- 1Animal Ecology, Bielefeld University, Bielefeld, Germany
- 2Ecossa, Starnberg, Germany
The accumulation of plastics in the environment is a major problem in the Anthropocene. As most plastic is produced, used and discarded on land, ∼4–23 times more plastics are deposited in soils than in the oceans. However, there is far too little knowledge on the ecological consequences of plastic pollution, especially for soil ecosystems. Microplastics (<5 mm), whether derived from larger plastic pieces through physical, chemical and biological degradation or produced as primary particles, is of considerable interest, as they can be ingested by organisms at the basis of the trophic net and transferred to higher trophic levels. Nonetheless, although the assessment of microplastic effects on soil invertebrates is of undeniable relevance, most studies have focussed on nano- and microplastics in aquatic environments. This review examines the current state of knowledge regarding the effects of microplastics on soil invertebrates. As part of the soil biota, these organisms are of utmost importance for carbon cycling, respiration and biodiversity. Based on strict quality criteria, the data of 45 papers reporting ecotoxicological effects on soil invertebrates were analyzed, considering various test organisms and types of microplastic (in terms of polymer, shape and size). However, although different impacts were demonstrated, a deduction of general effect tendencies of microplastics in soils was difficult due to the scarcity of data and the use of diverse methodological setups. Moreover, almost all experiments were based on short-term single-species testing involving only a small number of species and single microplastic types. The review concludes with a discussion of the remaining knowledge gap and the needs for a standardized approach allowing an ecologically relevant risk assessment of the impacts of microplastic on invertebrates in terrestrial ecosystems.
1 Introduction
Environmental contamination with plastic has rapidly become a major problem of global proportion. The mass production of plastics started in the 1940s and has steadily increased (Thompson et al., 2009a; Cole et al., 2011; Hohn et al., 2020; PlasticsEurope, 2020), given the numerous desirable features of this material, including its low-cost, durability, lightweight and resistance to biodegradation. However, the recalcitrance of plastic has led to its accumulation in the environment and therefore its environmental risk (Thompson et al., 2005; Gautam et al., 2007; Shah et al., 2008; Barnes et al., 2009; Thompson et al., 2009b; Imhof et al., 2013). In 2020, ∼367 million tonnes of plastics were produced globally (PlasticsEurope, 2021). About 54% of anthropogenic waste discarded into the environment consists of plastic (Hoellein et al., 2014). In the environment plastics can be found in different particle sizes: macroplastic (>25 mm), mesoplastic (5–25 mm), microplastic (<5 mm) and nanoplastics (<100 nm) (Zalasiewicz et al., 2016; Horton et al., 2017; Alimi et al., 2018). The focus of this study is microplastics, which are highly abundant in the environment, consist of a wide range of polymers (e.g. polyethylene, polystyrene, polypropylene) and forming various shapes (e.g. beads, fragments, fibers or films) (He et al., 2018). Microplastics are also defined according to their source: Primary microplastics are plastic particles produced directly for commercial or industrial use and most commonly consists of polypropylene, polyethylene and polystyrene (Horton et al., 2017; Helmberger et al., 2020). Secondary microplastics emerge through the physical, chemical and biological degradation of macroplastics (Thompson et al., 2005; Ryan et al., 2009; Gewert et al., 2015). Both plastic types can reach the soil via improper waste management, tire abrasion or the application of sewage sludge and waste water on agricultural fields, which is a common practice throughout the world (Blaesing & Amelung, 2018; Li et al., 2018; Corradini et al., 2019).
Plastic production, usage and disposal mainly happen in terrestrial ecosystems rather than in oceans. It was estimated that 80% of the litter found in oceans derives from terrestrial sources (Jambeck et al., 2015). Terrestrial ecosystems have therefore a high risk of being contaminated with plastic debris, and, consequently, have to be considered as long-term sinks or at least intermediate transport pathways (Zubris & Richards, 2005; Rillig, 2012). In fact, soils contain 4–23 times more plastic than oceans (Horton et al., 2017). It is thus surprising that most studies have focussed on marine or freshwater systems rather than soil ecosystems (Andrady, 2011; Browne et al., 2011; Wright et al., 2013; Erkes-Medrano et al., 2015; He et al., 2018; Wang et al., 2019). The first study of microplastics in soil was published in 2012 (Rillig, 2012). The scarcity of studies has continued as a Web of Science query revealed that in 2021 there were 418 publications examining the effects of microplastics in the marine environment, with far fewer studies of freshwater systems (124 publications) and even fewer studies that focussed on soil (113 publications) (Figure 1). If the several false positive hits provided by the Web of science search for microplastic research in soils are excluded, a small number of 17 publications remain for the year 2021, which states a relative count of 0–3% between 2012 and 2021.
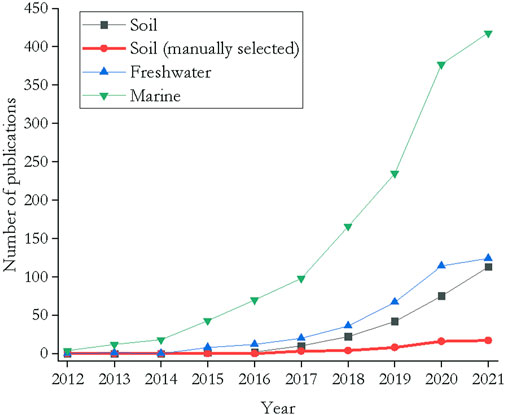
FIGURE 1. Number of studies published between 2012 and 2021 that examined the impact of micro- and nanoplastics in different environments. Search terms: for marine ecosystems (microplastic* OR nanoplastic*) AND (marine* OR sea*) AND (invertebrate* OR mesofauna* OR meiobenthos* OR organism*); for freshwater systems (microplastic* OR nanoplastic*) AND (freshwater* OR limn*) AND (invertebrate* OR mesofauna* OR meiobenthos* OR organism*); for soil (microplastic* OR nanoplastic*) AND (soil* OR terrestr*) AND (invertebrate* OR mesofauna* OR organism*). A manual selection of the hits was performed by excluding all publications that did not match the search terms (exclusion of false positives). Query performed on 12 October 2021.
Soil is proposed to provide most of the living biomass and to be one of the most biodiverse habitats, harbouring about 25% of all species on Earth. Soil organisms are key drivers for ecosystem functions and processes, such as nutrient cycling and water transfer (Bar-On et al., 2018; Bardgett & van der Putten, 2014; Bowker et al., 2010; Lavelle & Spain, 2001; Porazinska et al., 2003; Roger-Estrade et al., 2010; van den Hoogen et al., 2019). The heterotrophic soil biota are responsible for up to half of the soil respiration and soil animals including the mesofauna considerably determine decomposition rates of organic matter (Cisneros-Dozal et al., 2006; Frouz, 2018).
Arrived in the soil, plastics exert both short- and long-term impacts on soil organisms (Steinmetz et al., 2016). The ingestion of the omnipresent micro- and nanoplastic by soil invertebrates is of particular concern as these organisms form the base of the trophic net and allow the transfer of plastic particles to higher trophic levels (Duis & Coors, 2016; Carbery et al., 2018). The physical or mechanical effects of microplastics occur outside the body of the organism or after ingestion (Anbumani & Kakkar, 2018; Padervand et al., 2020). The strength of the effect was shown to inversely correlate with the plastic size, with smaller particles more likely to be ingested (da Costa et al., 2016; Horton et al., 2017). However, independent of particle size, the ecotoxicological effects of microplastics could be accurately predicted by their total surface area (shown for PS beads; Mueller et al., 2020). Furthermore, in addition to direct toxicity, indirect effects, via the interaction of microplastics with the food of the tested organisms, have been reported (e.g. Mueller et al., 2020b; Rauchschwalbe et al., 2021; Besseling et al., 2013; Wright et al., 2013; Scherer et al., 2018; Oganowski et al., 2018). Finally, the additives used in plastic production, such as plasticizers and stabilizers, can leach into the soil and accumulate in the food chain, leading to chemical effects and biomagnification (Teuten et al., 2009; Haegerbaeumer et al., 2019a; Halle et al., 2020).
The ecotoxicological effects of microplastic on soil biota have been examined in several studies but drawing conclusions from those studies is hindered by differences in experimental methods and the concentration, type, shape and color of the tested microplastics (e.g. Boots et al., 2019). In addition, detailed quantitative analyses of the data are lacking (e.g. He et al., 2018; Wang et al., 2019; Kim Y.-N. et al., 2020). Therefore, in this review we evaluated the current state of knowledge regarding the effects of microplastics on soil invertebrates. To identify future research needs, the reviewed studies were differentiated in terms of (i) the test organism and toxicity endpoints and (ii) the type, shape, size and concentration of the microplastic. By this, we could compare effect threshold concentrations for various types of plastics and organisms and provided insights into possible toxicity mechanisms.
2 Methods of the literature research
The literature search was performed to identify all published results regarding ecotoxicological impacts of microplastics on soil invertebrates. The Web of Science and Google Scholar search was executed on the 12 November 2021 using ‘Harzing’s Publish or Perish’ (Windows GUI Edition) 8.1.3625.7987 under usage of the following search term: TOPIC ((microplastic* OR nanoplastic*) AND (soil* OR terrestri*) AND (invertebrate* OR mesofauna* OR organism*)). As the first study of the effects of microplastics on soil organisms was published in 2012 (Rillig, 2012), the query was performed for the years 2012–2021. The publications in the resulting list were assessed based on several quality criteria. First, the publications within the raw list (I) were evaluated manually for their title and abstract to remove false positives (II). In the final step, the following quality criteria were applied: the use of soil as the testing medium in at least one experiment; a detailed description of type (shape and/or polymer), size and concentration of the microplastic and, if applied, the concentration of environmental chemicals (e.g. heavy metals); and the use of negative controls and statistical analyses (III). The steps comprising the literature search and the quality criteria are summarized in Figure 2.

FIGURE 2. Step-by-step procedure used in the literature search. Web of Science and Google Scholar search performed on the 12.11.2021. With Harzing’s Publish or Perish’ (Windows GUI Edition) 8.1.3625.7987.
Bar charts (OriginPro 2020 64-bit) were created to condense the general characteristics of the reviewed publications: experimental setup/soil, microplastic type/shape, the taxonomic group of the test organisms and the effect categories cellular response, (gut) microbiota, mortality, development, behavior, reproduction and community structure.
As not indicating an impact, any results regarding the ingestion, egestion, bioturbation of microplastic and the microplastic-related accumulation of other environmental chemicals were separately examined (Tables 1–3).
The effect-related results were condensed in lists (Tables 4–6) summarizing the tested species, associated taxa, the microplastic type (polymer, shape), size and concentration, the quantity and quality of the observed effects on the test organism, the corresponding author and the publication date. For clarity the effects were grouped within the above-mentioned parameter categories, whereby community structure was only physically affected, and can therefore be found in Table 4 only.
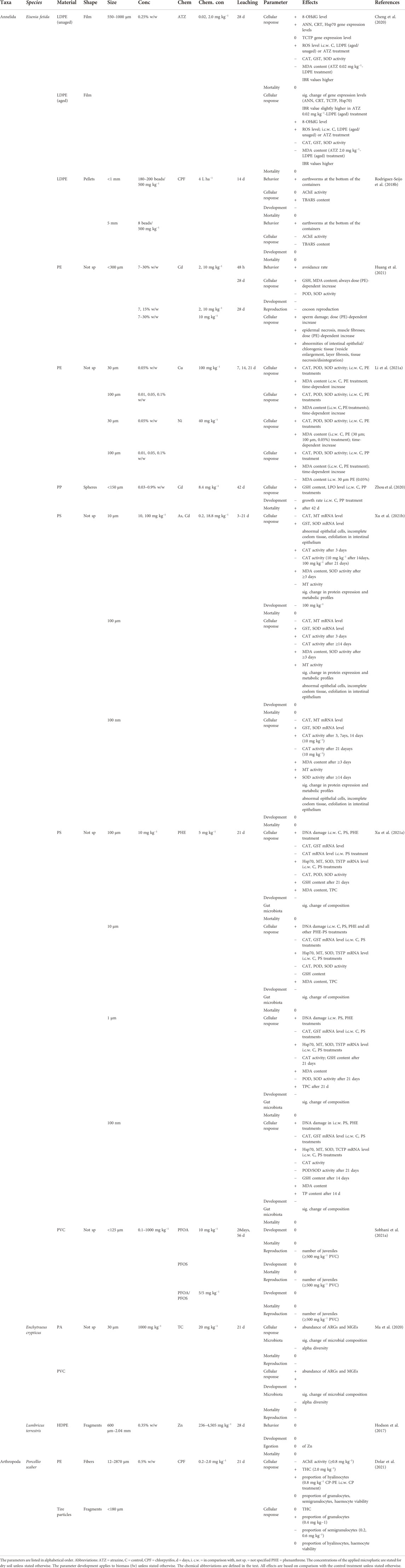
TABLE 6. Influence of microplastics on the bioavailability of environmental chemicals in soil invertebrates.
2.1 Chemical vs physical interactions
In this paper, we differentiate between chemical effects and the physical interactions of the studied organisms with microplastics. When considered together, they constitute an observed adverse effect (OAE) (according to ECETOC, 2018):
where EiT is the intrinsic toxic effect and EPInt the physical interaction.
We provide following definitions for these terms:
Physical interactions are related to the physical properties of microplastics, including size, shape, rigidity, specific density, surface area and structure. In contrast to dissolved chemicals, insoluble, particulate microplastics can interact directly with the organism to cause physical injury or congestion. Indirect impacts can also occur, such as when microplastics interact with the soil to cause food dilution (Table 4).
The adverse effects of chemicals are related to the ability of the compounds to enter the cells of organisms, interact with chemical receptors and thus cause a molecular, sub-organismic or organismic response. For microplastics, chemical effects arise from the leaching of chemicals associated with the microplastics, including additives (e.g. plasticizers or flame retardants), monomers, or compounds absorbed from the environment (e.g. polychlorinated biphenyls (PCBs), phenanthrene) (Tables 5, 6) (Teuten et al., 2009; Carbery et al., 2018).
3 Results
3.1 General characterization of the reviewed publications
From the initial raw list of 4,068 publications, 45 recently published papers (since 2017) were finally included in this literature review (Figure 2).
The publications describe experiments with exposure times between 3 min and 287 days. Physical effects of microplastics on soil invertebrates were examined in 32 of the 45 publications. Eighteen studies considered the effects of leached chemicals and environmental chemicals associated with microplastics. Overall, seven different effect endpoints were evaluated, with the most frequent being mortality, cellular response and development, analyzed in 29, 25, and 24 publications respectively, followed by reproduction and behavior (16 and 15 papers). The influence of microplastics on the invertebrate’s (gut) microbiota was examined in six papers and the effects on the structure of the soil invertebrate communities in two papers (Figure 3).
Microplastics consisting of 13 different polymers were applied, namely polyethylene (PE, including high-density PE: HDPE, low-density PE: LDPE, linear low-density PE: LLDPE), polystyrene (PS), polyvinyl chloride (PVC), synthetic clothing fibers, polyethylene terephthalate (PET), polyester, polyamide (PA; nylon), polyacrylonitrile (PAN), synthetic clothing fibers (SCF), melamine-formaldehyde fragrance encapsulates (MFR), antifouling paint particles (APP) and biodegradable polylactic acid (PLA). PE was the most frequently studied polymer (22 publications) followed by PS, which was used in 11 publications. PP, tires abrasions, PVC and PET were applied in three to six publications each (Figure 4A).
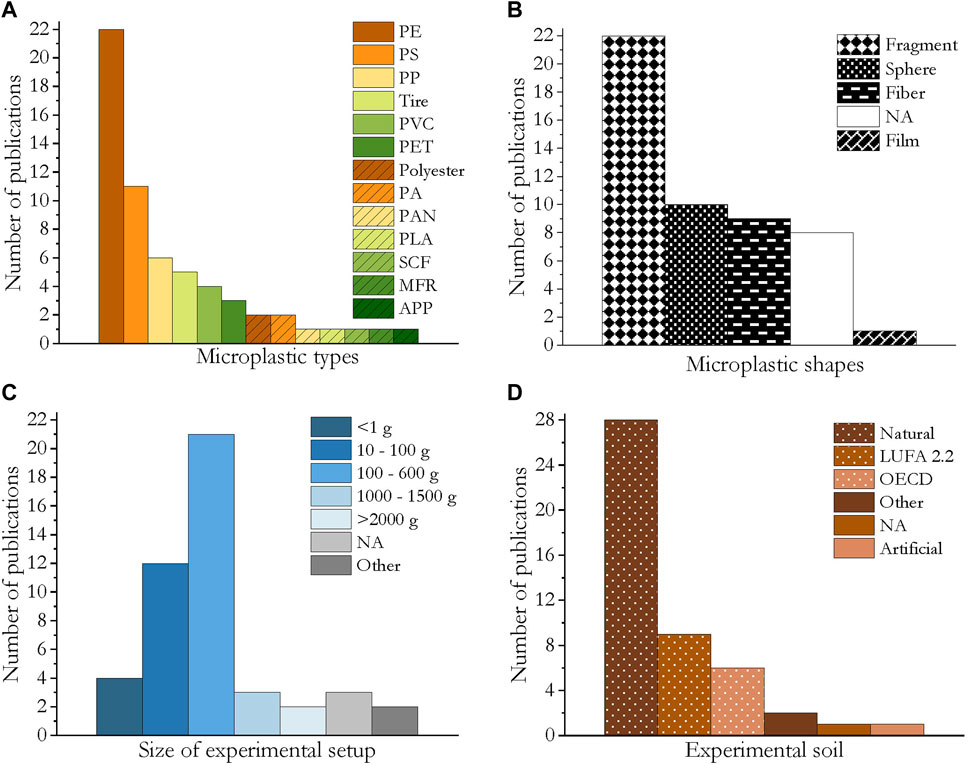
FIGURE 4. Bar charts of the experimental design and the characteristics of the applied microplastics in the reviewed publications. (A) Applied microplastic types. (B) Microplastic shapes (NA = microplastic shape not stated); abbreviations: SCF = synthetic clothing fiber, MFR = melamine-formaldehyde resin. (C) Size of experimental setup (NA = not stated.) (D) Experimental soil (NA = soil type not stated).
Four different microplastic shapes were evaluated for their effects on soil invertebrates: spheres, fragments, fibers and films. Fragments were tested in 22 studies, spheres in 10, fibers in 9, and plastic films in one. In eight publications the microplastic shape was not specified (Figure 4B).
In the microplastic exposure experiments, the amounts of dry soil ranged from <1 g (four publications) to >1 kg (five publications). Most approaches used 100–600 g (21 publications), followed by 10–100 g (12 publications). In three papers the amount of soil was not specified. Only publications were considered in which the organisms were exposed to microplastics at least partly via the soil matrix (Figure 4C).
Most studies made use of field soils (28 publications), although the LUFA standard soil type 2.2 and OECD artificial soil were also commonly applied in nine and six publications, respectively. Additionally, a further artificial soil or other grounds were used (Figure 4D).
In general, 13 species of soil invertebrates were studied for their response to the addition of microplastics. In 34 publications annelids were tested, with the standard test organisms Eisenia fetida and Folsomia candida used in 15 and five publications respectively, collembolans in 12, Porcellio scaber (isopoda) in four, and the mite Oppia nitens in one. As representative of the nematodes and molluscs, Caenorhabditis elegans and Achatina fulica were used in three and one publication as test organism, respectively. In two studies, a natural occurring soil community was exposed to microplastics (Figure 5).
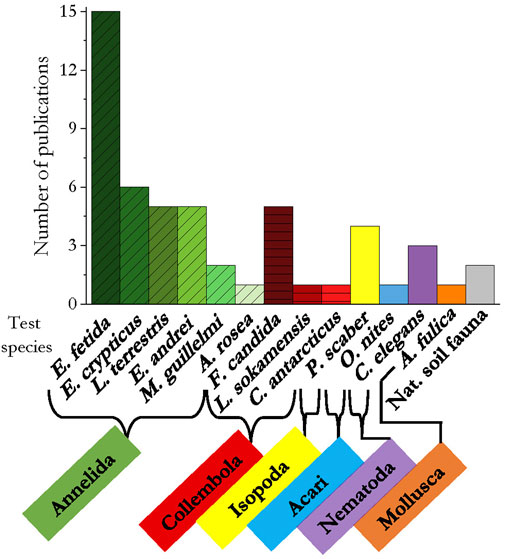
FIGURE 5. Test species per taxa used in the reviewed literature. Related colors of the species indicate affiliation with the same taxon.
3.2 The uptake and bioturbation of microplastics through soil invertebrates
While ingestion, egestion and bioturbation are not strictly ecotoxicological effects, they contribute to the adverse effects of microplastics in soil (Table 1) via their incorporation and transport by soil invertebrates (Duis & Coors, 2016; Rillig et al., 2017). These processes were considered in 16 of 45 papers, 14 of which focussed on ingestion and/or egestion and the remaining two on bioturbation (Table 1). Microplastics of several different types, shapes and concentrations and ranging in size from 100 nm to 2800 µm were shown to be ingested and egested by annelids, arthropods and molluscs. Among the Annelida, L. terrestris, E. andrei, E. fetida, E. crypticus and M. guillelmi ingested and/or egested PE, PS, PP and MFR spheres with sizes between 100 nm and 2800 µm (Rillig et al., 2017; Jiang et al., 2020; Zhou et al., 2020; Kuehr et al., 2021; Lahive et al., 2021). Other studies demonstrated that annelids ingested and/or egested fragments (8–2000 µm) of LLDPE, PP, tires, nylon, HDPE (Rodriguez-Seijo et al., 2017; Lahive et al., 2019; Zhou et al., 2020; Kwak & An, 2021a; Li M. et al., 2021; Cheng et al., 2021; Sheng et al., 2021), fibers (12–2400 µm) of PET, PP, PE (Selonen et al., 2020; Kwak and An, 2021a; Lahive et al., 2021), PE beads (180–300 μm; Kwak & An, 2021b) and particles (100 nm–300 µm) of PE and PS of unspecific shape (Wang et al., 2019; Xu et al., 2021a). The arthropods F. candida (Zhu et al., 2018; Kwak and An, 2021a) and P. scaber (Selonen et al., 2020) ingested and/or egested PP fiber-fragment mixtures (<300 µm), PVC particles (80–250 µm) and PE fibers (12–2400 µm). The study of Bergami et al. was the only one that analyzed the uptake of PS foam (size not specified) in a natural occurring population of the collembolan C. antarcticus (Bergami et al., 2020). The study of Song et al. (2019) was the only one that focussed on a molluscan species A. fulica, which was shown to ingest and egest PET fibers (12578.8 µm in length) (Table 1).
In most studies, the ingestion/egestion of microplastics was dose- or size-dependent (e.g. Selonen et al., 2020). Evidence of the retention of micro- and nanoplastics in the tissues and organs of soil invertebrates was provided in several cases (Jiang et al., 2020; Zhou et al., 2020; Lahive et al., 2021) (Table1).
Bioturbation by microplastics was analyzed in L. terrestris. Exposure to LDPE fragments increased the concentration of microplastic found in the earthworm’s burrows. The transport of LDPE and PE particles was size-dependent, as the fragments found in the burrows were smaller than those in the surface litter and in deeper soil layers (Huerta Lwanga et al., 2017; Rillig et al., 2017) (Table 1).
3.3 Accumulation of microplastic-associated chemicals
The accumulation of chemicals in tissues of soil invertebrates through microplastic exposure is also not a strictly ecotoxicological effect. Nevertheless, in contrast to microplastic particles, the body burdens of dissolved chemicals in organisms are clearly related to their adverse effects (McCarty et al., 2013) and therefore relevant in risk assessments (Schaefer et al., 2015). Microplastics can act as vectors for environmental chemicals in two ways. (1) by the leaching of their stabilizers, plasticizers and other associated chemical compounds into the environmental medium, allowing their subsequent ingestion by organisms and thus their bioaccumulation (Teuten et al., 2009; Halle et al., 2020) and (2) by absorbing environmental chemicals (Table 3), such as heavy metals, pesticides, or persistent organic pollutants (POPs), which are then ingested with the microplastics by soil organisms (Akdogan and Guven, 2019; Torres et al., 2021). The accumulation of microplastic-associated chemicals has been evaluated only in annelid species. Those studies generally demonstrated that microplastics are a potential source of hazardous leachates (Table 2) and facilitate the accumulation of toxic chemicals (Table 3).
3.3.1 Accumulation of leached chemicals
Two publications analyzed the accumulation of leached chemicals. A significant bioaccumulation of endogenous hexabromocyclododecanes (HBCDDs) from EPS (expanded polystyrene) was found in the digestive fluid of E. fetida, and M. guillelmi after 28 days. Accumulation increased with the decreasing size of EPS particles and prolonged exposure time (Li et al., 2019). Tire fragments induced an accumulation of metals (Zn, Cd, Pb) in E. fetida after 14 and 28 days. In that study, the metal concentrations in the earthworms’ tissues were related to microplastic size and exposure time (Sheng et al., 2021) (Table 2).
3.3.2 Accumulation of environmental chemicals
The influence of microplastics on the bioavailability of environmental chemicals was examined in eight studies, using E. fetida, L. terrestris and E. crypticus as the test species (Table 3). After the co-exposure of E. fetida to PE particles and Cd and Cu for 28 days, the levels of the metals in the worm’s tissues were significantly increased (Li B. et al., 2021; Huang et al., 2021). Exposure to PS particles in Cd- and As-contaminated soil resulted in significantly elevated metal levels in E. fetida’s tissues after 21 days. Microplastics induced a higher accumulation than did nanosized plastics (Xu et al., 2021b). Zhou and co-authors reported a significantly higher accumulation of Cd in the tissues of E. fetida when PE beads were added (Zhou et al., 2020). The bioaccumulation of phenanthrene in the tissues of E. fetida was higher in the presence than the absence of PS particles (Xu et al., 2021a). Perfluorooctanoic acid (PFOA), perfluorooctane sulfonate (PFOS) (both 10 mg kg−1) and a mixture of PFOA/PFOS (5/5 mg kg−1) accumulated in the tissues of E. fetida. Bioaccumulation was dependent on the concentration of PVC: 1000 mg PVC kg−1 significantly increased the incorporation of PFOA/PFOS, at least 500 mg PVC kg−1 was needed for a significant accumulation of PFOA, but only ≥1 mg PVC kg−1 facilitated the significant accumulation of PFOS (Sobhani et al., 2021a) (Table 3).
However, in other studies there was no increase in the accumulation of chemicals in the presence of microplastics. The addition of PA or PVC did not enhance the accumulation of tetracycline in E. crypticus after 21 days (Ma et al., 2020). In a co-contamination study with HDPE fragments, Zn levels in the gut or chloragog cells of L. terrestris were not elevated after 28 days (Hodson et al., 2017) (Table 3).
3.4 Physical effects of microplastics on soil invertebrates
The morphological features of microplastics, including their size, shape or surface structure, might pose mechanical hazards to organisms (Barnes et al., 2009), in turn affecting their cellular responses, microbiota, mortality, development, behavior and reproduction. Of the 45 reviewed papers, 34 dealt with physical effects (Figure 4). It should be noted that while the respective authors assumed a physical impact, additional effects of possibly leached endogenous chemicals from the experimentally applied microplastics could not be excluded.
In the following, the different effect endpoints considered as indicators of physical impacts of microplastics are considered from a sub-organismic to organismic to community level.
3.4.1 Cellular response
Microplastics can induce changes in cellular responses via genetic damages/alterations, gene expression (levels), metabolic pathways, enzyme activity/levels, the production of reactive oxygen species (ROS), miscellaneous inflammatory reactions, cell structure/lysis, or the lipid, carbohydrate and protein content of tissues (Table 4). In most experiments, a negative influence of microplastics on the cellular responses, including oxidative stress reactions and histopathological changes, of several organisms was determined (Table 4). The expression levels of several genes and oxidative indicators were used as indicators, including annetocin (ANN), calreticulin (CRT), catalase (CAT), glutathione (GSH), glutathione S-transferase (GST), glutathione peroxidase (GPx), heat shock protein 70 (Hsp70), metallothionein (MT), translationally controlled tumor protein (TCTP), thiobarbituric acid reactive substances (TBARS), lactate dehydrogenase (LDH), lipid hydroperoxide (LPO), malondialdehyde (MDA), phenoloxidase (PO) and superoxide dismutase (SOD). Changes in the levels of those biochemical markers indicated an oxidative stress response. For example, the radical scavengers CAT, SOD and the detoxifying enzyme GST were shown to be important for inactivating ROS and obviating cell damages (Liu et al., 2012; Zhang et al., 2014; Hu et al., 2016). High levels of ROS induce detectable changes in the synthesis or modification of enzymes responsible for inactivation (Liu et al., 2011; Wang et al., 2016; Cheng et al., 2020).
The effects of microplastics were analyzed in the annelids E. crypticus, E. andrei and, most often, E. fetida. Exposure to HDPE, LDPE, PP and PS induced DNA damages in the F0 and the F1 generation of E. fetida (Cheng et al., 2020; Jiang et al., 2020; Xu et al., 2021a; Li M. et al., 2021; Sobhani et al., 2021b). The extent of the damage was larger at higher particle concentrations and larger bead sizes (Jiang et al., 2020).
The levels of GSH, ROS, LDH, LPO, MDA, and TBARS, the activity of CAT, GST, POS, SOD and the transcript levels of ANN, CRT, Hsp70, MT, SOD and TCTP increased significantly in annelids exposed to several shapes of LDPE, PE, PP and PS (Rodriguez-Seijo et al., 2018a; Wang et al., 2019; Cheng et al., 2020; Jiang et al., 2020; Zhou et al., 2020; Li B. et al., 2021; Xu et al., 2021a). Conversely, exposure to HDPE, LDPE, PE, PP, PS significantly decreased CAT, SOD, and GST activity, MDA levels and CAT and GST transcript levels (Rodriguez-Seijo et al., 2018a; Wang et al., 2019; Cheng et al., 2020; Jiang et al., 2020; Xu et al., 2021a; Li M. et al., 2021). Finally, LDPE and PE had no impact on SOD and GST activity or on TBARS, MDA or ROS levels in E. fetida (Rodriguez-Seijo et al., 2018b; Wang et al., 2019; Cheng et al., 2020; Kwak and An, 2021b).
On the tissue level, PS spheres induced the enlargement and deformation of E. fetida cells, altered the size of cell nuclei and caused cell lysis after 14 days (Jiang et al., 2020). The cell viability of E. fetida was affected by PE beads, as the esterase activity of the coelomocytes was significantly lowered. While female reproductive organs were not affected, male reproductive organs were significantly damaged (Kwak & An, 2021b).
In E. andrei a LOEC of 62.5 mg LLDPE fragments kg−1 dry soil caused histopathological damage, by significantly inducing fibrosis, inflammatory infiltrates and congestion (Rodriguez-Seijo et al., 2017). In E. andrei and E. fetida, these effects were dose-dependent (Rodriguez-Seijo et al., 2017; Rodriguez-Seijo et al., 2018a). After exposure to a facemask-derived PP fiber-fragment mixture, spermatogenesis in E. andrei was significantly damaged. The cell viability of coelomocytes was affected, but there were no signs of oxidative stress nor was tissue damage, including bleeding, swelling and thinning, observed. Neither lysosomal stability nor the number of mature oocytes was impaired (Kwak & An, 2021a). Oxidative reactions were observed in E. crypticus as well, as HDPE fragments (4 mm) provoked a dose-dependent increase in GST and CAT activity (Pflugmacher et al., 2020). In the same organism, PA and PVC triggered an increase in the abundance of ARGs (antibiotic resistance genes), whereas MGEs (mobile genetic elements) were not affected (Ma et al., 2020). In L. terrestris, exposure to polyester microfibers caused a dose-dependent increase in the transcript level of mt-2. The expression of the hsp70 was significantly reduced but there was no effect on sod-1 expression (Prendergast-Miller et al., 2019).
The results for microplastic effects in springtail species are ambiguous. There was no evidence of oxidative stress, as esterase activity in F. candida was not affected by exposure to a facemask-derived PP fiber and fragment mixture (Kwak & An, 2021a). In F. candida’s tissues, PVC treatment for 28 and 56 days resulted in a significant enrichment of δ15N and δ13C while the C:N ratio itself was not altered. The nitrogen and carbon isotopes were used as indicator of the springtail’s feeding habits and metabolic turnover rates (Zhu et al., 2018). In the springtail P. scaber, neither the viability of haemocytes nor the lipid, carbohydrate and protein content was affected by PE fibers, whereas PO-like activity was increased in the presence of 0.5% PE. Tire fragments had no effect on the differential haemocyte count, haemocyte viability or PO-like activity but the total haemocyte count (THC) significantly increased (Selonen et al., 2020; Dolar et al., 2021).
Oxidative stress was also demonstrated in A. fulica, as GPx levels and total antioxidant capacity (T-AOC) significantly decreased and the MDA content was significantly increased after exposure of the mollusc to PET fibers for 28 days. Exposure also resulted in mechanical tissue damage in the stomach, intestines and the villi of the gastric wall of the snail, whereas kidney and liver were unaffected (Song et al., 2019).
3.4.2 Microbiota
Only four publications focussed on (gut) microbiota (Table 4). Different types of microplastics (PA, PVC, PE) were reported to significantly change the microbial composition in soil invertebrates (F. candida, E. crypticus). Bacterial alpha diversity was significantly decreased in microplastic treatments (Zhu et al., 2018; Ju et al., 2019; Ma et al., 2020). In comparison, there was no effect on the bacterial alpha diversity and density of M. guillemi by either HDPE or PP fragments (Cheng et al., 2021).
3.4.3 Mortality
Compared to all other analyzed parameters, the mortality of soil invertebrates, examined in 22 publications (65%), was the least sensitive towards microplastics exposure. In four studies, microplastics decreased survival at higher particle contents or a prolonged exposure time. The majority of studies revealed no effect of several microplastic types on the mortality of annelids (Boots et al., 2019; Cheng et al., 2020, 2021; Hodson et al., 2017; Kwak & An, 2021a, b; Lahive et al., 2019; Li M. et al., 2021; Ma et al., 2020; Prendergast-Miller et al., 2019; Rillig et al., 2017; Rodriguez-Seijo et al., 2017, ; Selonen et al., 2020; Sobhani et al., 2021a; Xu et al., 2021a) or P. scaber, O, nitens, F. candida or A. fulica (Kwak and An, 2021a; Selonen et al., 2020; Song et al., 2019; Zhu et al., 2018; Table 4). Jiang et al., 2020 found a significantly higher mortality of E. fetida exposed to 1000 μg PS kg−1). In another study, exposure to PP spheres (<150 µm) at concentrations >0.3% soil dry weight increased the mortality of E. fetida after 42 days (Zhou et al., 2020). A long-term experiment with natural soil demonstrated significantly higher dose- and time dependent mortality in the annelid species L. terrestris when exposed to LDPE for 60 days (Huerta Lwanga et al., 2016). In a 1-week experiment, Ju et al., 2019 showed no lethal effects of PE spheres on F. candida, whereas in an experiment lasting 28 days mortality was significantly higher. The mortality of C. elegans was not affected by exposure to tire microplastics for 2 days whereas survival was significantly reduced after a 10-day exposure (Kim et al., 2021) (Table 4).
3.4.4 Development
Developmental alterations caused by microplastics include effects on growth (rate), biomass and body length and were examined in 19 publications. No general impact tendencies of microplastic on soil invertebrates could be derived, as contradictory results were found. In A. rosea and L. terrestris both negative and no effects on development were reported. Similarly in E. fetida and F. candida both no impact or a striking elevation/reduction of the growth rate was determined. Tire fragments decreased the growth in C. elegans (Kim et al., 2021). LLDPE, PE and PET had no influence on the biomass of E. andrei, P. scaber and A. fulica (Rodriguez-Seijo et al., 2017; Selonen et al., 2020; Song et al., 2019; Table 4).
In A. rosea exposed for 30 days to HDPE, biomass was significantly reduced. The growth and weight of L. terrestris exposed to LDPE and PS were significantly reduced after 7, 14 and 60 days; the effect on the growth rate was dose-dependent (Huerta Lwanga et al., 2016). By contrast, HDPE, PE, PET, PLA, polyester fibers and synthetic clothing fibers had no effect on the weight of either L. terrestris (Hodson et al., 2017; Rillig et al., 2017; Prendergast-Miller et al., 2019; Lahive et al., 2021) or A. rosea (Boots et al., 2019).
LDPE pellets, PVC particles, PS fragments (pure and commercial)/particles and PE particles had no significant effects on the weight of E. fetida (Rodriguez-Seijo et al., 2018a; Sobhani et al., 2021a, Wang et al., 2019), whereas Jiang et al., 2020 reported a significantly increased growth rate after exposure of the worm to PS spheres for 14 days. Another study showed that exposure to PP spheres (<150 µm) at a concentration of ≥0.6% soil dry weight and to PS particles (100 nm–100 µm) reduced the growth and weight of E. fetida after 14 and 21 days, respectively (Zhou et al., 2020; Xu et al., 2021a). However, in E. crypticus exposed to PVC particles for 21 days, body weight was significantly increased (Ma et al., 2020). The collembolan F. candida was not affected by exposure to PVC (Zhu et al., 2018) whereas face-mask-derived PP microplastics significantly reduced its growth, by 92.9% after 28 days (Kwak and An, 2021a).
3.4.5 Behavior
Alterations in behavior by microplastics comprise changes in movement, foraging activity and the stimulation of avoidance behavior. Microplastics were shown to have significant impacts on the movement activities of soil invertebrates. Ambiguous results were found focussing on the feeding activity and avoidance behavior (Table 4).
The addition of PE/PS spheres and PE fragments significantly reduced the movement of L. sokamensis (Kim & An, 2019). Significantly more burrow formation and bioturbation activity were provoked in L. terrestris exposed to 7% LDPE over 2 weeks (Huerta Lwanga et al., 2017).
The food intake of the mollusc A. fulica was reduced by the ingestion of PET fibers (Song et al., 2019).
Barreto and co-authors analyzed the impacts of PE and PP fibers on a natural soil community. The feeding activity of microarthropods was not affected after 4 weeks, whereas exposure to PE but not PP increased the decomposition rate (Barreto et al., 2020). In a study of avoidance behavior, F. candida significantly evaded PE spheres in a dose-dependent manner (Ju et al., 2019). In a two-sided test arena, E. crypticus clearly avoided the side containing a higher HDPE concentration (0/2, 0/4, 0/8, 2/4, 2/8, 4/8% dw) (Pflugmacher et al., 2020). In comparison, PE/PET/polyester fibers, PP fragments and fibers triggered neither avoidance behavior in L. terrestris (Prendergast-Miller et al., 2019; Lahive et al., 2021) or F. candida (Kwak and An, 2021a) nor a change in the feeding activity of P. scaber (Selonen et al., 2020).
3.4.6 Reproduction
Reproduction was examined in 12 publications. There was no significant reduction in L. terrestris, P. scaber, O. nitens, E. andrei, E. crypticus, F. candida and C. elegans (Huerta Lwanga et al., 2016, Kim et al., 2020b, Rodriguez-Seijo et al., 2017 and Selonen et al., 2020; Table 4). Lahive et al. (2019) observed a polymer-dependent impact on E. crypticus reproduction with PVC particles having no effects, while after 21 days of exposure polyamide particles at very high concentrations (>90 g/kg soil) decreased reproduction significantly (Table 4). In E. crypticus exposed to PA particles, the reproduction rate was elevated but it decreased significantly in response to PVC (Ma et al., 2020). After 4 weeks the number of E. fetida juveniles was significantly reduced by 1000 mg PVC particles kg−1 (Sobhani et al., 2021a). In a long-term experiment, a significant a dose-dependent decrease in the reproduction of F0 and F1 generations of E. fetida was determined when the worm was exposed to pure or commercial PS fragments, thus revealing transgenerational effects of microplastic exposure (Sobhani et al., 2021b). A significant inhibition by PE and PP on the reproduction rate of F. candida after 28 days was reported as well (Ju et al., 2019; Kwak and An, 2021a). PS beads, PET, HDPE, PP, PS, tire fragments and PAN fibers significantly reduced the number of offspring in C. elegans (Kim et al., 2020a; Kim et al., 2021).
3.4.7 Community structure
The effects of microplastic on the community structure of soil invertebrates was analyzed in two studies. The composition of the trophic structure, species richness, and the abundance of taxa served as indicators.
In a natural soil community exposed to LDPE fragments for 287 days in a field experiment, significant impacts on nematode trophic structure and a reduction in the overall abundance of nematodes were determined (Table 4). The abundances of omnivorous, predatory and plant-feeding nematodes declined, whereas fungal- and bacterial-feeding members were unaffected. In general, microarthropod structure was significantly altered by the addition of LDPE. The abundance of dipteran/lepidopteran larvae, ants and oribatid mites significantly decreased. Only the abundance of non-oribatid mites and collembolans remained unaffected (Lin et al., 2020). However, Barreto and co-authors found no effects of microplastics on either the species richness or the abundance of several taxa in a natural soil community, tested in a climate chamber (Barreto et al., 2020).
3.5 Chemical effects of microplastic-associated compounds on soil invertebrates
As discussed above, the leaching of chemical compounds associated with microplastic (e.g. plasticizers or flame retardants) or absorbed from the environment (e.g. polychlorinated biphenyls (PCBs), phenanthrene) can result in harmful impacts on organisms (Teuten et al., 2009; Carbery et al., 2018). While most of the following publications assumed a chemical impact, additional effects of the physical properties of the applied microplastic could not be excluded.
3.5.1 Effects of leaching compounds
Seven publications examined the effects of chemical leachates from microplastics (Table 5). Three different annelid species and P. scaber, F. candida and C. elegans were exposed for 3–56 days.
3.5.1.1 Cellular response
The cellular responses of soil organisms towards microplastic leachates were examined in P. scaber and E. fetida (Table 5). Oxidative stress was induced in E. fetida exposed to tire particles. POD, CAT, GST activity and MDA levels were elevated, whereas SOD activity decreased (Sheng et al., 2021).
Tire particles did not affect electron transfer in P. scaber, whereas acetylcholinesterase (AChE) activity was significantly decreased, with a NOEC of 0.5% and an EC50 of 1.2% (Selonen et al., 2021). When P. scaber was exposed to virgin LDPE, neither the total haemocyte count nor haemocyte viability were affected. However, the proportion of granulocytes increased and that of semigranulocytes decreased. Recycled LDPE did not influence the total haemocyte count, haemocyte viability or the proportion of granulocytes, but the proportion of semigranulocytes decreased in response to virgin LDPE (Kokalj et al., 2021).
3.5.1.2 Gut microbiota
One publication analyzed the effect of leachates on one annelid species (Table 5). Applied tire fragments did not affect the alpha diversity of the gut microbiota in E. fetida, whereas both the bacterial and the fungal community were significantly changed after exposure for 21 days. The study showed that the gut microbiota was more sensitive than soil bacteria to tire particles (Ding et al., 2020).
3.5.1.3 Mortality
Ambiguous results on the mortality of soil invertebrates were reported in five publications. Neither tire particles, nor virgin/recycled LDPE fragments and ‘their leachates affected the survival of P. scaber, F. candida or E. crypticus (Kokalj et al., 2021; Selonen et al., 2021), whereas the survival rate of E. fetida and E. andrei exposed to tire fragments and APPs decreased significantly (Ding et al., 2020; Soroldoni et al., 2021). The mortality of C. elegans was not affected by exposure of the nematode to tire fragments for 2 days, independent of the preincubation time of the microplastics with the testing soil. However, in a longer experiment survival was significantly reduced after 8 days. Preincubation of the microplastic with the soil for 75 days resulted in a significant inhibition already after 6 days (Kim et al., 2021) (Table 5).
3.5.1.4 Development
Two publications found impacts of leachates on the development of invertebrates (Table 5). Antifouling paint particles (0.14%, dry soil w/w) scraped off from a boat’s hull reduced the biomass of E. andrei after 56 days (Soroldini et al., 2021). In C. elegans exposed to ≥100 mg tire fragments kg−1 for 2 days, growth was significantly reduced. A significant inhibition of growth at lower concentrations (1 mg kg−1 and higher) was obtained when the tire particles were preincubated with the testing soil for 30 or 75 days, indicating an effect of leaching compounds (Kim et al., 2021).
3.5.1.5 Behavior
The behavior of soil invertebrates exposed to leachates has been analyzed only in P. scaber (Table 5). Exposure of the woodlouse to 0.5 and 1.5% virgin LDPE fragments for 21 days significantly reduced its feeding rate whereas recycled LDPE or tire fragments had no effect on the feeding rate or activity (Kokalj et al., 2021; Selonen et al., 2021).
3.5.1.6 Reproduction
Five studies examined the impact of microplastic leachates on three annelid species, the nematode C. elegans and the collembolan F. candida (Table 5). Annelida were clearly impacted, as the exposure of E. fetida to tire fragments (≥0.12% microplastic content) for 21 days caused a dose-dependent decrease in reproduction. A logarithmic correlation between reproduction and the concentration of tire particles was determined (Ding et al., 2020). The number of juveniles and cocoons produced by E. andrei was reduced after 56 and 42 days, respectively, when the worm was exposed to 0.01% APPs (Soroldoni et al., 2021). Reproduction in E. crypticus was not affected by microplastic mixed with food, whereas 0.02 and 1.5% tire particles in soil significantly decreased the number of offspring (Selonen et al., 2021.)
The exposure of C. elegans to extractable additive solutions of PE, PAN, PP for 24 h did not affect reproduction, whereas extracts derived from PET significantly reduced the number of offspring. After the removal of those additives by extraction, the toxic effect was lost. The adverse impact of LDPE films and PAN fibers on C. elegans reproduction increased with prolonged exposure and frequent wet-dry cycles of the testing soil (Kim et al., 2020b). When the nematode was exposed to tire fragments for 2 days its brood size was significantly reduced. This decreasing effect was aggravated when the microplastic was preincubated with the testing soil (Kim et al., 2021). Finally, the number of juveniles in F. candida was not affected by tire particles added to soil or food (Selonen et al., 2021).
3.5.2 Influence of microplastic on the toxicity of environmental chemicals
Eleven of the reviewed papers examined the vector effects of microplastics on soil invertebrates, in three annelid species in ten publications and the arthropod P. scaber in one publication (Table 6). The impacts of the microplastics in co-exposure experiments with atrazine, chlorpyrifos, phenanthrene, PFOA, PFOS, tetracycline and the metals As, Cd, Cu, Ni and Zn were analyzed. The exposure time ranged between 7 and 56 days.
3.5.2.1 Cellular response
Microplastic-chemical-co-exposures triggered several oxidative stress responses in E. fetida, E. crypticus and P. scaber. E. fetida was the most well-studied (Table 6). Its co-exposures to PE-Cd, PS- phenanthrene and LDPE-atrazine induced DNA damage (Cheng et al., 2020; Xu et al., 2021a; Huang et al., 2021). LDPE-atrazine, LDPE-chlorpyrifos, PP-Cd, PE-Cd, -Cu or -Ni, and PS-phenanthrene, -Cd or -As triggered increases in LPO and ROS levels, GSH, MDA and TBARS content, total protein, IBR, the activity of CAT, MT, POD, SOD and GST as well as the mRNA levels of HSP70, MT, SOD and TCTP (Cheng et al., 2020; Huang et al., 2021; Li B. et al., 2021; Rodriguez-Seijo et al., 2018b; Xu et al., 2021a; Zhou et al., 2020). In general, cellular responses were aggravated by co-exposure compared to the chemical or microplastic treatment alone (Ma et al., 2020; Zhou et al., 2020; Li B. et al., 2021; Xu et al., 2021a).
By contrast, the levels/activities of several indicators of oxidative stress were decreased in E. fetida after its co-exposure for 28 days. Thus LDPE-atrazine, LDPE-chlorpyrifos, PE-Cd and PS-phenanthrene, -Cd or -As resulted in significantly lower AChE, CAT, GST, POD, SOD activities, GSH, MDA, TBARS contents and CAT, GST MT mRNA levels (Cheng et al., 2020; Huang et al., 2021; Rodriguez-Seijo et al., 2018b; Xu et al., 2021a,). No effect on the AChE activity were observed when E. fetida was exposed to 0.25–1 mm LDPE pellets in combination with chlorpyrifos (Rodriguez-Seijo et al., 2018b).
On tissue level, in E. fetida exposed for 21 days to PS particles (100 μm, 10 μm, 100 nm) in Cd- and As-contaminated soil cellular damage such as abnormal epithelial cells, incomplete coelom tissues and exfoliated intestinal epithelium were observed (Xu et al., 2021b; Huang et al., 2021). The exposure of E. crypticus to PA or PVC particles in combination with tetracycline for 21 days resulted in a significantly higher amount of ARGs and MGEs (Ma et al., 2020).
AChE activity was significantly lower in P. scaber exposed to PE fibers (12–2870 µm) and 0.2–2 mg kg−1 CP for 3 weeks. Both the total haemocyte count (THC) and the proportion of hyalinocytes were elevated whereas the proportion of granulocytes, semigranulocytes and the viability of haemocytes were not affected. The co-exposure of P. scaber to tire fragments (<180 µm) and CP for 3 weeks increased the proportion of granulocytes and decreased that of semigranulocytes. Haemocyte viability and the proportion of hyalinocytes were not affected (Dolar et al., 2021).
3.5.2.2 Gut microbiota
Only two publications focussed on the impacts of co-exposure on the gut microbiota. Thus, the exposure of E. fetida to PS of several sizes and to phenanthrene significantly changed the composition of the gut microbial community (Xu et al., 2021a). Similar results were found in E. crypticus, in which PA/PVC-tetracycline treatment induced a significant change in microbial composition and a reduction in microbial alpha diversity (Ma et al., 2020) (Table 6).
3.5.2.3 Mortality
Almost all publications with experiments lasting 3–56 days reported no effects on the mortality of the test species (Table 6). Most data are available for E. fetida: The combinations LDPE-chlorpyrifos (Rodriguez-Seijo et al., 2018b), aged/unaged LDPE-atrazine (Cheng et al., 2020), PA-Cd or -As (Xu et al., 2021b), PS-phenanthrene (Xu et al., 2021a) and PVC-PFOA and/or PFOS (Sobhani et al., 2021a) did not influence the annelid’s mortality. However, co-exposure to Cd-PS spheres for >42 days significantly reduced survival. In another study the effect of PS-phenanthrene was mostly mediated by the PS component, as phenanthrene addition did not aggravate the effect (Zhou et al., 2020).
3.5.2.4 Development
Eight publications examined the effects of environmental chemicals on developmental traits, with E. fetida as the test organism in most of those studies. After a maximum of 28 days of co-exposure to PE-Cd or PS-phenanthrene, -As or -Cd, the weight of the worm was significantly reduced (Xu et al., 2021a Huang et al., 2021) (Table 3). Additionally, in E. fetida exposed to PP-Cd for 42 days the growth rate was significantly decreased. The decline was greater in the co-exposure treatment than in the Cd alone treatment (Zhou et al., 2020). However, the exposure of E. fetida to LDPE pellets contaminated with chlorpyrifos caused significant weight loss after 28 days (Rodriguez-Seijo et al., 2018b). Similar results were found for L. terrestris exposed to PVC-tetracycline for 21 days (Ma et al., 2020) whereas the co-exposure of E. fetida or L. terrestris to LDPE beads and chlorpyrifos, PS particles and Cd or As, PVC-PFOA and/or PFOS and HDPE-Zn had no impact on the weight of either organism (Hodson et al., 2017; Rodriguez-Seijo et al., 2018b; Sobhani et al., 2021a; Xu et al., 2021b) (Table 6).
3.5.2.5 Behavior
The effects of environmental chemicals and microplastic on the behavior of soil invertebrates were investigated in three studies. Significant avoidance behavior was reported for E. fetida exposed to PE-Cd or to LDPE pellets contaminated with chlorpyrifos (Rodriguez-Seijo et al., 2018b; Huang et al., 2021) whereas L. terrestris did not avoid contaminated HDPE fragments (Hodson et al., 2017) (Table 6).
3.5.2.6 Reproduction
Co-exposure treatments significantly reduced the reproduction of soil invertebrates (Table 6). Cocoon production by E. fetida exposed to Cd and PE particles decreased (Huang et al., 2021), and PVC combined with PFOA, PFOS or a mixture of both significantly reduced the number of juveniles (Sobhani et al., 2021a). In E. crypticus exposed for 21 days to PVC or PA combined with tetracycline, reproduction was significantly reduced (Ma et al., 2020).
4 Discussion
Soils can be hotspots of microplastic pollution, as here dumping, decay, fragmentation and accumulation of plastic takes place. Moreover, soils play a key role in the transition to aquatic environments. Consequently, soil invertebrates are likely to be exposed to elevated concentrations of microplastics, potentially leading to adverse effects on soil ecosystems and thus higher trophic levels. Due to patchy data availability and the heterogenous, non-standardized methodologies used in the different studies, general microplastic-specific effect mechanism in soils could not be deduced. However, our literature review suggests trends that should be the basis of future research.
4.1 Variability in species sensitivity
In general, there was little diversity in the test organisms, as 64% of all studies analyzed only annelid species. As comparisons of species sensitivity with environmental thresholds are the basis of risk assessments, including the risk posed by the specific or unspecific impacts of microplastics (Koelmans et al., 2017), toxicity data must be required for a larger number of soil invertebrate species, to cover at least the most dominant ones.
Nonetheless, the published data revealed differences in the sensitivity of different soil invertebrates to microplastics. Annelida tended to be more sensitive than Collembola. The levels of stress biomarkers were increased in the annelid E. fetida exposed to 0.05% PE particles (Li B. et al., 2021) (Table 4). Cellular responses, development, mortality, and reproduction were negatively impacted in E. andrei, E. fetida and E. crypticus exposed to APP and tire fragments and/or its possible leachates (Ding et al., 2020; Selonen et al., 2021; Sheng et al., 2021; Soroldoni et al., 2021) (Table 5). In comparison, a concentration of at least 0.1% was needed to reduce the reproduction rate in the springtail F. candida (Ju et al., 2019) (Table 4). In F. candida, neither mortality nor reproduction was affected by the leachates of tire fragments (Selonen et al., 2021) (Table 5). However, studies with other chemicals, such as pesticides, showed that the toxicity ranking among soil invertebrates is highly substance specific (Frampton et al., 2006). For example, reproduction in F. candida was more sensitive than E. fetida to the insecticide toxaphene (EC50 = 3.6 mg kg−1 soil dry weight vs EC50 = 54.5 mg kg−1 soil dry weight) (Bezchlebova et al., 2007) and to Ag nanoparticles (Heckmann et al., 2011; Zhang and Filser, 2020) whereas the effect of nickel was comparably (E. fetida: EC50 = 362 mg Ni kg−1 soil dry weight; F. candida: EC50 = 391–461 mg Ni kg−1 soil dry weight) (Lock and Janssen, 2002).
Considering the large heterogeneity of microplastics as a contaminant group, also here a substantial variability in the sensitivity of soil organisms can be expected. Furthermore, the lack of standardized test protocols (including with respect to concentration: mass, and particle numbers; dry or wet weight) hampers reliable comparisons between studies (Cunningham and Sigwart, 2019; De Ruijter et al., 2020). The development of standardized experimental and analytical methods will enable the comparability and reproducibility of results and in turn allow the identification of valid trends in the sensitivities within soil communities.
4.2 Role of the properties of microplastics (polymer/shape/size)
The toxicity of microplastics differing in their polymer composition, shape and type and of mixtures thereof remains to be reliably determined. None of the reviewed papers except one simultaneously tested the effect of more than one microplastic type or shape (Kim and An, 2021a). While one-third of all publications evaluated the interference of PE, PS and PP with soil invertebrates (Figure 4A; Tables 4–6), there are roughly 30,000 EU-approved types of plastic and several plastic types have already been detected in a diversity of soils around the world, including their spatial temporal dynamics (Geyer et al., 2017; Horton et al., 2017; Huerta Lwanga et al., 2018; Büks and Kaupenjohann, 2020; Wong et al., 2020).
Given the limited data on the impacts of microplastics on soil invertebrates, systematic investigations of the influence of particle properties on the ecotoxicological behavior of microplastic in soil are rare and the determination of toxicity mechanisms related to microplastic properties accordingly difficult. Studies using bacteria (Aliivibrio fischeri), plants (Nelumbo nucifera) or fish (Danio rerio) suggest that PVC is more toxic than PS (Lei et al., 2018; Zimmermann et al., 2019; Esterhuizen and Kim, 2022), but this could not be shown for soil invertebrates. Several studies demonstrated that PS affects the cellular responses of soil organisms (Sobhani et al., 2021b; Jiang et al., 2020; Wang et al., 2019; Xu et al., 2021a; Table 4), but a direct comparison of the effects of PS vs those of other polymers is difficult, as in the respective studies the PS particles were smaller (<250 µm) than particles of other microplastic types (e.g. up to 2.4 mm for PE). Particle size is known to play a decisive role in the toxic effects of microplastics on soil invertebrates (e.g. Lei et al., 2018; Mueller et al., 2020), as ingestion is dependent on the size of the organism’s mouth opening (Fueser et al., 2019; Koelmans et al., 2020).
Fragments and fibers are the most common shape found in soil environments (Büks and Kaupenjohann, 2020). In several studies, fragments were shown to negatively impact cellular responses (Rodriguez-Seijo et al., 2017, Sobhani et al., 2021b; Li M. et al., 2021; Table 4) which suggests shape-specific effects. Unfortunately, none of the reviewed publications directly compared the effects of the microplastic shapes with a standardized polymer type, size and concentration. Sublethal effects in Daphnia magna were found to be related to microplastic size, polymer type and shape, with beads and fragments being more toxic than fibers (Schwarzer et al., 2022). Further studies are needed to evaluate the role of microplastic shape in the toxicity of microplastics to soil invertebrates (Büks and Kaupenjohann, 2020).
4.3 Distinguishing physical from chemical effects
To distinguish between the major effect pathways of microplastics, studies were categorized into those examining physical and chemical effects of microplastics. The former accounted for 71% of the reviewed publications. In fact, this ascription neglects that in most studies, due to their simultaneous occurrence, the two toxicity pathways are not readily distinguished. For example, some publications reported DNA damages after microplastic exposure, but this could not be unambiguously attributed to strictly chemical vs strictly physical effects (e.g. Cheng et al., 2020; Sobhani et al., 2021b; Jiang et al., 2020; Table 4). Thus, test setups are required that the experimental separation of these two effect pathways, such as by testing extracted leachates separately from the particles to test for strictly chemical effects (Kim et al., 2020b) and by testing (non-plastic) reference particles with characteristics similar to those of microplastics to account for the physical effects of particles (Koelmans et al., 2022).
4.4 Experimental approaches
Most of the studies evaluating the potential adverse effects of microplastics on soil invertebrates consisted of single-species toxicity tests. Only two studies assessed the impacts of microplastics on natural soil communities (Barreto et al., 2020; Lin et al., 2020; Table 4). Although lower-tier testing is a valuable approach for obtaining reliable toxicity thresholds, assuming standardized methods are used, realistic exposure scenarios are best achieved in community-based or long-term population-based approaches, such as in micro- or mesocosm studies. These systems have several advantages over acute, single-species tests:
1) Whole-community tests allow the toxicity of microplastics to be synchronously assessed in several species, thus significantly expanding the species database on microplastic toxicity.
2) The impacts of microplastics on whole food webs and the transfer of microplastics from one trophic level to another are unclear but can only be elucidated in multispecies systems (Haegerbaeumer et al., 2019b). Several studies have shown that microplastic particles can exert their toxic effects on organisms not only directly, but also indirectly, such as via the dilution of food through or the formation of food-microplastic agglomerates (Hanna et al., 2018; Mueller et al., 2020; Rauchschwalbe et al., 2021). Higher-tier studies, especially terrestrial model ecosystems (TMEs), can reveal both the direct and indirect effects of microplastics on several taxa, trophic, population levels or developmental stages of organisms (Gestel et al., 2020; Mueller et al., 2020).
3) In publications dealing with chemical effects, it could be reported that the exposure time influenced the magnitude of leaching or vector impact (Xu et al., 2021b; Soroldoni et al., 2021). Moreover, as microplastics are highly persistent in the environment (Thompson et al., 2005; Thompson et al., 2009a; Barnes et al., 2009; Horton et al., 2017; Selonen et al., 2020) chronic effects on the soil fauna can be expected, such that risk assessments should be conducted in long-term studies, as has been done in freshwater and marine ecosystems (e.g. Santana et al., 2018; Chisada et al., 2019; Redendo-Hasselerharm et al., 2020; Rauchschwalbe et al., 2022). As transgenerational effects of microplastics on soil invertebrates have been reported (Sobhani et al., 2021b), these should be further analyzed in model ecosystems to evaluate long-term effects of microplastic in soil.
The experimental approaches in the reviewed studies were highly diverse, ranging from single to multiple species set ups, and therefore so were their findings. Terrestrial and aquatic studies with environmental chemicals often yielded more sensitive responses of soil and sediment communities when in microcosms than as single-species tests (Clements et al., 2013; Haegerbaeumer et al., 2016; Yin et al., 2018; Boots et al., 2019). However, a microcosm study using freshwater nematodes showed that the sensitivity of a native nematode community to microplastics in the sediment of the microcosms (Rauchschwalbe et al., 2022) was similar to that determined in a single-species sediment toxicity test (Höss et al., 2022). Analogous to the risk assessment of other environmental chemicals, lower-tier testing with representative species is necessary to determine reliable toxicity thresholds, with higher-tier testing using microcosms or semi-field approaches allowing the validation of these thresholds under more realistic exposure conditions.
4.5 Differences between different soils
Since microplastics can affect the physical properties of soil, including soil structure, pH, size distribution of water-stable soil aggregates and the availability of nutrients, the impact of microplastics on soil invertebrates may depend on the nature of the tested soil (Liu et al., 2017; Machado et al., 2018; Boots et al., 2019; Machado et al., 2019). For example, the toxicity of the fungicide mancozeb on the reproduction of the two soil invertebrates was shown to be highly dependent on the soil type (Carniel et al., 2019). Among the reviewed studies, only one investigated the toxic effect of PS spheres on C. elegans in different soil types. The results showed that the physicochemical properties of the soil influenced the response of C. elegans to soil microplastics (Kim et al., 2020a). However, a comparable study in freshwater sediments found no influence of physico-chemical properties on the response of this nematode (Höss et al., 2022). Microplastic exposure in OECD soil, but not natural field soil (LUFA) significantly increased the mortality of E. fetida (Zhou et al., 2020; Xu et al., 2021a). Nonetheless, although these two studies used microplastics of similar concentration and size, the different experimental set ups hamper a direct comparison of the tested soils.
4.6 Risk assessment: Comparing effects with exposure
The use of effect assessment data, as presented in this review, in a microplastic risk assessment requires realistic exposure estimates, i.e. predicted environmental concentrations. However, for the smaller size fractions of microplastics (<20 µm), reliable data on environmental concentrations are still missing, due to the obvious analytical challenges posed by the presence of these particles in complex matrices, such as soils. In agricultural or horticultural sites, microplastic concentrations of up to 4.5 mg kg−1 dry soil have been determined, whereby and concentrations at industrial used sites exceeded such values up to four times (Büks & Kaupenjohann, 2020). Based on the lowest effect concentrations resulting in toxicity, 100 μg kg−1 of dry soil, as demonstrated in E. fetida (Jiang et al., 2020; Table 4), an environmental risk for soil invertebrates at microplastic hotspots cannot be ruled out. Moreover, including the small fraction, still not reliably covered by analytical studies, an even higher risk might be realistic.
A second important component in a risk assessment is the identification of reliable toxicity thresholds, such as those derived from dose-response curves (effect concentrations: ECx; LOEC, NOEC). However, for microplastics, toxicity thresholds comparable to those available for pesticides and other chemicals are scarce (Table 4; Rodriguez-Seijo et al., 2017; Selonen et al., 2021). A study consisting of an alignment of available microplastic effect thresholds for a variety of organisms (Koehlmans et al., 2020) was unable to include findings for soil invertebrates, due to the lack of threshold data. Therefore, studies producing effect thresholds on the basis of dose-response curves are needed for soil ecosystems.
5 Recommendations
• A larger number of soil invertebrate species should be included in the routine testing of microplastics in soil.
• Robust toxicity thresholds (i.e. effect concentrations) determined on the basis of dose-response testing remain to be established for microplastics. Chronic toxicity endpoints should be included to account for subtle effects and the time-dependent leaching of chemicals from plastic particles.
• Toxicity thresholds for microplastics, based on single-species testing, should be verified by testing under realistic exposure scenarios, including multiple species (whole communities), trophic interactions (and thus also indirect effects) and long-term exposure.
• Test systems are required that distinguish between chemical and physical effects. This includes the testing of extracted leachates and the use of non-plastic reference particles as a negative control for physical particle effects.
• As also reported for microplastics research in aquatic environments, harmonized, standardized methods are urgently needed in tests of the effects of microplastics on soil organisms, as these will allow comparisons of reported effects.
Author contributions
ACFM created the first draft of the manuscript, prepared data organization/collection and contributed to manuscript revision. AH guided manuscript revision and contributed to the organization of the database. SH was responsible for study conceptualization, manuscript writing and editing, and WT for study conceptualization and manuscript revision.
Funding
This work was funded by the German Federal Ministry of Education and Research (BMBF) as part of the project MikroPlaTaS–Microplastics in Dams and Reservoirs: Sedimentation, Spread, Effects (BMBF grant no. 02WPL1448D and 02WPL1448E).
Acknowledgments
We acknowledge support for the Article Processing Charge by the Deutsche Forschungsgemeinschaft and the Open Access Publication Fund of Bielefeld University.
Conflict of interest
The authors declare that the research was conducted in the absence of any commercial or financial relationships that could be construed as a potential conflict of interest.
Publisher’s note
All claims expressed in this article are solely those of the authors and do not necessarily represent those of their affiliated organizations, or those of the publisher, the editors and the reviewers. Any product that may be evaluated in this article, or claim that may be made by its manufacturer, is not guaranteed or endorsed by the publisher.
References
Akdogan, Z., and Guven, B. (2019). Microplastics in the environment: A critical review of current understanding and identification of future research needs. Environ. Pollut. 254, 113011. UNSP 113011. doi:10.1016/j.envpol.2019.113011
Alimi, O. S., Budarz, J. F., Hernandez, L. M., and Tufenkji, N. (2018). Microplastics and nanoplastics in aquatic environments: Aggregation, deposition, and enhanced contaminant transport. Environ. Sci. Technol. 52, 1704–1724. doi:10.1021/acs.est.7b05559
Anbumani, S., and Kakkar, P. (2018). Ecotoxicological effects of microplastics on biota: A review. Environ. Sci. Pollut. Res. 25, 14373–14396. doi:10.1007/s11356-018-1999-x
Andrady, A. L. (2011). Microplastics in the marine environment. Mar. Pollut. Bull. 62, 1596–1605. doi:10.1016/j.marpolbul.2011.05.030
Bar-On, Y. M., Phillips, R., and Milo, R. (2018). The biomass distribution on Earth. Proc. Natl. Acad. Sci. U. S. A. 115, 6506–6511. doi:10.1073/pnas.1711842115
Bardgett, R. D., and van der Putten, W. H. (2014). Belowground biodiversity and ecosystem functioning. Nature 515, 505–511. doi:10.1038/nature13855
Barnes, D. K. A., Galgani, F., Thompson, R. C., and Barlaz, M. (2009). Accumulation and fragmentation of plastic debris in global environments. Phil. Trans. R. Soc. B 364, 1985. doi:10.1098/rstb.2008.0205
Barreto, C., Rillig, M., and Lindo, Z. (2020). Addition of polyester in soil affects litter decomposition rates but not microarthropod communities. Soil Org. 92, 109–119. doi:10.25674/so92iss2pp109
Bergami, E., Caruso, T., Birarda, G., Vaccari, L., and Corsi, I. (2020). Plastics everywhere: First evidence of polystyrene fragments inside the common antarctic collembolan Cryptopygus antarcticus. Biol. Lett. 16, 20200093. doi:10.1098/rsbl.2020.0093
Besseling, E., Wegner, A., Foekema, E. M., van den Heuvel-Greve, M. J., and Koelmans, A. A. (2013). Effects of microplastic on fitness and PCB bioaccumulation by the lugworm Arenicola marina (L.). Environ. Sci. Technol. 47, 593–600. doi:10.1021/es302763x
Bezchlebová, J., Černohlávková, J., Lána, J., Sochová, I., Kobetičová, K., and Hofman, J. (2007). Effects of toxaphene on soil organisms. Ecotoxicol. Environ. Saf. 68, 326–334. doi:10.1016/j.ecoenv.2007.05.009
Blaesing, M., and Amelung, W. (2018). Plastics in soil: Analytical methods and possible sources. Sci. Total Environ. 612, 422–435. doi:10.1016/j.scitotenv.2017.08.086
Boots, B., Russell, C. W., and Green, D. S. (2019). Effects of microplastics in soil ecosystems: Above and below ground. Environ. Sci. Technol. 53, 11496–11506. doi:10.1021/acs.est.9b03304
Bowker, M. A., Maestre, F. T., and Escolar, C. (2010). Biological crusts as a model system for examining the biodiversity-ecosystem function relationship in soils. Soil Biol. Biochem. 42, 405–417. doi:10.1016/j.soilbio.2009.10.025
Browne, M. A., Crump, P., Niven, S. J., Teuten, E., Tonkin, A., Galloway, T., et al. (2011). Accumulation of microplastic on shorelines woldwide: Sources and sinks. Environ. Sci. Technol. 45, 9175–9179. doi:10.1021/es201811s
Büks, F., and Kaupenjohann, M. (2020). Global concentrations of microplastics in soils – A review. SOIL 6, 649–662. doi:10.5194/soil-6-649-2020
Carbery, M., O’Connor, W., and Thavamani, P. (2018). Trophic transfer of microplastics and mixed contaminants in the marine food web and implications for human health. Environ. Int. 115, 400–409. doi:10.1016/j.envint.2018.03.007
Carniel, C. L. S., Niemeyer, J. C., Iuñes de Oliveira Filho, L. C., Alexandre, D., Gebler, L., and Klauberg-Filho, O. (2019). The fungicide mancozeb affects soil invertebrates in two subtropical Brazilian soils. Chemosphere 232, 180–185. doi:10.1016/j.chemosphere.2019.05.179
Cheng, Y., Song, W., Tian, H., Zhang, K., Li, B., Du, Z., et al. (2021). The effects of high-density polyethylene and polypropylene microplastics on the soil and earthworm Metaphire guillelmi gut microbiota. Chemosphere 267, 129219. doi:10.1016/j.chemosphere.2020.129219
Cheng, Y., Zhu, L., Song, W., Jiang, C., Li, B., Du, Z., et al. (2020). Combined effects of mulch film-derived microplastics and atrazine on oxidative stress and gene expression in earthworm (Eisenia fetida). Sci. Total Environ. 746, 141280. doi:10.1016/j.scitotenv.2020.141280
Chisada, S., Yoshida, M., and Karita, K. (2019). Ingestion of polyethylene microbeads affects the growth and reproduction of medaka, Oryzias latipes. Environ. Pollut. 254, 113094. doi:10.1016/j.envpol.2019.113094
Cisneros-Dozal, L. M., Trumbore, S., and Hanson, P. J. (2006). Partitioning sources of soil-respired CO2 and their seasonal variation using a unique radiocarbon tracer. Glob. Chang. Biol. 12, 194–204. doi:10.1111/j.1365-2486.2005.001061.x
Clements, W. H., Cadmus, P., and Brinkman, S. F. (2013). Responses of aquatic insects to Cu and Zn in stream microcosms: Understanding differences between single species tests and field responses. Environ. Sci. Technol. 47, 7506–7513. doi:10.1021/es401255h
Cole, M., Lindeque, P., Halsband, C., and Galloway, T. S. (2011). Microplastics as contaminants in the marine environment: A review. Mar. Pollut. Bull. 62, 2588–2597. doi:10.1016/j.marpolbul.2011.09.025
Corradini, F., Meza, P., Eguiluz, R., Casado, F., Huerta-Lwanga, E., and Geissen, V. (2019). Evidence of microplastic accumulation in agricultural soils from sewage sludge disposal. Sci. Total Environ. 671, 411–420. doi:10.1016/j.scitotenv.2019.03.368
Cunningham, E. M., and Sigwart, J. D. (2019). Environmentally accurate microplastic levels and their absence from exposure studies. Integr. Comp. Biol. 59, 1485–1496. doi:10.1093/icb/icz068
da Costa, J. P., Santos, P. S. M., Duarte, A. C., and Rocha-Santos, T. (2016). Nano)plastics in the environment - sources, fates and effects. Sci. Total Environ. 566, 15–26. doi:10.1016/j.scitotenv.2016.05.041
de Ruijter, V. N., Redondo-Hasselerharm, P. E., Gouin, T., and Koelmans, A. A. (2020). Quality criteria for microplastic effect studies in the context of risk assessment: A critical review. Environ. Sci. Technol. 54, 11692–11705. doi:10.1021/acs.est.0c03057
Ding, J., Dong, Z., Hongtao, W., Lassen, S., Chen, Q., Li, G., et al. (2020). Dysbiosis in the gut microbiota of soil fauna explains the toxicity of tire tread particles. Environ. Sci. Technol. 54, 12.doi:10.1021/acs.est.0c00917
Dolar, A., Selonen, S., Gestel, C., Perc, V., Drobne, D., and Kokalj, A. (2021). Microplastics, chlorpyrifos and their mixtures modulate immune processes in the terrestrial crustacean Porcellio scaber. Sci. Total Environ. 772, 144900. doi:10.1016/j.scitotenv.2020.144900
Duis, K., and Coors, A. (2016). Microplastics in the aquatic and terrestrial environment: Sources (with a specific focus on personal care products), fate and effects. Environ. Sci. Eur. 28, 2. doi:10.1186/s12302-015-0069-y
ECETOC (2018). An evaluation of the challenges and limitations associated with aquatic toxicity and bioaccumulation studies for sparingly soluble and manufactured particulate substances - technical Report No. 132. Brussels, Belgium: European Centre for Ecotoxicology and Toxicology of Chemicals.
Eerkes-Medrano, D., Thompson, R. C., and Aldridge, D. C. (2015). Microplastics in freshwater systems: A review of the emerging threats, identification of knowledge gaps and prioritisation of research needs. Water Res. 75, 63–82. doi:10.1016/j.watres.2015.02.012
Esterhuizen, M., and Kim, Y. J. (2022). Effects of polypropylene, polyvinyl chloride, polyethylene terephthalate, polyurethane, high-density polyethylene, and polystyrene microplastic on Nelumbo nucifera (Lotus) in water and sediment. Environ. Sci. Pollut. Res. 29, 17580–17590. doi:10.1007/s11356-021-17033-0
Frampton, G. K., Jänsch, S., Scott-Fordsmand, J. J., Römbke, J., and van den Brink, P. J. (2006). Effects of pesticides on soil invertebrates in laboratory studies: A review and analysis using species sensitivity distributions. Environ. Toxicol. Chem. 25, 2480–2489. doi:10.1897/05-438R.1
Frouz, J. (2018). Effects of soil macro- and mesofauna on litter decomposition and soil organic matter stabilization. Geoderma 332, 161–172. doi:10.1016/j.geoderma.2017.08.039
Fueser, H., Mueller, M.-T., Weiss, L., Hoess, S., and Traunspurger, W. (2019). Ingestion of microplastics by nematodes depends on feeding strategy and buccal cavity size. Environ. Pollut. 255, 113227. doi:10.1016/j.envpol.2019.113227
Gautam, R., Bassi, A. S., and Yanful, E. K. (2007). A review of biodegradation of synthetic plastic and foams. Appl. Biochem. Biotechnol. 141, 85–108. doi:10.1007/s12010-007-9212-6
Gestel, C., Mommer, L., Montanarella, L., Pieper, S., Coulson, M., Toschki, A., et al. (2020). Soil biodiversity: State‐of‐the‐Art and possible implementation in chemical risk assessment. Integr. Environ. Assess. Manag. 17, 541–551. doi:10.1002/ieam.4371
Gewert, B., Plassmann, M. M., and MacLeod, M. (2015). Pathways for degradation of plastic polymers floating in the marine environment. Environ. Sci. Process. Impacts 17, 1513–1521. doi:10.1039/c5em00207a
Geyer, R., Jambeck, J. R., and Law, K. L. (2017). Production, use, and fate of all plastics ever made. Sci. Adv. 3, e1700782. doi:10.1126/sciadv.1700782
Haegerbaeumer, A., Höss, S., Ristau, K., Claus, E., Möhlenkamp, C., Heininger, P., et al. (2016). A comparative approach using ecotoxicological methods from single-species bioassays to model ecosystems. Environ. Toxicol. Chem. 35, 2987–2997. doi:10.1002/etc.3482
Haegerbaeumer, A., Mueller, M.-T., Fueser, H., and Traunspurger, W. (2019a). Impacts of micro- and nano-sized plastic particles on benthic invertebrates: A literature review and gap analysis. Front. Environ. Sci. 7, 17. doi:10.3389/fenvs.2019.00017
Haegerbaeumer, A., Raschke, R., Reiff, N., Traunspurger, W., and Höss, S. (2019b). Comparing the effects of fludioxonil on non-target soil invertebrates using ecotoxicological methods from single-species bioassays to model ecosystems. Ecotoxicol. Environ. Saf. 183, 109596. doi:10.1016/j.ecoenv.2019.109596
Halle, L. L., Palmqvist, A., Kampmann, K., and Khan, F. R. (2020). Ecotoxicology of micronized tire rubber: Past, present and future considerations. Sci. Total Environ. 706, 135694. doi:10.1016/j.scitotenv.2019.135694
Hanna, S. K., Bustos, A. R. M., Peterson, A. W., Reipa, V., Scanlan, L. D., Coskun, S. H., et al. (2018). Agglomeration of Escherichia coli with positively charged nanoparticles can lead to artifacts in a standard Caenorhabditis elegans toxicity assay. Environ. Sci. Technol. 52, 5968–5978. doi:10.1021/acs.est.7b06099
He, D., Luo, Y., Lu, S., Liu, M., Song, Y., and Lei, L. (2018). Microplastics in soils: Analytical methods, pollution characteristics and ecological risks. TrAC Trends Anal. Chem. 109, 163–172. doi:10.1016/j.trac.2018.10.006
Heckmann, L.-H., Hovgaard, M. B., Sutherland, D. S., Autrup, H., Besenbacher, F., and Scott-Fordsmand, J. J. (2011). Limit-test toxicity screening of selected inorganic nanoparticles to the earthworm Eisenia fetida. Ecotoxicology 20, 226–233. doi:10.1007/s10646-010-0574-0
Helmberger, M. S., Tiemann, L. K., and Grieshop, M. J. (2020). Towards an ecology of soil microplastics. Funct. Ecol. 34, 550–560. doi:10.1111/1365-2435.13495
Hodson, M. E., Duffus-Hodson, C. A., Clark, A., Prendergast-Miller, M. T., and Thorpe, K. L. (2017). Plastic bag derived-microplastics as a vector for metal exposure in terrestrial invertebrates. Environ. Sci. Technol. 51, 4714–4721. doi:10.1021/acs.est.7b00635
Hoellein, T., Rojas, M., Pink, A., Gasior, J., and Kelly, J. (2014). Anthropogenic litter in urban freshwater ecosystems: Distribution and microbial interactions. PLoS ONE 9, e98485. doi:10.1371/journal.pone.0098485
Hohn, S., Acevedo-Trejos, E., Abrams, J. F., Fulgencio de Moura, J., Spranz, R., and Merico, A. (2020). The long-term legacy of plastic mass production. Sci. Total Environ. 746, 141115. doi:10.1016/j.scitotenv.2020.141115
Horton, A. A., Walton, A., Spurgeon, D. J., Lahive, E., and Svendsen, C. (2017). Microplastics in freshwater and terrestrial environments: Evaluating the current understanding to identify the knowledge gaps and future research priorities. Sci. Total Environ. 586, 127–141. doi:10.1016/j.scitotenv.2017.01.190
Höss, S., Rauchschwalbe, M.-T., Füser, H., and Traunspurger, W. (2022). Food availability is crucial for effects of 1-μm polystyrene beads on the nematode Caenorhabditis elegans in freshwater sediments. Chemosphere 298, 134101. doi:10.1016/j.chemosphere.2022.134101
Hu, S., Zhang, W., Li, J., Lin, K., and Ji, R. (2016). Antioxidant and gene expression responses of Eisenia fetida following repeated exposure to BDE209 and Pb in a soil-earthworm system. Sci. Total Environ. 556, 163–168. doi:10.1016/j.scitotenv.2016.02.194
Huang, C., Ge, Y., Yue, S., Zhao, L., and Qiao, Y. (2021). Microplastics aggravate the joint toxicity to earthworm Eisenia fetida with cadmium by altering its availability. Sci. Total Environ. 753, 142042. doi:10.1016/j.scitotenv.2020.142042
Huerta Lwanga, E., Gertsen, H., Gooren, H., Peters, P., Salanki, T., van der Ploeg, M., et al. (2017). Incorporation of microplastics from litter into burrows of Lumbricus terrestris. Environ. Pollut. 220, 523–531. doi:10.1016/j.envpol.2016.09.096
Huerta Lwanga, E., Gertsen, H., Gooren, H., Peters, P., Salanki, T., van der Ploeg, M., et al. (2016). Microplastics in the terrestrial ecosystem: Implications for Lumbricus terrestris (Oligochaeta, lumbricidae). Environ. Sci. Technol. 50, 2685–2691. doi:10.1021/acs.est.5b05478
Huerta Lwanga, E., Thapa, B., Yang, X., Gertsen, H., Salanki, T., Geissen, V., et al. (2018). Decay of low-density polyethylene by bacteria extracted from earthworm’s guts: A potential for soil restoration. Sci. Total Environ. 624, 753–757. doi:10.1016/j.scitotenv.2017.12.144
Imhof, H. K., Ivleva, N. P., Schmid, J., Niessner, R., and Laforsch, C. (2013). Contamination of beach sediments of a subalpine lake with microplastic particles. Curr. Biol. 23, R867–R868. doi:10.1016/j.cub.2013.09.001
Jambeck, J. R., Geyer, R., Wilcox, C., Siegler, T. R., Perryman, M., Andrady, A., et al. (2015). Plastic waste inputs from land into the ocean. Science 347, 768–771. doi:10.1126/science.1260352
Jiang, X., Chang, Y., Zhang, T., Qiao, Y., Klobucar, G., and Li, M. (2020). Toxicological effects of polystyrene microplastics on earthworm (Eisenia fetida). Environ. Pollut. 259, 113896. doi:10.1016/j.envpol.2019.113896
Ju, H., Zhu, D., and Qiao, M. (2019). Effects of polyethylene microplastics on the gut microbial community, reproduction and avoidance behaviours of the soil springtail, Folsomia candida. Environ. Pollut. 247, 890–897. doi:10.1016/j.envpol.2019.01.097
Kim, S. W., and An, Y.-J. (2019). Soil microplastics inhibit the movement of springtail species. Environ. Int. 126, 699–706. doi:10.1016/j.envint.2019.02.067
Kim, S. W., Kim, D., Jeong, S.-W., and An, Y.-J. (2020a). Size-dependent effects of polystyrene plastic particles on the nematode Caenorhabditis elegans as related to soil physicochemical properties. Environ. Pollut. 258, 113740. doi:10.1016/j.envpol.2019.113740
Kim, S. W., Leifheit, E., Maaß, S., and Rillig, M. (2021). Time-dependent toxicity of tire particles on soil nematodes. Front. Environ. Sci. 9, 681939. doi:10.3389/fenvs.2021.744668
Kim, S. W., Waldman, W. R., Kim, T.-Y., and Rillig, M. C. (2020b). Effects of different microplastics on nematodes in the soil environment: Tracking the extractable Additives using an ecotoxicological approach. Environ. Sci. Technol. 54, 13868–13878. doi:10.1021/acs.est.0c04641
Kim, Y.-N., Yoon, J.-H., and Kim, K.-H. (2020c). Microplastic contamination in soil environment -a review. Soil Sci. Ann. 71, 300–308. doi:10.37501/soilsa/131646
Koelmans, A. A., Besseling, E., Foekema, E., Kooi, M., Mintenig, S., Ossendorp, B. C., et al. (2017). Risks of plastic debris: Unravelling fact, opinion, perception, and belief. Environ. Sci. Technol. 51, 11513–11519. doi:10.1021/acs.est.7b02219
Koelmans, A. A., Redondo-Hasselerharm, P. E., Mohamed Nor, N. H., and Kooi, M. (2020). Solving the nonalignment of methods and approaches used in microplastic research to consistently characterize risk. Environ. Sci. Technol. 54, 12307–12315. doi:10.1021/acs.est.0c02982
Koelmans, A. A., Redondo-Hasselerharm, P. E., Nor, N. H. M., de Ruijter, V. N., Mintenig, S. M., and Kooi, M. (2022). Risk assessment of microplastic particles. Nat. Rev. Mat. 7, 138–152. doi:10.1038/s41578-021-00411-y
Kokalj, A. J., Dolar, A., Titova, J., Visnapuu, M., Skrlep, L., Drobne, D., et al. (2021). Long term exposure to virgin and recycled LDPE microplastics induced minor effects in the freshwater and terrestrial Crustaceans Daphnia magna and Porcellio scaber. Polymers 13, 771. doi:10.3390/polym13050771
Kuehr, S., Windisch, H., Schlechtriem, C., Leon, G., Gasparini, G., and Gimeno, S. (2021). Are fragrance encapsulates taken up by aquatic and terrestrial invertebrate species? Environ. Toxicol. Chem. 41, 931–943. doi:10.1002/etc.5202
Kwak, J. I., and An, Y.-J. (2021a). Microplastic digestion generates fragmented nanoplastics in soils and damages earthworm spermatogenesis and coelomocyte viability. J. Hazard. Mater. 402, 124034. doi:10.1016/j.jhazmat.2020.124034
Kwak, J. I., and An, Y.-J. (2021b). Post COVID-19 pandemic: Biofragmentation and soil ecotoxicological effects of microplastics derived from face masks. J. Hazard. Mat. 416, 126169. doi:10.1016/j.jhazmat.2021.126169
Lahive, E., Cross, R., Saarloos, A., Horton, A., Svendsen, C., Hufenus, R., et al. (2021). Earthworms ingest microplastic fibres and nanoplastics with effects on egestion rate and long-term retention. Sci. Total Environ. 807, 151022. doi:10.1016/j.scitotenv.2021.151022
Lahive, E., Walton, A., Horton, A. A., Spurgeon, D. J., and Svendsen, C. (2019). Microplastic particles reduce reproduction in the terrestrial worm Enchytraeus crypticus in a soil exposure. Environ. Pollut. 255, 113174. doi:10.1016/j.envpol.2019.113174
Lei, L., Wu, S., Lu, S., Liu, M., Song, Y., Fu, Z., et al. (2018). Microplastic particles cause intestinal damage and other adverse effects in zebrafish Danio rerio and nematode Caenorhabditis elegans. Sci. Total Environ. 619, 1–8. doi:10.1016/j.scitotenv.2017.11.103
Li, B., Lan, Z., Wang, L., Sun, H., Yao, Y., Zhang, K., et al. (2019). The release and earthworm bioaccumulation of endogenous hexabromocyclododecanes (HBCDDs) from expanded polystyrene foam microparticles. Environ. Pollut. 255, 113163. doi:10.1016/j.envpol.2019.113163
Li, B., Song, W., Cheng, Y., Zhang, K., Tian, H., Du, Z., et al. (2021a). Ecotoxicological effects of different size ranges of industrial-grade polyethylene and polypropylene microplastics on earthworms Eisenia fetida. Sci. Total Environ. 783, 147007. doi:10.1016/j.scitotenv.2021.147007
Li, M., Liu, Y., Xu, G., Wang, Y., and Yu, Y. (2021b). Impacts of polyethylene microplastics on bioavailability and toxicity of metals in soil. Sci. Total Environ. 760, 144037. doi:10.1016/j.scitotenv.2020.144037
Li, X., Chen, L., Mei, Q., Dong, B., Dai, X., Ding, G., et al. (2018). Microplastics in sewage sludge from the wastewater treatment plants in China. Water Res. 142, 75–85. doi:10.1016/j.watres.2018.05.034
Lin, D., Yang, G., Dou, P., Qian, S., Zhao, L., Yang, Y., et al. (2020). Microplastics negatively affect soil fauna but stimulate microbial activity: Insights from a field-based microplastic addition experiment. Proc. R. Soc. B 287, 20201268. doi:10.1098/rspb.2020.1268
Liu, H., Yang, X., Liu, G., Liang, C., Xue, S., Chen, H., et al. (2017). Response of soil dissolved organic matter to microplastic addition in Chinese loess soil. Chemosphere 185, 907–917. doi:10.1016/j.chemosphere.2017.07.064
Liu, S., Zhou, Q., and Chen, C. (2012). Antioxidant enzyme activities and lipid peroxidation in earthworm Eisenia fetida exposed to 1, 3, 4, 6, 7, 8-hexahydro-4, 6, 6, 7, 8, 8-hexamethyl-cyclopenta-gamma-2-benzopyr an. Environ. Toxicol. 27, 472–479. doi:10.1002/tox.20661
Liu, S., Zhou, Q., and Wang, Y. (2011). Ecotoxicological responses of the earthworm Eisenia fetida exposed to soil contaminated with HHCB. Chemosphere 83, 1080–1086. doi:10.1016/j.chemosphere.2011.01.046
Lock, K., and Janssen, C. R. (2002). Ecotoxicity of nickel to Eisenia fetida, Enchytraeus albidus and Folsomia candida. Chemosphere 46, 197–200. doi:10.1016/S0045-6535(01)00112-6
Ma, J., Sheng, G. D., and O’Connor, P. (2020). Microplastics combined with tetracycline in soils facilitate the formation of antibiotic resistance in the Enchytraeus crypticus microbiome. Environ. Pollut. 264, 114689. doi:10.1016/j.envpol.2020.114689
Machado, A. A. de S., Lau, C. W., Kloas, W., Bergmann, J., Bacheher, J. B., Faltin, E., et al. (2019). Microplastics can change soil properties and affect plant performance. Environ. Sci. Technol. 53, 6044–6052. doi:10.1021/acs.est.9b01339
Machado, A. A. de S., Lau, C. W., Till, J., Kloas, W., Lehmann, A., Becker, R., et al. (2018). Impacts of microplastics on the soil biophysical environment. Environ. Sci. Technol. 52, 9656–9665. doi:10.1021/acs.est.8b02212
McCarty, L. S., Arnot, J. A., and Mackay, D. (2013). Evaluation of critical body residue data for acute narcosis in aquatic organisms. Environ. Toxicol. Chem. 32, 2301–2314. doi:10.1002/etc.2289
Mueller, M.-T., Fueser, H., Trac, L. N., Mayer, P., Traunspurger, W., and Hoess, S. (2020). Surface-related toxicity of polystyrene beads to nematodes and the role of food availability. Environ. Sci. Technol. 54, 1790–1798. doi:10.1021/acs.est.9b06583
Ogonowski, M., Gerdes, Z., and Gorokhova, E. (2018). What we know and what we think we know about microplastic effects – a critical perspective. Curr. Opin. Environ. Sci. Health 1, 41–46. doi:10.1016/j.coesh.2017.09.001
Padervand, M., Lichtfouse, E., Robert, D., and Wang, C. (2020). Removal of microplastics from the environment. A review. Environ. Chem. Lett. 18, 807–828. doi:10.1007/s10311-020-00983-1
Pflugmacher, S., Huttunen, J., Von Wolff, M. A., Penttinen, O.-P., Kim, Y., Kim, S., et al. (2020). Enchytraeus crypticus avoid soil spiked with microplastic. Toxics 8, 10. doi:10.3390/toxics8010010
PlasticsEurope (2021). Plastics - the facts 2021. An analysis of European plastics production. Brussels, Belgium: Demand and Waste Data. Available at: https://plasticseurope.org/de/knowledge-hub/plastics-the-facts-2020-2/.
Porazinska, D. L., Bardgett, R. D., Blaauw, M. B., Hunt, H. W., Parsons, A. N., Seastedt, T. R., et al. (2003). Relationships at the aboveground–belowground interface: Plants, soil biota, and soil processes. Ecol. Monogr. 73, 377–395. doi:10.1890/0012-9615(2003)073[0377:rataip]2.0.co;2
Prendergast-Miller, M. T., Katsiamides, A., Abbass, M., Sturzenbaum, S. R., Thorpe, K. L., and Hodson, M. E. (2019). Polyester-derived microfibre impacts on the soil-dwelling earthworm Lumbricus terrestris. Environ. Pollut. 251, 453–459. doi:10.1016/j.envpol.2019.05.037
Rauchschwalbe, M.-T., Fueser, H., Traunspurger, W., and Höss, S. (2021). Bacterial consumption by nematodes is disturbed by the presence of polystyrene beads: The roles of food dilution and pharyngeal pumping. Environ. Pollut. 273, 116471. doi:10.1016/j.envpol.2021.116471
Rauchschwalbe, M.-T., Höss, S., Hägerbäumer, A., and Traunspurger, W. (2022). Long-term exposure of a free-living freshwater micro- and meiobenthos community to microplastic mixtures in microcosms. Sci. Total Environ. 827, 154207. doi:10.1016/j.scitotenv.2022.154207
Redondo-Hasselerharm, P. E., Gort, G., Peeters, E. T. H. M., and Koelmans, A. A. (2020). Nano- and microplastics affect the composition of freshwater benthic communities in the long term. Sci. Adv. 6, eaay4054. doi:10.1126/sciadv.aay4054
Rillig, M. C. (2012). Microplastic in terrestrial ecosystems and the soil? Environ. Sci. Technol. 46, 6453–6454. doi:10.1021/es302011r
Rillig, M. C., Ziersch, L., and Hempel, S. (2017). Microplastic transport in soil by earthworms. Sci. Rep. 7, 1362. doi:10.1038/s41598-017-01594-7
Rodríguez-Seijo, A., da Costa, J. P., Rocha-Santos, T., Duarte, A. C., and Pereira, R. (2018a). Oxidative stress, energy metabolism and molecular responses of earthworms (Eisenia fetida) exposed to low-density polyethylene microplastics. Environ. Sci. Pollut. Res. 25, 33599–33610. doi:10.1007/s11356-018-3317-z
Rodriguez-Seijo, A., Lourenco, J., Rocha-Santos, T. a. P., da Costa, J., Duarte, A. C., Vala, H., et al. (2017). Histopathological and molecular effects of microplastics in Eisenia andrei Bouche. Environ. Pollut. 220, 495–503. doi:10.1016/j.envpol.2016.09.092
Rodríguez-Seijo, A., Santos, B., Silva, E. F. d., Cachada, A., and Pereira, R. (2018b). Low-density polyethylene microplastics as a source and carriers of agrochemicals to soil and earthworms. Environ. Chem. 16, 8–17. doi:10.1071/en18162
Roger-Estrade, J., Anger, C., Bertrand, M., and Richard, G. (2010). Tillage and soil ecology: Partners for sustainable agriculture. Soil Tillage Res. 111, 33–40. doi:10.1016/j.still.2010.08.010
Ryan, P. G., Moore, C. J., van Franeker, J. A., and Moloney, C. L. (2009). Monitoring the abundance of plastic debris in the marine environment. Phil. Trans. R. Soc. B 364, 1999. doi:10.1098/rstb.2008.0207
Santana, M. F. M., Moreira, F. T., Pereira, C. D. S., Abessa, D. M. S., and Turra, A. (2018). Continuous exposure to microplastics does not cause physiological effects in the cultivated mussel Perna perna. Arch. Environ. Contam. Toxicol. 74, 594–604. doi:10.1007/s00244-018-0504-3
Schaefer, S., Buchmeier, G., Claus, E., Duester, L., Heininger, P., Körner, A., et al. (2015). Bioaccumulation in aquatic systems: Methodological approaches, monitoring and assessment. Environ. Sci. Eur. 27, 5. doi:10.1186/s12302-014-0036-z
Scherer, C., Weber, A., Lambert, S., and Wagner, M. (2018). “Interactions of microplastics with freshwater biota,” in Handbook of environmental chemistry, 153–180.
Schwarzer, M., Brehm, J., Vollmer, M., Jasinski, J., Xu, C., Zainuddin, S., et al. (2022). Shape, size, and polymer dependent effects of microplastics on Daphnia magna. J. Hazard. Mater. 426, 128136. doi:10.1016/j.jhazmat.2021.128136
Selonen, S., Dolar, A., Jemec Kokalj, A., Sackey, L. N. A., Skalar, T., Cruz Fernandes, V., et al. (2021). Exploring the impacts of microplastics and associated chemicals in the terrestrial environment – exposure of soil invertebrates to tire particles. Environ. Res. 201, 111495. doi:10.1016/j.envres.2021.111495
Selonen, S., Dolar, A., Kokalj, A. J., Skalar, T., Dolcet, L. P., Hurley, R., et al. (2020). Exploring the impacts of plastics in soil - the effects of polyester textile fibers on soil invertebrates. Sci. Total Environ. 700, 134451. UNSP 134451. doi:10.1016/j.scitotenv.2019.134451
Shah, A. A., Hasan, F., Hameed, A., and Ahmed, S. (2008). Biological degradation of plastics: A comprehensive review. Biotechnol. Adv. 26, 246–265. doi:10.1016/j.biotechadv.2007.12.005
Sheng, Y., Liu, Y., Wang, K., Cizdziel, J. V., Wu, Y., and Zhou, Y. (2021). Ecotoxicological effects of micronized car tire wear particles and their heavy metals on the earthworm (Eisenia fetida) in soil. Sci. Total Environ. 793, 148613. doi:10.1016/j.scitotenv.2021.148613
Sobhani, Z., Fang, C., Naidu, R., and Megharaj, M. (2021a). Microplastics as a vector of toxic chemicals in soil: Enhanced uptake of perfluorooctane sulfonate and perfluorooctanoic acid by earthworms through sorption and reproductive toxicity. Environ. Technol. Innovation 22, 101476. doi:10.1016/j.eti.2021.101476
Sobhani, Z., Panneerselvan, L., Fang, C., Naidu, R., and Mallavarapu, M. (2021b). Chronic and transgenerational effects of polystyrene microplastics at environmentally relevant concentrations in earthworms (Eisenia fetida). Environ. Toxicol. Chem. 40, 2240–2246. doi:10.1002/etc.5072
Song, Y., Cao, C., Qiu, R., Hu, J., Liu, M., Lu, S., et al. (2019). Uptake and adverse effects of polyethylene terephthalate microplastics fibres on terrestrial snails (Achatina fulica) after soil exposure. Environ. Pollut. 250, 447–455. doi:10.1016/j.envpol.2019.04.066
Soroldoni, S., Honscha, L., Reis, F., Duarte, F. A., Da Silva Júnior, F., and Pinho, G. (2021). Antifouling paint particles in soils: Toxic impact that goes beyond the aquatic environment. Ecotoxicology 30, 1161–1169. doi:10.1007/s10646-021-02418-1
Steinmetz, Z., Wollmann, C., Schaefer, M., Buchmann, C., David, J., Tröger, J., et al. (2016). Plastic mulching in agriculture. Trading short-term agronomic benefits for long-term soil degradation? Sci. Total Environ. 550, 690–705. doi:10.1016/j.scitotenv.2016.01.153
Teuten, E. L., Saquing, J. M., Knappe, D. R. U., Barlaz, M. A., Jonsson, S., Bjorn, A., et al. (2009). Transport and release of chemicals from plastics to the environment and to wildlife. Phil. Trans. R. Soc. B 364, 2027–2045. doi:10.1098/rstb.2008.0284
Thompson, R. C., Moore, C. J., vom Saal, F. S., and Swan, S. H. (2009a). Plastics, the environment and human health: Current consensus and future trends. Phil. Trans. R. Soc. B 364, 2153–2166. doi:10.1098/rstb.2009.0053
Thompson, R. C., Swan, S. H., Moore, C. J., and vom Saal, F. S. (2009b1973–1976). Our plastic age. Phil. Trans. R. Soc. B 364, 1973–1976. doi:10.1098/rstb.2009.0054
Thompson, R., Moore, C., Andrady, A., Gregory, M., Takada, H., and Weisberg, S. (2005). New directions in plastic debris. Science 310, 1117. doi:10.1126/science.310.5751.1117b
Torres, F. G., Carolina Dioses-Salinas, D., Ivan Pizarro-Ortega, C., and De-la-Torre, G. E. (2021). Sorption of chemical contaminants on degradable and non-degradable microplastics: Recent progress and research trends. Sci. Total Environ. 757, 143875. doi:10.1016/j.scitotenv.2020.143875
van den Hoogen, J., Geisen, S., Routh, D., Ferris, H., Traunspurger, W., Wardle, D. A., et al. (2019). Soil nematode abundance and functional group composition at a global scale. Nature 572, 194–198. doi:10.1038/s41586-019-1418-6
Wang, J., Li, Y., Lu, L., Zheng, M., Zhang, X., Tian, H., et al. (2019). Polystyrene microplastics cause tissue damages, sex-specific reproductive disruption and transgenerational effects in marine medaka (Oryzias melastigma). Environ. Pollut. 254, 113024. doi:10.1016/j.envpol.2019.113024
Wang, J., Wang, J., Wang, G., Zhu, L., and Wang, J. (2016). DNA damage and oxidative stress induced by imidacloprid exposure in the earthworm Eisenia fetida. Chemosphere 144, 510–517. doi:10.1016/j.chemosphere.2015.09.004
Wong, J. K. H., Lee, K. K., Tang, K. H. D., and Yap, P.-S. (2020). Microplastics in the freshwater and terrestrial environments: Prevalence, fates, impacts and sustainable solutions. Sci. Total Environ. 719, 137512. doi:10.1016/j.scitotenv.2020.137512
Wright, S. L., Thompson, R. C., and Galloway, T. S. (2013). The physical impacts of microplastics on marine organisms: A review. Environ. Pollut. 178, 483–492. doi:10.1016/j.envpol.2013.02.031
Xu, G., Liu, Y., Song, X., Li, M., and Yu, Y. (2021a). Size effects of microplastics on accumulation and elimination of phenanthrene in earthworms. J. Hazard. Mater. 403, 123966. doi:10.1016/j.jhazmat.2020.123966
Xu, G., Yang, Y., and Yu, Y. (2021b). Size effects of polystyrene microplastics on the accumulation and toxicity of (semi-)metals in earthworms. Environ. Pollut. 291, 118194. doi:10.1016/j.envpol.2021.118194
Yin, X. H., Brock, T. C. M., Barone, L. E., Belgers, J. D. M., Boerwinkel, M.-C., Buijse, L., et al. (2018). Exposure and effects of sediment-spiked fludioxonil on macroinvertebrates and zooplankton in outdoor aquatic microcosms. Sci. Total Environ. 610, 1222–1238. doi:10.1016/j.scitotenv.2017.08.155
Zalasiewicz, J., Waters, C. N., Ivar do Sul, J. A., Corcoran, P. L., Barnosky, A. D., Cearreta, A., et al. (2016). The geological cycle of plastics and their use as a stratigraphic indicator of the Anthropocene. Anthropocene 13, 4–17. doi:10.1016/j.ancene.2016.01.002
Zhang, L., Ji, F., Li, M., Cui, Y., and Wu, B. (2014). Short-term effects of Dechlorane Plus on the earthworm Eisenia fetida determined by a systems biology approach. J. Hazard. Mat. 273, 239–246. doi:10.1016/j.jhazmat.2014.03.018
Zhang, X., and Filser, J. (2020). Low concentration effects and different outcome in repeated reproduction tests with silver nanoparticles, silver nitrate and Folsomia candida (Collembola). Environ. Sci. Eur. 32, 136–138. doi:10.1186/s12302-020-00413-7
Zhou, Y., Liu, X., and Wang, J. (2020). Ecotoxicological effects of microplastics and cadmium on the earthworm Eisenia foetida. J. Hazard. Mater. 392, 122273. doi:10.1016/j.jhazmat.2020.122273
Zhu, D., Chen, Q.-L., An, X.-L., Yang, X.-R., Christie, P., Ke, X., et al. (2018). Exposure of soil collembolans to microplastics perturbs their gut microbiota and alters their isotopic composition. Soil Biol. Biochem. 116, 302–310. doi:10.1016/j.soilbio.2017.10.027
Zimmermann, L., Dierkes, G., Ternes, T. A., Völker, C., and Wagner, M. (2019). Benchmarking the in vitro toxicity and chemical composition of plastic consumer products. Environ. Sci. Technol. 53, 11467–11477. doi:10.1021/acs.est.9b02293
Keywords: invertebrates, mesofauna, microplastic, nanoplastic, soil, toxicity
Citation: Möhrke ACF, Haegerbaeumer A, Traunspurger W and Höss S (2022) Underestimated and ignored? The impacts of microplastic on soil invertebrates—Current scientific knowledge and research needs. Front. Environ. Sci. 10:975904. doi: 10.3389/fenvs.2022.975904
Received: 22 June 2022; Accepted: 04 August 2022;
Published: 27 October 2022.
Edited by:
John Scott, University of Illinois, United StatesReviewed by:
Wenbing Tan, Chinese Research Academy of Environmental Sciences, ChinaSadasivam Anbumani, Indian Institute of Toxicology Research (CSIR), India
Copyright © 2022 Möhrke, Haegerbaeumer, Traunspurger and Höss. This is an open-access article distributed under the terms of the Creative Commons Attribution License (CC BY). The use, distribution or reproduction in other forums is permitted, provided the original author(s) and the copyright owner(s) are credited and that the original publication in this journal is cited, in accordance with accepted academic practice. No use, distribution or reproduction is permitted which does not comply with these terms.
*Correspondence: Anne Christel Franka Möhrke, bW9laHJrZUB1bmktYmllbGVmZWxkLmRl