- 1Hubei Key Laboratory of Mineral Resources Processing and Environment, Wuhan University of Technology, Wuhan, China
- 2School of Resources and Environmental Engineering, Wuhan University of Technology, Wuhan, China
- 3School of Public Policy and Management, Tsinghua University, Beijing, China
- 4Instituto de Física de la Universidad Autónoma de San Luis Potosí, San Luis Potosí, Mexico
The clay–microbial consortium is ubiquitous in the soil near the mining area and plays an important role in the transport of heavy metals. In this study, Chlorella sorokiniana FK was separated from lead–zinc mine tailings. The montmorillonite–Chlorella sorokiniana FK system as a typical case was applied to study Pb(II) biomineralization in the presence of usually co-existent Ca(II) and further reduce the migration and transformation of Pb(II) in the Ca(II) environment. Chlorella sorokiniana FK showed good resistance to Pb(II), and the addition of montmorillonite provided a more stable pH environment, which is conducive to the stability of Pb(II)-bearing bio-minerals. Montmorillonite created a low-biotoxicity environment in the overall process, especially less Pb(II) bio-adsorption capacity of individual Chlorella to protect the process of mineralization effectively. Batch experiment results also demonstrated that montmorillonite as the formation site of bio-minerals results in dispersed minerals on the surface of Chlorella and Chlorella–MMT composite, which is beneficial to the survival of Chlorella. Moreover, Pb(II)-bearing phosphate minerals tended to form in the Ca(II) environment rather than without Ca(II). This study demonstrated the mechanism of Pb(II) immobilization induced by Chlorella in the Ca(II) environment, further presenting a green, sustainable, and effective strategy for Pb(II) bio-immobilization combining clay minerals and microorganisms.
1 Introduction
Mining waste deposits (MWDs) as an important and constant source of lead pollution in the global environment brings a lot of environmental problems (Miler et al., 2022). Heavy metals {including lead [Pb(II)]} continuously leach from long-term stacked tailings and are further transported and transferred through wind, rain, surface water, and groundwater (Senn et al., 2014; Wang et al., 2019; Jiang et al., 2021). The stability and functions of the soil’s ecological environment are affected by heavy metal ions seriously (Zhang et al., 2022). Even long after mining stops, river systems near mining sites remain heavily contaminated with heavy metals (Obiri-Yeboah et al., 2021). Taking the Pearl River region as an example (Wu et al., 2020), the Pb(II) content in sediments is two orders of magnitude higher than the corresponding soil background value even downstream of the river. Lead is one of the most hazardous pollutants in nature (Yang et al., 2022), while divalent lead [Pb(II)] is the main form of lead in the environment (Krishnamoorthy et al., 2019), which is extremely bio-toxic (Priyanka et al., 2021) and easy to migrate (Zeng et al., 2022). Pb(II) always positively correlated with Ca(II) concentrations in the mining area (Li et al., 2020). Pb(II) in the environment accumulates in the human body through the food chain (Xu et al., 2021; Gao et al., 2022), and its accumulation shows significant renal toxicity (Bidanchi et al., 2022) and decreases bone strength in humans (Zeng et al., 2016; Osorio-Yanez et al., 2021). Thus, it is a priority to control Pb(II) and its migration in the mining area and further reduce the Pb(II) content in the environment.
In recent years, biomineralization induced by microorganisms has been widely studied as a potential sustainable technique for Pb(II) bio-remediation (Rajasekar et al., 2021; Shan et al., 2021; Yu et al., 2021). The clay–microbial consortium is universal and plays an important role in the transport of heavy metals in the mining area. Compared to conventional methods, the induction of bio-minerals containing Pb(II) can reduce its biotoxicity and migration effectively (Chen et al., 2021). Microalgae as the main producers in the ecosystem are cheap and easy to obtain (Ma et al., 2022; Ummalyma and Singh, 2022) and are widely applied to biological remediation (Cheng et al., 2023). Lee et al. (2014) effectively removed water-soluble radiostrontium by converting it to carbonate through the microalgal photosynthetic process (Lee et al., 2014). However, microalgae cannot survive prolonged periods under the stress of heavy metals (Wu et al., 2016; Li et al., 2022). Moreover, the pH changes mediated by microorganisms, during the overall process of biomineralization (Li et al., 2018), were not conducive to cell breathing and the stability of bio-minerals (Ivanina et al., 2020; Wang et al., 2021). Therefore, it is necessary to create a low toxic and pH-stable environment for the biomineralization process of microalgae.
Montmorillonite (MMT) is a three-layer-structured clay mineral that possesses excellent pH-buffering capacity (Bhattacharyya and Gupta, 2008; Tang et al., 2015) and has been demonstrated to facilitate the formation of cationic bridges between MMT and Chlorella in the process of Pb(II) bio-remediation, which effectively decreases the bio-toxicity caused by Pb(II) (Tan et al., 2022). In fact, the formation of cationic bridges was also revealed by Su et al. (2021) to prove the potential protection of Enterobacter sp. from Pb(II) stress by clay (Su et al., 2021). Moreover, MMT as clay minerals was demonstrated to provide nutrients and habitats for microorganisms (Li et al., 2019a). Ca(II) in the medium could also effectively reduce the Pb(II) accumulation in biological cells when co-existed with MMT (Hernandez-Garnica et al., 2021). In conclusion, both MMT and Ca(II) show great potential for protecting microbially induced Pb(II) remediation.
In this study, the MMT–Chlorella sorokiniana FK system was applied to Pb(II) biomineralization in the presence of Ca(II). The buffering capacity of MMT effectively reduced the pH changes caused by Chlorella and Chlorella–MMT composite. The Pb(II) immobilization efficiency induced by different bio-materials was compared to demonstrate the protective mechanism of MMT for algae. Also, the study found that adding MMT reduced the biotoxicity of Pb(II) and Pb(II)-bearing minerals, and MMT was considered the location where Pb(II)-bearing minerals were formed, helping the Chlorella survival.
2 Materials and methods
2.1 Preparation of Chlorella and Chlorella–MMT composite
In the overall experiment, Chlorella was cultured in a BG-11 medium containing 300 mg NaNO3, 36 mg CaCl2⋅2H2O, 6 mg ammonium citrate monohydrate, 6 mg ammonium ferric citrate, 1 mg EDTA, 2.86 μg H3BO3, 1.81 μg MnCl4⋅H2O, 0.222 μg ZnSO4⋅7 H2O, 0.39 μg NaMoO4⋅5H2O, 0.079 μg CuSO4⋅5H2O, 0.050 μg CoCl2⋅6 H2O, and 40 mg K2HPO4 in 1 L sterile distilled water. Moreover, microalgae were cultivated at 25°C with a light–dark cycle of 14/10 h under artificial radiation of 200 μmol proton−1m−2s−1. Furthermore, the microalgae cells were obtained at the stationary phase and washed three times with ultrapure water for subsequent experiments. Chlorella used in this experiment was isolated from Fan Kou lead–zinc mine tailings, Shaoguan City, and it is strong homology with Chlorella sorokiniana identified based on 18 S rDNA profiles as described by Koloren et al. (2016). The montmorillonite in this study was obtained from Ningcheng County, Chifeng City (Inner Mongolia, China), was sterilized under ultraviolet light for half an hour, and was further composited with Chlorella. Next, Chlorella and montmorillonite were thoroughly mixed in the ratio of 1:0, 1:1, and 1:5 (Chlorella: MMT, W/W) and stirred for 24 h. In addition, total microalgal biomass dry weight was controlled to 0.272 g/L.
2.2 Adsorption and mineralization
The concentrations of Pb(II) and Ca(II) in Chlorella and Chlorella–MMT composite suspension were measured to demonstrate the improved Pb(II) removal efficiency with MMT, and the influence of Ca(II) in the overall process was revealed. In this study, Pb(II) stock solution (15 g/L) and Ca(II) stock solution (1 mol/L) were prepared with Pb(NO3)2 and CaCl2. At the sampling time, 10 ml Pb(II) and Ca(II) stock solutions were added to the 1 L composite system. The initial concentration of Pb(II) was set at 150 mg/L, while the initial concentration of Ca(II) was 10 mmol/L. After all, a certain volume of suspension was taken and centrifuged at 5,000 rpm for 5 min. The centrifuged supernatant was used to determine the concentration of Pb(II) and Ca(II). Furthermore, the centrifuged sediment was freeze-dried and stored at −18°C for subsequent experiments.
2.3 Measurements
The atomic absorption spectrometer (Agilent 240FS flame atomic absorber) was used to detect the concentrations of Pb(II) and Ca(II). The composition analysis of crystalline materials was compiled by X-ray diffraction (XRD) (Zhang et al., 2013), and particular attention was paid to the parts of the clay mineral surface containing Pb(II) and Ca(II). The Bruker D8 advance diffractometer was used for diffraction analysis of mineral crystals on the surface of Chlorella and Chlorella–MMT composites under different conditions in this study. It is mainly used for the analysis of clay minerals (referring to montmorillonite) and minerals containing Pb(II)/Ca(II) on the surface of Chlorella and Chlorella–MMT composite. Scan diffraction angles were set from 10° to 80° at a scanning speed of 2°min. Furthermore, the effects of different montmorillonite contents and dissolved Ca(II) on the biologically induced formation of Pb-bearing minerals were explored. The laser Doppler electrophoresis technique using a Malvern Zetasizer (NanoZS90, United Kingdom) was used to investigate the zeta potential of Chlorella, montmorillonite, and their composites (1:1 and 1:5 Chlorella/MMT mass ratios) at a pH range of 5.0–7.5. The scanning electron microscope (SEM) and energy-dispersive spectrometer (EDS) (Phenom 6.0, Thermo Fisher Scientific, United States) were used to characterize the distribution of Pb(II)/Ca(II) on Chlorella, MMT, and their composite. Transmission electron microscopy and select area electrical diffraction (TEM-SAED, JEM-2100F) images of the samples were taken to reveal the morphology and main composition of mineral particles on the Chlorella–MMT composite. Fourier transform infrared spectroscopy (FT-IR, Thermo Scientific) analysis of Chlorella, MMT, and their composite (Chlorella: MMT = 1:1) ranged from 400 to 4,000 cm-1, further investigating functional groups involved in Pb(II)/Ca(II) adsorption and mineralization. X-ray photoelectron spectroscopy (Thermo Scientific ESCALAB 250Xi) measurements were performed to explain the change of elemental forms of C 1s, O 1s, and P 2p before and after Pb(II)/Ca(II) loaded, and the main elemental forms of Pb 4f, Ca 2p, Si 2p, and Al 2p after Pb(II)/Ca(II) loaded.
3 Results and discussion
3.1 Mineralization of Pb(II)
3.1.1 The structure of Pb(II)-bearing minerals
In this study, Pb(II) in the environment adsorbed by Chlorella, MMT, and their composite further formed stable metal minerals induced by microorganisms (Cheng et al., 2022). The structure of Pb(II)-bearing minerals induced by Chlorella was successfully observed on the Chlorella–MMT (1:1) composite via TEM and SAED. After 167 h Pb(II)/Ca(II) loaded, a single Chlorella cell was completely encapsulated by mineral particles. The minerals on the surface of Chlorella showed a collection of nanospherical particles with an average size of 10 nm in Figure 1 (a). Combing the SAED patterns in Figures 1F,G, Pb(II)-bearing minerals showed the typical aggregation of polycrystalline nanoparticles. The d-spacing of 3.23 and 7.38 Å matches well with the (202) and (310) planes of PbCO3, respectively. A wide amorphous separation ring observed at the periphery showed the amorphous-edged nanoparticles (Liu et al., 2019). As shown in Figure 1A, the lattice structures of parts c and d of region b were magnified and observed, and the lattice spacings equal to 0.46 and 0.27 nm correspond to the (200) and (400) planes of PbCO3, respectively. Pb(II) was successfully loaded as carbonate Pb(II)-bearing minerals, which showed a well-structured crystal. The formation of PbCO3 and other possible minerals on the surface of the Chlorella–MMT composite is necessary to investigate the process of biomineralization.
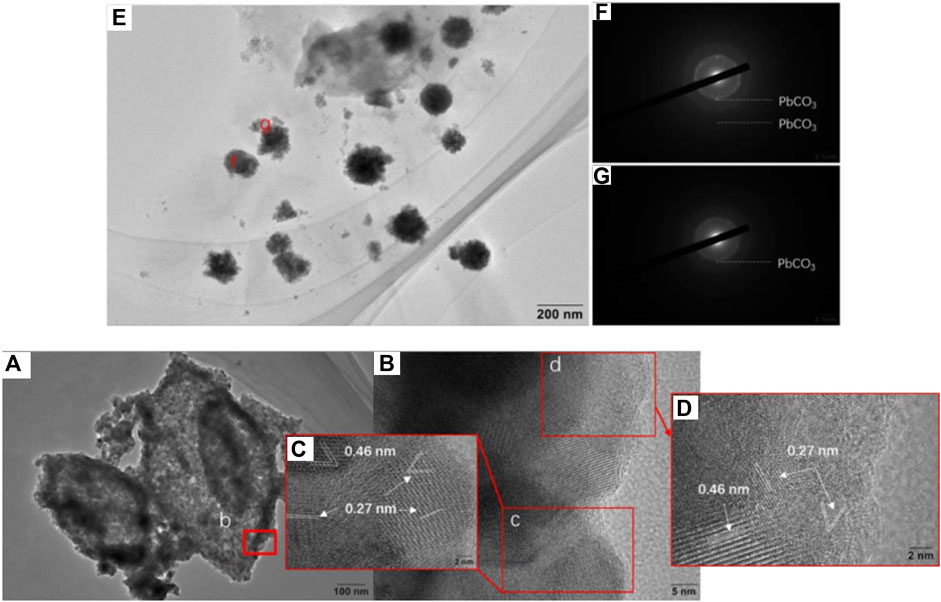
FIGURE 1. (A) HRTEM images of Chlorella–MMT (1:1) composites after Pb(II)/Ca(II) loaded. (B–E) Enlarged images of the corresponding positions. (F,G) SAED pattern of the corresponding position.
3.1.2 Bio-mineral compositions
XRD was used to characterize the mineral compositions of polycrystals under different treatments. Bio-minerals on the surface of Chlorella, MMT, Chlorella–MMT composite (1:1), and Chlorella–MMT composite (1:5) after Pb(II)/Ca(II) loaded were characterized to investigate the biomineralization induced by Chlorella. There was a broadening but less intense diffraction peak at 20 as in Figure 2A, which shows a distinctly amorphous shape without the diffraction peak of mineral crystals (Samsudin and Saadiah, 2018). The XRD patterns of MMT before and after Pb(II) loaded are shown in Figures 2B–D, and the reflections at 2θ = 22.0, 22.9, 23.4, 32.3, and 24.9° shown in Figure 2D were assigned to PbCl2 as chemical precipitate, which formed through the reaction of Cl-ion and Pb(II) in this solution (An and Dultz, 2008). Meanwhile, the reflections at 2θ = 21.0, 36.3, 41.8, and 46.4° were assigned to Pb5O8, and Pb(II) successfully broke the Si-O-Si bond and remained in the oxide matrix, surrounded by the O ion (Liu et al., 2014). Figures 2C–E show the XRD pattern of the Chlorella–MMT (1:1) composite before and after Pb(II)/Ca(II) loaded, respectively. Before Pb(II)/Ca(II) loaded, the reflections in the XRD pattern at 2θ = 17.8, 19.8, 23.6, 54.1, and 61.8° were completely assigned to MMT, while the reflections at 2θ = 19.8, 20.9, 36.0, 40.3, 54.1, and 73.2° were assigned to Pb3O(CO3)2H2O, and the XRD pattern at 2θ = 20.8, 34.6, 43.4, and 61.7° belong to PbCO3 after Pb(II)/Ca(II) loaded. The decreased pH shown in Figure 3 may be caused by the production of carbonates and CO2 in this study, with which the formation of Pb3O(CO3)2H2O/PbCO3 is mainly associated. Pb3O(CO3)2H2O/PbCO3 did not form on the surface of MMT alone, which suggests that the bio-minerals are mainly induced by Chlorella. Pb3O(CO3)2H2O observed on the surface of the Chlorella–MMT composite was mainly formed by PbO2 (Eq. 3) (Cowley, 1956), while PbCO3 and PbO2 were formed by Pb5O8 (Eqs 1, 2) (Winning et al., 2017). Pb(II) first entered MMT and further formed Pb(II)-bearing minerals induced by Chlorella, and this process facilitated the formation sites of Pb3O(CO3)2H2O/PbCO3 transfer to MMT. MMT as the formation site of Pb(II)-bearing minerals is conducive to reducing the bio-toxicity of Pb(II)-bearing minerals to Chlorella.
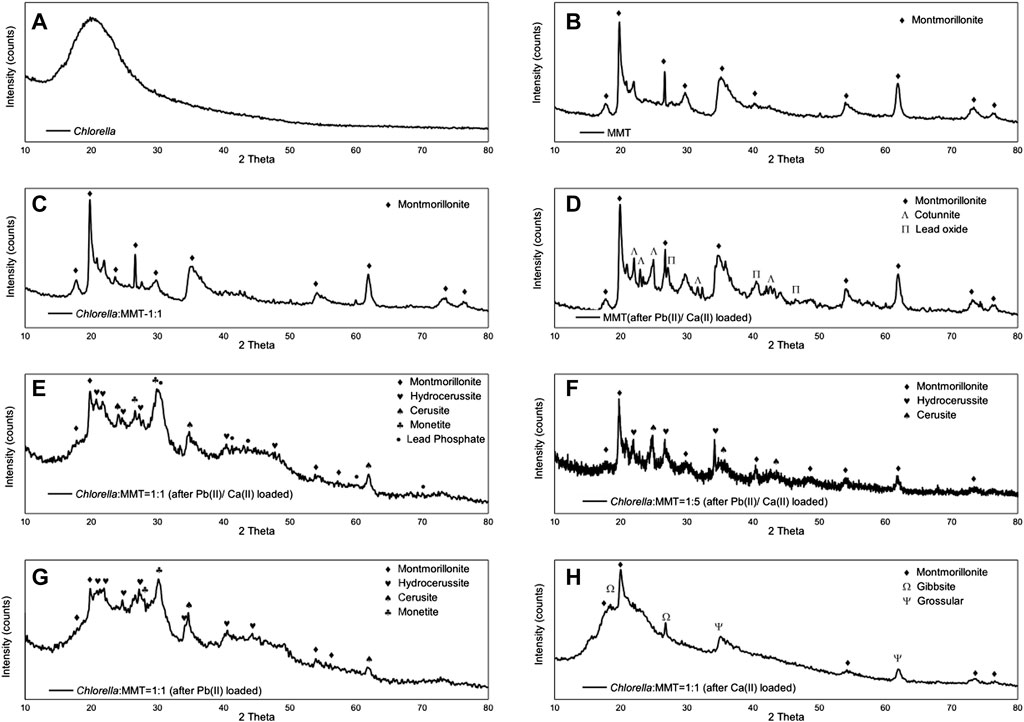
FIGURE 2. XRD spectra of (A) Chlorella; (B) MMT; (C) Chlorella: MMT = 1:1; (D) MMT (after Pb(II)/Ca(II) loaded); (E) Chlorella: MMT = 1:1 (after Pb(II)/Ca(II) loaded); (F) Chlorella: MMT = 1:5 (after Pb(II)/Ca(II) loaded); (G) Chlorella: MMT = 1.1 (after Pb(II) loaded); and (H) Chlorella: MMT = 1:1 [after Ca(II) loaded].
In addition, the mineral compositions on the surface of the Chlorella–MMT composite (1:1) after Pb(II) loaded and after Ca(II) loaded were compared to demonstrate the influence of Ca(II). Interestingly, the Pb(II)-bearing phosphate minerals were found in the presence of Ca(II). The reflections at 2θ = 30.3, 41.1, 44.3, 60.5, and 70.2° were assigned to Pb3(PO4)2 as shown in Figure 2E. In the process of Pb(II) remediation, Chlorella not only induced the formation of carbonate minerals but also phosphate minerals, whereas phosphate minerals are more stable than carbonate minerals in an acid environment. More stable Pb(II)-bearing minerals like phosphate minerals also is more conducive to reducing the migration of Pb(II) in the mining area. Similarly, we compared the results of the Chlorella–MMT (1:1) composite with the X-ray diffraction pattern of the Chlorella–MMT (1:5) composite. After Pb(II)/Ca(II) loaded, both Pb3O(CO3)2H2O and PbCO3 were detected rather than phosphate minerals. Phosphate minerals are mainly formed on the surface of Chlorella. Conversely, excess MMT as the formation site was not conducive to this process. The comparison of the results is shown in Figures 2G,H. When only Pb(II) is loaded, minerals on Chlorella–MMT (1:1) composite is mainly Pb3O(CO3)2H2O and PbCO3. Conversely, the XRD diffraction peaks at 2θ = 36.6, 37.9, 41.8, and 63.8° and 2θ = 36.6, 39.4, and 50.6° in Figure 2H were assigned to Al (OH)3 and Ca3Al2(SiO4)3, respectively. Chlorella did not induce Ca(II) carbonate biomineralization in the absence of Pb(II) stress.
3.2 Pb(II) remediation in coexistence with Ca(II)
3.2.1 pH stability
In Figure 3A, Pb(II) continues to decrease instead of re-release to the environment after being adsorbed by materials. The biomineralization process induced by Chlorella is always associated with the variation of pH in the environment. Therefore, it is necessary to investigate the role of biomineralization in the variation of pH values mediated by Chlorella. In fact, the acidification of the solution in the mineralization process could lead to the re-release of Pb(II)-bearing minerals, especially carbonate Pb(II)-bearing minerals. The pH variations in the three groups are shown in Figure 3. The Pb(II) immobilization induced by Chlorella alone caused larger environment pH changes, which is not conducive to the stability of Pb(II)-bearing minerals, especially carbonate Pb(II)-bearing minerals. At the same time, the addition of MMT from 1:1 to 1:5 in the group of Chlorella–MMT composite resulted in a more flatted pH change than Chlorella alone. In detail, the variation of pH mediated by Chlorella alone changed from 5.07 to 6.73, while the variation of pH mediated by Chlorella–MMT (1:1 and 1:5) composites ranged from 5.23 to 6.27 and 5.49 to 6.25, respectively, as shown in Figure 3. CO2 released from Chlorella in the presence of Pb(II) stress caused pH to continuously decline (Carfagna et al., 2013), further inducing the formation of Pb(II)-bearing carbonate minerals mentioned in Figure 1 and Figure 2. The increasing MMT content results in an environment with higher or lower pH values at the same periods in the process of mineralization. The increasing pH within 1 h is possibly caused by the assimilation of nitrate contained in the medium (Wang et al., 2016). MMT has provided a stable environment for Pb(II) remediation induced by Chlorella, while the pH-buffering capacity of MMT is favorable for reducing the release of Pb(II) in minerals.
3.2.2 Pb(II)/Ca(II) concentration
In the overall bio-remediation process, the concentration of Pb(II) and Ca(II) continues to decrease instead of removal stops after adsorption saturation as shown in Figure 4. There is a brief recovery of Pb(II) concentration that can be observed at 1–3 h, which is associated with increasing pH consistent with that pH ranging from 5.0 to 6.5 conducive to the adsorption of Pb(II) (Slaveykova and Wilkinson, 2003). The Pb(II) concentration in the group of Chlorella–MMT composite (1:1 and 1:5) was 1.06 times and 1.64 times as much as that in Chlorella alone on average. Moreover, Pb(II) in the environment will be continuously remediated by Chlorella, MMT, and Chlorella–MMT composite, not only adsorbed but also formed Pb(II)-bearing minerals induced by Chlorella. MMT as the formation site of Pb(II)-bearing minerals mentioned in Figure 2 also effectively reduces the concentration of Pb(II) and further protects the process of biomineralization induced by Chlorella in this way. The adsorption capacity of Chlorella was 1.86 times and 4.44 times as much as that of the Chlorella–MMT composite (1:1 and 1:5), respectively. MMT reduces the stress of Pb(II) to Chlorella in solution effectively. Generally, MMT has created a low-biotoxicity environment and led to less Pb(II) bio-adsorption by individual Chlorella. However, the addition of MMT seems to exhibit few significant differences in Ca(II) adsorption, and the variation of Ca(II) in the three groups is almost alike, which suggests that decreased Ca(II) is mainly associated with Chlorella rather than MMT. The uptake of Ca(II) by Chlorella is conducive to reducing intracellular Pb(II) accumulation (Li et al., 2019a). These are beneficial for the survival of Chlorella and further facilitated the biomineralization induced by Chlorella.
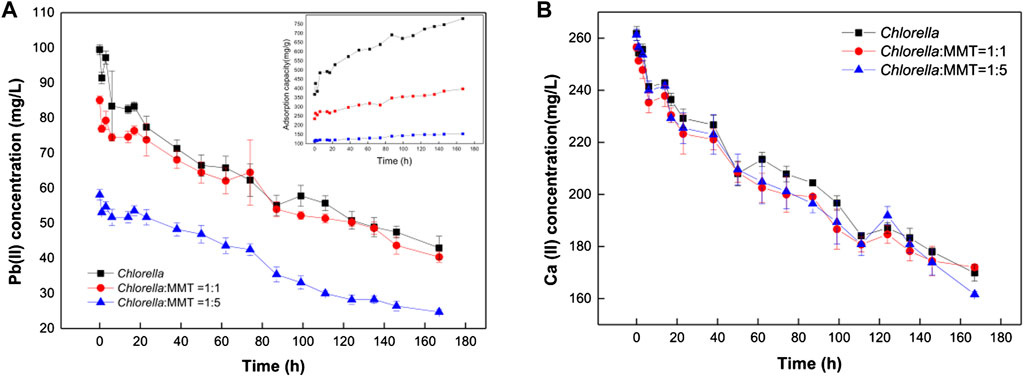
FIGURE 4. Variation of Pb(II) concentration (A) and Ca(II) concentration (B) in Chlorella and Chlorella–MMT (1:1 and 1:5) composite suspension.
3.2.3 Less bio-toxicity of Pb(II) and Pb(II)-bearing minerals
The clay–microbial consortium, Chlorella, MMT, and Chlorella–MMT composite exhibited significant electronegativity in the pH range from 5.0 to 7.5, while Figure 3 indicates that pH changed from 5.0 to 6.8 during the overall process. The results in Figure 5 showed that Chlorella had more Pb(II)-binding sites on its surface than MMT, which investigated the effect of negatively charged MMT on the whole process to illustrate the decrease of Pb(II) around Chlorella. The zeta potential of Chlorella changed from −19.9 mV to −29.8 mV, whereas that of MMT changed from −10.7 mV to −16.3 mV as shown in Figure 5. The increased MMT content led to a lower Pb(II)-binding capacity of the Chlorella–MMT composite. These results demonstrated that compounding Chlorella with MMT is beneficial to reducing the surface adsorption capacity of individual Chlorella, which also corresponds to the lower Pb(II) adsorption capacity exhibited by the Chlorella–MMT composite in Figure 4A and further facilitates Pb(II) mineralization induced by Chlorella in the presence of Ca(II).
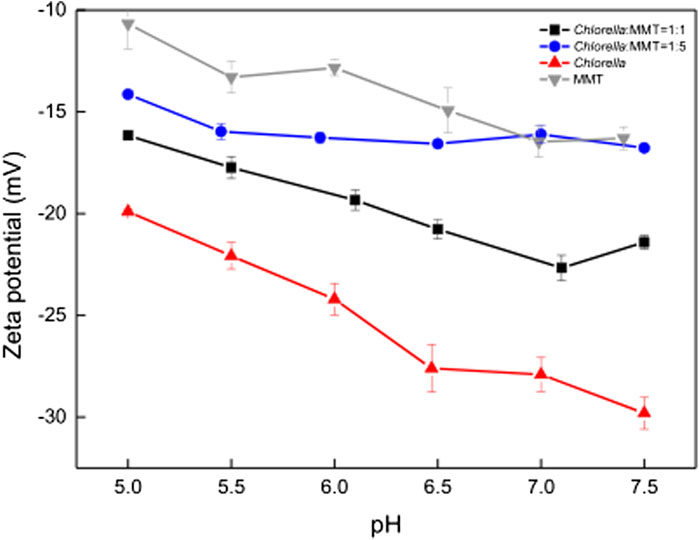
FIGURE 5. Zeta potential of MMT, Chlorella, Chlorella–MMT composite (1:1), and Chlorella–MMT (1:5) composite.
In addition, the SEM-EDS images of Chlorella and Chlorella–MMT (1:1) composite are shown in Figures 6A–C, respectively. Combining the crystal positions with Pb(II) distribution sites as shown in Figure 6, Pb(II) was successfully loaded on the surface of Chlorella and Chlorella–MMT composites and also demonstrated the position of Pb(II)-bearing minerals induced by Chlorella and Chlorella–MMT composites. In detail, the formed Pb minerals observed on the Chlorella cells were larger and more clustered, which is conducive to killing the Chlorella cells rather than protecting them because the larger Pb(II) minerals are easier to reduce the nutrition exchange for Chlorella (Rahman and Singh, 2020). Moreover, only small crystals were observed in the group of Chlorella–MMT composites (1:1 and 1:5), suggesting that the addition of MMT led to more dispersed minerals and was beneficial for the Chlorella by persistently inducing the formation of Pb(II) minerals.
3.3 Mechanism of Pb(II) bio-remediation
3.3.1 FT-IR
Pb(II) adsorbed is always associated with the functional groups on the surface of Chlorella, MMT, and Chlorella–MMT composites. Furthermore, the functional groups involved in the process of bio-remediation were revealed by the FT-IR spectra of MMT, Chlorella, and their composites (1:1) before and after Pb(II)/Ca(II) loaded. In Figure 7A, the characteristic peaks at 3622.08(Al-OH) and 3421.38(H-OH) cm−1 were assigned to -OH vibration of MMT and water molecules, respectively. For Chlorella, the peaks at 3308.20 and 1,394.41 cm−1 in Figure 7B were attributed to -OH stretching vibrations of carbohydrates, proteins, and lipids (Li et al., 2019b). As for the Chlorella–MMT composite, the peaks at 1,659.68 and 1,446.23 cm-1 belong to COO- stretching, whereas the peaks at 1,649.30 and 1,452.83 cm−1 in Figure 7B are also due to COO- stretching, which proved that Ca(II) and Pb(II) coordinate with the carboxylic acid group in a bidentate bridging manner (Ibrahim et al., 2011). For MMT and Chlorella–MMT composites, the assignments for the 518.32 and 518.06 cm−1 bands are Si-O and Si-O-Al stretching, respectively (Kumar and Lingfa, 2020). There is a medium sharp band at 465.88 and 466.10 cm−1, which is allocated due to Si-O-Si stretching. The microstructure of montmorillonite changed because of the adsorption and biomineralization process of Pb(II)/Ca(II). The disappearance of the peak at 3728.53 cm−1 (Al-OH) in Figure 7C could be also due to the ion exchange of MMT to adsorbed Ca(II)/Pb(II). The peaks at 1,047.24 and 1,044.46 cm−1 were assigned to asymmetric stretching modes of P-O bonds in (PO4)3− units (Nzaba Madila et al., 2022), shifted from 1,047.24 and 1,044.46 cm−1 to 1,047.97 and 1,040.74 cm−1 in Figures 7B,C due to the formation of phosphate minerals, which was induced by Chlorella after Pb(II)/Ca(II) adsorption. The shifted functional groups are summarized in Supplementary Table S1.
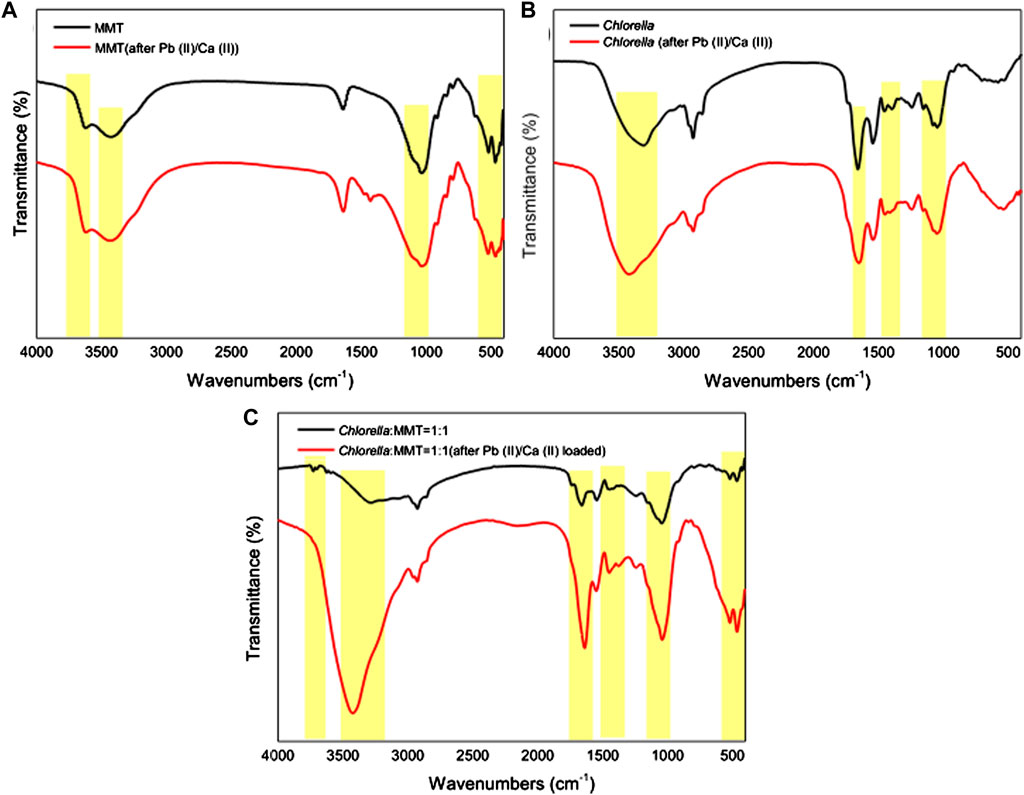
FIGURE 7. FT-IR spectra of (A) MMT, (B) Chlorella, and (C) Chlorella–MMT (1:1) composite before and after Pb(II)/Ca(II) loaded.
3.3.2 XPS
The XPS of the Chlorella–MMT (1:1) composites in Figure 8 was used to elucidate the Pb(II)/Ca(II) immobilization mechanism, especially the formation of Pb(II)-bearing minerals and the widely differing P chemical shifts. Supplementary Tables S2A, B show the surface elemental composition and relative data of the Chlorella–MMT composite detected from XPS analyses. The survey XPS spectrum of the Chlorella–MMT composite is shown in Figure 8A, which also revealed that Pb(II) was successfully loaded onto the Chlorella–MMT composite after Pb(II)/Ca(II) was contacted. In addition, Figures 8B–D show C 1s, O 1s, and P 2p XPS spectra in Chlorella–MMT composites before and after Pb(II)/Ca(II) loaded, respectively. The peaks at 284.80, 286.32, 288.02, and 289.05 eV in Figure 8B belong to C-C/C-H, C-O, C=O, and O=C-O, which could be assigned to aromatic, hydroxyl, alcohol, carboxyl, and ketone groups of Chlorella, respectively (Tan et al., 2022). In Figure 8C, the peaks at 532.25 eV assigned to C-OH (Huang et al., 2019) were consistent with the results in Figures 8A–C. The changes in peak area and binding energy demonstrated that carboxyl and hydroxyl groups played key roles in the process of Pb(II) adsorbed by the Chlorella–MMT composite. In addition, the peaks at 133.33 and 134.58 eV were attributed to the overlapped peaks of orthophosphate (ortho-P) and pyrophosphate (pyro-P) (Qian et al., 2014), which were widely distributed on the surface and inside of Chlorella (Kang et al., 2018), respectively. After Pb(II)/Ca(II) loaded, the original peaks disappeared and a new single peak at 139 eV appeared that belongs to PO43− (Nzaba Madila et al., 2022). Thus, the widely differing P chemical shifts among orthophosphate and pyrophosphate species are readily distinguishable after Pb(II)/Ca(II) is loaded. Ortho-P and pyro-P being hydrolyzed into PO43− by various phosphatases may be related to the increase in phosphatase activity caused by Ca(II) (Zhu et al., 2018; Hernandez-Garnica et al., 2021). Pb3(PO4)2 was formed further, which was consistent with the results in Figure.8E.
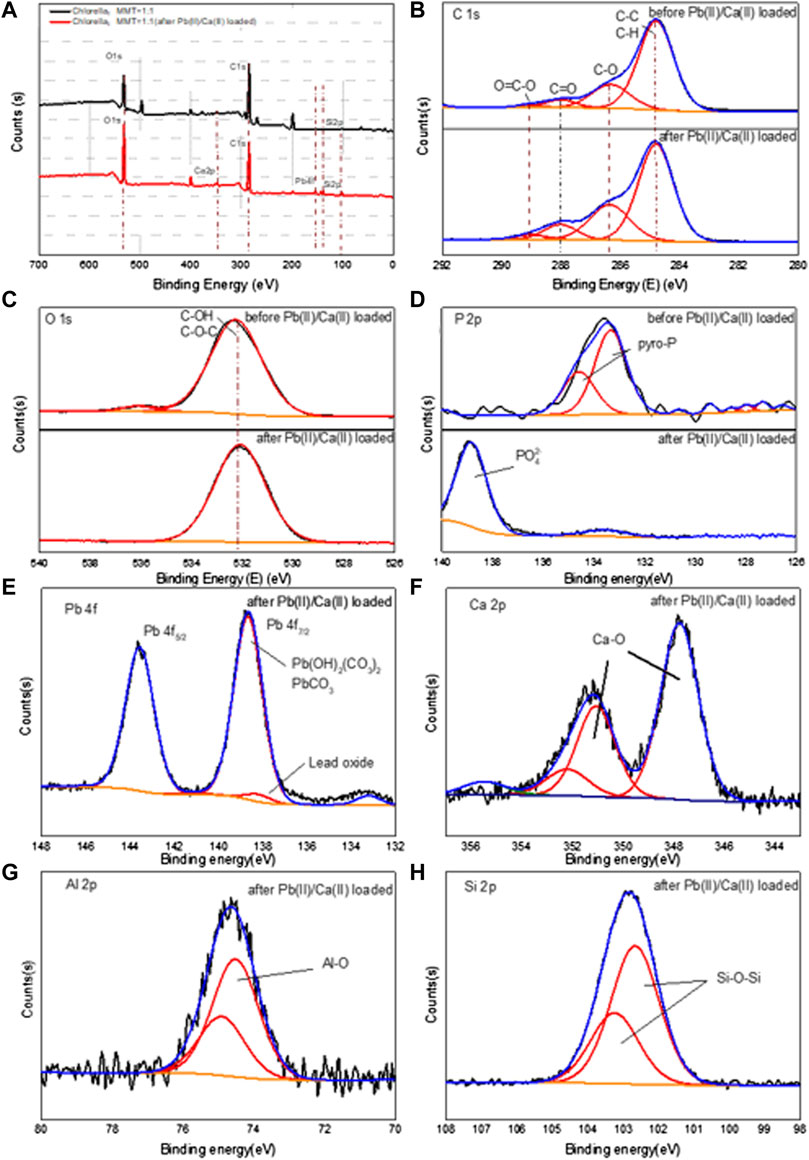
FIGURE 8. XPS spectra of full spectrum (A), C ls (B), O 1s (C), and P 2p (D) before and after Pb(II)/Ca(II) loaded; Pb 4f (E), Ca 2p (F), Al 2p (G), and Si 2p (H) after Pb(II)/Ca(II) loaded on Chlorella–MMT (1:1) composite.
After Pb(II)/Ca(II) loaded, Pb 4f, Ca 2p, Al 2p, and Si 2p XPS spectra in the Chlorella–MMT (1:1) composite are shown in Figures 8E–H. The Pb 4f peak can be fitted to two components centered at 138.67 eV and 143.57 eV, which were attributed to PbCO3/Pb3O(CO3)2H2O, respectively, while there is a single peak at 139 eV that was assigned to lead oxide, corresponding to the results shown in Figure 8E. Also, two distinct peaks for Ca 2p were attributed to Ca 2p3/2 (347.76 eV) and Ca 2p1/2 (351.34 eV) in Figure 8 (h). The binding energy interval between Ca 2p3/2 and Ca 2p1/2 is 3.58 eV, which indicated the spin-orbit splitting (binding energy separation) related to Ca-O (CaCO3) (Dhankhar et al., 2017; Saisopa et al., 2020). As shown in Figure 8G, the main peak in the Al 2p spectrum was located around 74.32 and 74.71 eV, which was assigned to the Al–O (Al2O3) bond in the composite after Pb(II) loaded; the presence of the small shoulder in the higher energy region of Al 2p spectra may be caused by Pb(II)/Ca(II) doping (Figueiredo et al., 2011). In Figure 8H, the Chlorella–MMT (1:1) composites showed a strong Si 2p peak at 102.46 and 103.04 eV after Pb(II)/Ca(II) loaded, which corresponded to the Si-O-Si bond of MMT. Similar results were also obtained in the XRD diffraction pattern and FT-IR spectra.
4 Conclusion
This study revealed the protective mechanism of MMT for the biomineralization of Pb(II) induced by Chlorella: one is providing a more stable pH environment, which is conducive to the stability of Pb(II)-bearing carbonated minerals. Second is beneficial to creating a low-biotoxicity environment and less Pb(II) bio-adsorption capacity of individual Chlorella. MMT as the formation sites of Pb(II)-bearing minerals rather than Chlorella results in more dispersed minerals on the surface of the Chlorella–MMT composite, both are conducive to decrease the bio-toxicity of Pb(II) and Pb(II)-bearing minerals. Additionally, the formation of stable phosphate minerals was observed in the presence of Ca(II) when ortho-P and pyro-P on the surface of Chlorella were hydrolyzed to PO43−. In general, this study expands the knowledge of the clay–microbial consortium boosting Pb(II) bioremediation induced by Chlorella and demonstrated the mechanism of Pb(II) immobilization, providing a new insight into the bioremediation of Pb(II) in the soil of mining with high Ca(II) content.
Data availability statement
The original contributions presented in the study are included in the article/Supplementary Material; further inquiries can be directed to the corresponding author.
Author contributions
ZW: writing—original draft preparation, methodology, data curation, and investigation. JC: validation. JT: methodology, data curation, and investigation. ZL: writing—review and editing. XW: data curation. JL: writing—reviewing and editing, conceptualization, and supervision.
Funding
This work was financially supported by the National Natural Science Foundation of China (Grant No. 32061123009).
Acknowledgments
The authors thank their colleagues for providing technical assistance and scientific support, as well as their college and supervisors for providing analytical equipment.
Conflict of interest
The authors declare that the research was conducted in the absence of any commercial or financial relationships that could be construed as a potential conflict of interest.
Publisher’s note
All claims expressed in this article are solely those of the authors and do not necessarily represent those of their affiliated organizations, or those of the publisher, the editors, and the reviewers. Any product that may be evaluated in this article, or claim that may be made by its manufacturer, is not guaranteed or endorsed by the publisher.
Supplementary material
The Supplementary Material for this article can be found online at: https://www.frontiersin.org/articles/10.3389/fenvs.2022.983430/full#supplementary-material
References
An, J.-H., and Dultz, S. (2008). Adsorption of Cr(VI) and as(V) on chitosan-montmorillonite: Selectivity and pH dependence. Clays Clay Min. 56, 549–557. doi:10.1346/CCMN.2008.0560508
Bhattacharyya, K. G., and Gupta, S. S. (2008). Adsorption of a few heavy metals on natural and modified kaolinite and montmorillonite: A review. Adv. Colloid Interface Sci. 140, 114–131. doi:10.1016/j.cis.2007.12.008
Bidanchi, R. M., Lalrindika, L., Khushboo, M., Bhanushree, B., Dinata, R., Das, M., et al. (2022). Antioxidative, anti-inflammatory and anti-apoptotic action of ellagic acid against lead acetate induced testicular and hepato-renal oxidative damages and pathophysiological changes in male Long Evans rats. Environ. Pollut. 302, 119048. doi:10.1016/j.envpol.2022.119048
Carfagna, S., Lanza, N., Salbitani, G., Basile, A., Sorbo, S., and Vona, V. (2013). Physiological and morphological responses of lead or cadmium exposed Chlorella sorokiniana 211-8K (Chlorophyceae). SpringerPlus 2, 147. doi:10.1186/2193-1801-2-147
Chen, M., Li, Y., Jiang, X., Zhao, D., Liu, X., Zhou, J., et al. (2021). Study on soil physical structure after the bioremediation of Pb pollution using microbial-induced carbonate precipitation methodology. J. Hazard. Mat. 411, 125103. doi:10.1016/j.jhazmat.2021.125103
Cheng, Y., Zhang, T., Chen, S., Li, F., Qing, R., Lan, T., et al. (2023). Unusual uranium biomineralization induced by green algae: Behavior investigation and mechanism probe. J. Environ. Sci. 124, 915–922. doi:10.1016/j.jes.2022.02.028
Cheng, Y., Zhang, T., Zhang, L., Ke, Z., Kovarik, L., and Dong, H. (2022). Resource recovery: Adsorption and biomineralization of cerium by Bacillus licheniformis. J. Hazard. Mat. 426, 127844. doi:10.1016/j.jhazmat.2021.127844
Cowley, J. M. (1956). Electron-diffraction study of the structure of basic lead carbonate, 2 PbCO3. Pb(OH)2. Acta Crystallogr. 9, 391–396. doi:10.1107/S0365110X56001133
Dhankhar, S., Bhalerao, G., Ganesamoorthy, S., Baskar, K., and Singh, S. (2017). Growth and comparison of single crystals and polycrystalline brownmillerite Ca2Fe2O5. J. Cryst. Growth 468, 311–315. doi:10.1016/j.jcrysgro.2016.09.051
Figueiredo, N. M., Carvalho, N. J. M., and Cavaleiro, A. (2011). An XPS study of Au alloyed Al–O sputtered coatings. Appl. Surf. Sci. 257, 5793–5798. doi:10.1016/j.apsusc.2011.01.104
Gao, P. P., Zhang, X. M., Xue, P. Y., Dong, J. W., Dong, Y., Zhao, Q. L., et al. (2022). Mechanism of Pb accumulation in Chinese cabbage leaves: Stomata and trichomes regulate foliar uptake of Pb in atmospheric PM2.5. Environ. Pollut. 293, 118585. doi:10.1016/j.envpol.2021.118585
Hernandez-Garnica, M., García-García, R. J. D., Moreno-S anchez, R., and Sanchez-Thomas, R. (2021). Lead accumulation in photosynthetic Euglena gracilis depends on polyphosphates and calcium. Environ. Pollut. 272, 116007. doi:10.1016/j.envpol.2020.116007
Huang, R., Huo, G., Song, S., Li, Y., Xia, L., and Gaillard, J. F. (2019). Immobilization of mercury using high-phosphate culture-modified microalgae. Environ. Pollut. 254, 112966. doi:10.1016/j.envpol.2019.112966
Ibrahim, A. A., Adel, A. M., El–Wahab, Z. H. A., and Al–Shemy, M. T. (2011). Utilization of carboxymethyl cellulose based on bean hulls as chelating agent. Synthesis, characterization and biological activity. Carbohydr. Polym. 83, 94–115. doi:10.1016/j.carbpol.2010.07.026
Ivanina, A. V., Jarrett, A., Bell, T., Rimkevicius, T., Beniash, E., and Sokolova, I. M. (2020). Effects of seawater salinity and pH on cellular metabolism and enzyme activities in biomineralizing tissues of marine bivalves. Comp. Biochem. Physiology Part A Mol. Integr. Physiology 248, 110748. doi:10.1016/j.cbpa.2020.110748
Jiang, L., Sun, H., Peng, T., Ding, W., Liu, B., and Liu, Q. (2021). Comprehensive evaluation of environmental availability, pollution level and leaching heavy metals behavior in non-ferrous metal tailings. J. Environ. Manage. 290, 112639. doi:10.1016/j.jenvman.2021.112639
Kang, J. H., Han, J., Lee, H., Lim, M. H., Kim, K.-T., and Kim, C. (2018). A water-soluble fluorescence chemosensor for the sequential detection of Zn2+ and pyrophosphate in living cells and zebrafish. Dyes Pigm. 152, 131–138. doi:10.1016/j.dyepig.2018.01.039
Koloren, O., Koloren, Z., and Eker, S. (2016). Molecular phylogeny of Artemisia species based on the internal transcribed spacer (ITS) of 18S-26S rDNA in Ordu Province of Turkey. Biotechnol. Biotechnol. Equip. 30, 929–934. doi:10.1080/13102818.2016.1188674
Krishnamoorthy, R., Govindan, B., Banat, F., Sagadevan, V., Purushothaman, M., and Show, P. L. (2019). Date pits activated carbon for divalent lead ions removal. J. Biosci. Bioeng. 128, 88–97. doi:10.1016/j.jbiosc.2018.12.011
Kumar, A., and Lingfa, P. (2020). Sodium bentonite and kaolin clays: Comparative study on their FT-IR, XRF, and XRD. Mater. Today Proc. 22, 737–742. doi:10.1016/j.matpr.2019.10.037
Lee, S. Y., Jung, K. H., Lee, J. E., Lee, K. A., Lee, S. H., Lee, J. Y., et al. (2014). Photosynthetic biomineralization of radioactive Sr via microalgal CO2 absorption. Bioresour. Technol. 172, 449–452. doi:10.1016/j.biortech.2014.09.023
Li, F., Wang, W., Li, C., Zhu, R., Ge, F., Zheng, Y., et al. (2018). Self-mediated pH changes in culture medium affecting biosorption and biomineralization of Cd(2+) by Bacillus cereus Cd01. J. Hazard. Mat. 358, 178–186. doi:10.1016/j.jhazmat.2018.06.066
Li, G. L., Zhou, C. H., Fiore, S., and Yu, W. H. (2019). Interactions between microorganisms and clay minerals: New insights and broader applications. Appl. Clay Sci. 177, 91–113. doi:10.1016/j.clay.2019.04.025
Li, J., Li, Z., Brandis, K. J., Bu, J., Sun, Z., Yu, Q., et al. (2020). Tracing geochemical pollutants in stream water and soil from mining activity in an alpine catchment. Chemosphere 242, 125167. doi:10.1016/j.chemosphere.2019.125167
Li, X., Yang, C., Lin, Y., Hu, T., and Zeng, G. (2022). Effects of oxytetracycline and zinc ion on nutrient removal and biomass production via microalgal culturing in anaerobic digester effluent. Bioresour. Technol. 346, 126667. doi:10.1016/j.biortech.2021.126667
Li, Y., Song, S., Xia, L., Yin, H., García Meza, J. V., and Ju, W. (2019). Enhanced Pb(II) removal by algal-based biosorbent cultivated in high-phosphorus cultures. Chem. Eng. J. 361, 167–179. doi:10.1016/j.cej.2018.12.070
Liu, M., Zhao, L., Liu, Y., Lan, Z., Chang, L., Li, Y., et al. (2014). Role of heavy metal ions in the formation of oxyfluoride glasses and glass ceramics. J. Mater. Sci. Technol. 30 (30), 1213–1216. doi:10.1016/j.jmst.2014.02.003
Liu, X., Cao, J., Li, Y., Hu, G., and Wang, G. (2019). A study of metal-bearing nanoparticles from the Kangjiawan Pb-Zn deposit and their prospecting significance. Ore Geol. Rev. 105, 375–386. doi:10.1016/j.oregeorev.2018.12.025
Ma, Y., Shen, W., Tang, T., Li, Z., and Dai, R. (2022). Environmental estrogens in surface water and their interaction with microalgae: A review. Sci. Total Environ. 807, 150637. doi:10.1016/j.scitotenv.2021.150637
Miler, M., Bavec, S., and Gosar, M. (2022). The environmental impact of historical Pb-Zn mining waste deposits in Slovenia. J. Environ. Manage. 308, 114580. doi:10.1016/j.jenvman.2022.114580
Nzaba Madila, E. E., Rousselot, S., Rioux, M., Dollé, M., and Duong, A. (2022). Comparative studies of synthesis, structure, optical properties and conductivity of the monoclinic phases Na2Co6Mn2(PO4)6 and Na2Co6Ni2(PO4)6. Solid State Sci. 124, 106779. doi:10.1016/j.solidstatesciences.2021.106779
Obiri-Yeboah, A., Nyantakyi, E. K., Mohammed, A. R., Yeboah, S. I. I. K., Domfeh, M. K., and Abokyi, E. (2021). Assessing potential health effect of lead and mercury and the impact of illegal mining activities in the Bonsa river, Tarkwa Nsuaem, Ghana. Sci. Afr. 000, e00876. doi:10.1016/j.sciaf.2021.e00876
Osorio-Yanez, C., Sanchez-Guerra, M., Solano, M., Baccarelli, A., Wright, R., Sanders, A. P., et al. (2021). Metal exposure and bone remodeling during pregnancy: Results from the PROGRESS cohort study. Environ. Pollut. 282, 116962. doi:10.1016/j.envpol.2021.116962
Priyanka, N., Geetha, N., Manish, T., Sahi, S. V., and Venkatachalam, P. (2021). Zinc oxide nanocatalyst mediates cadmium and lead toxicity tolerance mechanism by differential regulation of photosynthetic machinery and antioxidant enzymes level in cotton seedlings. Toxicol. Rep. 8, 295–302. doi:10.1016/j.toxrep.2021.01.016
Qian, T. T., Li, D. C., and Jiang, H. (2014). Thermochemical behavior of tris(2-butoxyethyl) phosphate (TBEP) during co-pyrolysis with biomass. Environ. Sci. Technol. 48, 10734–10742. doi:10.1021/es502669s
Rahman, Z., and Singh, V. P. (2020). Bioremediation of toxic heavy metals (THMs) contaminated sites: Concepts, applications and challenges. Environ. Sci. Pollut. Res. 27, 27563–27581. doi:10.1007/s11356-020-08903-0
Rajasekar, A., Wilkinson, S., and Moy, C. K. S. (2021). MICP as a potential sustainable technique to treat or entrap contaminants in the natural environment: A review. Environ. Sci. Ecotechnology 6, 100096. doi:10.1016/j.ese.2021.100096
Saisopa, T., Klaiphet, K., Songsiriritthigul, P., Pokapanich, W., Tangsukworakhun, S., Songsiriritthigul, C., et al. (2020). Investigation of solvated calcium dication structure in pure water, methanol, and ethanol solutions by means of K and L2, 3-edges X-ray absorption spectroscopy. J. Electron Spectros. Relat. Phenom. 244, 146984. doi:10.1016/j.elspec.2020.146984
Samsudin, A. S., and Saadiah, M. A. (2018). Ionic conduction study of enhanced amorphous solid bio-polymer electrolytes based carboxymethyl cellulose doped NH 4 Br. J. Non-Crystalline Solids 497, 19–29. doi:10.1016/j.jnoncrysol.2018.05.027
Senn, D. B., Estes, E. R., Brabander, D. J., and Shine, J. P. (2014). Sources and fates of heavy metals in a mining-impacted stream: Temporal variability and the role of iron oxides. Sci. Total Environ. 490, 456–466. doi:10.1016/j.scitotenv.2014.04.126
Shan, B., Hao, R., Xu, H., Li, J., Li, Y., Xu, X., et al. (2021). A review on mechanism of biomineralization using microbial-induced precipitation for immobilizing lead ions. Environ. Sci. Pollut. Res. Int. 28, 30486–30498. doi:10.1007/s11356-021-14045-8
Slaveykova, V. I., and Wilkinson, K. J. (2003). Effect of pH on Pb biouptake by the freshwater alga Chlorella kesslerii. Environ. Chem. Lett. 1, 185–189. doi:10.1007/s10311-003-0041-8
Su, M., Han, F., Wang, M., Ma, J., Wang, X., Wang, Z., et al. (2021). Clay-assisted protection of Enterobacter sp. from Pb (II) stress. Ecotoxicol. Environ. Saf. 208, 111704. doi:10.1016/j.ecoenv.2020.111704
Tan, J., Yi, H., Zhang, Z., Meng, D., Li, Y., Xia, L., et al. (2022). Montmorillonite facilitated Pb(II) biomineralization by Chlorella sorokiniana FK in soil. J. Hazard. Mat. 423, 127007. doi:10.1016/j.jhazmat.2021.127007
Tang, Q., Katsumi, T., Inui, T., and Li, Z. (2015). Influence of pH on the membrane behavior of bentonite amended Fukakusa clay. Sep. Purif. Technol. 141, 132–142. doi:10.1016/j.seppur.2014.11.035
Ummalyma, S. B., and Singh, A. (2022). Biomass production and phycoremediation of microalgae cultivated in polluted river water. Bioresour. Technol. 351, 126948. doi:10.1016/j.biortech.2022.126948
Wang, J., Rosov, T., Wensel, P., McGowen, J., and Curtis, W. R. (2016). A preliminary implementation of metabolic-based pH control to reduce CO2 usage in outdoor flat-panel photobioreactor cultivation of Nannochloropsis oceanica microalgae. Algal Res. 18, 288–295. doi:10.1016/j.algal.2016.07.001
Wang, M., Wu, S., Guo, J., Liao, Z., Yang, Y., Chen, F., et al. (2021). Immobilization and migration of arsenic during the conversion of microbially induced calcium carbonate to hydroxylapatite. J. Hazard. Mater. 412, 125261. doi:10.1016/j.jhazmat.2021.125261
Wang, P., Sun, Z., Hu, Y., and Cheng, H. (2019). Leaching of heavy metals from abandoned mine tailings brought by precipitation and the associated environmental impact. Sci. Total Environ. 695, 133893. doi:10.1016/j.scitotenv.2019.133893
Winning, L. D., Gorczyca, B., and Brezinski, K. (2017). Effect of total organic carbon and aquatic humic substances on the occurrence of lead at the tap. Water Qual. Res. J. 52, 2–10. doi:10.2166/wqrjc.2017.028
Wu, W., Qu, S., Nel, W., and Ji, J. (2020). The impact of natural weathering and mining on heavy metal accumulation in the karst areas of the Pearl River Basin, China. Sci. Total Environ. 734, 139480. doi:10.1016/j.scitotenv.2020.139480
Wu, Y., Wang, Y., Du, J., Wang, Z., and Wu, Q. (2016). Effects of yttrium under lead stress on growth and physiological characteristics of Microcystis aeruginosa. J. Rare Earths 34, 747–756. doi:10.1016/S1002-0721(16)60089-3
Xu, Q., Gao, Y., Wu, X., Ye, J., Ren, X., Zhou, Z., et al. (2021). Derivation of empirical model to predict the accumulation of Pb in rice grain. Environ. Pollut. 274, 116599. doi:10.1016/j.envpol.2021.116599
Yang, B., Shan, J., Xing, F., Dai, X., Wang, G., Ma, J., et al. (2022). Distribution, accumulation, migration and risk assessment of trace elements in peanut-soil system. Environ. Pollut. 304, 119193. doi:10.1016/j.envpol.2022.119193
Yu, X., Jiang, J., Liu, J., and Li, W. (2021). Review on potential uses, cementing process, mechanism and syntheses of phosphate cementitious materials by the microbial mineralization method. Constr. Build. Mater. 273, 121113. doi:10.1016/j.conbuildmat.2020.121113
Zeng, Q., Zhu, T., Wen, Y., Li, F., Cheng, Y., Chen, S., et al. (2022). The dynamic behavior and mechanism of uranium (VI) biomineralization in Enterobacter sp. X57. Chemosphere 298, 134196. doi:10.1016/j.chemosphere.2022.134196
Zeng, X., Xu, X., Boezen, H. M., and Huo, X. (2016). Children with health impairments by heavy metals in an e-waste recycling area. Chemosphere 148, 408–415. doi:10.1016/j.chemosphere.2015.10.078
Zhang, H. Y., Xia, Y. M., Liu, X. J., and Li, T. D. (2013). Research on influence of diffractometer slits on XRD pattern. Key Eng. Mat. 544, 441–444. doi:10.4028/www.scientific.net/kem.544.441
Zhang, M., Zhang, T., Zhou, L., Lou, W., Zeng, W., Liu, T., et al. (2022). Soil microbial community assembly model in response to heavy metal pollution. Environ. Res. 213, 113576. doi:10.1016/j.envres.2022.113576
Keywords: mining area, clay–microbial consortium, montmorillonite, microalgae, bio-immobilization, lead, calcium
Citation: Wang Z, Chen J, Tan J, Lu Z, Wang X and Jianbo Li (2022) The bio-immobilization of Pb(II) induced by the Chlorella–montmorillonite composite in the Ca(II) environment. Front. Environ. Sci. 10:983430. doi: 10.3389/fenvs.2022.983430
Received: 01 July 2022; Accepted: 21 July 2022;
Published: 23 August 2022.
Edited by:
Delong Meng, Central South University, ChinaCopyright © 2022 Wang, Chen, Tan, Lu, Wang and Jianbo Li. This is an open-access article distributed under the terms of the Creative Commons Attribution License (CC BY). The use, distribution or reproduction in other forums is permitted, provided the original author(s) and the copyright owner(s) are credited and that the original publication in this journal is cited, in accordance with accepted academic practice. No use, distribution or reproduction is permitted which does not comply with these terms.
*Correspondence: Jianbo Li, lijianbo3051@163.com