- 1College of Agriculture, Bahauddin Zakariya University, Bahadur Sub-Campus Layyah, Layyah, Pakistan
- 2Department of Pharmaceutics, College of Pharmacy, Umm Al-Qura University, Makkah, Saudi Arabia
- 3Department of Pharmaceutics and Industrial Pharmacy, College of Pharmacy, Minia University, Minia, Egypt
- 4Department of Agricultural Engineering, Khwaja Fareed University of Engineering and Information Technology, Rahim Yar Khan, Pakistan
- 5Pharmacy Practice Department, College of Pharmacy, Shaqra University, Shaqra, Saudi Arabia
- 6Clinical Pharmacy Department, National Cancer Institute, Cairo University, Giza, Egypt
- 7Agricultural Botany Department, Faculty of Agriculture (Saba Basha), Alexandria University, Alexandria, Egypt
- 8Bioinformatics Department, Genetic Engineering and Biotechnology Research Institute, University of Sadat City, Sadat City, Egypt
- 9Department of Agronomy, College of Agriculture, University of Sargodha, Punjab, Pakistan
Arsenic (As) contamination in the soil adversely affects crop productivity, grain quality, and human health. A pot experiment was performed to assess the sole and combined effects of Si and biochar on growth, physiological and antioxidant defense mechanisms, yield, and grain quality of maize. Soil treatments comprised of control (no treatment), As, Si, biochar, Si + biochar, As + Si, As + biochar, and As + Si + biochar placed in a completely randomized design with three replications. As toxicity significantly reduced chlorophyll a (5.18%), chlorophyll b (33.87%), chlorophyll a + b (11.67%), and primary metabolites [soluble protein (54.93%), amino acids (24.85%), total soluble sugars (39.77%), and phenolic contents (25.88%)], while increasing the activities of enzymatic antioxidants such as superoxide dismutase (SOD) by 43.51%, peroxidase (POD) by 47.93%, catalase (CAT) by 47.98%, and ascorbate peroxidase (APX) by 59.02%, as well as that of lipid peroxidation in the leaves of maize. In addition, As contamination reduced the grain yield and yield-related attributes relative to the respective controls. Among the soil applications, the interactive effect of Si and biochar improved maize grain yield (12.12%) by triggering activities of enzymatic antioxidants and proline contents and reducing the H2O2 and MDA contents. The combined application of Si and biochar enhanced the Si contents in shoots of the control and As-contaminated plants, while significantly reducing As concentration in shoots (69%) and grains (142%). In conclusion, the combined application of Si and biochar was found to be a fruitful soil amendment strategy to improve the yield of maize and reduce the toxic limit of As under As-contaminated soil. The results of this study may be useful for the cultivation of food crops under AS-contaminated soils, but before commercial recommendation, more trails are required under field conditions.
Introduction
Globally, maize (Zea mays L.) is an important cereal crop after wheat and rice. Hence, the hazard of heavy metal impurities in cereal grains, especially of As, is a major threat to human well-being concerning health issues (Suriyagoda et al., 2018; Bianucci et al., 2020; Shah et al., 2021). Plant cells accumulate As due to the uptake and translocation of As via aquaporins and phosphate transporters (Kertulis -Tartar et al., 2009; Farooq et al., 2019; Hammad et al., 2022). Therefore, various functions of plants such as energy production, nutrient uptake, plant metabolism imbalance, and physiology are disrupted (Bakhat et al., 2017; Muhammad et al., 2020).
Heavy metals are a major concern for plant and human nutrition as these not only affect plant growth (Mehmood et al., 2018; Haseeb et al., 2022; Javad et al., 2022) and development but also cause health issues in humans and livestock when they consume food grown in the soils contaminated with heavy metals (Abdul et al., 2015). Arsenic (As) is a nonessential poisonous element for plants and animals and has no proven biological role in living tissues (Abdul et al., 2015; Farooq et al., 2019). As enters the food chain primarily from cereals grown in soils having As contamination (Awasthi et al., 2017). The maximum permissible concentration of As in corn grain in certain countries has been reported to be up to 0.2–0.3 mg kg−1 dry weight (Rosas-Castor et al., 2014). High concentrations of As in food can cause various health issues, such as malfunctioning of the renal system, cardiovascular system, and immune system (Abdul et al., 2015). The concentration of As in cereal grains could be influenced by improving our understanding of the As bioavailability in soils and studying its interactions among plants, soils, and the environment (Zhao and Wang, 2020). The adverse effects of As accumulation on functioning and chemical processes has been impairment of the development and maturity of crops (Du et al., 2017), resulting in lower grain yields and poor crop quality (Abdul, 2020). As accumulation in plants triggers the production of reactive oxygen species (ROS), which restrains antioxidant processes, impairs DNA development, hinders metabolic activities in roots, and lowers photosynthetic activity in leaves (Hu et al., 2020).
Plants can tolerate abiotic stresses with the application of silicon (Kabir et al., 2016; Malhotra and Kapoor., 2019; Bukhari et al., 2020). In the literature, different scientists (Bogdan and Schenk, 2009; Ning et al., 2016) have observed the antagonistic behavior of Si and As in various plant species (Ma et al., 2008; Bogdan and Schenk, 2009; Ning et al., 2016). Due to variations in the processes of elemental uptake and translocation, the stress response in plants also varies among species (Ma et al., 2008; Kabir et al., 2016; Suriyagoda et al., 2018). Ma et al. (2008) reported that As accumulation and movement in rice roots follow a similar route (OsNIP2;1) to that of Si. Moreover, Si can change the interchangeable and soluble forms of As into stable structures (Ning et al., 2016). Arsenic accumulation was reduced in rice with an increase in soil Si concentration (Bogdan and Schenk, 2009; Bakhat et al., 2017), which is ascribed to lower the expression of Si transporter genes (i.e., Lsi1 and Lsi2). The Si transporter genes also facilitate As uptake (Bienert et al., 2008). The toxicity of metals in various plants can be ameliorated with the application of Si because Si alters the accumulation and translocation routes of various toxic heavy metals in plants (Ning et al., 2016; Farooq et al., 2019).
Immobilization is considered another way of remediating heavy metals–contaminated soils. Biochar application is an environment-friendly and economical immobilizer for heavy metal contamination (Srivatsav et al., 2019). Soil-applied biochar facilitates the stability of heavy metals via various activities such as adsorption, elemental exchanges, and complexation surfaces (Gao et al., 2019; Kumar and Bhattacharya, 2020; Kim et al., 2021). Biochar application in rice diminishes the interchangeable and bioavailable parts of As through its immobilization into a more steady structure, which reduces the toxic metal in plants (Irshad et al., 2020; Kabir et al., 2016). Soil amendment with biochar improves Si uptake by increasing the pH of the soil (Kim et al., 2021). Although the uptake of As also increases with an increase in pH (Marin et al., 1993; Dai et al., 2019). At the molecular level, it was found that the combination of Si and biochar (Si-rich biochar) downregulates the expression of Si-As transporter genes (Wang et al., 2019, 2020), which in return reduces the uptake of As, as both share the same transport channel. Therefore, it may be beneficial to use Si with biochar in As-contaminated soils to minimize the root transport of As and its translocation to above-ground plant parts.
Several studies have reported the amelioration effects of Si (Kashif et al., 2021) and/or biochar (Ibrahim et al., 2016) in different crops grown in heavy metals–contaminated soils. However, only a few considerable works have explored the combined impact of Si and biochar application in ameliorating heavy metal toxicity, especially in maize. As reviewed earlier, Si and biochar both have antagonistic effects on the uptake of AS; therefore, in this study, we hypothesized that a combined application of Si and biochar will reduce the uptake of As and ultimately the toxic effects of As. Therefore, the current study was designed to explore the impacts of Si and biochar applications as the sole amendments or their combined application on the morphological growth, physiological and biochemical processes, and yield attributes of maize grown in As-contaminated soils.
Materials and methods
Experimental conditions
A pot study was performed in a greenhouse under normal conditions (temperature 29/19°C day/night with 72% humidity) during the spring season of 2018. Seeds of maize hybrid NK-6654 were used for this research trial. Two plants were maintained in earthen pots (18 cm in diameter, 75 cm in height) filled with 15 kg of well-ground sandy loam soil. The soil contained 298 mg kg−1 total nitrogen (N), 16 mg kg−1 available phosphorus (P2O5), 78.84 mg kg−1 available potassium (K2O), and 0.61% organic matter, with pH 8.50 and 2.48 dS m−1 electrical conductivity. The recommended doses of N, P2O5, and K2O (100, 75, and 60 mg kg−1 of soil) as urea, diammonium phosphate, and sulfate of potash, respectively, were added to the soil.
Experimental treatments
The experiment comprised eight soil treatments: control (without any soil amendment), As (12 mg kg−1), Si (100 mg kg−1), biochar (50 g kg−1), Si + biochar (100 mg kg−1 + 50 g kg−1), As + Si (12 mg kg−1 + 100 mg kg−1), As + biochar (12 mg kg−1 + 50 g kg−1), and As + Si + biochar (12 mg kg−1 + 100 mg kg−1 + 50 g kg−1 soil) applied at the time of sowing. Already optimized concentrations of Si and biochar (Sattar et al., 2020; Kashif et al., 2021) were used in this experiment. The experiment was arranged in a completely randomized design (CRD) with three replications per treatment. Each replication had three pots with two plants per pot. Arsenic stress (12 mg kg−1 soil) was imposed by spiking the soil using sodium arsenite (NaAsO2) as the source. Silicon was added in the form of analytical-grade sodium silicate. Wheat straw was used for the preparation of biochar according to the method elaborated by Qayyum et al. (2015). After air drying, the wheat straw was put in a biochar machine for pyrolysis at 500°C for 30 min in an oxygen-limited environment. Before passing through a sieve (2 mm), the subsequent material was crushed to ensure uniform mixing in the soil. The chemical properties of wheat straw biochar are given in Table 1. Si, As, and biochar were thoroughly mixed in the soil as per treatments, and afterward, the pots were kept for 60 days in the open air for the stabilization of the treatments.
Observations and measurements
For physiological and biochemical analyses, plant samples were collected by clipping the top-second leaf at the grain filling stage (BBCH 80) of the crop in the late morning, 11:00 a.m., and stored at −20°C before further analyses.
Photosynthetic pigments
For the determination of photosynthetic pigments (chlorophyll a and b), 0.5 g of leaf samples was homogenized in 5 ml acetone (80%) (Lichtenthaler, 1987). The supernatant was separated after centrifugation (10,000g for 5 min). The absorbance of the supernatant was measured at 645 nm and 663 nm for chlorophyll a and b, respectively, using a UV-Vis spectrophotometer (Hitachi U-1800; Tokyo, Japan).
Determination of primary metabolites
Total soluble proteins in the leaf samples were determined through the Bradford’s (1976) assay using Coomassie blue dye. In total, 100 µL of the leaf sample and 2 ml of Bradford reagent were mixed in a test tube. The mixture was incubated in dark for 20 min and then absorbance measured at 595 nm.
In 50 mM chilled potassium phosphate buffer (5 ml; pH 7.5), fresh leaf tissues (0.2 g) were homogenized and centrifuged for 20 min at 10,000g. In a test tube, equal volumes of 10% pyridine and acid ninhydrin were homogenized with 1 mL of the supernatant. Afterward, the mixture was warmed at 95°C for 30 min using a water bath. Then, by using distilled water, the volume of the reaction mixture was made up to 7.5 ml. Then at 570 nm, the absorbance of the reaction mixture was observed (Hamilton and Van Slyke, 1943).
To measure the total soluble sugars, 5 ml of aqueous ethanol (80%) and 0.2 g of fresh leaf tissue were homogenized and centrifuged for 10 min at 3,500g. The sample extract (100 μL) obtained was then reacted with anthrone reagent (3 ml). Then, using a water bath, the mixture was heated for 10 min at 95°C. The mixture was cooled, and using a spectrophotometer, absorbance was observed at 625 nm (Yemm and Willis, 1954).
By using the method of Julkenen-Titto (1985), the total phenolic contents of fresh leaf samples was determined. For this purpose, 1 ml of acetone (80%) and 0.2 g fresh leaf tissues were homogenized and then centrifuged at 12,000 rpm for 15 min. Then, 20% Na2CO3 (2.5 ml), 0.5 -ml Folin–Ciocalteu’s phenol reagent, and supernatant (100 μL) were added to a test tube and shaken and then to make 5 ml final volume, distilled water was added. Finally, absorbance was recorded at 750 nm after 20 min.
Lipid peroxidation and free proline determination
To determine H2O2 content, 1.5 ml of trichloroacetic acid (0.1%) and fresh leaf tissue (0.15 g) were homogenized. Then, centrifugation of the homogenate was done at 12,000g for 15 min. Then, 0.5 ml of potassium sulfate buffer (10 mM, pH 7.0) and 1 ml of potassium iodide were added to the supernatant. At 390 nm, using a spectrophotometer, the absorbance of the reaction mixture and trichloroacetic acid (0.1%) was observed. By comparing the absorbance with a standard curve, the amount of H2O2 content was measured (Velikova et al., 2000). Hodges et al. (1999) described a method called the TBA method, which was used to measure MDA contents. Fresh leaves (0.15 g) were ground in 5.0 ml of 5% (w/v) TCA, and the extract was centrifuged. The MDA contents were measured at 600 nm and 532 nm. To measure proline contents, 5 ml sulphosalicylic acid (3%) and fresh leaf tissue (0.1 g) were homogenized, and the extract was centrifuged. Then, ninhydrin and glacial acetic acid reagent were added to the supernatant. The reaction mixture was boiled for 1 h in a water bath. After boiling, the reaction mixture was cooled for 10 min in an ice bath. Proline was extracted from a mixture with toluene, and absorbance was recorded at 520 nm (Bates et al., 1973). Using the method of Dionisio-Sese and Tobita (1998), electrolyte leakage was measured. In a test tube, 10 ml of distilled water and small and equal sized pieces of fresh leaf tissue (0.5 g) were added and stirred for 10 s. The test tubes were left to rest overnight, and electrical conductivity (EC1) was calculated. To measure EC2, the test tubes were autoclaved at 100°C for 1 h.
Determination of enzymatic antioxidants activities
Fresh leaf samples were homogenized with 5 ml of phosphate buffer (50 mM with 7.8 pH) and centrifuged (15,000g for 20 min). The inhibition of nitroblue tetrazolium (NBT) reduction provides the basis for superoxide dismutase (SOD) activity estimation, recorded at 560 nm (Giannopolitis and Ries, 1977). The reactants of the reaction mixture were 1 ml NBT (50 µM), 1 ml riboflavin (1.3 µM), 50 µL enzyme extract, 950 µL phosphate buffer (50 mM), 500 µL methionine (13 mM), and 500 µL EDTA (75 mM). The exposure of the reaction mixture to 30 W fluorescent lamp illuminance initiated the reaction, which was then stopped after 5 min by turning off the lamp. The blue formazan was formed due to NBT reduction and was observed at 560 nm. Using the same reactants but having no enzyme extract, blank reading was taken. Catalase activity (CAT) was recorded at 240 nm due to the production of H2O2. To initiate the reaction, 100 µL of the enzyme extract was added to the reaction mixture [900 µL H2O2 (5.9 mM) and 2 ml phosphate buffer (50 mM)]. Micromoles of H2O2 per minute per milligram of protein was used to define catalase activity (Chance and Maehly, 1955). The peroxidase (POD) activity was estimated using the protocol given by Kar and Mishra (1976). The reactants used comprised 5 ml of Tris-HCL buffer (0.1 M), 5 ml of pyrogallol (10 mM), 5 mM of H2O2 (5 mM), and 100 µL of enzyme extract. By noting the decline in absorbance at 425 nm, which was due to H2O2-dependent oxidation of pyrogallol, the POD activity was measured as POD IU per minute per milligram of the protein.
Morphological and yield-related attributes
At physiological maturity (BBCH 87), five plants were selected randomly, and their heights were measured from the soil surface to the tip of the plant by using a meter rod. From the same plants, leaves were removed manually, and the leaf area was measured with the help of a leaf area meter (Model CI-202; CID Bio Science Inc., WA). Cobs from the selected plants were separated with the help of scissors, and the cob length was measured with a measuring tape. The number of grains per cob was counted from five randomly selected cobs from each treatment. After the manual threshing of cobs, grain yield and 100-grain weight were calculated. Hundred seeds from each replication were counted using a digital seed counter, and then these were weighed on an electric balance to calculate the 100-grain weight.
Data analysis
Statistical analysis of data was done with the help of the Statistix 8.1 software (Analytical Software, Statistix; Tallahassee, FL, United States, 1985–2003) by using analysis of variance (ANOVA) techniques. For mean separation at a 5% probability level, Tukey’s test was used. The graphical presentation of data was done by using Sigma Plot.
Results
Morphological and yield attributes
It was observed that As stress significantly (p < 0.05) reduced the morphological attributes of maize (Table 2). When As stress was imposed on maize, it reduced the plant height by 19.88%, leaf area by 53.95%, and cob length by 18.18%. When Si was applied to As-contaminated plants, it increased the plant height by 4.17%, leaf area by 21.79%, and cob length by 7.14%. Likewise, biochar application on As-contaminated plants enhanced the plant height by 2.91%, cob length by 5.29%, and leaf area by 24.41%. While the application of combined Si + biochar enhanced the plant height by 5.08%, leaf area by 31.66%, and cob length by 10.62% when compared to As-contaminated maize plants. Under control conditions, Si application enhanced the morphological attributes of leaf area by 10.06% and cob length by 10.58%, while biochar application enhanced the leaf area by 6.59% and cob length by 10.58%. Similarly, the combined application of Si + biochar enhanced the leaf area by 13.78% and cob length by 14.64% (Table 2).
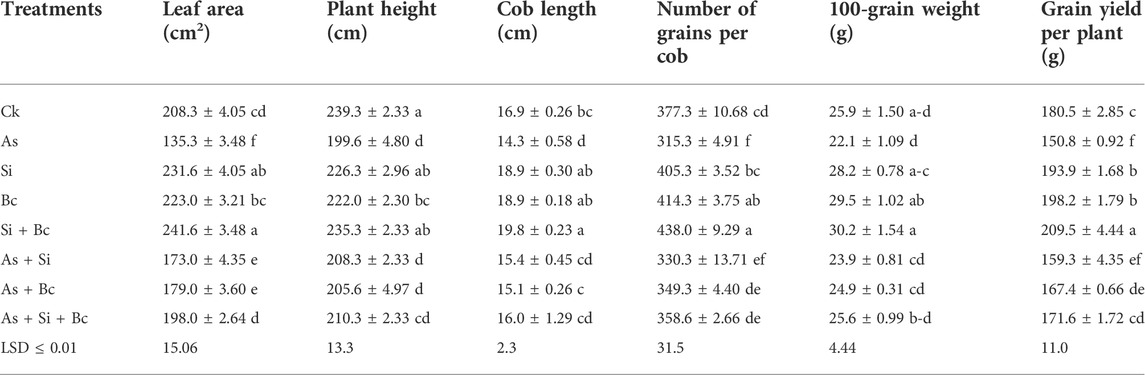
TABLE 2. Effect of sole and combined applications of Si and biochar (Bc) on leaf area, plant height, cob length, number of grains, 100-grain weight, and grain yield of maize under As toxicity (values are the average of three replication ± standard error).
Arsenic stress also affected negatively the yield attributes of maize plants. It reduced the number of grains per cob by 19.66%, 100-grain weight by 17.19%, and grain yield per plant by 19.69%, whereas Si application on As-contaminated plants enhanced the number of grains per cob by 4.54%, 100-grain weight by 7.53%, and grain yield per plant by 5.33%. Similarly, biochar application enhanced the number of grains per cob by 9.73%, 100-grain weight by 11.24%, and grain yield per plant by 9.91% when compared to As-treated plants. The combined application of Si + biochar on As treated plants enhanced the number of grains per cob by 12.07%, 100-grain weight by 13.67%, and grain yield per plant by 12.12%. Under normal conditions, the sole application of Si enhanced the number of grains per cob by 6.90%, 100-grain weight by 8.15%, and grain yield per plant by 6.91%, while biochar application enhanced the yield attributes such as the number of grains per cob by 8.93%, 100-grain weight by 25.08%, and grain yield per plant by 8.93%. Moreover, the combination of Si + biochar increased the number of grains per cob by 13.85%, 100-grain weight by 14.23%, and grain yield per plant by 13.84% when compared with the control treatment (Table 2).
Photosynthetic pigments
Analyses of variance depicted significant differences (p < 0.05) among the treatments for Chl a, Chl b, and Chl a + b (Figure 1). Chl a, Chl b, and Chl a + b contents in maize were significantly reduced by As toxicity. It was observed that As contamination reduced Chl a by 5.18%, Chl b by 33.87%, and Chl a + b by 11.67%, whereas the application of Si in As-contaminated plants improved Chl a by 9.86%, Chl b by 22.64%, and Chl a + b by 12.31% (Figure 1). Similarly, biochar application in As-treated plants improved Chl a by 9.45%, Chl b by 19.60%, and Chl a + b by 11.35%. However, the combined application of Si + biochar improved Chl a by 14.46%, Chl. b by 25.45%, and Chl a + b by 16.26%. The sole application of Si in non–As-contaminated plants (control) improved Chl a by 14.51%, Chl b by 19.48%, and Chl a + b by 15.69%. While biochar improved Chl a by 13.11%, Chl b by 7.46%, and Chl a + b by 11.89% in non-stressed plants. While the combination of Si + biochar improved the contents of Chl a by 20.30%, Chl b by 26.19%, and Chl a + b by 21.71% in maize leaves (Figure 1).
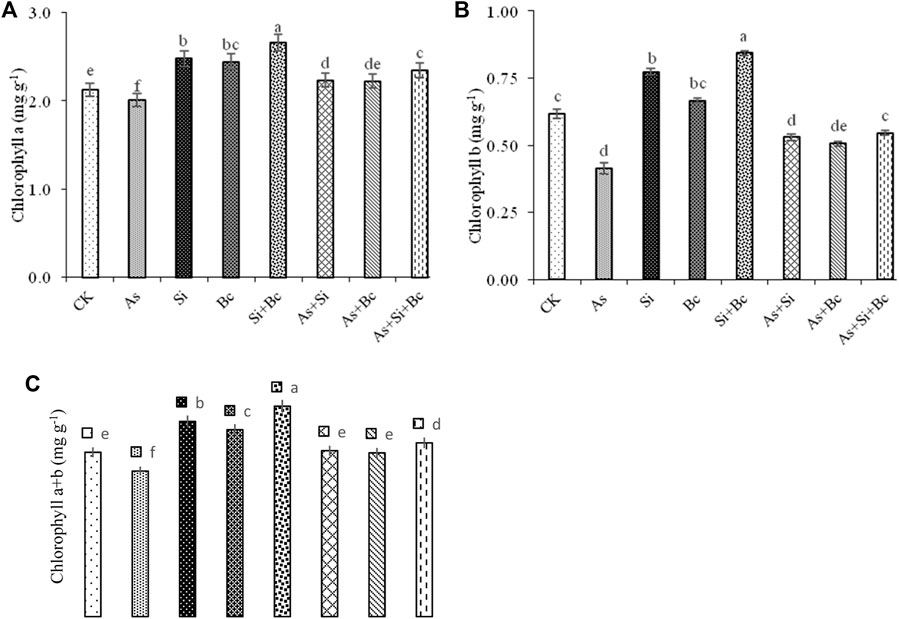
FIGURE 1. Effect of sole and combined applications of silicon and biochar on chlorophyll a (A), chlorophyll b (B), and chlorophyll a + b (C) of maize under As toxicity. Bars sharing the same letter do not have a significant (p < 0.05) difference. Error bars show the standard error of mean.
Primary metabolites accumulation
Arsenic stress significantly reduced the metabolites in maize leaves. The results revealed a significant difference (p < 0.05) among the treatments for organic osmolytes production (Figure 2). As toxicity reduced total soluble proteins by 54.93%, free amino acids by 38.59%, and total phenolic contents by 20.22% when compared with the control treatment. The application of Si enhanced total soluble proteins by 24.79%, free amino acids by 24.85%, total soluble sugars by 39.77%, and total phenolic contents by 25.88% under As toxicity. While biochar application on As-contaminated plants improved total soluble proteins by 26.76%, free amino acids by 26.50%, total soluble sugars by 46.95%, and total phenolic contents by 27.93%. Moreover, the combination of Si + biochar improved total soluble proteins by 38.21%, free amino acids by 38.13%, total soluble sugars by 50.90%, and total phenolic contents by 32.36%. However, in the non–As-contaminated treatment, the sole application of Si improved total soluble proteins by 2.5%, free amino acids by 2.6%, total soluble sugars by 7.79%, and total phenolic contents by 16.29%, while sole biochar application enhanced total soluble proteins by 10.62%, free amino acids by 10.60%, total soluble sugars by 28.57%, and total phenolic contents by 30.50% in non-contaminated plants. The combined application of Si and biochar improved total soluble proteins by 10.17%, free amino acids by 10.19%, total soluble sugars by 33.01%, and total phenolic contents by 29.38% when compared with control conditions (Figure 2).
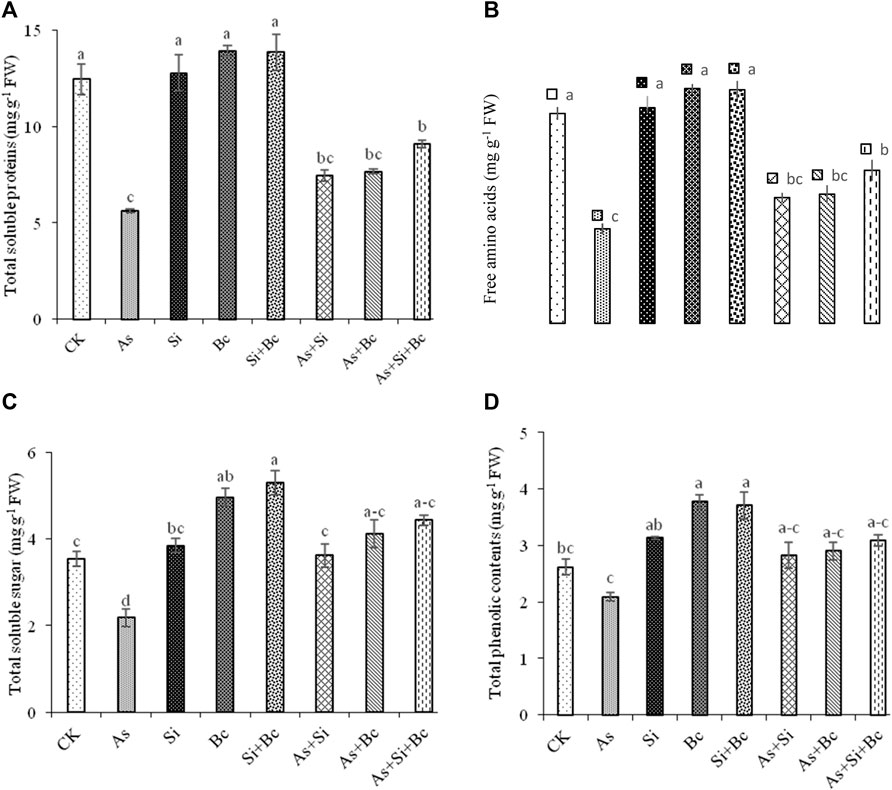
FIGURE 2. Effect of sole and combined applications of silicon and biochar on total soluble proteins (A), free amino acids (B), total soluble sugars (C), and total phenolic contents (D) of maize under As toxicity. Bars sharing the same letter do not have a significant (p < 0.05) difference. Error bars show the standard error of mean.
Enzymatic antioxidants activities
The results revealed a significant difference (p < 0.05) among the treatments for all the antioxidant enzymatic activities (Figure 3). It was observed that As stress significantly enhanced the antioxidants activities, i.e., 43.51% SOD, 47.93% POD, 47.98% CAT, and 59.02% APX in leaves of maize plants when compared with the control treatment (no As, no Si, and no biochar). When Si was applied in As-stressed plants, it increased SOD by 28.56%, POD by 20.14%, CAT by 19.14%, and APX by 31.25% when compared to sole As treatment. While the sole application of biochar on As-contaminated plants increased the antioxidant activities (SOD, POD, CAT, and APX) by 20.44, 16.91, 12.78, and 20%, respectively, and the combined application of Si and biochar significantly enhanced the antioxidant activities (SOD, POD, CAT, and APX) by 34.72, 23.12, 24.49, and 35.29%, respectively, when compared to As stress treatment. On non–As-contaminated plants, the application of Si enhanced the activities of SOD by 18.36%, POD by 28.39%, and APX by 14.5%, while biochar enhanced the activities of SOD by 22.58%, POD by 27.21%, CAT by 9.76%, and APX by 15.29% when compared with the control treatment. However, the combination of As with Si enhanced the antioxidant activities (SOD, POD, CAT, and APX) by 28.56, 20.14, 19.14, and 31.25%, respectively (Figure 3).
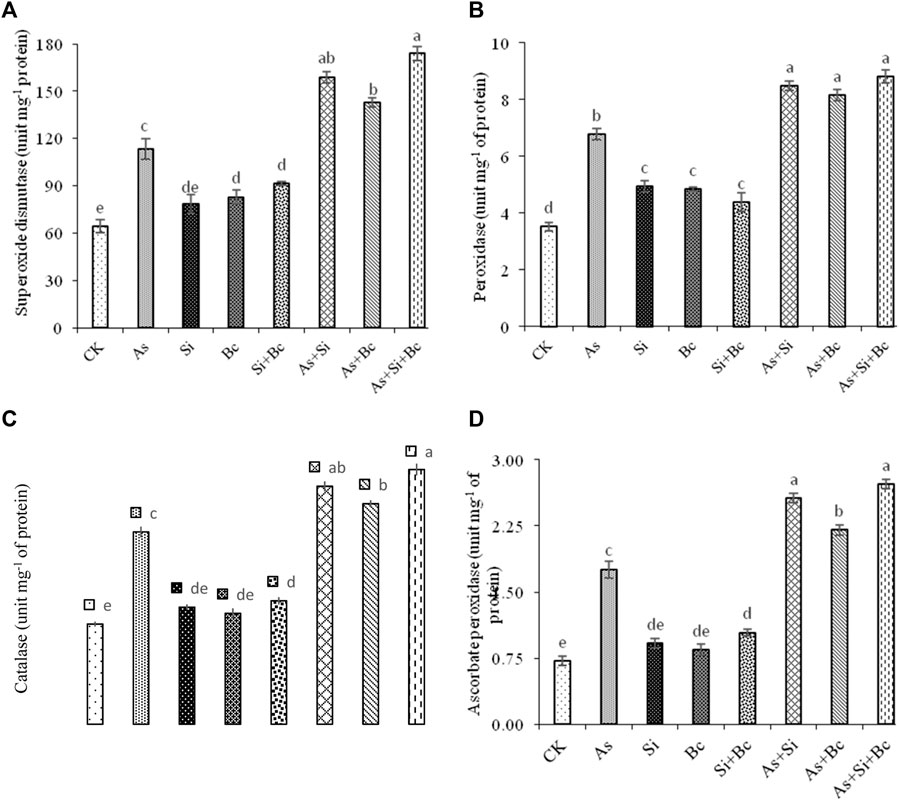
FIGURE 3. Effect of sole and combined application of silicon and biochar on superoxidase dismutase (A), peroxidase (B), catalase (C), and ascorbate peroxidase (D) of maize under As toxicity. Bars sharing the same letter do not have a significant (p < 0.05) difference. Error bars show the standard error of mean.
Lipid peroxidation and free proline
The results reveal that application of Si and biochar and their combination significantly (p < 0.05) improved the As tolerance in maize plants due to their lower malondialdehyde (MDA), hydrogen peroxide (H2O2), and electrolytes leakage (Figure 4). In As-contaminated plants, the sole application of Si reduced MDA contents by 49.24%, H2O2 by 53.13%, and electrolyte leakage by 40.86%. While biochar application in As-treated plants reduced MDA by 38.99%, H2O2 by 28.05%, and electrolyte leakage by 29.94%. The combined application of Si + biochar reduced MDA by 82.54%, H2O2 by 74.41%, and electrolyte leakage by 69.57% in As-treated plants. However, in controlled conditions, Si application reduced H2O2 by 1.48%, MDA by 13.80%, and electrolyte leakage by 32.97%. Likewise, biochar reduced H2O2 by 4.88%, MDA by 31.73%, and electrolyte leakage by 24.22%, while the combination of Si + biochar reduced H2O2 by 4.57%, MDA by 10.32%, and electrolyte leakage by 42.00% when compared with the control environment (Figure 4).
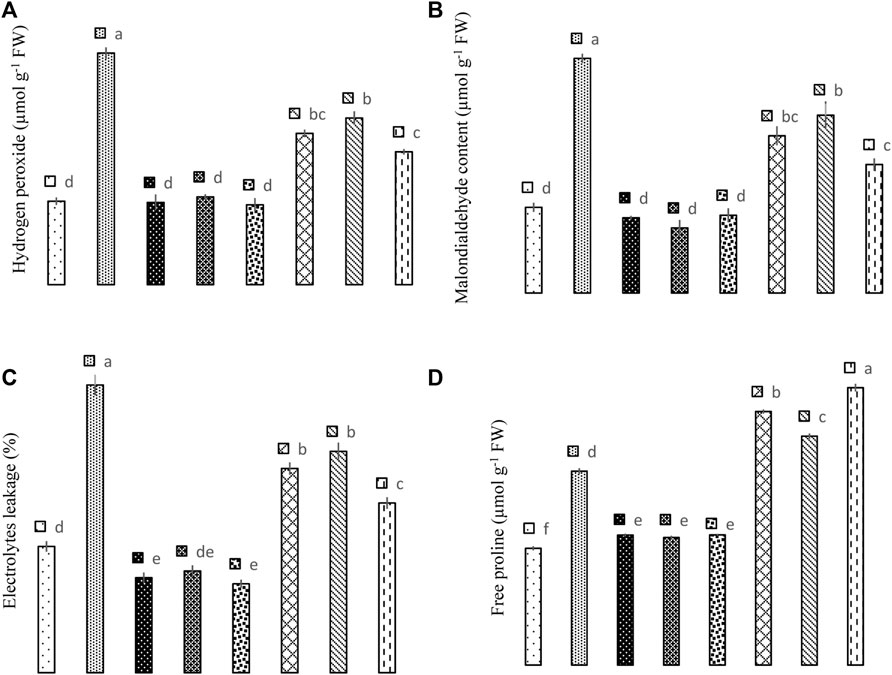
FIGURE 4. Effect of sole and combined application of silicon and biochar on hydrogen peroxide (A), malondialdehyde content (B), electrolytes leakage (C), and free proline (D) of maize under As toxicity. Bars sharing the same letter do not have a significant (p < 0.05) difference. Error bars show the standard error of mean.
Silicon and arsenic accumulation in shoots and grains
It was observed that when As stress was imposed on maize plants, it significantly (p < 0.05) reduced Si contents in shoots of maize by 33.67%. However, when Si was applied to As-treated plants, the Si contents increased up to 52.54%, while biochar application to As-treated plants enhanced the Si activity by 33.42%. The combined application of Si + biochar enhanced the Si contents by 53.70% in shoots of maize contaminated with As. Under control conditions, Si application enhanced the Si contents in shoots by 37.77%, while biochar application enhanced the Si contents in shoots by 5.15%. Moreover, the combination of Si and biochar increased the Si contents by 31.84% in shoots of maize. Arsenic stress enhanced the As concentration by 68.42% in shoots and by 68.96% in grains of maize. When Si was applied to As-contaminated plants, it reduced the As concentration by 40.74% in shoots and by 93.33% in grains of maize. While biochar application to As-contaminated plants reduced the As concentration by 31.03% in shoots and by 70.58% in grains of maize. The combination of Si + biochar reduced the As concentration by 68.88% in shoots and by 141.66% in grains of maize (Figure 5).
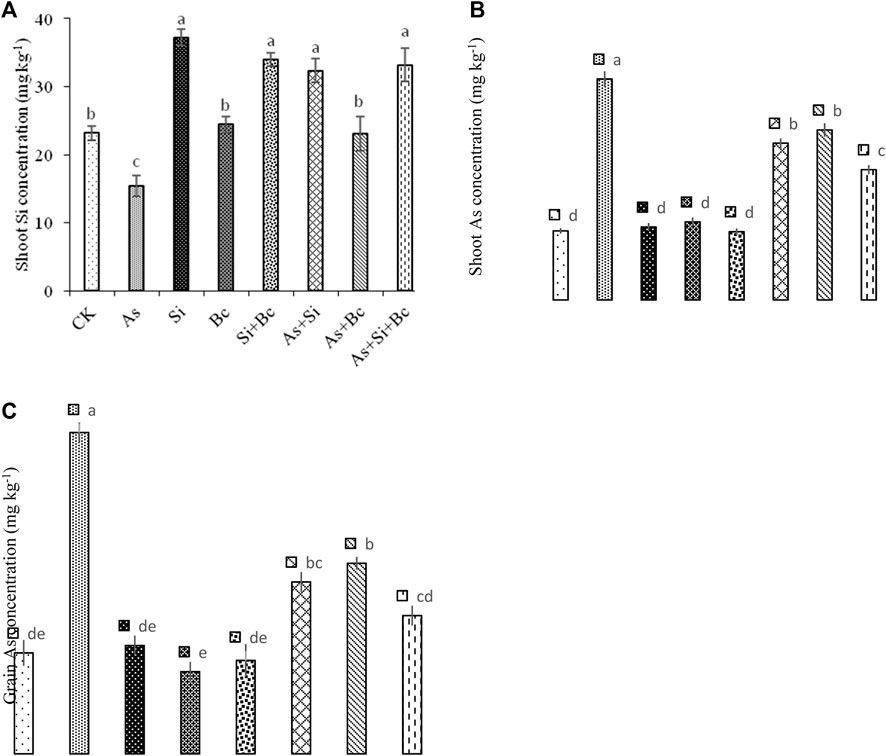
FIGURE 5. Effect of sole and combined application of silicon and biochar on shoot Si concentration (A), shoot As concentration (B), and grain As concentration (C) of maize under As toxicity. Bars sharing the same letter do not have a significant (p < 0.05) difference. Error bars show the standard error of mean.
Discussion
Silicon is the second most abundant element on Earth, and it is important for the alleviation of As toxicity as it shares the same uptake pathway with As (Vaculík et al., 2020; Koleva et al., 2022). Moreover, the interaction of biochar with Si is also important as biochar increases the pH of the soil (Kim et al., 2018), and higher pH facilitates the uptake of Si (Sirisuntornlak et al., 2021). Therefore, the current study aimed to investigate the interactive effect of Si and biochar on the growth, physiology, and productivity of maize grown under As-contaminated conditions. Arsenic accumulation in leaves deteriorates the green pigments and reduces iron uptake (Anjum et al., 2017; Mehmood et al., 2017; Zhou et al., 2020), which in return reduces the photosynthetic efficiency and ultimately hinders plant growth and development.
Effect of silicon and biochar on morphological and yield attributes under arsenic toxicity
Reduction in the growth and yield of maize due to As toxicity can be attributed to an increase in the production of ROS and higher electrolyte leakage (Figure 3). The combination of biochar and Si improved the chlorophyll pigments, organic osmolytes, and the antioxidant defense mechanism (Figures 1–3; Kashif et al., 2021; Alam et al., 2019; Abbas et al., 2018), which could have also contributed toward better yield attributes. For some attributes (plant height, leaf area, and cob length), differences were statistically on par for sole applications of Si and biochar and their combinations under As toxicity; nevertheless, for 100-grain weight and grain yield, the combination of Si and biochar performed better than their sole applications.
Effect of silicon and biochar on physiological and biochemical attributes under arsenic toxicity
In the current study, Si and biochar improved chlorophyll contents under both control and As toxicity conditions, indicating a lower As accumulation in leaves. Moreover, As toxicity promotes the production of ROS and reduces osmolyte production, which has detrimental effects on the physiology and enzymatic activities of plants (Abbas et al., 2018; Alam et al., 2019). In the current study, As toxicity reduced the production of osmolytes such as free amino acids, proline, total soluble sugars, and total soluble proteins (Figure 2) and enhanced the production of ROS and electrolyte leakage (Figure 4). Plants have an inbuilt defense mechanism for the production of antioxidant enzymes, which neutralizes the toxic effects of external elements. It has been reported in separate studies that biochar and Si improve the antioxidant defense mechanism and production of osmolytes under abiotic stress conditions (Ahmad et al., 2021; Kashif et al., 2021). Kashif et al. (2021) reported that Si application of 100 mg kg−1 soil alleviated As toxicity in maize seedlings by improving the antioxidant defense mechanism and production of osmolytes. These findings strengthen our results where biochar and Si improved the production of antioxidants and osmolytes (Figures 2 and 3) which might have reduced the production of ROS and electrolyte leakage (Figure 4) and improved plant growth and development. Pandey et al. (2016) have reported that the application of Si improved root traits and reduced the production of H2O2 and MDA in mustard (Brassica juncea L.) grown under As-contaminated conditions.
Silicon is an important nutrient that alleviates abiotic stress in plants by improving the physiochemical processes of the plant. In the current study, the interactive effect of Si and biochar improved crop productivity relative to the sole application of either Si or biochar. This interactive effect is attributed to heavy metal detoxification by biochar (Zhou et al., 2022) on the one hand and improvement in bioavailability of Si (Li et al., 2019) on the other hand, both of which are beneficial for crop productivity, thus increasing productivity and reducing the detrimental effects of As.
Effect of silicon and biochar on uptake of arsenic
The presence of Si in the soil reduces the uptake of heavy metals, and its presence in plants alleviates toxicity. Various methods have been implied to reduce As uptake by plants, out of which the use of inorganic nutrients which can compete with As uptake/contents is the most viable (Bakhat et al., 2017; Kim et al., 2021). Our results depicted that Si application decreases As uptake, which could be attributed to competition for similar transport channels (Lsi1 and Lsi2) between Si and As (Ma and Yamaji, 2006; Bogdan and Schenk, 2009; Fleck et al., 2013). Bogdan and Schenk (2009) found a significant inverse relationship between As and Si uptake in rice. Fleck et al. (2013) reported that Si reduces the uptake of As (III) in rice plants, which results in a lower concentration of As (III) in rice grains.
Biochar is an organic amendment that improves the nutrient status of the soil. Liu et al. (2014) conducted a comprehensive experiment across multiple locations and demonstrated that soil amendment with biochar improves Si uptake by increasing the pH of the soil. Although uptake of As increases with increase in pH (Marin et al., 1993; Dai et al., 2019), some researchers have also reported an increase in As uptake with the application of biochar (Brennan et al., 2014). On the contrary, this study has shown that biochar application increases Si uptake and reduces As contents (Figure 5). At the molecular level, it has been found that the combination of Si and biochar (Si-rich biochar) downregulates the expression of Si-As transporter genes (Wang et al., 2019, 2020), which in return reduces the uptake of As, as both share the same transport channel. Therefore, it may be beneficial to use Si with biochar in As-contaminated soils to minimize the root transport of As and its translocation to above-ground plant parts. Zama et al. (2018) reported that Si-rich biochar had decreased As accumulation in spinach leaves by 37% and improved dry matter yield by 67% when compared with control, and an increase in dry matter yield was correlated with Si uptake. Similar findings have also been reported in peas and turnips (Khan et al., 2015; Alam et al., 2020). Thus, for sustainable yield and to reduce As uptake in As-contaminated soils, the combined application of Si and biochar has proved to be more useful.
Conclusion
The interactive effect of Si and biochar alleviated the toxic effects of As by improving the chlorophyll pigments and production of more organic osmolytes, while enhancing the antioxidant enzymatic defense mechanism. The interactive effect of Si and biochar attributed to the competition of Si uptake with As, enhancement of Si availability by biochar, and reduction in uptake of As by biochar, all of which are evident from shoot As and Si contents. As there are only limited studies those depict alleviation of As toxicity along with improved grain yield of maize with combined application of Si and biochar, commercial recommendations may only be made after extensive field studies across different agroecological regions.
Data availability statement
The original contributions presented in the study are included in the article/Supplementary Material; further inquiries can be directed to the corresponding authors.
Author contributions
All authors listed have made a substantial, direct, and intellectual contribution to the work and approved it for publication.
Funding
The authors would like to thank the Deanship of scientific research at Umm Al-Qura University for supporting this work by grant code (22UQU4290565DSR57).
Acknowledgments
The author would like to thank the Deanship of Scientific Research at Shaqra University for supporting this work. The authors thank the Deanship of scientific research at Umm Al-Qura University for supporting this work by grant code (22UQU4290565DSR57). The authors would also like to acknowledge Alexandria University, Alexandria, Egypt for supporting the current research.
Conflict of interest
The authors declare that the research was conducted in the absence of any commercial or financial relationships that could be construed as a potential conflict of interest.
Publisher’s note
All claims expressed in this article are solely those of the authors and do not necessarily represent those of their affiliated organizations, or those of the publisher, editors, and reviewers. Any product that may be evaluated in this article, or claim that may be made by its manufacturer, is not guaranteed or endorsed by the publisher.
References
Abbas, G., Murtaza, B., Bibi, I., Shahid, M., Niazi, N. K., Khan, M. I., et al. (2018). Arsenic uptake, toxicity, detoxification, and speciation in plants: Physiological, biochemical, and molecular aspects. Int. J. Environ. Res. Public Health 15 (1), 59. doi:10.3390/ijerph15010059
Abdul, A. (2020). “Protecting rice grains from arsenic toxicity through cultural management: Bangladesh perspective,” in Protecting rice grains in the post-genomic era. Editor Y. JiaIn (London, SW7 2QJ, UK: Intech Open Limited Princes Gate Court).
Abdul, K. S. M., Jayasinghe, S. S., Chandana, E. P., Jayasumana, C., and De Silva, P. M. C. (2015). Arsenic and human health effects: A review. Environ. Toxicol. Pharmacol. 40 (3), 828–846. doi:10.1016/j.etap.2015.09.016
Ahmad, A., Khan, W. U., Shah, A. A., Yasin, N. A., Naz, S., Ali, A., et al. (2021). Synergistic effects of nitric oxide and silicon on promoting plant growth, oxidative stress tolerance and reduction of arsenic uptake in Brassica juncea. Chemosphere 262, 128384. doi:10.1016/j.chemosphere.2020.128384
Alam, M. Z., Hoque, M. A., and Carpenter-Boggs, L. (2020). Identification of practical amendments to mitigate soil arsenic levels in peas. Rhizo 16, 100268. doi:10.1016/j.rhisph.2020.100268
Alam, M. Z., McGee, R., Hoque, M., Ahammed, G. J., and Carpenter-Boggs, L. (2019). Effect of arbuscularmycorrhizal fungi, selenium and biochar on photosynthetic pigments and antioxidant enzyme activity under arsenic stress in mung bean (Vigna radiata). Front. Physiol. 10, 193. doi:10.3389/fphys.2019.00193
Anjum, S. A., Tanveer, M., Hussain, S., Ashraf, U., Khan, I., and Wang, L. (2017). Alteration in growth, leaf gas exchange, and photosynthetic pigments of maize plants under combined cadmium and arsenic stress. Water Air Soil Pollut. 228, 13. doi:10.1007/s11270-016-3187-2
Awasthi, S., Chauhan, R., Srivastava, S., and Tripathi, R. D. (2017). The journey of arsenic from soil to grain in rice. Front. Plant Sci. 8, 1007. doi:10.3389/fpls.2017.01007
Bakhat, H. F., Zia, Z., Fahad, S., Abbas, S., Hammad, H. M., Shahzad, A. N., et al. (2017). Arsenic uptake, accumulation and toxicity in rice plants: Possible remedies for its detoxification: A review. Environ. Sci. Pollut. Res. 24 (10), 9142–9158. doi:10.1007/s11356-017-8462-2
Bates, L. S., Waldren, R. P., and Teare, I. D. (1973). Rapid determination of free proline for water-stress studies. Plant Soil 39, 205–207. doi:10.1007/bf00018060
Bianucci, E., Peralta, J. M., Furlan, A., Hernández, L. E., and Castro, S. (2020). Arsenic in wheat, maize, and other crops. Arsenic Drink. Water Food, 279–306. doi:10.1007/978-981-13-8587-2_9
Bienert, G. P., Thorsen, M., Schüssler, M. D., Nilsson, H. R., Wagner, A., Tamás, M. J., et al. (2008). A subgroup of plant aquaporins facilitate the bi-directional diffusion of As(OH) 3 and Sb(OH) 3 across membranes. BMC Biol. 6, 26. doi:10.1186/1741-7007-6-26
Bogdan, K., and Schenk, M. K. (2009). Evaluation of soil characteristics potentially affecting arsenic concentration in paddy rice (Oryza sativa L.). Environ. Pollut. 157, 2617–2621. doi:10.1016/j.envpol.2009.05.008
Bradford, M. M. (1976). A rapid and sensitive method for the quantitation of microgram quantities of protein utilizing the principle of protein-dye binding. Anal. Biochem. 72, 248–254. doi:10.1016/0003-2697(76)90527-3
Brennan, A., Jiménez, E. M., Puschenreiter, M., Alburquerque, J. A., and Switzer, C. (2014). Effects of biochar amendment on root traits and contaminant availability of maize plants in a copper and arsenic impacted soil. Plant Soil 379 (1), 351–360. doi:10.1007/s11104-014-2074-0
Bukhari, M. A., Ahmad, Z., Ashraf, M. Y., Afzal, M., Nawaz, F., Nafees, M., et al. (2020). Silicon mitigates drought stress in wheat (Triticum aestivum L.) through improving photosynthetic pigments, biochemical and yield characters. Silicon, 1–16. doi:10.1007/s12633-020-00797-4
Chance, B., and Maehly, A. C. (1955). Assay of catalase and peroxidase. Methods Enzymol. 2, 764–775. doi:10.1016/S0076-6879(55)02300-8
Dai, Y., Xu, W., Nasir, M., Zhang, Y., and Lyu, J. (2019). Reliable model established depending on soil properties to assess arsenic uptake by Brassica chinensis. Ecotoxicol. Environ. Saf. 167, 54–59. doi:10.1016/j.ecoenv.2018.09.088
Dionisio-Sese, M. L., and Tobita, S. (1998). Antioxidant responses of rice seedlings to salinity stress. Plant Sci. 135 (1), 1–9. doi:10.1016/s0168-9452(98)00025-9
Du, L., Xia, X., Lan, X., Xiping, L., Miao, L., Liyang, Z., et al. (2017). Influence of arsenic stress on physiological, biochemical, and morphological characteristics in seedlings of two cultivars of maize (Zea mays L.). Water Air Soil Pollut. 228, 55. doi:10.1007/s11270-016-3231-2
Farooq, S. H., Chandrasekharam, D., Dhanachandra, W., and Ram, K. (2019). Relationship of arsenic accumulation with irrigation practices and crop type in agriculture soils of Bengal Delta, India. Appl. Water Sci. 9, 119. doi:10.1007/s13201-019-0904-1
Fleck, A. T., Mattusch, J., and Schenk, M. K. (2013). Silicon decreases the arsenic level in rice grain by limiting arsenite transport. Z. Pflanzenernahr. Bodenk. 176 (5), 785–794. doi:10.1002/jpln.201200440
Gao, X., Peng, Y., Zhou, Y., Adeel, M., and Chen, Q. (2019). Effects of magnesium ferrite biochar on the cadmium passivation in acidic soil and bioavailability for packoi (Brassica chinensis L.). J. Environ. Manag. 251, 109610. doi:10.1016/j.jenvman.2019.109610
Giannopolitis, C. N., and Ries, S. K. (1977). Superoxide dismutases: I. Occurrence in higher plants. Plant Physiol. 59 (2), 309–314. doi:10.1104/pp.59.2.309
Hammad, H. M., Chawla, M. S., Jawad, R., Alhuqail, A., Bakhat, H. F., Farhad, W., et al. (2022). Evaluating the impact of nitrogen application on growth and productivity of maize under control conditions. Front. Plant Sci. 13, 885479. doi:10.3389/fpls.2022.885479
Hamilton, P. B., and Van Slyke, D. D. (1943). Amino acid determination with ninhydrin. J. Biol. Chem. 150, 231–250. doi:10.1016/s0021-9258(18)51268-0
Haseeb, M., Iqbal, S., Hafeez, M. B., Saddiq, M. S., Zahra, N., Raza, A., et al. (2022). Phytoremediation of nickel by quinoa: Morphological and physiological response. Plos one 17 (1), e0262309. doi:10.1371/journal.pone.0262309
Hodges, D. M., DeLong, J. M., Forney, C. F., and Prange, R. K. (1999). Improving the thiobarbituric acid-reactive-substances assay for estimating lipid peroxidation in plant tissues containing anthocyanin and other interfering compounds. Planta 207 (4), 604–611. doi:10.1007/s004250050524
Hu, Y., Li, J., Lou, B., Wu, R., Wang, G., Lu, C., et al. (2020). The role of reactive oxygen species in arsenic toxicity. Biomolecules 10, 240. doi:10.3390/biom10020240
HumayunKabir, M. D. (2020).Microwave and biochar soil treatment alleviates arsenic phytotoxicity in wheat and rice. arkville, Australia: School of Agriculture and Food, Faculty of Veterinary and Agricultural Sciences The University of Melbourne. PHD Thesis.
Ibrahim, M., Khan, S., Hao, X., and Li, G. (2016). Biochar effects on metal bioaccumulation and arsenic speciation in alfalfa (Medicago sativa L.) grown in contaminated soil. Int. J. Environ. Sci. Technol. 13 (10), 2467–2474. doi:10.1007/s13762-016-1081-5
Javad, S., Shah, A. A., Ramzan, M., Sardar, R., Javed, T., Al‐Huqail, A. A., et al. (2022). Hydrogen sulphide alleviates cadmium stress in Trigonella foenum‐graecum by modulating antioxidant enzymes and polyamine content. Plant Biol. (Stuttg). 24, 618–626. doi:10.1111/plb.13393
Julkenen-Titto, R. (1985). Phenolic constituents in the leaves of northern willows: Methods for the analysis of certain phenolics. J. Agric. Food Chem. 33, 213–217. doi:10.1021/jf00062a013
Kabir, A. H., Hossain, M. M., Khatun, M. A., Mandal, A., and Haider, S. A. (2016). Role of silicon counteracting cadmium toxicity in Alfalfa (Medicago sativa L.). Front. Plant Sci. 7, 1117. doi:10.3389/fpls.2016.01117
Kashif, M., Sattar, A., Ul-Allah, S., Sher, A., Ijaz, M., Butt, M., et al. (2021). Silicon alleviates arsenic toxicity in maize seedlings by regulating physiological and antioxidant defense mechanisms. J. Soil Sci. Plant Nutr. 21, 2032–2040. doi:10.1007/s42729-021-00499-9
Kertulis-Tartar, G. M., Rathinasabapathi, B., and Ma, L. Q. (2009). Characterization of glutathione reductase and catalase in the fronds of two Pteris ferns upon arsenic exposure. Plant Physiol. biochem. 47 (10), 960–965. doi:10.1016/j.plaphy.2009.05.009
Khan, S., Waqas, M., Ding, F., Shamshad, I., Arp, H. P. H., and Li, G. (2015). The influence of various biochars on the bioaccessibility and bioaccumulation of PAHs and potentially toxic elements to turnips (Brassica rapa L.). J. Hazard. Mat. 300, 243–253. doi:10.1016/j.jhazmat.2015.06.050
Kim, H., Kim, J., Kim, M., Hyun, S., and Moon, D. H. (2018). Sorption of sulfathiazole in the soil treated with giant miscanthus-derived biochar: Effect of biochar pyrolysis temperature, soil pH, and aging period. Environ. Sci. Pollut. Res. 25 (26), 25681–25689. doi:10.1007/s11356-017-9049-7
Kim, M. S., Lee, S. H., and Kim, J. G. (2021). Evaluation of factors affecting arsenic uptake by Brassica juncea in alkali soil after biochar application using partial least squares path modeling (PLS-PM). Chemosphere 275, 130095. doi:10.1016/j.chemosphere.2021.130095
Koleva, L., Umar, A., Yasin, N. A., Shah, A. A., Siddiqui, M. H., Alamri, S., et al. (2022). Iron oxide and silicon nanoparticles modulate mineral nutrient homeostasis and metabolism in cadmium-stressed Phaseolus vulgaris. Front. Plant Sci. 13, 806781. doi:10.3389/fpls.2022.806781
Kumar, A., and Bhattacharya, T. (2020). Biochar: A sustainable solution. Environ. Dev. Sustain. 23, 6642–6680. doi:10.1007/s10668-020-00970-0
Li, Z., Unzué-Belmonte, D., Cornelis, J. T., Linden, C. V., Struyf, E., Ronsse, F., et al. (2019). Effects of phytolithic rice-straw biochar, soil buffering capacity and pH on silicon bioavailability. Plant Soil 438 (1), 187–203. doi:10.1007/s11104-019-04013-0
Lichtenthaler, H. K. (1987). “Chlorophyll and carotenoids: Pigments of photosynthetic biomembranes,” in Methods in enzymology. Editors L. Paker, and R. Douce (Boca Raton: CRC Press), 350–382.
Liu, X., Li, L., Bian, R., Chen, D., Qu, J., WanjiruKibue, G., et al. (2014). Effect of biochar amendment on soil‐silicon availability and rice uptake. Z. Pflanzenernahr. Bodenk. 177 (1), 91–96. doi:10.1002/jpln.201200582
Ma, J. F., Yamaji, N., Mitani, N., Xu, X. Y., Su, Y. H., Mcgrath, S. P., et al. (2008). Transporters of arsenite in rice and their role in arsenic accumulation in rice grain. Proc. Natl. Acad. Sci. U. S. A. 105, 9931–9935. doi:10.1073/pnas.0802361105
Ma, J. F., and Yamaji, N. (2006). Silicon uptake and accumulation in higher plants. Trends Plant Sci. 11, 392–397. doi:10.1016/j.tplants.2006.06.007
Malhotra, C., and Kapoor, R. T. (2019). “Silicon: A sustainable tool in abiotic stress tolerance in plants,” in Plant abiotic stress tolerance (Berlin, Germany: Springer). doi:10.1007/978-3-030-06118-0_14
Marin, A. R., Masscheleyn, P. H., and Patrick, W. H. (1993). Soil redox-pH stability of arsenic species and its influence on arsenic uptake by rice. Plant Soil 152 (2), 245–253. doi:10.1007/bf00029094
Mehmood, S., Rizwan, M., Bashir, S., Ditta, A., Aziz, O., Yong, L. Z., et al. (2018). Comparative effects of biochar, slag and ferrous–Mn ore on lead and cadmium immobilization in soil. Bull. Environ. Contam. Toxicol. 100 (2), 286–292. doi:10.1007/s00128-017-2222-3
Mehmood, T., Bibi, I., Shahid, M., Niazi, N. K., Murtaza, B., Wang, H., et al. (2017). Effect of compost addition on arsenic uptake, morphological and physiological attributes of maize plants grown in contrasting soils. J. Geochem. Explor. 178, 83–91. doi:10.1016/j.gexplo.2017.03.018
Muhammad, K., Ma, M. G., Cao, Y., Mao, H., Ma, X., and Ma, W. (2019). Metal–organic frameworks‐derived mesoporous Si/SiOx@NCNanospheres as a long‐lifespan anode material for lithium‐ion batteries. Chemistry 25, 11991–11997. doi:10.1002/chem.201903043
Muhammad, I. A., Shah, A. N., Sun, J., and Song, Y. (2020). Comparative study on leaf gas exchange, growth, grain yield, and water use efficiency under irrigation regimes for two maize hybrids. Agriculture Basel. doi:10.3390/agriculture10090369
Naeem, M. A., Shabbir, A., Amjad, M., Abbas, G., Imaran, M., Murtaza, B., et al. (2020). Acid treated biochar enhances cadmium tolerance by restricting its uptake and physio-chemical attributes in quinoa (Chenopodim quinoa Wild) Ecotocicol. Ecotoxicol. Environ. Saf. 191, 110218. doi:10.1016/j.ecoenv.2020.110218
Ning, D., Liang, Y., Liu, Z., Xiao, J., and Duan, A. (2016). Impacts of steel-slag-based silicate fertilizer on soil acidity and silicon availability and metals-immobilization in a paddy soil. PLoS ONE 11, e0168163. doi:10.1371/journal.pone.0168163
Qayyum, M. F., Abid, M., and Danish, S. (2015). Effects of various biochars on seed germination and carbon mineralization in an alkaline soil. Pak. J. Agric. Sci. 51, 977–982.
Rosas-Castor, J. M., Guzmán-Mar, J. L., Hernández-Ramírez, A., Garza-González, M. T., and Hinojosa-Reyes, L. (2014). Arsenic accumulation in maize crop (Zea mays): A review. Sci. Total Environ. 488, 176–187. doi:10.1016/j.scitotenv.2014.04.075
Sattar, A., Sher, A., Ijaz, M., Ul-Allah, S., Butt, M., Irfan, M., et al. (2020). Interactive effect of biochar and silicon on improving morpho-physiological and biochemical attributes of maize by reducing drought hazards. J. Soil Sci. Plant Nutr. 20 (4), 1819–1826. doi:10.1007/s42729-020-00253-7
Shah, A. N., Tanveer, M., Abbas, A., Yildirim, M., Shah, A. A., Ahmad, M. I., et al. (2021). Combating dual challenges in maize under high planting density: Kernel abortion and stem lodging. Front. Plant Sci. 12, 699085. doi:10.3389/fpls.2021.699085
Shrivastava, A., Barla, A., Majumdar, A., Singh, S., and Bose, S. (2019). Arsenic mitigation in rice grain loading via alternative irrigation by proposed water management practices. Chemosphere 238, 124988. doi:10.1016/j.chemosphere.2019.124988
Sirisuntornlak, N., Ullah, H., Sonjaroon, W., Anusontpornperm, S., Arirob, W., and Datta, A. (2021). Interactive effects of silicon and soil pH on growth, yield and nutrient uptake of maize. Silicon 13 (2), 289–299. doi:10.1007/s12633-020-00427-z
Suriyagoda, L. D. B., Dittert, K., and Lambers, H. (2018). Mechanism of arsenic uptake, translocation and plant resistance to accumulate arsenic in rice grains. Agric. Ecosyst. Environ. 253, 23–37. doi:10.1016/j.agee.2017.10.017
Vaculík, M., Lukačová, Z., Bokor, B., Martinka, M., Tripathi, D. K., and Lux, A. (2020). Alleviation mechanisms of metal (loid) stress in plants by silicon: A review. J. Exp. Bot. 71 (21), 6744–6757. doi:10.1093/jxb/eraa288
Velikova, V., Yordanov, I., and Edreva, A. (2000). Oxidative stress and some antioxidant systems in acid rain-treated bean plants: Protective role of exogenous polyamines. Plant Sci. 151, 59–66. doi:10.1016/s0168-9452(99)00197-1
Wang, Y., Xiao, X., Xu, Y., and Chen, B. (2019). Environmental effects of silicon within biochar (sichar) and carbon–silicon coupling mechanisms: A critical review. Environ. Sci. Technol. 53 (23), 13570–13582. doi:10.1021/acs.est.9b03607
Wang, Y., Zhang, K., Lu, L., Xiao, X., and Chen, B. (2020). Novel insights into effects of silicon-rich biochar (Sichar) amendment on cadmium uptake, translocation and accumulation in rice plants. Environ. Pollut. 265, 114772. doi:10.1016/j.envpol.2020.114772
Yemm, E. W., and Willis, A. J. (1954). The estimation of carbohydrates in plant extracts by anthrone. Biochem. J. 57, 508–514. doi:10.1042/bj0570508
Zama, E. F., Reid, B. J., Sun, G. X., Yuan, H. Y., Li, X. M., and Zhu, Y. G. (2018). Silicon (Si) biochar for the mitigation of arsenic (As) bioaccumulation in spinach (Spinaciaoleracean) and improvement in the plant growth. J. Clean. Prod. 189, 386–395. doi:10.1016/j.jclepro.2018.04.056
Zhao, F. J., and Wang, P. (2020). Arsenic and cadmium accumulation in rice and mitigation strategies. Plant Soil 446, 1–21. doi:10.1007/s11104-019-04374-6
Keywords: arsenic contamination, maize, yield, physiology, biochemical attributes
Citation: Sattar A, Sher A, Abourehab MAS, Ijaz M, Nawaz M, Ul-Allah S, Abbas T, Shah AN, Imam MS, Abdelsalam NR, Hasan ME, Abbas A and Javaid MM (2022) Application of silicon and biochar alleviates the adversities of arsenic stress in maize by triggering the morpho-physiological and antioxidant defense mechanisms. Front. Environ. Sci. 10:979049. doi: 10.3389/fenvs.2022.979049
Received: 27 June 2022; Accepted: 10 October 2022;
Published: 24 October 2022.
Edited by:
Muhammad Azeem, Institute of Urban Environment (CAS), ChinaReviewed by:
Marwa Abdelmohsen, Agricultural Research Center, EgyptAhmad Ali, Nanjing Normal University, China
Muhammad Rahil Afzal, Jiangsu University, China
Copyright © 2022 Sattar, Sher, Abourehab, Ijaz, Nawaz, Ul-Allah, Abbas, Shah, Imam, Abdelsalam, Hasan, Abbas and Javaid. This is an open-access article distributed under the terms of the Creative Commons Attribution License (CC BY). The use, distribution or reproduction in other forums is permitted, provided the original author(s) and the copyright owner(s) are credited and that the original publication in this journal is cited, in accordance with accepted academic practice. No use, distribution or reproduction is permitted which does not comply with these terms.
*Correspondence: Adnan Noor Shah, YW5zLjc4NkB5YWhvby5jb20=; Mohamed S. Imam, c29saW1hbkBzdS5lZHUuc2E=