the “SSP narratives” as a set of five qualitative descriptions of future changes in demographics, human development, economy and lifestyle, policies and institutions, technology, and environment and natural resources. The narratives are intended to describe plausible future conditions at the level of large world regions that can serve as a basis for integrated emissions and land use scenarios, as well as climate impact, adaptation and vulnerability analyses.
Within the conceptual framework for integrated scenarios, the SSPs are designed to span a relevant range of uncertainty in societal futures, describing worlds in which societal trends result in making mitigation of, or adaptation to, climate change harder or easier, without explicitly considering climate change itself. Supplementary Table S1 summarizes the SSP implementation in GCAM. Note, water technology storylines and assumptions are not part of the SSP scenarios; that is water is considered indirectly in the SSPs through agricultural and energy water use and water technology assumptions are not varied across the five SSPs.
Here we provide the main characteristics of the five SSPs based on information compiled from O’Neill et al. (2015); O’Neill et al. (2016). The SSP1 represents a world with more optimistic trends for human development with the lowest demographic pressure across the SSPs, substantial income growth, reduction in inequality, and increasing focus on sustainable and environmental practices with a gradual move toward less resource-intensive lifestyles. The SSP5 is also relatively optimistic in terms of human development trends with substantial investments in education and health, well-functioning institutions and very high economic growth, which enables many development goals to be achieved within short time frames. However, the push for economic and social development is coupled with the exploitation of abundant fossil fuel resources and the adoption of resource- and energy-intensive lifestyles around the world. In the SSP5 world, there is relatively little effort to avoid potential global environmental impacts. SSPs 3 and 4 envision more pessimistic development trends, with little education or health investment, a fast-growing population, and increasing inequalities. In SSP3, countries prioritize regional security, economic development is slow, and consumption is material-intensive. A low international priority for addressing environmental concerns, slow technological change, growing resource intensity and fossil fuel dependency, and difficulty in achieving international cooperation lead to strong environmental degradation in some regions. In SSP4, large inequalities within and across countries dominate with substantial proportions of populations at low levels of development and weak institutions. Economic growth is moderate in industrialized and middle-income countries, and low in less developed countries, which face difficulties in providing adequate access to water and sanitation for the poor. Uncertainty in the fossil fuel markets leads to new investments in both carbon-intensive fuels like coal and unconventional oil and low-carbon energy sources. The SSP2 represents a central pathway in which trends continue their historical patterns without substantial deviations.
In this study, we pose two illustrative examples of water resources management scenarios to better understand the implications of different management approaches on water scarcity, energy and food in the MENA region. The purpose of this analysis is to provide a sample of the types of water management measures that can be employed and their implications throughout the region. Both of these scenarios incorporate RCP6.0 for climate and SSP2 for socioeconomic development.
This scenario assumes unlimited water resources where all economic sectors can achieve all their water demands without water constraints. In other words, this scenario assumes water resources that are physically unavailable, serving as a “counter-factual” benchmarking scenario to quantify the projected changes in the water, agricultural, and energy sectors under no water constraints.
This scenario focuses on constraining the water demands to the available water resources (renewable surface and groundwater, non-renewable groundwater resources, desalinated water) within each river basin. In this scenario, we employ the cost resources curves depicted in Supplementary Figure S3 to estimate the amount economically available groundwater resources in each MENA country. This scenario also incorporates adaptation measures to be deployed as a means to mitigate the water scarcity problem. More specifically, the expansion of desalination and more efficient irrigation technologies are included as adaptation measures. This is done to shed light on the level of necessary adaptation to close the water gap in the region and the associated investment costs that are associated with those measures. Also, by comparing this scenario to the UnlimitedWater scenario, we can estimate the economic impacts of water limitations on the region’s economy. For details on how GCAM estimates the irrigation demand and desalination water volumes in each region, the reader is referred to Chaturvedi et al., 2013; Hejazi et al., 2014a; Hejazi et al., 2014b, Kyle et al., 2021, and to the online documentation (JGCRI, 2022).
Results for runoff, water demand and WSI are shown in Tables 1, 2 at both the aggregate regional (i.e., North Africa and Middle East) and country scales. Maps highlighting the spatial distribution of these parameters across the MENA region are presented in Supplementary Figures S5–S7. These results illustrate three key trends for water scarcity in the MENA region.
TABLE 1. Total annual runoff based on 3 GCMs (top) and estimated total annual water demand under the UnlimitedWater scenario (bottom). Units: billion m3/year.
TABLE 2. Water scarcity index values at the country scalea using three GCMs.
First, the region overall has scarce renewable water resources availability; this result is consistent with numerous studies of water availability in the region (e.g., Verner, 2012; Hejazi et al., 2014b; Mekonnen and Hoekstra, 2016; Liu et al., 2022). These results appear to be robust based on the 3 GCMs used (see Table 1 for all GCM results and Supplementary Figure S5 for the GISS model). Some exceptions to this are found in the northern fringes of Morocco, Algeria, Tunisia, Libya, Egypt, Israel-Palestinian Territory, Jordan, Lebanon, Syria, Iraq and pockets in northwest Iran, where some relatively larger values of runoff, yet still characteristic of arid zones, are found.
Second, water scarcity increases over time in the region; this is reasonable to expect given increased pressure in water resources (increased demand) due to population growth, development and other factors (see Table 1 and Supplementary Figure S6). It also appears that moderate and higher values of water scarcity in the region advance significantly within the next few decades, and towards the second half of the century (Table 2 and Supplementary Figure S7).
Third, water scarcity is dominated by water demands rather than by climate-influenced water availability (surface and groundwater). The WSI results are fairly consistent among the three climate models used (see Table 2). This is consistent with previous findings (e.g., Vorosmarty et al., 2000; Graham et al., 2020) that have shown that human influence, rather than that posed by climate, drives water scarcity in the region. Importantly, this result highlights the potential value of careful management of water resources and demand-side measures in alleviating the water scarcity problem. Also, while the results are based on the AR5 climate data rather than on the most recent AR6 climate data, which have been shown to be less bias in the MENA region (Hamed et al., 2022), the finding that human systems dominate the scarcity signal in the MENA region is still robust given that the difference in renewable water supply due to climate change is much smaller than the delta in water demand (Table 1).
By implementing the SSP scenarios in GCAM including the assumptions shown in Supplementary Table S1, we simulate the water withdrawals associated with each of the five SSPs (Figure 4 and Supplementary Figure S8). This analysis shows that water demands are generally lowest in SSP4, where access to a range of basic water needs and service demands is low for a large share of the population, and highest in the SSP5 world due to resource-intensive lifestyles and low concerns about environmental impacts. Moreover, the total water demand increases substantially by the end of the century in SSP3 due to fast growing population and resource-intensive consumption patterns. Aside from SSP3, all scenarios show a decline in withdrawals in the second half of the century which are partially due to population declines. However, all five SSP scenarios show substantial increase in water withdrawals in the first half of the century, posing a great threat to the existing water scarcity challenge in the MENA region.
FIGURE 4. (A) Total annual water withdrawal in the MENA region under each of the SSPs. (B) and (C) Total annual water withdrawal in the two MENA subregions under each of the SSPs. All scenarios assume unlimited water resources such that all water demands can be achieved with no water constraints (note that the SSP2 scenario is the same as the UnlimitedWater scenario described in Section 2.4.3).
Supplementary Figure S9 shows the water scarcity map for the years 2050 and 2,100 produced using data from the GISS model, which is the GCM that represents average climate conditions in the MENA region according to the analysis presented in Supplementary Figure S4. Note, in all of these scenarios water is not constrained, and as such the total water withdrawal can exceed the total amount of runoff in a region. There are 15 scenarios for each country (5 SSPs x 3 GCMs). Supplementary Table S2 summarizes the range of water scarcity values for each of the countries in the MENA region. Almost all MENA countries have a WSI of greater than one (red tones in Supplementary Figure S9), which implies that the total projected water demand exceeds the total amount of runoff in that country. This means that a large fraction of the water demand cannot be met with runoff, which may lead to investments on non-conventional sources (e.g., desalinization, and non-renewable groundwater), efficiency and reallocation or, as in the case of Yemen, to massive overexploitation of unrenewable groundwater resources.
The highest WSI values in the MENA region are generally associated with countries in the Arabian Peninsula region such as Saudi Arabia, United Arab Emirates, and Qatar. This is mainly due to the lack of surface water resources (no rivers or lakes), combined with high per capita income, and population growth projections; i.e., extremely low renewable water resources with relatively high water demands. Many of these countries are also relatively young in terms of their population age distribution and have high fertility rates, compounding future water scarcity challenges. Some of these countries are already heavily dependent on non-traditional water sources such as desalination to meet most of their current demands. Note, existing desalination capacities and fossil groundwater reserves are not included in our definition of WSI.
The scenarios in Section 3.2 explored water scarcity in the MENA region under the assumption that there are no limits to water demand. We found that water withdrawals frequently exceeded runoff in MENA countries, which is a clear symptom of unsustainable water use. Here, we explore potential responses to limited water. In particular, we investigate the two water resources management scenarios: UnlimitedWater and LimitedWater. As noted, these scenarios are based on the SSP2 assumptions for socioeconomics and RCP6.0 for climate using the GISS climate model.
Figure 5 and Supplementary Figure S10 illustrate the impact of constraining water withdrawals to the amount of water available at the basin level (LimitedWater scenario) in comparison to unconstrained water withdrawal (UnlimitedWater scenario). Water demands drop when constraining water in GCAM since water demands in the MENA region would exceed available resources if assumed unlimited. In some regions, the implications of limits on water availability can be particularly extreme. This is especially evident in regions such as Arabian Peninsula where water demands already far exceed the limited runoff, and a sizable portion of the groundwater resource has been depleted over the past several decades, leaving them to the expensive desalination option. The impact is much larger in regions with existing low levels of water availability such as Arabian Peninsula and Yemen, and less pronounced in regions such as Algeria, Morocco-Western-Sahara, Egypt and Tunisia which have pockets of somewhat higher water availability (e.g., see Supplementary Figure S5).
FIGURE 5. Projections of total water demand by MENA subregion.(A, B) Comparison across water resources management scenarios. (C, D) Withdrawals broken by demand sectors under the LimitedWater scenario. (E, F) Withdrawals broken by the three sources of water (renewable, groundwater, and desalinated water) under the LimitedWater scenario.
Supplementary Figure S11 shows the distribution of water demand by source at the country level broken into three primary sources: renewable (surface water and groundwater), depletable (non-renewable) groundwater, and desalination. It is important to note that this distribution is driven by physical factors (availability of each source) as well as economic factors (cost of each source). For instance, except in Jordan, continued depletion of groundwater either represents a very small fraction of demand, or is halted completely in most countries in the region. Desalination gains prominence in several countries (e.g., Yemen, Jordan, UAE, Saudi Arabia, Kuwait) who use it to sustain a growing demand that is constrained to in-basin water availability (LimitedWater). Other countries like Tunisia, Egypt, Algeria, Iran, Libya and Morocco-Western-Sahara are able to sustain demand with renewable water sources.
Also, worth noting in these results is the interannual variability of the renewable water demand in some countries. These interannual changes are due to a combination of different factors. Some countries have no rivers and very limited surface water resources, with most of the renewable water resource in the form of renewable groundwater (e.g., UAE, Kuwait). These countries show little to no interannual variability in renewable water demand because the size of the renewable supply sources constitute only a small fraction of the total demand. As such the internal variability is masked out by the scale of the water demand and the none traditional water sources (fossil groundwater, desalination). In the rest of the region, the demand fluctuation can be attributed to the fluctuation in the renewable water term which is driven by the climate variability; when water supply is low, water demand also drops due to higher costs attributed to reliance on non-traditional sources. However, countries with sufficient groundwater resources that are competitive throughout the century end up balancing the internal variability in the renewable supply by pumping more groundwater (e.g., Jordan).
Future research efforts should focus on compiling country-specific data on the fractions of the various sources of water (i.e., renewable water, non-renewable water, and desalinated water) used to meet historical water demand to improve GCAM-MENA’s ability to account for existing projects of utilizing non-traditional water resources, e.g., the Great Man-Made River in Libya, which is not accounted for in our results. Also, future work could look into the feasibility of certain investments to materialize (e.g., installations of major desalination plants) in war-torn countries like Yemen, and as such future scenarios could explore devising delayed penetrations of certain technologies or investments.
A primary response to water scarcity in regions with severe water limitations is desalination. Desalination exceeds 10 percent of total water demand for the region under the LimitedWater scenario (compare Supplementary Figure S12A, S13B). These results greatly vary by country with the highest use of desalination in those countries with the greatest WSI. Without desalination, it would be necessary to reduce water withdrawals, which includes the Arabian Peninsula, Jordan, Kuwait, and the UAE. The diminishing slope over time (plateaus in the second half of century) is mainly explained by the shape of the water demand projections (see Figure 4A) which exhibit a similar trend. Also, the fluctuations and the drop toward the end of the century are driven by the inherent variability in the renewable water resource in the region; i.e., the period towards 2100 is relatively wet in the reference climate scenario used to generate these results. Thus, a smaller amount of desalinated water is produced. Note that different climate scenarios would lead to different results.
While desalination serves to some degree as a backup water supply, it does not come without a cost. The cumulative investment in the LimitedWater scenario reaches about $40 billion by 2050 and exceeds $100 billion by 2,100 (Figure 6). It is important to note that the demand for desalination and the associated investment needs would vary noticeably among the SSPs (SSP2 is used in LimitedWater), based on the demands for water in the different scenarios. Also, the projected increase in desalination using current technologies would have serious implications on marine ecosystems due to brine disposal, and mitigating such negative environmental concerns would incur additional costs to the MENA region.
FIGURE 6. Cumulative investment cost that is required to meet the projected desalinated water demand in MENA subregions (LimitedWater scenario).
Limits on water availability will have important impacts across economic sectors. Given the necessity for water in agriculture, the impacts on that sector are particularly important. The overall agricultural production is considerably reduced at the regional level, particularly in the Middle East (Figures 7A–D). However, there are pronounced differences at the country level. Under the LimitedWater scenario, the effect of limiting water is a large reduction in total withdrawals in regions that have water demands exceeding their renewable water supplies. In these regions, their water demands dropped substantially under the LimitedWater scenario compared to the UnlimitedWater scenario (as shown in Supplementary Figures S10, S13). Thus, the most pronounced effects on total crop production are found in the Arabian Peninsula and Yemen, and to a lower extent in Kuwait (Supplementary Figures S14, S15). Agricultural production decreases almost three-fold in Arabian Peninsula and approximately 60 percent in Yemen.
FIGURE 7. (A–D) Total agricultural production per crop type in the MENA region (in Mt). (E–H) Total demand (consumption) for agricultural crops in the MENA region (in Mt).
At the same time, the reduction in domestic production of agricultural commodities that arises as a result of constraining water in the MENA region can significantly impact the patterns of agricultural trade in the region. If supplies of agricultural commodities drop in one region, other regions will make up for that loss, and demands can still be met. In other words, when water is constrained in the MENA region, agricultural production is reduced due to lack of water. These regions are forced to import their agricultural needs from other regions where water and land resources are not limited. Thus, demand decrease is negligible in the region (Figures 7E–H and Supplementary Figures S16, S17). At the country level, the effects on the agricultural trade are relatively more pronounced for regions such as Iran, Arabian Peninsula and Yemen (Supplementary Figures S18A, B). As these countries face higher costs and depleting water resources, they become even more reliant on importing agricultural commodities; hence, they experience a large decline in their net agricultural exports. The result of these changes in terms of trade is a change in agricultural revenues (Figure 8 and Supplementary Figure S18C). The Middle East region experiences a cumulative loss of over $2 trillion in comparison to the hypothetical UnlimitedWater scenario. This is approximately twice the loss in agricultural revenues in the Middle East region if we were to assume the current investments to stay constant throughout the remainder of the century. Non-etheless, it is important to note that these estimates are based on a comparison to a hypothetical benchmark scenario (i.e., UnlimitedWater) which assumes that all necessary water resources are available. Future research could explore such losses in terms of forgone agricultural revenues across different water management scenarios beyond the one explored in this study.
FIGURE 8. Implications for the agricultural exports in the MENA region. Total net exports (billion $) in the agricultural sector under the (A) UnlimitedWater and (B) LimitedWater (scenarios. (C) Differences in net agricultural exports in the MENA region. (D) Cumulative change (losses) in net exports (billion $) in the agricultural sector in MENA (LimitedWater vs. UnlimitedWater scenarios).
It is also important to note that there are several assumptions behind these results, notably a robust market for international trade in agricultural products and a willingness of countries to increase their dependence on imported agricultural goods. Were either of these assumptions not to hold in reality—for example, for reasons of food security and self-sufficiency—this might imply smaller changes in domestic production, the use of lower-water crops, greater use of highly-efficient irrigation technologies, and lower domestic consumption. All of these implications would be valuable to explore in future analyses. Also, sensitivity of the investment cost results to changes in interest rate is an issue that should be explored further. The capital recovery factor formulation used in this work is sensitive to changes in interest rates. Interest rates differ from one country to another as well, so this implies the need for more detailed financial analyses of different nexus configurations among countries in the region.
Agriculture is not the only sector influenced by limits on water availability. Because electricity generation uses water for cooling, this sector is also subject to the effects of water availability. Note that a description on how electricity generation is produced in GCAM can be found in Calvin et al., 2019 and in the online GCAM documentation (JGCRI 2022). Overall, the results of this work suggest that the effect on electricity production is negligible (on the order of 0.1 EJ, or 0.5 percent difference between the two scenarios; Supplementary Figure S19). The reason for this is the inherent flexibility of the electricity sector to reduce its water footprint through shifting fuels and water-cooling technologies (Supplementary Figure S20). In addition, the MENA region already relies extensively on cooling technologies with limited withdrawals of renewable water, such as the use of seawater, recirculating cooling, and dry cooling. This means that only modest adjustments are necessary to address water limitations. Indeed, the modest percentage of once-through cooling used in the region shown in Supplementary Figure S20 for the UnlimitedWater scenario is dramatically decreased in the presence of water limits. The water withdrawal-intensity of the once-through cooling technology is roughly an order of magnitude higher than the recirculating cooling technology, so even a small change in fraction in once-through may still yield large reductions in water withdrawals. Non-etheless, water limits do alter the electricity investment profile in the region (Figure 9 and Supplementary Figure S21). Regions that are faced with the most stringent water stress conditions (e.g., Iraq, Arabian Peninsula, Yemen) are likely to incur the highest cost due to additional investments in more expensive power technologies and more expensive cooling options (Supplementary Figure S21A). While most countries experience only a modest increase in investment, the cumulative total through the end of the century exceeds $100 billion for the difference between the LimitedWater and UnlimitedWater scenarios considered here (Figure 9A). In part, this is due to increases of needed investments in more expensive, but lower water-intensive technologies such as solar and wind power (Figures 9B, C). It is interesting to note that these investments would be incurred in scenarios focused on reducing greenhouse gas emissions. Multiple studies (e.g., Kyle et al., 2013; Strzepek et al., 2014; Wallis et al., 2014; Liu et al., 2015) have demonstrated how efforts to decrease greenhouse gas emissions are synergistic with efforts to reduce water consumption for electricity.
FIGURE 9. (A) Difference in cumulative total investment in the electricity sector between the LimitedWater and UnlimitedWater scenarios. The dashed-black line is the total sum across all 15 GCAM-MENA regions. (B, C) Difference in cumulative total investment in the electricity sector between the LimitedWater and UnlimitedWater scenarios, and by electricity technology. The dashed-black line is the total sum across all 17 technologies (as well as for the entire MENA region).
Through this study, an analytical framework to analyze the water-energy-food nexus in the MENA region has been developed and illustrated so that it can be used to help formulate integrated (nexus) approaches for water, energy and food activities in the MENA countries. This analytical framework is based on an IAM for the MENA region (GCAM-MENA), which implements an integrated resource (nexus) modeling approach. This research places focus on: i) using GCAM-MENA to analyze the current status of water resources in the region; ii) a scenario analysis focused on water scarcity and potential impacts on other sectors in the region (i.e., agriculture, electricity); and iii) recommendations for further analysis that can inform policy making and contribute to ongoing efforts towards integrated planning at the regional level.
The analysis of current and projected water scarcity results obtained from the GCAM-MENA model show a general trend upwards in the majority of countries of the MENA region, under a variety of climate (RCP) and socioeconomic development (SSP) scenarios. This is reasonable to expect given increased pressure in water resources (increased demand) as a result of population growth, development and other factors. The water scarcity index results are found to be fairly consistent among three climate models used (dry, average and wet), suggesting that water scarcity is dominated by water demands rather than by the climate-influenced water availability (surface and groundwater). It appears that severe and moderate water scarcity around the region advance significantly within the next few decades (i.e., through 2050) throughout the region. Therefore, it is important for MENA countries to be proactive on both the supply side (expand sources, e.g., desalination, water reuse) and demand side (e.g., agricultural efficiency) moving forward.
Two water resources management scenarios (UnlimitedWater and LimitedWater) were comparatively analyzed to understand the effects of constraining water use at the basin level in an effort to curb water demand for multiple uses. Constraining water is found to translate into impacts on water use for agricultural production across a number of crops. Countries can optimize their return on water by choosing different crop mixes, which will lead to different returns on the agricultural water used. Relatively large reductions in agricultural production occur in the Arabian Peninsula (almost 3-fold reduction when limiting water) and Yemen (approx. 60 percent overall reduction) as a result of constraining water demand. This reduction in production of agricultural commodities that arises as a result of constraining water in the MENA region does not necessarily imply a reduction in consumption of agricultural goods, assuming robust international trade, willingness to increase reliance on agricultural imports and existence of financial resources for agricultural imports. Even in this circumstance, however, the reduction in production does have an important impact on the magnitude of agricultural exports from the region; these impacts were found to be more pronounced for regions such as Iran, Arabian Peninsula and Yemen. The result of these changes in terms of trade is a reduction in agricultural revenues through 2,100; The Arabian Peninsula region experiences a cumulative loss of over $1.2 trillion, followed by Iran (over $400 billion), Yemen (over $200 billion) and other countries in the region.
With respect to energy security, countries with the most stringent water stress conditions (e.g., Iraq, Arabian Peninsula, Yemen) are likely to incur the highest cost due to additional investments in more expensive power technologies and cooling options. We estimate over $100 billion in additional investments for the difference between the LimitedWater and UnlimitedWater scenarios. However, there are other dimensions to the investment story. For example, wind and solar PV investments in the region have the dual benefit of less water use and less greenhouse gas emissions from the power sector as opposed to having these electrons generated from fossil fuels such as liquid fuels or natural gas. Furthermore, many countries in the MENA region (e.g., Morocco, Saudi Arabia, United Arab Emirates, among others) have made ambitious climate mitigation targets to achieve net-zero emissions by mid-century (Climate Action Tracker, 2022), thus, understanding the interplay between climate security and the WEF nexus in the MENA region is critically important especially given that infrastructural investments often last for decades.
By providing an economic quantitative framework for integrated analysis of water supply and demand, multiple demand sectors, climate inputs, and other forcing factors such as land use change, policy interventions and technological developments, integrated assessment models such as GCAM-MENA provide a viable tool to explore additional issues related to the water-energy-food nexus. As noted by Albrecht et al. (2018), there is a lack of models and analytical tools to address nexus trade-offs. Further research along these lines can be focused on such issues as the implications of water reuse (particularly wastewater recycling) as a future water supply and its effect on urban services, food and energy security; the effects of sudden extreme events or shocks of physical or socioeconomic natures; the repercussions of removing existing distortions (i.e., subsidies) in water availability and distribution in the future; the economic costs (of inaction) of non-cooperation across basins/countries/regions and the potential benefits of cooperation; quantify tradeoffs in water availability and its impact on major economic sectors; define effective adaptation strategies/investments that are necessary to mitigate the impact of climate change on water scarcity and stress; identify and plan key investments at regional and country levels to address economic water scarcity.
The analysis performed through this research can contribute to identify synergies to meet sectoral needs in a manner consistent with regional goals of environmental sustainability, water-energy-food security and socioeconomic development. The results of this analysis can be used to incorporate nexus approaches in the formulation of planning practices and design investments in the region. This work can contribute to building integrated planning capabilities in MENA countries and help flag any potential constraints and opportunities that may arise from an integrated long-term view at water, energy and food needs in the region. Climate change impacts are also incorporated in this exercise in order to facilitate robust and resilient WEF sector development planning. It is important to note that the analytical framework developed for this analysis could be transferrable to other geographical regions for extensive and holistic WEF nexus research.
Managing the water-energy-food nexus in the region and satisfying the future water needs of all sectors is a strategic challenge for the MENA region for the coming years. In some parts of the region, the combined effects of population growth, increasing hydrological variability and climate change may result in increased reliance on relatively energy-intensive water supply options. At the same time, agriculture is expected to continue to pose major pressures on the region’s diminishing water supplies. The nexus poses not only challenges for sustainability in the MENA region, but also for the region’s food, energy and water security, and improving its social, economic and political stability.
The raw data supporting the conclusion of this article will be made available by the authors, without undue reservation.
MH: conceptualization, formal analysis, methodology, supervision, writing—original draft, writing—review. FM-W: conceptualization, formal analysis, methodology, project administration, supervision, writing—original draft, writing—review. All other authors listed have made contributions to the overall investigation and/or formal analyses. The final version of the manuscript was reviewed by all the authors. The final edits were compiled by SRSdS who converted the manuscript to the final format for this journal.
This research was funded by The World Bank Group, contract No. 7179474. The Nature Conservancy provided support to FM-W under Project P118989.
The authors would like to thank Catherine Yonkofski and David Watson for their valuable efforts to the development of this research. The original research reported in this manuscript was part of a report that supported a study led by The World Bank Group entitled “The Water-Energy-Food Nexus in the Middle East and North Africa: Scenarios for a Sustainable Future.” The original report is available online at: https://openknowledge.worldbank.org/handle/10986/30185.
Author YL was employed by Kettle Reinsurance Ltd.
The remaining authors declare that the research was conducted in the absence of any commercial or financial relationships that could be construed as a potential conflict of interest.
All claims expressed in this article are solely those of the authors and do not necessarily represent those of their affiliated organizations, or those of the publisher, the editors and the reviewers. Any product that may be evaluated in this article, or claim that may be made by its manufacturer, is not guaranteed or endorsed by the publisher.
The Supplementary Material for this article can be found online at: https://www.frontiersin.org/articles/10.3389/fenvs.2023.1082930/full#supplementary-material
Ajjur, S. B., and Al-Ghamdi, S. G. (2021). Evapotranspiration and water availability response to climate change in the Middle East and North Africa. Clim. Change 166, 28. doi:10.1007/s10584-021-03122-z
Al-Badi, A., and AlMubarak, I. (2019). Growing energy demand in the GCC countries. Arab J. Basic Appl. Sci. 26 (1), 488–496. doi:10.1080/25765299.2019.1687396
Albrecht, T. R., Crootof, A., and Scott, C. A. (2018). The water-energy-food nexus: A systematic review of methods for nexus assessment. Environ. Res. Lett. 13 (4), 043002. doi:10.1088/1748-9326/aaa9c6
Bao, Y., Song, Z., and Qiao, F. (2020). FIO-ESM version 2.0: Model description and evaluation. J. Geophys. Res. Oceans 125, e2019JC016036. doi:10.1029/2019JC016036
Bazilian, M., Rogner, H., Howells, M., Hermann, S., Arent, D., Gielen, D., et al. (2011). Considering the energy, water and food nexus: Towards an integrated modelling approach. Energy Policy 39 (12), 7896–7906.
Caldera, U., Bogdanov, D., Afanasyeva, S., and Breyer, C. (2018). Role of seawater desalination in the management of an integrated water and 100% renewable energy based power sector in Saudi Arabia. Water 10 (1), 3. doi:10.3390/w10010003
Calvin, K., Patel, P., Clarke, L., Asrar, G., Bond-Lamberty, B., Cui, R. Y., et al. (2019). GCAM v5. 1: Representing the linkages between energy, water, land, climate, and economic systems. Geosci. Model. Dev. 12, 677–698. doi:10.5194/gmd-12-677-2019
Campbell, A. A., de Pee, S., Sun, K., Kraemer, K., Thorne-Lyman, A., Moench-Pfanner, R., et al. (2010). Household rice expenditure and maternal and child nutritional status in Bangladesh. J. Nutr. 140, 189S–194S. doi:10.3945/jn.109.110718
Chaturvedi, V., Hejazi, M. I., Edmonds, J., Clarke, L., Kyle, P., Davies, E., et al. (2013). Climate mitigation policy implications for global irrigation water demand. Mitig. Adapt. Strategies Glob. Change 20, 1–19. doi:10.1007/s11027-013-9497-4
Clarke, L. (2014). “Assessing transformation pathways. Climate change 2014: Mitigation of climate change,” in Contribution of working group III to the fifth assessment report of the intergovernmental panel on climate change. Editor O. Edenhofer (Cambridge University Press).
Climate Action Tracker (2022). Climate action tracker. Available at: https://climateactiontracker.org/global/cat-net-zero-target-evaluations/.
Cui, R. Y., Waldhoff, S., Clarke, L., Hultman, N., and Gilmore, E. (2016). An integrated assessment of regional food availability and access along alternative futures. Working Paper.
D’Odorico, P., Davis, K. F., Rosa, L., Carr, J. A., Chiarelli, D., Dell'Angelo, J., et al. (2018). The global food-energy-water nexus. Rev. Geophys. 56 (3), 456–531. doi:10.1029/2017rg000591
Dorward, A. (2012). The short- and medium-term impacts of rises in staple food prices. Food Sec 4, 633–645. doi:10.1007/s12571-012-0210-3
Droogers, P., Immerzeel, W. W., Terink, W., Hoogeveen, J., Bierkens, M. F. P., van Beek, L. P. H., et al. (2012). Water resources trends in Middle East and North Africa towards 2050, hydrol. Earth Syst. Sci. 16, 3101–3114. doi:10.5194/hess-16-3101-2012
du Plessis, A. (2017). “Water scarcity and other significant challenges for South Africa,” in Freshwater challenges of South Africa and its upper vaal river (Cham: Springer Water. Springer). doi:10.1007/978-3-319-49502-6_7
Feijoo, F., Iyer, G., Binsted, M., and Edmonds, J. (2020). US energy system transitions under cumulative emissions budgets. Clim. Change 162, 1947–1963. doi:10.1007/s10584-020-02670-0
Gent, P., Danabasoglu, G., Donner, L. J., Holland, M. M., Hunke, E. C., Jayne, S. R., et al. (2011). The community climate system model version 4. J. Clim. 24 (19), 4973–4991. doi:10.1175/2011JCLI4083.1
Graham, N. T., Hejazi, M. I., Chen, M., Davies, E. G. R., Edmonds, J. A., Kim, S. H., et al. (2020). Humans drive future water scarcity changes across all Shared Socioeconomic Pathways. Environ. Res. Lett. 15, 014007. doi:10.1088/1748-9326/ab639b
Hamed, M. M., Nashwan, M. S., Shiru, M. S., and Shahid, S. (2022). Comparison between CMIP5 and CMIP6 models over MENA region using historical simulations and future projections. Sustainability 14 (16), 10375. doi:10.3390/su141610375
Hejazi, M. I., Edmonds, J., Clarke, L., Kyle, P., Davies, E., Chaturvedi, V., et al. (2014a). Long-term global water projections using six socioeconomic scenarios in an integrated assessment modeling framework. Technol. Forecast. Soc. Change 81, 205–226. doi:10.1016/j.techfore.2013.05.006
Hejazi, M. I., Edmonds, J., Clarke, L., Kyle, P., Davies, E., Chaturvedi, V., et al. (2014b). Integrated assessment of global water scarcity over the 21st century under multiple climate change mitigation policies. Hydrol. Earth Syst. Sci. 18 (8), 2859–2883. doi:10.5194/hess-18-2859-2014
Hertel, T. W. (2016). Food security under climate change. Nat. Clim. Change 6, 10–13. doi:10.1038/nclimate2834
Hijioka, Y., Lin, E., Pereira, J. J., Corlett, R. T., Cui, X., Insarov, G. E., et al. (2014). “Asia,” in Climate change 2014: Impacts, adaptation, and vulnerability. Part B: Regional aspects. Contribution of working group II to the fifth assessment report of the intergovernmental panel on climate change. Editors V. R. Barros, C. B. Field, D. J. Dokken, M. D. Mastrandrea, K. J. Mach, T. E. Biliret al. (Cambridge, United Kingdom and New York, NY, USA: Cambridge University Press), 1327–1370.
Hoff, H., Alrahaife, S. A., Hajj, R. E., Lohr, K., Mengoub, F. E., Farajalla, N., et al. (2019). A nexus approach for the MENA region—from concept to knowledge to action. Front. Environ. Sci., Sec. Freshw. Sci. 7. doi:10.3389/fenvs.2019.00048
Hussain, I., and Bhattarai, M. (2004). “Comprehensive assessment of socio-economic impacts of agricultural water uses: Concepts, approaches and analytical tools,” in Draft report of work carried out at the international water management Institute (IWMI) under its multi-country projects: (a) comprehensive assessment of costs and benefits of agricultural water management, and (b) an ADB funded project on pro-poor intervention strategies in irrigated agriculture in asia (CGSpace), 38. Available at: http://hdl.handle.net/10568/39777.
Huttner, K. R. (2013). Overview of existing water and energy policies in the MENA region and potential policy approaches to overcome the existing barriers to desalination using renewable energies. Desalination Water Treat. 51 (1-3), 87–94. doi:10.1080/19443994.2012.704741
Iannotti, L., Robles, M., Pachón, H., and Chiarella, C. (2012). Food prices and poverty negatively affect micronutrient intakes in Guatemala. J. Nutr. 142, 1568–1576. doi:10.3945/jn.111.157321
Immerzeel, W., Droogers, P., Terink, W., Hoogeveen, J., Hellegers, P., and Bierkens, M. (2011). Middle-East and northern Africa water outlook. Wageningen: World Bank Report. Available at: https://www.futurewater.eu/projects/mena/
JGCRI (2022). GCAM v6 documentation: Joint global change research institute. Available at: http://jgcri.github.io/gcam-doc/index.html (Accessed September 09, 2022).
Khan, Z., Wild, T. B., Iyer, G. C., Hejazi, M., and Vernon, C. R. (2021). The future evolution of energy-water-agriculture interconnectivity across the US. Environ. Res. Lett. 16, 065010. doi:10.1088/1748-9326/ac046c
Khatib, H. (2014). Oil and natural gas prospects: Middle East and North Africa. Energy Policy 64, 71–77. ISSN 0301-4215. doi:10.1016/j.enpol.2013.07.091
Kyle, G. P., Davies, E. G. R., Dooley, J. J., Smith, S. J., Clarke, L. E., Edmonds, J. A., et al. (2013). Influence of climate change mitigation technology on global demands of water for electricity generation. Int. J. Greenh. Gas Control 13, 112–123. doi:10.1016/j.ijggc.2012.12.006
Kyle, P., Müller, C., Calvin, K., and Thomson, A. (2014). Meeting the radiative forcing targets of the representative concentration pathways in a world with agricultural climate impacts. Earth's Future 2, 83–98. doi:10.1002/2013ef000199
Lelieveld, J., Proestos, Y., Hadjinicolaou, P., Tanarhte, M., Tyrlis, E., and Zittis, G. (2016). Strongly increasing heat extremes in the Middle East and North Africa (MENA) in the 21st century. Clim. Change 137, 245–260. doi:10.1007/s10584-016-1665-6
Liu, L., Hejazi, M. I., Patel, P., Kyle, P., Davies, E., Zhou, Y., et al. (2015). Water demands for electricity generation in the U.S.: Modeling different scenarios for the water-energy nexus. Technol. Forecast. Soc. Change 94, 318–334. doi:10.1016/j.techfore.2014.11.004
Liu, W., Liu, X., Yang, H., Ciais, P., and Wada, Y. (2022). Global water scarcity assessment incorporating green water in crop production. Water Resour. Res. 58, e2020WR028570. doi:10.1029/2020WR028570
Mekonnen, M. M., and Hoekstra, A. Y. (2016). Four billion people facing severe water scarcity. Sci. Adv. 2, e1500323. doi:10.1126/sciadv.1500323
Moss, R., Babiker, M., Brinkman, S., Calvo, E., Carter, T., Edmonds, J., et al. (2008). Towards new scenarios for analysis of emissions, climate change, impacts, and response strategies. Geneva: Intergovernmental Panel on Climate Change, 132.
Nelson, G. C., Valin, H., Sands, R. D., Havlik, P., Ahammad, H., Deryng, D., et al. (2014). Climate change effects on agriculture: Economic responses to biophysical shocks. Proc. Natl. Acad. Sci. 111, 3274–3279. doi:10.1073/pnas.1222465110
Niazi, H., Wild, T., Graham, N., Yonkofski, C., Watson, D., Hejazi, M., et al. (2022). Cost and accessibility of global nonrenewable groundwater resources. (In-prep).
O’Neill, B., Kriegler, E., Ebi, K., Kemp-Benedict, E., Riahi, K., Rothman, D., et al. (2015). The roads ahead: Narratives for shared socioeconomic pathways describing world futures in the 21st century. Glob. Environ. Change 42, 169–180. doi:10.1016/j.gloenvcha.2015.01.004
O’Neill, B., Tebaldi, C., van Vuuren, D. P., Eyring, V., Friedlingstein, P., Hurtt, G., et al. (2016). The scenario model intercomparison project (ScenarioMIP) for CMIP6. Model. Dev. 9, 3461–3482. doi:10.5194/gmd-9-3461-2016
Ozturk, T., Ceber, Z. P., Türkeş, M., and Kurnaz, M. L. (2015). Projections of climate change in the Mediterranean Basin by using downscaled global climate model outputs. Int. J. Climatol. 35, 4276–4292. doi:10.1002/joc.4285
Paltán, H. A., Pant, R., Plummer Braeckman, J., and Dadson, S. J. (2021). Increased water risks to global hydropower in 1.5 °C and 2.0 °C Warmer Worlds. J. Hydrology 599, 126503. doi:10.1016/j.jhydrol.2021.126503
Parkinson, S. C., Djilali, N., Krey, V., Oliver, F., Johnson, N., Khan, Z., et al. (2016). Impacts of groundwater constraints on Saudi arabia’s low-carbon electricity supply strategy. Environ. Sci. Technol. 50 (4), 1653–1662. doi:10.1021/acs.est.5b05852
Parkinson, S. C., Krey, V., Huppmann, D., Kahil, T., McCollum, D., Fricko, O., et al. (2019). Balancing clean water-climate change mitigation trade-offs. Environ. Res. Lett. 14, 014009. doi:10.1088/1748-9326/aaf2a3
Qiao, F., Song, Z., Bao, Y., Song, Y., Shu, Q., Huang, C., et al. (2013). Development and evaluation of an Earth System Model withsurface gravity waves. J. Geophys. Res. Oceans 118, 4514–4524. doi:10.1002/jgrc.20327
Radcliffe, J. C. (2018). The water energy nexus in Australia – the outcome of two crises. Water-Energy Nexus 1 (1), 66–85. doi:10.1016/j.wen.2018.07.003
Rosenzweig, C., Elliott, J., Deryng, D., Ruane, A. C., Muller, C., Arneth, A., et al. (2014). Assessing agricultural risks of climate change in the 21st century in a global gridded crop model intercomparison. Proc. Natl. Acad. Sci. 111, 3268–3273. doi:10.1073/pnas.1222463110
Schmidt, G. A., Kelley, M., Nazarenko, L., Ruedy, R., Russell, G. L., Aleinov, I., et al. (2014). Configuration and assessment of the GISS ModelE2 contributions to the CMIP5 archive. J. Adv. Model. Earth Syst. 6 (1), 141–184. doi:10.1002/2013MS000265
Shaw, R., Luo, Y., Cheong, T. S., Abdul Halim, S., Chaturvedi, S., Hashizume, M., et al. (2022). “Asia,” in Climate change 2022: Impacts, adaptation and vulnerability. Contribution of working group II to the sixth assessment report of the intergovernmental panel on climate change. Editors H.-O. Pörtner, D. C. Roberts, M. Tignor, E. S. Poloczanska, K. Mintenbeck, A. Alegríaet al. (Cambridge, UK and New York, NY, USA: Cambridge University Press), 1457–1579. doi:10.1017/9781009325844.012
Siddiqi, A., and Anadon, L. D. (2011). The water–energy nexus in Middle East and North Africa. Energy Policy 39 (8), 4529–4540. doi:10.1016/j.enpol.2011.04.023
Strzepek, K., Smith, J., Martinich, J., Neumann, J., Boehlert, B., Hejazi, M. I., et al. (2014). Benefits of greenhouse gas mitigation on the supply, management, and use of water resources in the United States. Clim. Change 131, 1–15. special issue. doi:10.1007/s10584-014-1279-9
Torlesse, H., Kiess, L., and Bloem, M. W. (2003). Association of household rice expenditure with child nutritional status indicates a role for macroeconomic food policy in combating malnutrition. J. Nutr. 133, 1320–1325. doi:10.1093/jn/133.5.1320
Trisos, C. H., Adelekan, I. O., Totin, E., Ayanlade, A., Efitre, J., Gemeda, A., et al. (2022). “Africa,” in Climate change 2022: Impacts, adaptation and vulnerability. Contribution of working group II to the sixth assessment report of the intergovernmental panel on climate change. Editors H.-O. Pörtner, D. C. Roberts, M. Tignor, E. S. Poloczanska, K. Mintenbeck, A. Alegríaet al. (Cambridge, UK and New York, NY, USA: Cambridge University Press), 1285–1455. doi:10.1017/9781009325844.011
Turner, S., Hejazi, M. I., Yonkofski, C., Kim, S., and Kyle, P. (2019a). Influence of groundwater extraction costs and resource depletion limits on simulated global nonrenewable water withdrawals over the twenty-first century. Earth's Future 7 (2), 123–135. doi:10.1029/2018EF001105
Turner, S., Hejazi, M., Calvin, K., Page, K., and Kim, S. (2019b). A pathway of global food supply adaptation in a world with increasingly constrained groundwater. Sci. Total Environ. 673, 165–176. doi:10.1016/j.scitotenv.2019.04.070
D. Verner (Editor) (2012). Adaptation to a changing climate in the Arab countries (Washington, DC: World Bank). doi:10.1596/978-0-8213-9458-8
Voisin, N., Li, H., Ward, D., Huang, M., Wigmosta, M., and Leung, L. R. (2013). On an improved sub-regional water resources management representation for integration into Earth system models. Hydrol. Earth Syst. Sci. 17 (9), 3605–3622. doi:10.5194/hess-17-3605-2013
Vorosmarty, C. J., Green, P., Salisbury, J., and Lammers, R. B. (2000). Global water resources: Vulnerability from climate change and population growth. Science 289 (5477), 284–288. doi:10.1126/science.289.5477.284
Waha, K., Krummenauer, L., Adams, S., Aich, V., Baarsch, F., Coumou, D., et al. (2017). Climate change impacts in the Middle East and Northern Africa (MENA) region and their implications for vulnerable population groups. Reg. Environ. Change 17 (6), 1623–1638. doi:10.1007/s10113-017-1144-2
Wallis, P. J., Ward, M. B., Pittock, J., Hussey, K., Bamsey, H., Denis, A., et al. (2014). The water impacts of climate change mitigation measures. Clim. Change 125, 209–220. doi:10.1007/s10584-014-1156-6
Weyant, J., Azar, C., Kainuma, M., Kejun, J., Nakicenovic, N., Shukla, P., et al. (2009). Report of 2.6 versus 2.9 watts/m2 RCPP evaluation panel (PDF). Geneva, Switzerland: IPCC Secretariat.
Wise, M., Calvin, K., Thomson, A., Clarke, L., Bond-Lamberty, B., Sands, R., et al. (2009). Implications of limiting CO2 concentrations for land use and energy. Science 324 (5931), 1183–1186. doi:10.1126/science.1168475
Keywords: nexus, water, energy, food, water scarcity, sustainability, GCAM, mena
Citation: Hejazi M, Santos Da Silva SR, Miralles-Wilhelm F, Kim S, Kyle P, Liu Y, Vernon C, Delgado A, Edmonds J and Clarke L (2023) Impacts of water scarcity on agricultural production and electricity generation in the Middle East and North Africa. Front. Environ. Sci. 11:1082930. doi: 10.3389/fenvs.2023.1082930
Received: 28 October 2022; Accepted: 30 January 2023;
Published: 27 February 2023.
Edited by:
Charles Vörösmarty, CUNY Advanced Science Research Center, United StatesReviewed by:
Yang Hong, University of Oklahoma, United StatesCopyright © 2023 Hejazi, Santos Da Silva, Miralles-Wilhelm, Kim, Kyle, Liu, Vernon, Delgado, Edmonds and Clarke. This is an open-access article distributed under the terms of the Creative Commons Attribution License (CC BY). The use, distribution or reproduction in other forums is permitted, provided the original author(s) and the copyright owner(s) are credited and that the original publication in this journal is cited, in accordance with accepted academic practice. No use, distribution or reproduction is permitted which does not comply with these terms.
*Correspondence: Silvia R. Santos Da Silva, c3NhbnRvc2RAZ211LmVkdQ==
Disclaimer: All claims expressed in this article are solely those of the authors and do not necessarily represent those of their affiliated organizations, or those of the publisher, the editors and the reviewers. Any product that may be evaluated in this article or claim that may be made by its manufacturer is not guaranteed or endorsed by the publisher.
Research integrity at Frontiers
Learn more about the work of our research integrity team to safeguard the quality of each article we publish.