- 1Aquatic Toxicology Lab, Department of Zoology, Guru Nanak Dev University, Amritsar, Punjab, India
- 2Department of Biosciences, University Institute of Biotechnology, Chandigarh University, Mohali, Punjab, India
- 3Molecular Microbiology Lab, Department of Molecular Biology and Biochemistry, Guru Nanak Dev University, Amritsar, Punjab, India
Triclosan 5-chloro-2-(2, 4-dichlorophenoxy) phenol (TCS) is widely used as a biocide in human and veterinary medicines, personal care products and household articles. To obtain biomarkers for the acute stress of Triclosan, the hatchlings of Labeo rohita were exposed for 96 h to 0.06, 0.067 and 0.097 mg/L TCS. Morphological deformities, cell viability, frequency of micronucleated and aberrant cells, transcriptomic and biomolecular alterations were recorded after exposure and a depuration period of 10 days. The exposed hatchlings had a pointed head, curved trunk, lean body, deformed caudal fin, haemorrhage, hypopigmentation and tissue degeneration at 0.067 and 0.097 mg/L only. The frequency of viable cells declined but that of necrotic, apoptotic, micronucleated and abnormal cells increased (p ≤ 0.01) in a concentration dependent manner after exposure as well as the depuration period. After recovery, the frequency of viable and micronucleated cells increased, but that of necrotic, apoptotic, and aberrant cells declined in comparison to their respective 96 h values. The mRNA level of HSP47, HSP70, HSc71 and α-tropomyosin increased (p ≤ 0.01), while that of HSP60, HSP90, DHPR, myosin light polypeptide 3, desmin b and lamin b1 declined (p ≤ 0.01) after exposure. Ten days post exposure, a significant increase (p ≤ 0.01) over control was observed in the expression of all the heat shock and cytoskeletal genes and the values (except for HSc71) were higher than the respective 96 h values also. Infrared spectra showed that band area of amide A, amide I, amide II and phospholipids increased significantly (p ≤ 0.01) but peak intensity of lipid, glycogen and nucleic acids decreased after exposure. After recovery, area of the peaks for most of the biomolecules [except lipids (2924–2925, 1455–1457 cm−1) and glycogen (1163–1165 cm−1)] declined significantly over control and 96 h values. Collectively these changes seem to be responsible not only for the onset of paralysis but also for the concentration dependent increase in larval and cellular abnormalities as well as no/sporadic swimming movement in exposed hatchlings. It is evident that HSP60, HSc71, HSP90, α-tropomyosin and DHPR were strongly affected but DHPR can be used as the most sensitive marker for the toxicity of TCS. This is the first study reporting effect of TCS on the selected heat shock and cytoskeletal genes in a single model.
Introduction
Triclosan [TCS, 5-chloro-2-(2, 4-dichlorophenoxy) phenol] is a non-antibiotic antimicrobial biocide that is commonly used in personal, household and healthcare products (Lu et al., 2018). It mainly enters water bodies and land through the wastewater and biosolids that are discharged from the wastewater treatment plants (Dann and Hontela, 2011). Excessive use of triclosan containing sanitizers and antiseptics during Covid-19 pandemic has raised concern about its risks to the environment and public health (Lu and Guo, 2021). High bioaccumulation factor (2.7–90) and octanol/water partition coefficient (4.8) of TCS increase the possibility of its build up in non-target aquatic organisms (Dhillon et al., 2015; Yao et al., 2018). High concentrations of TCS ranging between 13 and 1040 μg/kg and 91.1–589 μg/kg were detected in Gomti river (Uttar Pradesh) and Torsa river (West Bengal) of India, respectively (Nag et al., 2018; Sarkar et al., 2020). Presence of TCS in water has been reported to cause abnormal and delayed embryonic development (Dar et al., 2019), neurological and endocrine disruption (Wang and Tian, 2015; Kim et al., 2018), oxidative stress (Dar et al., 2020a; Dar et al., 2020b), histological changes (Gyimah et al., 2020), transcriptomic alterations and DNA damage in fish (Sharma et al., 2021a). TCS impairs excitation-contraction of skeletal and cardiac muscles by altering the interaction between dihydropyridine receptors (DHPRs) and Ca2+ release channels of sarcoplasmic reticulum (Ahn et al., 2008; Cherednichenko et al., 2012). These skeletal proteins play an important role in dendritic growth and synaptic plasticity (Pessah et al., 2010; Wayman et al., 2012) while disruption of Ca2+ homeostasis in fish and mammals leads to abnormal neuronal development and muscle contraction. Earlier reports show that TCS induces alterations in the expression of cytoskeletal genes in fresh water mussel Dreissena polymorpha (Riva et al., 2012), fathead minnow (Fritsch et al., 2013), zebra fish larvae (Falisse et al., 2017) and early life stages of zebrafish (Ma et al., 2019).
Previous studies reported cytotoxic and genotoxic effects of TCS in Closterium ehrenbergii (Ciniglia et al., 2005), Mytilus galloprovincialis (Canesi et al., 2007), Haliotis tuberculate (Gaume et al., 2012), Dreissena polymorpha (Binelli et al., 2009a; 2009b), Oreochromis niloticus (Vijitha et al., 2017), Carassius auratus (Wang et al., 2018), Danio rerio (Parenti et al., 2019), Pangasianodon hypophthalmus (Paul et al., 2019, 2020) and Clarias gariepinus (Jimoh and Sogbanmu, 2021). Cell viability and micronucleus/aberrant cell assay are the most commonly used approaches to assess cyto-genotoxic potential of such xenobiotics.
During stress, the most common type of cellular response in fish is the production of heat shock proteins (HSPs) for maintenance of protein integrity and function (Iwama et al., 1999). HSPs are the highly conserved families and play an essential role in folding, repair and transportation of proteins (Liu et al., 2013). They are also called “stress proteins” because HSPs show marked variations in their expression under the influence of stressors like reactive oxygen species, heavy metals, xenobiotics, pathogens etc. (Ponomarenko et al., 2013). TCS has been observed to alter mRNA copy number of HSPs in zebra mussel (Riva et al., 2012), Eisenia fetida (Lin et al., 2014), Brachionus koreanus (Han et al., 2016), zebrafish larvae (Falisse et al., 2017), rainbow trout (Capkin et al., 2017) and Caenorhabditis elegans (García-Espiñeira et al., 2018). Fourier transform infrared spectroscopy (FT-IR) is an important and sensitive technique for observing cellular changes at molecular level (Cakmak et al., 2006). It can detect structural and functional alterations in biomolecules (protein, lipid and nucleic acids) and the results can be used to predict the possible effects of stressors on the vital functions of an organism (Palaniappan and Vijayasundaram, 2008a). FT-IR has been successfully used to highlight changes in the biomolecules due to 17β-estradiol in the juveniles of rainbow trout (Cakmak et al., 2006), arsenic in the fingerlings of Labeo rohita (Palaniappan and Vijayasundaram, 2008a; Palaniappan and Vijayasundaram, 2008b; Palaniappan and Vijayasundaram, 2009a; Palaniappan and Vijayasundaram, 2009b) and TCS in the embryos of four food fishes (Dar et al., 2022).
TCS affects development and functioning of skeletal muscles by impairing the excitation-contraction coupling of skeletal muscles. HSPs participate in stabilization of cytoskeleton (Pijanowska and Kloc, 2004) and HSCs play significant role in support and repair of damaged cytoskeleton and maintenance of membrane lipoproteins of nuclear and cellular membrane (Roberts et al., 2010). Expression of HSPs is much more sensitive to stress than traditional health indices (Eder et al., 2009). Therefore, the present study envisaged to evaluate acute toxicity of TCS by taking into account larval abnormalities, cell viability, nucleo-cellular aberrations, expression of cytoskeletal and heat shock genes and secondary structure of functional groups of the hatchlings of Labeo rohita, an important fresh water food fish of India, Nepal, Pakistan, Vietnam, Bangladesh and Myanmar. Although small amount of TCS enters in aquatic ecosystems from domestic and industrial sectors but accidental release of TCS from manufacturing plants or waste water treatment plants cannot be ruled out and it warrants studies in non-target organisms like fish which are at the top of aquatic food chains and an important link in the food chain of man. SCHER et al. (2012) that prediction of the toxic effects of a pollutant based on the results of a single model species may not adequately represent the extremely diverse group Pisces. In addition, there is hardly any report wherein the expression of selected heat shock protein genes and cytoskeletal genes have been studied in one model fish. The current study also holds importance because early life stages display a vast array of morphological, cellular and molecular changes on exposure to even minute concentrations of toxic pollutants (Hallare et al., 2005), and are therefore considered to be effective indicators of aquatic pollution (Osman et al., 2007).
Materials and methods
Test chemical
Triclosan (TCS, cat. No. 72779-25G-F and purity ≥97%) was purchased from Sigma Aldrich, and stock solution of TCS (10 mg/ml) was prepared in acetone.
Experimental fish and treatment
Fertilized eggs/embryos of L. rohita were obtained from the Government Fish Seed Farm, Rajasansi, Amritsar and maintained in 40 L plastic pools containing dechlorinated tap water at 25 ± 1.2°C, under 14:10 h light/dark photoperiod. 3 days post hatching (3 dph), the larvae were exposed for 96 h in 8 L plastic tubs to control, solvent control (acetone), 0.06 (LC0), 0.067 (LC10) and 0.097 (LC30) mg/L TCS (selected according to Sharma et al., 2021a). Exposures were given in pentuplicate (50 hatchlings/tub, 250/exposure group and total 1250 hatchlings). The test water was replaced daily and the hatchlings were fed with boiled egg yolk, 1 h before changing the water. After 96 h, about half of the hatchlings from each treatment group were pooled separately and used for cell viability assay, micronucleus/aberrant cell frequency assay, expression profiling of heat shock and cytoskeletal genes and FT-IR analysis. The other half was kept for observing the reversal or remedial effect of a recovery period of 10 days on the selected parameters.
Cell viability test
It was performed by the protocol of Sharma et al. (2021b). Cell suspension was prepared by homogenizing 10 hatchlings in 500 µl of phosphate buffer saline (7.4) followed by centrifugation at 500 × g for 10 min. 5 μl cell suspension and 25 µl dye mixture (100 µg/ml acridine orange and ethidium bromide) were taken on a slide. Three types of cells i.e., green (viable), red (necrotic) and yellow (apoptotic) were scanned under fluorescent microscope (Nikon ECLIPSE E200), photographed with Nikon D5300 camera. 1500 cells/exposure group (500cells/replicate) were recorded for this assay.
Micronucleus/aberrant cell frequency assay
This assay was performed by the method of Sharma et al. (2021b) with slight modifications. Twenty hatchlings were kept in 1 ml of Carnoy’s fixative (6: 3: 1: ethanol: chloroform: acetic acid) for 1 h, homogenized, centrifuged and finally cell suspension was prepared in 100 µl of acetic acid. The cells were smeared on a clean glass slide, dried properly at room temperature and stained for 30 min with 5% Giemsa. 1500 cells/treatment group and 500 cells/replicate were scanned under microscope (Olympus BX43) at 100X magnification for calculating micronucleated (MN) and aberrant cell (AC) frequency.
Gene expression
The primers used in the present study were designed according to NCBI and EMBL database and are listed in Table 1. The protocols used for RNA isolation, DNase treatment, cDNA preparation and thermocycling conditions were same as in our previous study (Sharma et al., 2021a). EF-1α was used as housekeeping gene for normalizing the expression of target genes. Samples were run in triplicate. Expression of the housekeeping gene showed non-significant variation throughout the experiment. The results of gene expression were assessed by
Fourier transform infrared spectroscopy
Around 50 larvae from each treatment were freeze dried with Heto Power Dry (Allerod, Denmark) at −55°C and powdered with mortar and pestle. FT-IR spectra were recorded from 1 mg of sample with IR-Tracer-100 spectrometer (Shimadzu, Japan) equipped with diamond attenuated total reflectance (ATR) cell. The samples were scanned in triplicate at room temperature in the spectral range 3300–900 cm−1. The variations in band areas were analysed using Origin 8.6 software.
Statistical analysis
One-way ANOVA and student’s t-test were used to find out significance level (p ≤ 0.05 and p ≤ 0.01) between treatments and durations, respectively. Tukey’s HSD post hoc test was performed to obtain differences among the means. The results have been presented as Mean ± SD and all statistical analyses were carried with the help of SPSS 16.0 version.
Results
After 96h, haemorrhage, hypopigmentation, deformed caudal fin, curved trunk, pointed head, lean body, withering of muscles, degeneration of digestive tract, paralysed hatchlings (could not swim or showed erratic swimming) and a small and deflated swim bladder were observed to increase with concentration at 0.067 and 0.097 mg/L (Figure 1). No abnormality was observed in control, solvent control and 0.06 mg/L.
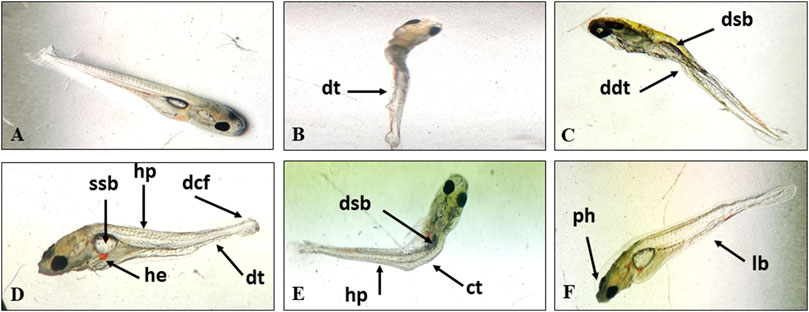
FIGURE 1. Normal larva (A) and TCS induced deformities in the hatchlings of L. rohita, dt, degeneration of tissue; ddt, degenerated digestive tract; dsb, deflated swim bladder; ssb, small swim bladder; hp, hypopigmentation; dcf, deformed caudal fin; he, hemorrhage; ct, curved trunk; ph, pointed head; lb, lean body (B–F).
The frequency of viable cells decreased but that of necrotic and apoptotic cells increased significantly (p ≤ 0.01) in a concentration dependent manner after 96 h exposure and 10 days of the recovery period (Figures 2, 3). Comparison of recovery values with the 96 h values showed a significant increase (p ≤ 0.05) in viable cell frequency but a significant decrease (p ≤ 0.05) in necrotic and apoptotic cell frequency at 0.067 and 0.097 mg/L. However, at 0.06 mg/L, the frequency of viable and apoptotic cells increased non-significantly while the frequency of necrotic cells decreased non-significantly in comparison to their respective 96 h values.
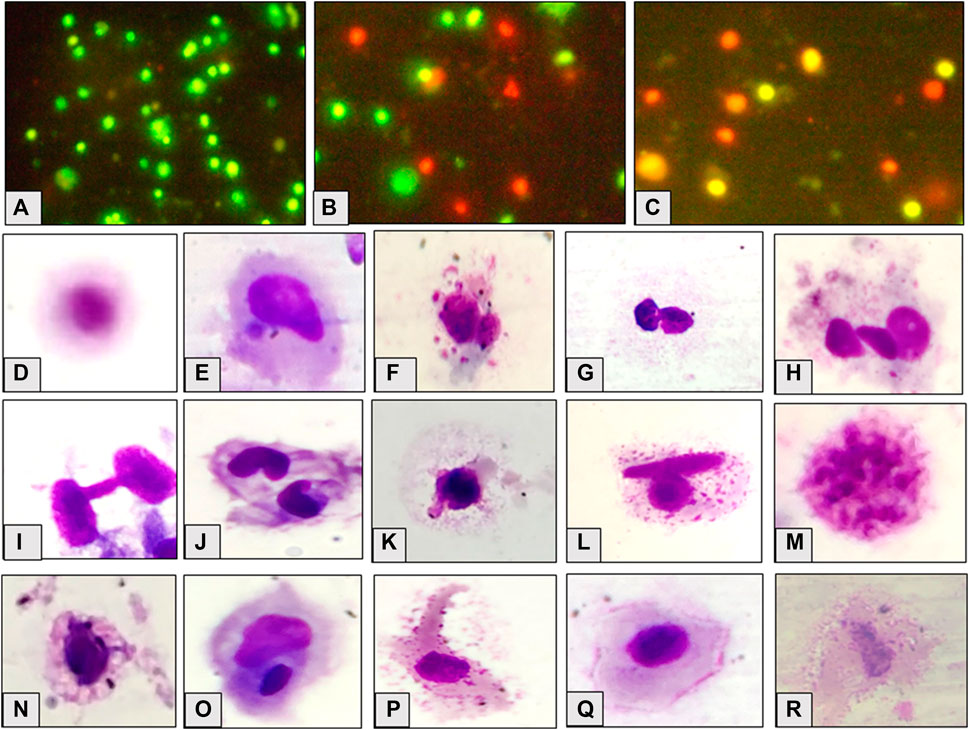
FIGURE 2. Appearance of cells, viable (A), necrotic (B) and apoptotic (C); observed cellular abnormalities, normal cell (D) micronucleated cell (E) multi-micronuclei (F), binucleate (G), trinucleate (H), cytoplasmic bridge (I), notched nuclei (J), nuclear bud (K), binucleate and vesicles (L), nuclear fragmentation (M), vacuolation (N), degmacyte (O), finger like projection (P), membrane folding (Q), necrotic cell (R) in the hatchlings of L. rohita after 96 h exposure to TCS and 10 days of the recovery period.
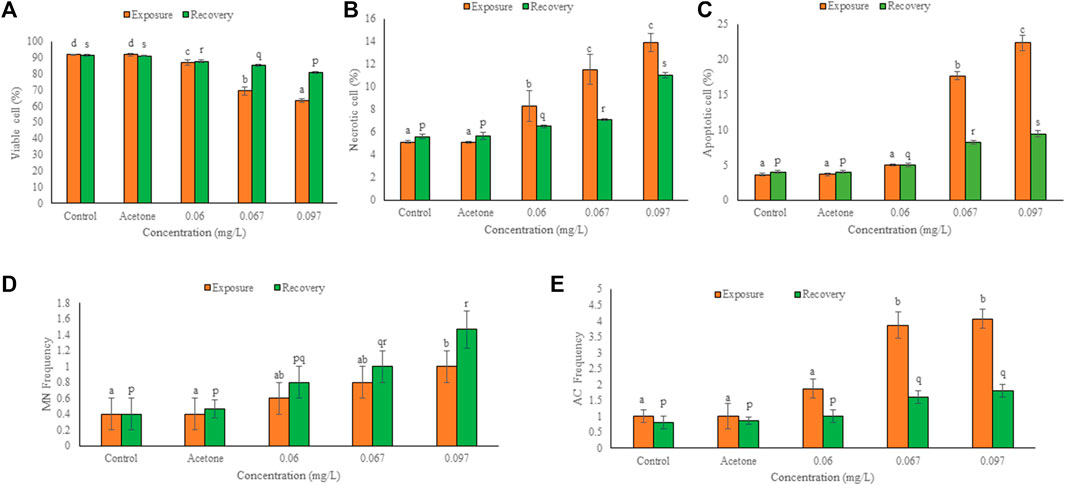
FIGURE 3. Frequency of viable (A), necrotic (B), apoptotic (C), micronucleated (D) and aberrant cells (E) in the hatchlings of L. rohita after 96 h exposure to TCS and 10 days of the recovery period. Values are, mean ± SD, n = 3, different alphabets represent significant (p ≤ 0.01) difference with in the treatments after exposure (a-d) and recovery (p-s).
Figure 2 shows the micronucleated and aberrant cells. The frequency of binucleated cells was maximum after exposure as well as recovery period. Significant (p ≤ 0.05) concentration dependent increase was observed in the frequency of MN and AC after 96 h exposure and 10 days of the recovery period (Figure 3). Respective increase over control in the frequency of MN and ACs at the highest concentration was 1.50 and 3.07-fold after exposure, but 2.67 and 1.25-fold after recovery, respectively. Compared to 96 h values, MN increased non-significantly while ACs decreased significantly (p ≤ 0.01) after 10 days of recovery period.
Significant transcriptomic alterations over control were observed in HSPs and cytoskeletal genes after 96 h exposure and 10 days of the recovery period. In solvent control, non-significant change over control was observed in the expression of selected genes after both the durations. There was a concentration dependent significant (p ≤ 0.01) increase over control in the expression of HSP47, HSP70, and HSc71 but a significant decrease (p ≤ 0.01) in the expression of HSP60 and HSP90 (Figure 4), after exposure. At 0.06, 0.067 and 0.097 mg/L, the respective elevation in gene expression was 53, 70 and 87% for HSP47, 41, 47 and 94% for HSP70, and 17, 26 and 108% for HSc71. However, the respective decline in gene expression was 60, 70 and 85% for HSP60, and 70, 74 and 75% for HSP90. After exposure, minimum and maximum decline in expression was observed for HSP60 at 0.06 and 0.097 mg/L, respectively whereas HSc71 showed minimum and maximum elevation at 0.06 and 0.097 mg/L TCS, respectively. During the recovery period, mRNA copy number of all the heat shock proteins increased significantly (p ≤ 0.01) in a concentration dependent manner. The elevation in expression at 0.06, 0.067 and 0.097 mg/L TCS was 98, 133 and 144% for HSP47, 54, 60 and 110% for HSP70, 11, 13 and 56% for HSc71, 43, 61 and 75% for HSP60, and 151, 125 and 211% for HSP90, respectively. HSc71 (0.06 mg/L) and HSP90 (0.097 mg/L) showed minimum and maximum induction, respectively. In comparison to 96h, HSP60 and HSP90 increased significantly (p ≤ 0.05), HSP47 and HSP70 increased non-significantly, while HSc71 showed non-significant decline after recovery.
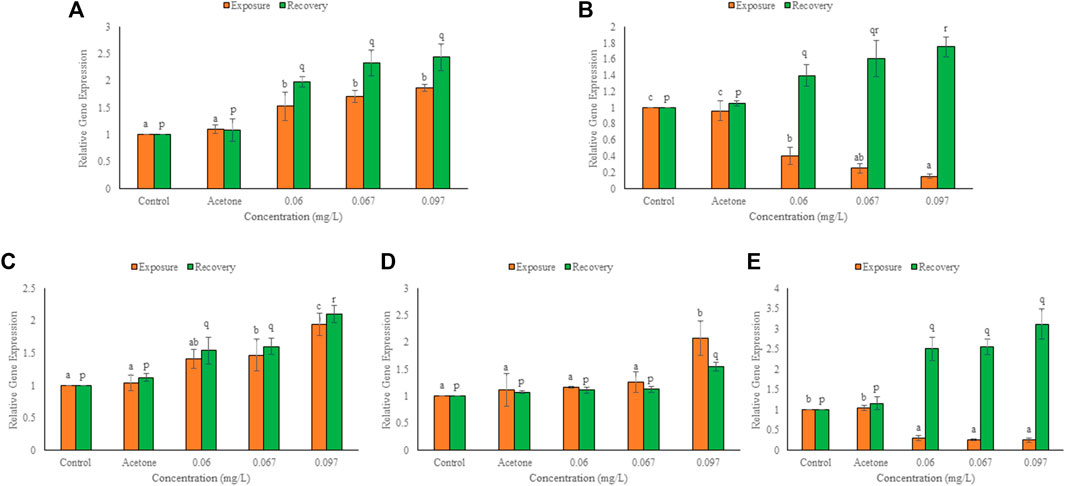
FIGURE 4. Expression of hsp47 (A), hsp60 (B), hsp70 (C), hsc71 (D), hsp90 (E), in the hatchlings of L. rohita after 96 h and 10 days post exposure to TCS. Values are, mean ± SD, n = 3, different alphabets represent significant (p ≤ 0.01) difference within the treatments after 96 h (a-c) and after 10 days of recovery period (p-r).
After 96 h exposure, mRNA copy number increased (p ≤ 0.01) over control for α-tropomyosin but declined (p ≤ 0.01) over control for the other cytoskeletal genes in a concentration dependent manner (Figure 5). At 0.06, 0.067 and 0.097 mg/L TCS, percent change over control was 40, 66 and 73 for DHPR, 26, 31 and 58 for myosin light peptide, 38, 42 and 44 for desmin b, 46, 50 and 66 for laminb1 and 44, 99 and 128 for α-tropomyosin, respectively. Reduction was minimum for myosin light polypeptide (0.06 mg/L) but maximum for DHPR (0.097 mg/L). After 10 days of the recovery period, expression of α-tropomyosin increased (p ≤ 0.01) further, and the expression of other cytoskeletal genes also increased significantly (p ≤ 0.01) in a concentration dependent manner (except for a non-significant increase in desmin b). The induction after recovery at 0.06, 0.067 and 0.097 mg/L TCS, was 206, 228 and 277% for DHPR, 171, 202 and 272% for myosin light peptide, 167, 180 and 266% for α-tropomyosin, 29, 34 and 41% for desmin b and 39, 123 and 134% for lamin b1, respectively. Desmin b showed minimum (0.06 mg/L) while DHPR (0.097 mg/L) showed maximum increase in expression after recovery. Expression of all cytoskeletal genes during recovery was significantly (p ≤ 0.01) higher than the respective 96 h values.
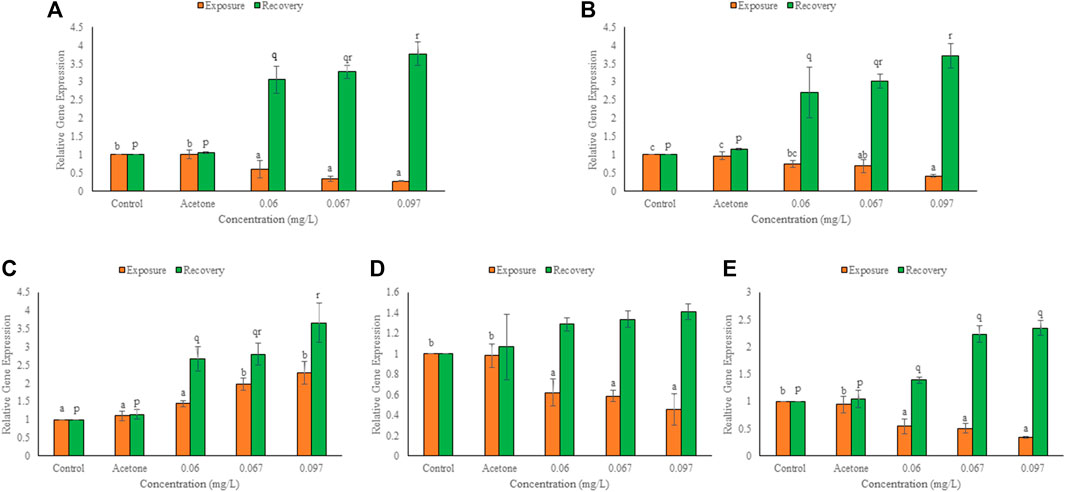
FIGURE 5. Expression of DHPR (A), myosin light polypeptide 3 (B), α-tropomyosin (C), desmin b (D), lamin b1 (E) in the hatchlings of L. rohita after 96 h and 10 days post exposure to TCS. Values are, mean ± SD, n = 3, different alphabets represent significant (p ≤ 0.01) difference within the treatments after 96 h (a-c) and after 10 days of recovery period (p-r).
FT-IR spectra of secondary structure of the biomolecules of hatchlings after 96 h exposure and 10 days of the recovery period have been presented in Figure 6 and Table 2 and the tentative assignment of different peaks is given in Table 3. After 96 h exposure, there was a significant (p ≤ 0.01) concentration dependent decrease in band area (%) at wave no. 1082–1083 and 1398–1401 cm−1 while a significant increase (p ≤ 0.01) was observed at wave no. 1235–1237, 1543–1546, 1651–1653 and 3290–3296 cm−1. Peak no. 1163–1165, 1455–1457, 2852–2855 and 2924–2925 cm−1, on the other hand, showed a non-significant decline in percent band area after exposure. Significant concentration dependent decrease in percent band area (p ≤ 0.01) was observed for all the peaks (except at peak 1163–1165, 1455–1457 and 2924–2925 cm−1), after 10 days of recovery period. At 0.097 mg/L, decline in the band area of wave no. 1082–1083 cm−1 was 66.28% after exposure and 75.67% after recovery. However, maximum increase in band area, after exposure and recovery period was in wave no. 1543–1546 cm−1 (33.45%) and 1455–1457 cm−1 (100.75%), respectively. After recovery, a significant (p ≤ 0.05) difference was observed in the percent band area of all the peaks compared to the respective 96 h values. Area of all the bands (except for a significant (p ≤ 0.05) increase in peaks 1163–1165, 1455–1457 and 2924–2925 cm−1) decreased significantly (p ≤ 0.05) over 96 h values, after recovery.
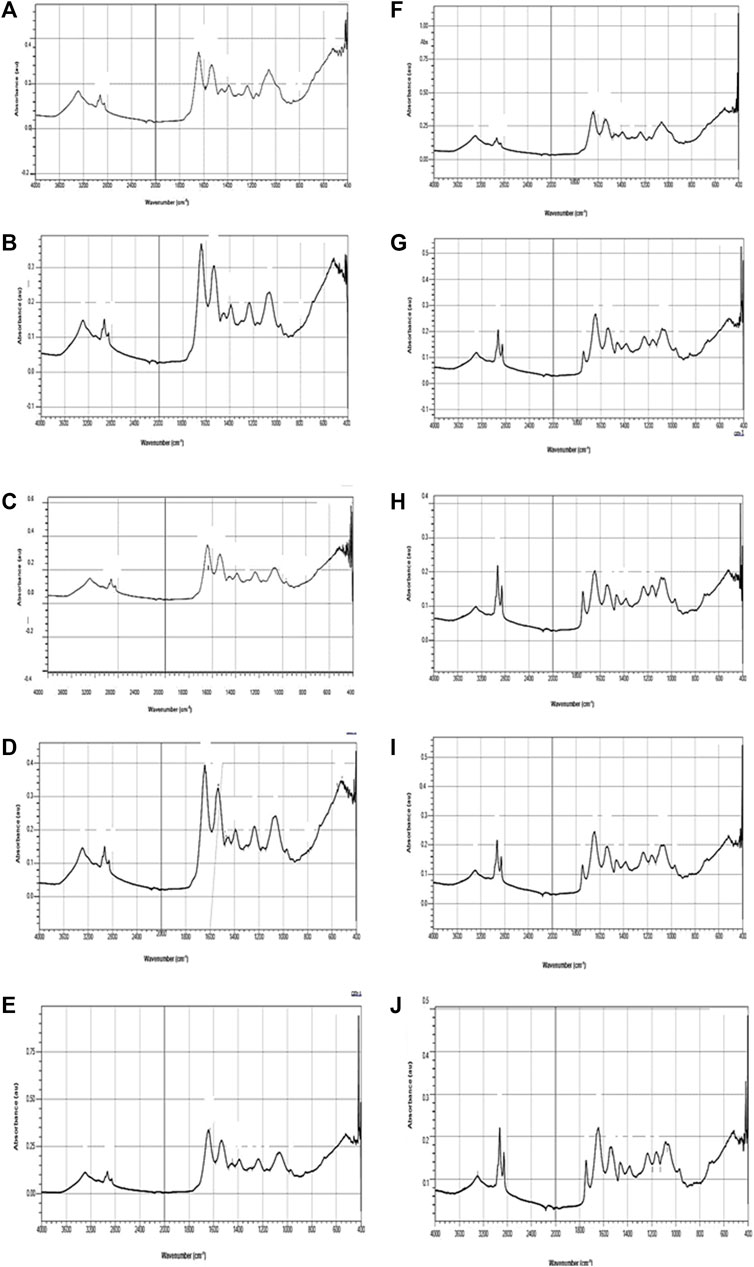
FIGURE 6. FT-IR spectra of L. rohita hatchlings after 96 h exposure (A–E) to TCS and 10 days of the recovery period (F–J). Control (A,F), acetone (B,G), 0.06 mg/L (C,H), 0.067 mg/L (D,I), 0.097 mg/L (E,J).
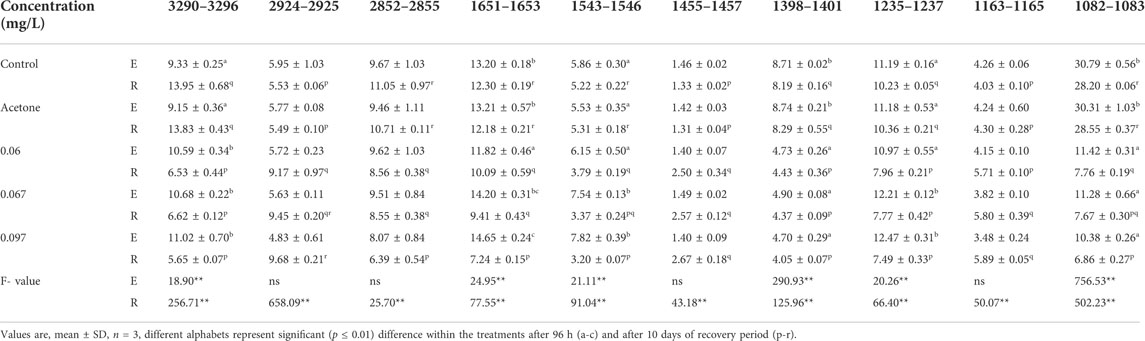
TABLE 2. Variations in the percent band area of L. rohita hatchlings after 96 h (E) and 10 days post exposure to TCS (R).
Discussion
Probable toxicity of a particular pesticide can be easily evaluated through observational studies related to morphological and/or biochemical alterations in fish. Embryos and larvae/early developmental stages of fish are highly sensitive to the effects of even small quantities of xenobiotics and the changes observed in them can be used as important toxicity biomarkers (Dar et al., 2019).
The concentration dependent increase in larval abnormalities in the present study seems mainly due to the high octanol water coefficient (4.8) and organic carbon water partition coefficient (3.8–4.0) of TCS that lead to its bioaccumulation in organisms. This also indicates its potential for biomagnification in the food chain (Zhou et al., 2017). The abnormal development of caudal fin, trunk, head, digestive tract and swim bladder in the larvae of L. rohita can be linked with the observed alterations in mRNA and/or protein expression of cytoskeletal genes under the stress of TCS and gets support from the studies of Fritsch et al. (2013), Falisse et al. (2018) and Ma et al. (2019). Teratogenic effects of TCS have also been reported in zebra fish (Oliveira et al., 2009), Japanese medaka (Nassef et al., 2010) and four carps (Dar et al., 2019).
The concentration dependent decrease in cell viability while increase in the percentage of necrotic and apoptotic cells confirm the cytotoxic potential of TCS and the observation is directly supported by Binelli et al. (2009a, 2009b), Parenti et al. (2019) and Guidony et al. (2020) who observed similar effects of TCS in zebra mussel, zebrafish embryos and zebrafish hepatocytes, respectively. Apoptosis or necrosis is induced by TCS either due to its action as an intercalating agent (through dioxins, the degraded product of TCS) directly or through induction of oxidative stress indirectly (Aranami and Readman, 2007). Schweizer (2001), suggested that TCS inhibits synthesis of biomolecules and activity of membrane bound ATPase, which promote cell lysis and membrane perturbations. TCS has also been reported to induce apoptosis by the activation of caspase 3, fragmentation of DNA and formation of apoptotic bodies (Szychowski et al., 2015). Oxidative stress may also damage the biomolecules and organelles that in turn also activate apoptotic machinery (Redza-Dutordoir and Averill-Bates, 2016). 10–100 µM TCS for 24 h is reported to cause dose dependent cell death in B cell acute lymphoblastic leukemia cell lines by Alfhili et al. (2021). The authors associated the cell death with impaired membrane permeability, cell shrinkage, increased concentration of intracellular Ca2+, redox imbalance, chromatin condensation and fragmentation of DNA. Canesi et al. (2007) observed that TCS at low µM (1–100) range destabilized the lysosomal membrane of Mytilus galloprovincialis hemocytes. Gaume et al. (2012) observed that 2–10 µm TCS induced dose dependent cytotoxicity in marine gastropod Haliotis tuberculate and Ciniglia et al. (2005) reported that TCS decreased cell viability up to 25 and 40% in Closterium ehrenbergii at 0.187 and 0.25 mg/L, respectively. TCS induced necrosis has also been reported in the gill lamellae of L. rohita (Hemalatha et al., 2019) and muscle tissue of Oreochromis mossambicus (Deepika et al., 2021).
The micronucleus assay is the microscopic assessment of genotoxic potential of a xenobiotic in the exposed organisms. Micronucleus is a laggard chromosome or chromosomal fragment formed due to aneugenesis or clastogenesis (Emery, 2012), while binucleated cells are formed due to abnormal cell division or blocked cytokinesis (Kaur and Kaur, 2015; Kaur and Dua, 2016). Clastogenic pollutants like TCS impair DNA replication and normal cell division that may lead to formation of aberrant cells (Sharma and Chadha, 2020). Significant concentration dependent rise in the frequency of MN and ACs in the present study is supported by the findings of Binelli et al. (2009a), Emery (2012), Vijitha et al. (2017), Wang et al. (2018) and Jimoh and Sogbanmu (2021). These workers gave exposure of TCS to Dreissena polymorpha (1, 2 and 3 nM TCS for 96 h), Lithobates catesbeianus (0.0023, 0.023, 0.23 mg/L TCS for 1, 8 and 15 days), Oreochromis niloticus (0.28 and 0.56 mg/L TCS for 96 h), Carassius auratus (0.14, 0.28 and 0.56 mg/L TCS for 14 days) and Clarias gariepinus (0.027 mg/L TCS for 28 days), respectively.
HSPs are intracellular chaperones that remain conserved from bacteria to humans (Lindquist et al., 1988) and are involved in cellular defense during stressful conditions (Sanders, 1993). Due to their role in stability, translocation and folding of proteins (Xing et al., 2015), HSPs can be used as an important biomarker to monitor the effect of environmental stressors on animals including fish (Lewis et al., 1999). These proteins, important for immune function are generally altered during stress (Xing et al., 2013). Results of the present study show that expression of HSP47, HSP70 and HSc71 increased after exposure and recovery period, while the expression of HSP60 and HSP90 declined after exposure but showed reverse trend after recovery. Change in the expression of HSPs is an indicator of TCS induced oxidative stress in the hatchlings of L. rohita. Transcription of HSPs in eukaryotes is regulated by heat shock transcription factor (HSF) and the oxidative stress generated by the pesticides has been reported to directly activate HSF and induce the expression of HSPs (Lee and Corry, 1998). HSP47 is a resident of endoplasmic reticulum and generally acts as a molecular chaperone for folding of collagen fibrils (Pearson, 1996). The elevated expression of HSP47 was consequent with the TCS induced deformities in the head, trunk, fins and gut of the hatchlings in the present study. Elevated expression of HSP47 has been reported in gill and liver of Epinephelus guaza after exposure of polycyclic aromatic hydrocarbons by Abdel-Gawad and Khalil (2013). Heavy metals and hydro soluble organic pollutants have also been observed to elevate the expression of HSP47 in Chinese hamster (Guizani et al., 2012). HSP70 plays multiple roles under stress conditions, such as avoidance of protein aggregation, uncoupling of protein aggregates and suppression of apoptosis (Wiech et al., 2012). So, the increased frequency of necrotic and apoptotic cells in the present study can be related with altered expression of HSP70. This family comprises of both stress inducible (HSP70) and constitutively expressed (HSc70) genes. Xing et al. (2013) observed that HSP70 levels were strongly induced while HSc70 was slightly induced in liver, brain, kidney and gill of Cyprinus carpio under the stress of atrazine and chlorpyrifos individually and their mixture. TCS upregulated the expression of HSP70 in Eisenia fetida (Lin et al., 2014) and Caenorhabditis elegans (García-Espiñeira et al., 2018) also. HSP60 is an important chaperone involved in assembly, stability and folding of proteins and their import into endoplasmic reticulum while HSP90 is necessary for assembly of oligomeric proteins, folding of monomeric proteins and formation of steroid receptor (Cechetto et al., 2000; Roberts et al., 2010; Liu et al., 2013). Decline in HSP60 and HSP90 in our study is corroborated by Eder et al. (2009), who reported that 96 h exposure of 1.2 and 7.3 μg/L chlorpyrifos declined the proteomic expression of both these genes in Oncorhynchus tshawytscha.
Cytoskeletal proteins are involved in various cellular functions such as maintenance of cell shape, transport, locomotion, intracellular organization etc. (Nawaz et al., 2005). These proteins are generally differentially expressed after exposure of xenobiotics and reflect general stress response of organisms (Groh and Suter, 2015). Expression of these genes was observed to be highly dynamic under the stress of TCS by Loh et al. (2000) and Costa et al. (2002), they suggested that TCS disrupted the organization of cytoskeletal proteins. No swimming or erratic swimming of TCS exposed hatchlings in the present study may have been due to the alterations in the expression of cytoskeletal genes that gradually caused paralysis. Fritsch et al. (2013) also observed same behaviour in TCS exposed fathead minnow. We observed that after exposure, mRNA levels of all cytoskeletal genes except for α-tropomyosin declined but a reverse trend was observed after recovery. An increase in the expression of α-tropomyosin has also been reported by Lebreton et al. (2021) in oxazepam exposed gastropod Radix balthica. DHPR plays a key role in the development of trunk musculature (Ma et al., 2019), therefore the observed decline can be linked with the concentration dependent increase in curved trunk, lean body, pointed head and abnormal swimming in the exposed hatchlings in the present study. DHPR is a voltage gated Ca2+ channel and is responsible for intracellular Ca2+ release, its lower expression could have reduced the level of Ca2+ in the cells (Ma et al., 2019). In the absence of Ca2+, tropomyosin blocks interaction of actin with myosin that could have resulted in erratic swimming under the stress of TCS. MLP-3 was observed to be the second most sensitive gene, this is the master regulator of muscle function. Reduced mRNA levels of myosin regulatory light chain A have been reported by Lebreton et al. (2021) in fresh water gastropod, Radix balthica after exposure of 0.8 and 10 μg/L oxazepam for 28 days. Desmin and lamin, the two important cytoskeletal proteins are susceptible to the stress of a variety of xenobiotics (Groh and Suter, 2015). Decline in desmin expression in the hatchlings of the present study may also be related to muscular degeneration and deformed caudal fin as desmin is a component of intermediate filaments in muscles (skeletal, cardiac and smooth) and its expression is correlated with the development of fin muscles during embryogenesis (Loh et al., 2000). Lamin is an important constituent of nuclear lamina which supports nuclear envelope (Peter and Stick, 2012) while lamin b1 maintains the structure and stability of chromosomal territories (Camps et al., 2015). Decline in the expression of lamin b1 seems to be the probable cause of observed concentration dependent nuclear abnormalities in the present study. Along with it, lamin b1 also plays a key role in the development of neurons and decrease in its expression has been linked with impaired neuronal development (Razafsky et al., 2016). Our results are consistent with the study of Falisse et al. (2017), who also observed elevated proteomic expression of α-tropomyosin and decline in myosin light polypeptide 3 and lamin D1 in the larvae of zebrafish after exposure of 50 and 100 μg/L of TCS for 7 days.
Fourier transform infrared spectroscopy is a physical method used to study alterations in functional groups and biomolecules (Cakmak et al., 2006). The non-significant decline in stretching (symmetric and asymmetric) and bending of CH2 after exposure while significant elevation in lipid related peaks (except 2852–2855 cm−1) after recovery indicate impaired lipid metabolism. Significant induction in the band area of amide A, amide I and amide II in TCS exposed larvae suggests that the increased concentration of protein during stress was a general adaptive response for synthesizing important enzymes for detoxification of the xenobiotic and gets support from Bharti and Rasool (2021) who observed a significant decline in protein content of liver and kidney tissue of C. punctatus after exposure to 0.4 mg/L malathion for 4, 8 and 12 days. Significant decrease in the intensity of these peaks after recovery may be linked with either oxidation of proteins under the influence of accumulated ROS or their metabolism to fulfil higher energy demand in the stressed hatchlings. In our previous studies (Sharma et al., 2021a; 2021b), we observed a significant decline in protein content in the TCS exposed hatchlings and this directly supports the present observations. Similarly, altered intensity of amide A, amide I and amide II has also been reported in the TCS exposed embryos/larvae of four food fishes (Dar et al., 2022) and in liver, muscle, brain and kidney of arsenic exposed fingerlings of L. rohita (Palaniappan and Vijayasundaram, 2008a; Palaniappan and Vijayasundaram, 2008b; Palaniappan and Vijayasundaram, 2009a; Palaniappan and Vijayasundaram, 2009b). Increased area of the band at 1235–1237 cm−1 (phospholipids and amino acids) after exposure and its decline after recovery while decline in the band area at 1398–1401 cm−1 till the end of recovery clearly suggests that the fluctuations in the contents of fatty acids and amino acids in the hatchlings could have been for maintaining homeostasis or repair of wear and tear during stress. These results are supported by the TCS induced lipid peroxidation and proteolysis in carps by Dar et al. (2020a); Dar et al. (2020b). Non-significant decline in the intensity of CO-O-C symmetric stretching (1163–1165 cm−1) after exposure and significant induction after recovery suggests that TCS affected metabolic performance and energy levels in the larvae by altering the level of glycogen and disruption of carbohydrate metabolism and is corroborated by Velmurugan et al. (2018). The band at 1082–1083 cm−1 specifies asymmetric stretching of phosphodiester group of nucleic acids. Significant decline in the area of this band indicates low contents of nucleic acids (DNA and RNA) in the hatchlings and is also indicative of the genotoxic potential of TCS (Hemalatha et al., 2019; Gyimah et al., 2020; Sharma et al., 2021a).
Conclusion
The increasing global use of insecticides and pesticides raises concern due to their persistence in the environment and several negative impacts on humans and other non-target terrestrial and aquatic organisms. Significant alterations in the secondary structure of biomolecules and the expression of heat shock and cytoskeletal genes seem to be the underlying cause for an increase in morphological abnormalities along with a significant decline in the viable cell frequency but an increase in the frequencies of necrotic, apoptotic, micronucleated and aberrant cells in the TCS exposed hatchlings. The results highlight that out of the selected genes, DHPR (cytoskeletal genes) and HSP90 (heat shock genes) may be used as the highly sensitive markers for the acute stress of TCS.
Data availability statement
The raw data supporting the conclusions of this article will be made available by the authors, without undue reservation. The above statement is replaced with All data generated during this study are included in the manuscript.
Ethics statement
Ethical review and approval was not required for the animal study because embryos of food fishes do not come under the purview of animal ethics in India.
Author contributions
SS: Conceptualization, experimentation and writing the original draft. OD, MA, SS, and AB: Experimentation and writing. ST and AK: RT-PCR study. AK: conceptualization, supervision, writing, review and editing.
Funding
Financial assistance received from Council of Scientific and Industrial research (CSIR), India vide Grant No. 09/254(0272)-2017-EMR-1 and University Grants Commission Special Assistance Programme (UGC-SAP) vide Grant No. F. 4-4/2016/DRS-1 (SAP II) are duly acknowledged.
Conflict of interest
The authors declare that the research was conducted in the absence of any commercial or financial relationships that could be construed as a potential conflict of interest.
Publisher’s note
All claims expressed in this article are solely those of the authors and do not necessarily represent those of their affiliated organizations, or those of the publisher, the editors and the reviewers. Any product that may be evaluated in this article, or claim that may be made by its manufacturer, is not guaranteed or endorsed by the publisher.
References
Abdel-Gawad, F. K., and Khalil, W. K. B. (2013). Modulation of stress related protein genes in the bass (Epinephelus guaza) caught from the Gulf of Suez, the Red Sea, Egypt. Ecotoxicol. Environ. Saf. 96, 175–181. doi:10.1016/j.ecoenv.2013.05.032
Ahn, K. C., Zhao, B., Chen, J., Cherednichenko, G., Sanmarti, E., Denison, M. S., et al. (2008). In vitro biologic activities of the antimicrobials triclocarban, its analogs, and triclosan in bioassay screens: Receptor-based bioassay screens. Environ. Health Perspect. 116, 1203–1210. doi:10.1289/ehp.11200
Alfhili, M. A., Hussein, H. A. M., Park, Y., Lee, M. H., and Akula, S. M. (2021). Triclosan induces apoptosis in Burkitt lymphoma-derived BJAB cells through caspase and JNK/MAPK pathways. Apoptosis 26, 96–110. doi:10.1007/s10495-020-01650-0
Aranami, K., and Readman, J. W. (2007). Photolytic degradation of triclosan in freshwater and seawater. Chemosphere 66, 1052–1056. doi:10.1016/j.chemosphere.2006.07.010
Bharti, S., and Rasool, F. (2021). Analysis of the biochemical and histopathological impact of a mild dose of commercial malathion on Channa punctatus (Bloch) fish. Toxicol. Rep. 8, 443–455. doi:10.1016/j.toxrep.2021.02.018
Binelli, A., Cogni, D., Parolini, M., Riva, C., and Provini, A. (2009a). Cytotoxic and genotoxic effects of in vitro exposure to Triclosan and Trimethoprim on zebra mussel (Dreissena polymorpha) hemocytes. Comp. Biochem. Physiology Part C Toxicol. Pharmacol. 150, 50–56. doi:10.1016/j.cbpc.2009.02.005
Binelli, A., Cogni, D., Parolini, M., Riva, C., and Provini, A. (2009b). In vivo experiments for the evaluation of genotoxic and cytotoxic effects of Triclosan in Zebra mussel hemocytes. Aquat. Toxicol. 91, 238–244. doi:10.1016/j.aquatox.2008.11.008
Cakmak, G., Togan, I., and Severcan, F. (2006). 17β-Estradiol induced compositional, structural and functional changes in rainbow trout liver, revealed by FT-IR spectroscopy: a comparative study with nonylphenol. Aquat. Toxicol. 77, 53–63. doi:10.1016/j.aquatox.2005.10.015
Camps, J., Erdos, M. R., and Ried, T. (2015). The role of lamin B1 for the maintenance of nuclear structure and function. Nucleus 6, 8–14. doi:10.1080/19491034.2014.1003510
Canesi, L., Ciacci, C., Lorusso, L. C., Betti, M., Gallo, G., Pojana, G., et al. (2007). Effects of Triclosan on Mytilus galloprovincialis hemocyte function and digestive gland enzyme activities: Possible modes of action on non target organisms. Comp. Biochem. Physiology Part C Toxicol. Pharmacol. 145, 464–472. doi:10.1016/j.cbpc.2007.02.002
Capkin, E., Ozcelep, T., Kayis, S., and Altinok, I. (2017). Antimicrobial agents, triclosan, chloroxylenol, methylisothiazolinone and borax, used in cleaning had genotoxic and histopathologic effects on rainbow trout. Chemosphere 182, 720–729. doi:10.1016/j.chemosphere.2017.05.093
Cechetto, J. D., Soltys, B. J., and Gupta, R. S. (2000). Localization of mitochondrial 60-kD heat shock chaperonin protein (Hsp60) in pituitary growth hormone secretory granules and pancreatic zymogen granules. J. Histochem. Cytochem. 48, 45–56. doi:10.1177/002215540004800105
Cherednichenko, G., Zhang, R., Bannister, R. A., Timofeyev, V., Li, N., Fritsch, E. B., et al. (2012). Triclosan impairs excitation-contraction coupling and Ca2+ dynamics in striated muscle. Proc. Natl. Acad. Sci. U. S. A. 109, 14158–14163. doi:10.1073/pnas.1211314109
Ciniglia, C., Cascone, C., Lo Giudice, R., Pinto, G., and Pollio, A. (2005). Application of methods for assessing the geno- and cytotoxicity of Triclosan to C. ehrenbergii. J. Hazard. Mater. 122, 227–232. doi:10.1016/j.jhazmat.2005.03.002
Costa, M. L., Escaleira, R. C., Rodrigues, V. B., Manasfi, M., and Mermelstein, C. S. (2002). Some distinctive features of zebrafish myogenesis based on unexpected distributions of the muscle cytoskeletal proteins actin, myosin, desmin, α-actinin, troponin and titin. Mech. Dev. 116, 95–104. doi:10.1016/S0925-4773(02)00149-1
Dann, A. B., and Hontela, A. (2011). Triclosan: Environmental exposure, toxicity and mechanisms of action. J. Appl. Toxicol. 31, 285–311. doi:10.1002/jat.1660
Dar, O. I., Sharma, S., Singh, K., and Kaur, A. (2019). Teratogenicity and accumulation of triclosan in the early life stages of four food fish during the bioassay. Ecotoxicol. Environ. Saf. 176, 346–354. doi:10.1016/j.ecoenv.2019.03.102
Dar, O. I., Sharma, S., Singh, K., Sharma, A., Bhardwaj, R., and Kaur, A. (2020a). Biochemical markers for prolongation of the acute stress of triclosan in the early life stages of four food fishes. Chemosphere 247, 125914. doi:10.1016/j.chemosphere.2020.125914
Dar, O. I., Sharma, S., Singh, K., Sharma, A., Bhardwaj, R., and Kaur, A. (2020b). Biomarkers for the toxicity of sublethal concentrations of triclosan to the early life stages of carps. Sci. Rep. 10, 17322–17416. doi:10.1038/s41598-020-73042-y
Dar, O. I., Aslam, R., Sharma, S., Jia, A.-Q., Kaur, A., and Faggio, C. (2022). Biomolecular alterations in the early life stages of four food fish following acute exposure of Triclosan. Environ. Toxicol. Pharmacol. 91, 103820. doi:10.1016/j.etap.2022.103820
Deepika, S., Padmavathy, P., Srinivasan, A., Sugumar, G., and Jawahar, P. (2021). Effect of triclosan (TCS) on the protein content and associated histological changes on tilapia, Oreochromis mossambicus (Peters, 1852). Environ. Sci. Pollut. Res. 28, 59899–59907. doi:10.1007/s11356-021-14990-4
Dhillon, G. S., Kaur, S., Pulicharla, R., Brar, S. K., Cledón, M., Verma, M., et al. (2015). Triclosan: Current status, occurrence, environmental risks and bioaccumulation potential. Int. J. Environ. Res. Public Health 12, 5657–5684. doi:10.3390/ijerph120505657
Eder, K. J., Leutenegger, C. M., Köhler, H. R., and Werner, I. (2009). Effects of neurotoxic insecticides on heat-shock proteins and cytokine transcription in Chinook salmon (Oncorhynchus tshawytscha). Ecotoxicol. Environ. Saf. 72, 182–190. doi:10.1016/j.ecoenv.2008.04.020
Emery, D. (2012). Assessing the genotoxicity of triclosan in tadpoles of the American bullfrog, Lithobates catesbeianus. Virginia: Virginia Commonwealth University Richmond.
Falisse, E., Voisin, A.-S., and Silvestre, F. (2017). Impacts of triclosan exposure on zebrafish early-life stage: Toxicity and acclimation mechanisms. Aquat. Toxicol. 189, 97–107. doi:10.1016/j.aquatox.2017.06.003
Falisse, E., Ducos, B., Stockwell, P. A., Morison, I. M., Chatterjee, A., and Silvestre, F. (2018). DNA methylation and gene expression alterations in zebrafish early-life stages exposed to the antibacterial agent triclosan. Environ. Pollut. 243, 1867–1877. doi:10.1016/j.envpol.2018.10.004
Fritsch, E. B., Connon, R. E., Werner, I., Davies, R. E., Beggel, S., Feng, W., et al. (2013). Triclosan impairs swimming behavior and alters expression of excitation-contraction coupling proteins in fathead minnow (Pimephales promelas). Environ. Sci. Technol. 47, 2008–2017. doi:10.1021/es303790b
García-Espiñeira, M. C., Tejeda-Benítez, L. P., and Olivero-Verbel, J. (2018). Toxic effects of bisphenol A, propyl paraben, and triclosan on Caenorhabditis elegans. Int. J. Environ. Res. Public Health 15 (4), 684. doi:10.3390/ijerph15040684
Gaume, B., Bourgougnon, N., Auzoux-Bordenave, S., Roig, B., Le Bot, B., and Bedoux, G. (2012). In vitro effects of triclosan and methyl-triclosan on the marine gastropod Haliotis tuberculata. Comp. Biochem. Physiology Part C Toxicol. Pharmacol. 156, 87–94. doi:10.1016/j.cbpc.2012.04.006
Groh, K. J., and Suter, M. J. F. (2015). Stressor-induced proteome alterations in zebrafish: A meta-analysis of response patterns. Aquat. Toxicol. 159, 1–12. doi:10.1016/j.aquatox.2014.11.013
Guidony, N. S., Luís, J., Scaini, R., Bandeira, M. W., Machado, K. S., Bastos, C., et al. (2020). ABC proteins activity and cytotoxicity in zebrafish hepatocytes exposed to triclosan. Environ. Pollut. 271, 116368. doi:10.1016/j.envpol.2020.116368
Guizani, M., Nogoshi, Y., Ben Fredj, F., Han, J., Isoda, H., and Funamizu, N. (2012). Heat shock protein 47 stress responses in Chinese hamster ovary cells exposed to raw and reclaimed wastewater. J. Environ. Monit. 14, 492–498. doi:10.1039/c1em10519a
Gyimah, E., Dong, X., Qiu, W., Zhang, Z., and Xu, H. (2020). Sublethal concentrations of triclosan elicited oxidative stress, DNA damage, and histological alterations in the liver and brain of adult zebrafish. Environ. Sci. Pollut. Res. 27, 17329–17338. doi:10.1007/s11356-020-08232-2
Hallare, A. V., Schirling, M., Luckenbach, T., Köhler, H. R., and Triebskorn, R. (2005). Combined effects of temperature and cadmium on developmental parameters and biomarker responses in zebrafish (Danio rerio) embryos. J. Therm. Biol. 30, 7–17. doi:10.1016/j.jtherbio.2004.06.002
Han, J., Won, E. J., Hwang, U. K., Kim, I. C., Yim, J. H., and Lee, J. S. (2016). Triclosan (TCS) and Triclocarban (TCC) cause lifespan reduction and reproductive impairment through oxidative stress-mediated expression of the defensome in the monogonont rotifer (Brachionus koreanus). Comp. Biochem. Physiology Part C Toxicol. Pharmacol. 186, 131–137. doi:10.1016/j.cbpc.2016.04.002
Hemalatha, D., Nataraj, B., Rangasamy, B., Shobana, C., and Ramesh, M. (2019). DNA damage and physiological responses in an Indian major carp Labeo rohita exposed to an antimicrobial agent triclosan. Fish. Physiol. Biochem. 45, 1463–1484. doi:10.1007/s10695-019-00661-2
Iwama, G. K., Vijayan, M. M., Forsyth, R. B., and Ackerman, P. A. (1999). Heat shock proteins and physiological stress in fish. Am. Zool. 39, 901–909. doi:10.1093/icb/39.6.901
Jimoh, R. O., and Sogbanmu, T. O. (2021). Sublethal and environmentally relevant concentrations of triclosan and triclocarban induce histological, genotoxic, and embryotoxic effects in Clarias gariepinus (Burchell, 1822). Environ. Sci. Pollut. Res. Int. 28, 31071–31083. doi:10.1007/s11356-021-12820-1
Kaur, R., and Dua, A. (2016). Fish liver and gill cells as cytogenotoxic indicators in assessment of water quality. Environ. Sci. Pollut. Res. 23, 18892–18900. doi:10.1007/s11356-016-7080-8
Kaur, K., and Kaur, A. (2015). Fish erythrocytes as biomarkers for the toxicity of sublethal doses of an azo dye, basic violet-1 (CI: 42535). Microsc. Microanal. 21, 264–273. doi:10.1017/S1431927614013609
Kim, J., Oh, H., Ryu, B., Kim, U., Lee, J. M., Jung, C. R., et al. (2018). Triclosan affects axon formation in the neural development stages of zebrafish embryos (Danio rerio). Environ. Pollut. 236, 304–312. doi:10.1016/j.envpol.2017.12.110
Lebreton, M., Sire, S., Carayon, J. L., Malgouyres, J. M., Vignet, C., Géret, F., et al. (2021). Low concentrations of oxazepam induce feeding and molecular changes in Radix balthica juveniles. Aquat. Toxicol. 230, 105694. doi:10.1016/j.aquatox.2020.105694
Lee, Y. J., and Corry, P. M. (1998). Metabolic oxidative stress-induced HSP70 gene expression is mediated through SAPK pathway: Role of Bcl-2 and c-Jun NH2-terminal kinase. J. Biol. Chem. 273, 29857–29863. doi:10.1074/jbc.273.45.29857
Lewis, S., Handy, R. D., Cordi, B., Billinghurst, Z., and Depledge, M. H. (1999). Stress proteins (HSP’s): Methods of detection and their use as an environmental biomarker. Ecotoxicology 8, 351–368. doi:10.1023/A:1008982421299
Lin, D., Li, Y., Zhou, Q., Xu, Y., and Wang, D. (2014). Effect of triclosan on reproduction, DNA damage and heat shock protein gene expression of the earthworm Eisenia fetida. Ecotoxicology 23, 1826–1832. doi:10.1007/s10646-014-1320-9
Lindquist, S., Biology, C., and Craig, E. A. (1988). The heat -shock proteins. Annu. Rev. Genet. 22, 631–677. doi:10.1146/annurev.ge.22.120188.003215
Liu, T., Zhang, Z., Chen, D., Wang, L., Yao, H., Zhao, F., et al. (2013). Effect of atrazine and chlorpyrifos exposure on heat shock protein response in the brain of common carp (Cyprinus carpio L.). Pestic. Biochem. Physiol. 107, 277–283. doi:10.1016/j.pestbp.2013.09.002
Livak, K. J., and Schmittgen, T. D. (2001). Analysis of relative gene expression data using real-time quantitative PCR and the 2-ΔΔCT method. Methods 25, 402–408. doi:10.1006/meth.2001.1262
Loh, S. H., Chan, W. T., Gong, Z., Lim, T. M., and Chua, K. L. (2000). Characterization of a zebrafish (Danio rerio) desmin cDNA: An early molecular marker of myogenesis. Differentiation 65, 247–254. doi:10.1046/j.1432-0436.2000.6550247.x
Lu, J., and Guo, J. (2021). Disinfection spreads antimicrobial resistance. Science 371, 474. doi:10.1126/science.abg4380
Lu, J., Wang, Y., Li, J., Mao, L., Hoang, S., Duarte, T., et al. (2018). Triclosan at environmentally relevant concentrations promotes horizontal transfer of multidrug resistance genes within and across bacterial genera. Environ. Int. 121, 1217–1226. doi:10.1016/j.envint.2018.10.040
Ma, Z., Liu, H., and Yu, H. (2019). Triclosan affects Ca2+ regulatory module and musculature development in skeletal myocyte during early life stages of zebrafish (Danio rerio). Environ. Sci. Technol. 53, 11988–11998. doi:10.1021/acs.est.9b03231
Nag, S. K., Das Sarkar, S., and Manna, S. K. (2018). Triclosan–an antibacterial compound in water, sediment and fish of River Gomti, India. Int. J. Environ. Health Res. 28, 461–470. doi:10.1080/09603123.2018.1487044
Nassef, M., Kim, S. G., Seki, M., Kang, I. J., Hano, T., Shimasaki, Y., et al. (2010). In ovo nanoinjection of triclosan, diclofenac and carbamazepine affects embryonic development of medaka fish (Oryzias latipes). Chemosphere 79, 966–973. doi:10.1016/j.chemosphere.2010.02.002
Nawaz, M., Manzl, C., and Krumschnabel, G. (2005). In vitro toxicity of copper, cadmium, and chromium to isolated hepatocytes from carp, Cyprinus carpio L. Bull. Environ. Contam. Toxicol. 75, 652–661. doi:10.1007/s00128-005-0802-0
Oliveira, R., Domingues, I., Grisolia, C. K., and Soares, A. M. V. M. (2009). Effects of triclosan on zebrafish early-life stages and adults. Environ. Sci. Pollut. Res. 16, 679–688. doi:10.1007/s11356-009-0119-3
Osman, A. G. M., Wuertz, S., Mekkawy, I. A., Exner, H.-J., and Kirschbaum, F. (2007). Lead induced malformations in embryos of the african catfish Clarias gariepinus (burchell, 1822). Environ. Toxicol. 22, 375–389. doi:10.1002/tox.20272
Palaniappan, P. L. R. M., and Vijayasundaram, V. (2008a). FTIR study of arsenic induced biochemical changes on the liver tissues of fresh water fingerlings Labeo rohita. Rom. J. Biophys. 18, 135–144.
Palaniappan, P. L. R. M., and Vijayasundaram, V. (2008b). Fourier transform infrared study of protein secondary structural changes in the muscle of Labeo rohita due to arsenic intoxication. Food Chem. Toxicol. 46, 3534–3539. doi:10.1016/j.fct.2008.09.001
Palaniappan, P. L. R. M., and Vijayasundaram, V. (2009a). The FT-IR study of the brain tissue of Labeo rohita due to arsenic intoxication. Microchem. J. 91, 118–124. doi:10.1016/j.microc.2008.08.014
Palaniappan, P. L. R. M., and Vijayasundaram, V. (2009b). Arsenic-induced biochemical changes in Labeo rohita kidney: An FTIR study. Spectrosc. Lett. 42, 213–218. doi:10.1080/00387010902893033
Parenti, C. C., Ghilardi, A., Della Torre, C., Mandelli, M., Magni, S., Del Giacco, L., et al. (2019). Environmental concentrations of triclosan activate cellular defence mechanism and generate cytotoxicity on zebrafish (Danio rerio) embryos. Sci. Total Environ. 650, 1752–1758. doi:10.1016/j.scitotenv.2018.09.283
Paul, T., Shukla, S. P., Kumar, K., Poojary, N., and Kumar, S. (2019). Effect of temperature on triclosan toxicity in Pangasianodon hypophthalmus (Sauvage, 1878): Hematology, biochemistry and genotoxicity evaluation. Sci. Total Environ. 668, 104–114. doi:10.1016/j.scitotenv.2019.02.443
Paul, T., Kumar, S., Shukla, S. P., Pal, P., Kumar, K., Poojary, N., et al. (2020). A multi-biomarker approach using integrated biomarker response to assess the effect of pH on triclosan toxicity in Pangasianodon hypophthalmus (Sauvage, 1878). Environ. Pollut. 260, 114001. doi:10.1016/j.envpol.2020.114001
Pearson, D. S., Kulyk, W. M., Kelly, G. M., and Krone, P. H. (1996). Cloning and characterization of a cDNA encoding the collagen-binding stress protein hsp47 in zebrafish. DNA Cell Biol. 15, 263–272. doi:10.1089/dna.1996.15.263
Pessah, I. N., Cherednichenko, G., and Lein, P. J. (2010). Minding the calcium store: Ryanodine receptor activation as a convergent mechanism of PCB toxicity. Pharmacol. Ther. 125, 260–285. doi:10.1016/j.pharmthera.2009.10.009
Peter, A., and Stick, R. (2012). Evolution of the lamin protein family: What introns can tell. Nucleus 3, 44–59. doi:10.4161/nucl.18927
Pijanowska, J., and Kloc, M. (2004). Daphnia response to predation threat involves heat-shock proteins and the actin and tubulin cytoskeleton. Genesis 38, 81–86. doi:10.1002/gene.20000
Ponomarenko, M., Stepanenko, I., and Kolchanov, N. (2013). “Heat shock proteins,” in Brenner’s encyclopedia of genetics. Second Edition (Elsevier). doi:10.1016/B978-0-12-374984-0.00685-9
Razafsky, D., Ward, C., Potter, C., Zhu, W., Xue, Y., Kefalov, V. J., et al. (2016). Lamin B1 and lamin B2 are long-lived proteins with distinct functions in retinal development. Mol. Biol. Cell 27, 1928–1937. doi:10.1091/mbc.E16-03-0143
Redza-Dutordoir, M., and Averill-Bates, D. A. (2016). Activation of apoptosis signalling pathways by reactive oxygen species. Biochim. Biophys. Acta - Mol. Cell Res. 1863, 2977–2992. doi:10.1016/j.bbamcr.2016.09.012
Riva, C., Cristoni, S., and Binelli, A. (2012). Effects of triclosan in the freshwater mussel Dreissena polymorpha: A proteomic investigation. Aquat. Toxicol. 118–119, 62–71. doi:10.1016/j.aquatox.2012.03.013
Roberts, R. J., Agius, C., Saliba, C., Bossier, P., and Sung, Y. Y. (2010). Heat shock proteins (chaperones) in fish and shellfish and their potential role in relation to fish health: A review. J. Fish. Dis. 33, 789–801. doi:10.1111/j.1365-2761.2010.01183.x
Sanders, B. M. (1993). Stress proteins in aquatic organisms: An environmental perspective. Crit. Rev. Toxicol. 23, 49–75. doi:10.3109/10408449309104074
Sarkar, S. D., Nag, S. K., Kumari, K., Saha, K., Bandyopadhyay, S., Aftabuddin, M., et al. (2020). Occurrence and safety evaluation of antimicrobial compounds triclosan and triclocarban in water and fishes of the multitrophic niche of river Torsa , India. Arch. Environ. Contam. Toxicol. 79, 488–499. doi:10.1007/s00244-020-00785-0
SCHERSCCSSCENIHR (2012). Opinion on the toxicity and assessment of chemical mixtures. Available at: http://ec.europa.eu/health/scientific_committees/environmental_risks/members _wg/index_en.htm (Accessed July 28, 2022).
Schweizer, H. P. (2001). Triclosan: A widely used biocide and its link to antibiotics. FEMS Microbiol. Lett. 202, 1–7. doi:10.1111/j.1574-6968.2001.tb10772.x
Sharma, M., and Chadha, P. (2020). Genotoxicity induction to recovery in different organs in fish channa punctatus after sub chronic exposure to 4- nonylphenol. Toxicol. Int. 27, 34–43. doi:10.18311/ti/2020/v27i1&2/25234
Sharma, S., Dar, O. I., Singh, K., Thakur, S., Kesavan, A. K., and Kaur, A. (2021a). Genomic markers for the biological responses of Triclosan stressed hatchlings of Labeo rohita. Environ. Sci. Pollut. Res. 28, 67370–67384. doi:10.1007/s11356-021-15109-5
Sharma, S., Dar, O. I., Andotra, M., Sharma, S., Kaur, A., and Faggio, C. (2021b). Environmentally relevant concentrations of triclosan induce cyto-genotoxicity and biochemical alterations in the hatchlings of Labeo rohita. Appl. Sci. (Basel). 11, 10478. doi:10.3390/app112110478
Szychowski, K. A., Sitarz, A. M., and Wojtowicz, A. K. (2015). Triclosan induces Fas receptor-dependent apoptosis in mouse neocortical neurons in vitro. Neuroscience 284, 192–201. doi:10.1016/j.neuroscience.2014.10.001
Velmurugan, B., Senthilkumaar, P., and Karthikeyan, S. (2018). Toxicity impact of fenvalerate on the gill tissue of Oreochromis mossambicus with respect to biochemical changes utilizing FTIR and principal component analysis. J. Biol. Phys. 44, 301–315. doi:10.1007/s10867-018-9484-9
Vijitha, C. K., Asifa, K, P., and Chitra, K, C. (2017). Assessment of genotoxic and haematological consequence of triclosan in the fish , Oreochromis niloticus (Linnaeus, 1758). Int. J. Appl. Res. 3, 101–109.
Wang, C. F., and Tian, Y. (2015). Reproductive endocrine-disrupting effects of triclosan: Population exposure, present evidence and potential mechanisms. Environ. Pollut. 206, 195–201. doi:10.1016/j.envpol.2015.07.001
Wang, F., Xu, R., Zheng, F., and Liu, H. (2018). Effects of triclosan on acute toxicity, genetic toxicity and oxidative stress in goldfish (Carassius auratus). Exp. Anim. 67, 219–227. doi:10.1538/expanim.17-0101
Wayman, G. A., Bose, D. D., Yang, D., Lesiak, A., Bruun, D., Impey, S., et al. (2012). PCB-95 modulates the calcium-dependent signaling pathway responsible for activity-dependent dendritic growth. Environ. Health Perspect. 120, 1003–1009. doi:10.1289/ehp.1104833
Wiech, M., Olszewski, M. B., Tracz-Gaszewska, Z., Wawrzynow, B., Zylicz, M., and Zylicz, A. (2012). Molecular mechanism of mutant p53 stabilization: The role of HSP70 and MDM2. PLoS One 7, e51426. doi:10.1371/journal.pone.0051426
Xing, H., Li, S., Wang, X., Gao, X., Xu, S., and Wang, X. (2013). Effects of atrazine and chlorpyrifos on the mRNA levels of HSP70 and HSC70 in the liver, brain, kidney and gill of common carp (Cyprinus carpio L). Chemosphere 90, 910–916. doi:10.1016/j.chemosphere.2012.06.028
Xing, H., Wang, Z., Wu, H., Zhao, X., Liu, T., Li, S., et al. (2015). Assessment of pesticide residues and gene expression in common carp exposed to atrazine and chlorpyrifos: Health risk assessments. Ecotoxicol. Environ. Saf. 113, 491–498. doi:10.1016/j.ecoenv.2014.12.040
Yao, L., Zhao, J. L., Liu, Y. S., Zhang, Q. Q., Jiang, Y. X., Liu, S., et al. (2018). Personal care products in wild fish in two main Chinese rivers: Bioaccumulation potential and human health risks. Sci. Total Environ. 621, 1093–1102. doi:10.1016/j.scitotenv.2017.10.117
Keywords: abnormalities, cyto-genotoxicity, gene expression, hatchlings, Triclosan
Citation: Sharma S, Dar OI, Andotra M, Sharma S, Bhagat A, Thakur S, Kesavan AK and Kaur A (2022) Cellular, molecular and genomic alterations in the hatchlings of Labeo rohita after exposure to Triclosan. Front. Environ. Sci. 10:992435. doi: 10.3389/fenvs.2022.992435
Received: 12 July 2022; Accepted: 12 August 2022;
Published: 12 September 2022.
Edited by:
Pedro Neves Carvalho, Aarhus University, DenmarkReviewed by:
Swarndeep Hundal, Punjab Agricultural University, IndiaJuyoung Katherine Shim, University of Maine at Augusta, United States
Jaigopal Sharma, Delhi Technological University, India
Copyright © 2022 Sharma, Dar, Andotra, Sharma, Bhagat, Thakur, Kesavan and Kaur. This is an open-access article distributed under the terms of the Creative Commons Attribution License (CC BY). The use, distribution or reproduction in other forums is permitted, provided the original author(s) and the copyright owner(s) are credited and that the original publication in this journal is cited, in accordance with accepted academic practice. No use, distribution or reproduction is permitted which does not comply with these terms.
*Correspondence: Arvinder Kaur, YXJ2aW5kZXIxNjVAZ21haWwuY29t