- 1Energy, Climate & Environment Program, International Institute of Applied Systems Analysis (IIASA), Laxenburg, Austria
- 2Department of Civil Engineering, Institute for Integrated Energy Systems, University of Victoria, Victoria, BC, Canada
- 3International Water Management Institute, Colombo, Sri Lanka
- 4Centre for Water Informatics and Technology, Lahore University of Management Sciences, Lahore, Pakistan
The Indus River Basin (IRB) is a severely water-stressed and rapidly developing home to an estimated 250 million people in South Asia. An acute deficit of environmental flows (EFs) in the basin’s delta negatively impacts geomorphology and surrounding ecosystems. Here, a sub-national model of the IRB’s integrated water–energy–land systems is applied to quantify multi-sector transformations and system costs for enhancing EFs to the Indus Delta. The results show that increasing the average outflows from the basin relative to historical policy levels by 2.5 and 5 times would increase sectoral costs for upstream water users between 17–32 and 68–72% for low and high ecological potential targets. The enhanced EFs result in more energy for pumping and treating water upstream from the delta and a net increase in irrigation and energy investments. The EF policy costs are minimized by 7–14% through cooperation across countries and 6–9% through the coordinated implementation of water efficiency measures in the irrigation, conveyance, power plant cooling, and water treatment sectors. The results underscore the crucial role of a multi-sector, multi-scale collaboration in achieving EF targets in water-stressed river basins for ecosystem adaptation to climate vulnerability, restoration of the delta, and socio-economic benefits.
1 Introduction
Environmental flows (EFs) are defined as the quality, quantity, and patterns of the river flow required for maintaining ecosystem services and are associated with a river’s natural flow regime before human settlement (LeRoy Poff et al., 1997; Richter et al., 2006). EFs support sedimentation transfer to coastal deltas formed at the river mouth. Globally, deltas are critical economic regions, occupying only 1% of the total land area but supporting 7% of the world population (Ericson et al., 2006). Reduced EFs impact the geomorphology of delta regions, re-shaping river connections, creating seismic hazards, and lowering land and groundwater quality (LeRoy Poff et al., 1997; Arthington, 2012). Reduced EFs concentrate pollutants released into rivers and reduce oxygenation levels for aquatic species (Arthington, 2012). Maintaining EFs under future population growth is, thus, an essential strategy for the sustainable development of delta regions (Tharme, 2003).
The environmental flow assessment methodologies currently in use depend on linkages to other local policy recommendations and stakeholder engagement (Hill Clarvis et al., 2014; Hannaford, 2015). There are ongoing efforts to define standards and methods to account for environmental flow methods in rivers based on the ecohydrological relationships in rivers (Richter et al., 2006). More than 200 environmental flow assessment methods exist globally (Tharme, 2003). There are numerous studies on environmental flow assessment methods and the practicality of methods for river users from hydrological, hydraulic, habitat-simulation, and holistic methods applied in several case studies and global scale hydrological models (Pastor et al., 2014). Supplementary Section S1 discusses EF methods in detail.
Defining presumptive standards and constraining the environmental flows in highly modified rivers can be challenging since it becomes infeasible to see the river in terms of the natural flow regime when it is so disturbed (Acreman et al., 2014). Yarnell et al. (2015) characterized highly modified rivers as follows: i) a high proportion converted to reservoirs, ii) most of their annual streamflow is diverted for human uses, and iii) have a high proportion of their length channelized/lined. Although not all characteristics can occur in the same river at one time, the presence of any one of the characteristics in a river can make it a highly modified river (Yarnell et al., 2015).
Maintaining a trade-off as per human demands and fulfilling the environmental flow requirements can be quite a complicated and challenging task for river managers. Therefore, a designer approach to defining the environmental flow was proposed for highly modified rivers. A designer approach suits such rivers, where achieving ecological potential and saving biodiversity is a target, considering the societal demands for highly modified rivers instead of taking the natural flow regime as a reference (Acreman et al., 2014). Constraining water withdrawals enhances environmental flows in deltas (Richter, 2009; Gleeson and Richter, 2018) but limits growth in upstream activities reliant on water. This trade-off is challenging for regions where water is scarce but also in high demand because of the relative cost of alternative freshwater supply options. Wu et al. (2021) carried out a detailed study for the environmental flow planning for highly modified rivers in China which also supports the narrative of the designer approach for constraining flows in such rivers.
With increasing climate vulnerability and limited water, energy, and land resources, developing regions face complex challenges in providing enough resources to the growing population. The issues and challenges in these sectors are complex and have a high degree of interdependency (Rasul, 2014). Therefore, a multi-sector approach to solve the challenge is required for policy analysis and climate adaptation to the vulnerability of exposed resources. South Asia has been recognized as one of the most vulnerable regions in the world due to pressure on limited resources (Rasul, 2014; Byers et al., 2018). Thus, an integrated model for energy, water, and land systems is beneficial to inform stakeholders and policymakers about the utility of different sectors and national and international planning for a sustainable and healthy future (Khan et al., 2017). However, an integrated assessment that considers water, energy, and land systems as interdependent systems is crucial for sustainable decision-making regarding the threats of climate change, rising population demands, and stressed resources. These interdependent systems are crucial for a sustainable future to meet demands and ecosystem services (Khan et al., 2017; Parkinson et al., 2018; Cazcarro et al., 2021).
Several integrated analyses have addressed the challenges of water, energy, and food for the Indus River Basin (IRB). Yang et al. (2016) developed a hydro–agro–economic model, considering the impacts of climate change scenarios on water allocation mechanisms and infrastructure developments. Yang et al. (2013) developed a hydro-economic model for analyzing relationships between climate, water, and agriculture sectors for the IRB to prioritize and plan future investments in the basin. Also, some studies addressed the energy use dimension of conjunctive water management and quantified future water gaps for water supply under projected socio-economic and climate change or gaps for estimating energy demand variation due to groundwater pumping for agriculture (Siddiqi and Wescoat, 2013). However, there is no explicit representation of all energy, water, and land technologies in terms of capacities, activities, and efficiencies across spatial regions to tackle challenging and complex systems research questions (Vinca et al., 2020a).
In this study, we focus on South Asia’s Indus River Basin (IRB). The IRB is one of the most water-stressed basins in the world due to irrigated food production and high population (Byers et al., 2018). Freshwater in the IRB is derived from meltwater in the Hindu-Kush-Himalaya mountain region that flows from China, India, and Afghanistan downstream into Pakistan and drains into the Indus Delta and the Arabian Sea. Since 1869, upstream activities have caused a sediment flux reduction of 13 Mt/yr, and the delta has lost 15 out of the 16 main river channels connecting it to the sea (Syvitski et al., 2009, 2013). Policies targeting enhanced EFs are urgently needed to improve the long-term outlook for the Indus Delta region (Ali et al., 2017).
Despite the critical role of EFs in Indus Delta sustainability, there is a lack of quantitative analysis linking enhanced EF allocation policies to the adaptation costs of upstream sectors (Day et al., 2021). Memon and Thapa (2016) analyzed policy and institutional analyses of the Indus Delta to address the importance of complexity inherent in protecting the Indus Delta. EFs in the Indus Delta are discussed in the context of the Kotri Barrage (a barrage located downstream of the IRB for flow management) to determine saltwater intrusions and accommodate fisheries (Anwar and Bhatti, 2017). Due to high demands and poor management of the IRB, all the river flows are fully utilized except during the September–June season (also known as the Kharif period). The use of water upstream is limited for agricultural purposes unless additional storage reservoirs are provided. The planned hydropower projects by 2030 could enable better management of flows downstream of the Kotri Barrage to the Arabian Sea (Tilmant et al., 2010). Suppose enough fresh water is available downstream of the Kotri Barrage; in that case, it helps recharge aquifers and provides irrigation for agriculture in the riverine sea, maintains the salinity level in the coastal area, and provides livelihoods to the people living in the delta (González-villareal and Schultz, 2005; Anwar et al., 2014).
The CWRAS (Country Water Resources Assistance Strategy) of Pakistan identified the ecological threats to the Indus Delta. It commented that improving the environmental flows for restoring the Indus estuary’s ecosystems is essential for flow-related issues (Hirji and Davis, 2009). However, there is neither an explicit EF policy mentioned for the IRB as a whole nor an institutional mechanism in place to regulate EF allocations, even in the Indus Water Treaty that governs the flow between India and Pakistan (IRSA, 1991; Anwar et al., 2014; Anwar and Bhatti, 2017). The Water Apportionment Accord (WAA), signed in 1991, provides a legislative structure for the distribution of water to the Indus River and among the provincial and command areas in Pakistan. The WAA describes the minimum flow requirements below the Kotri Barrage as 33.79 MCM/day (MCM = thousand cubic meters) (IRSA, 1991), which were reviewed by a panel of experts and changed to 12.23 MCM/day during the June–October season (also known as the Rabi season) and 46.21 MCM/day during November–May (also known as the Kharif season) in 2005 (González-villareal and Schultz, 2005). Yet there has not been an integrated analysis examining how these or more ambitious targets influence upstream infrastructure decision-making or investments required for EF allocation downstream.
In this paper, we incorporate enhanced delta EF policies into a multi-sector model of the IRB to address the following research questions: 1) what are the potential system costs that support the allocation of delta EFs across multiple sectors and uncertainties? and 2) what are the least-cost technological changes in water, energy, and land sectors that enable enhanced EF allocation for ecosystem adaptation to climate vulnerability? The study uses an engineering economic multi-sector model to answer research questions and inform policy decisions. The analysis in this paper identifies investment regimes categorized by the combined EF ambition level and co-evolution of basin-wide water demands. It also underscores the importance of a multi-sector, multi-decadal, basin-wide approach when assessing the costs of enhanced EF allocation in water-stressed delta regions.
2 Methodology
2.1 Modeling framework and setup
This study uses an integrated economic optimization model, NEST (Vinca et al., 2020a), to co-optimize sub-national energy, water, and land (EWL) system representation over a multi-decadal time horizon. The NExus Solutions Tool (NEST) integrates the EWL framework into the capacity expansion modeling process by optimizing water, energy, and land management decisions (Vinca et al., 2020a). The objective function is the total discounted cost across all managed processes, decisions, regions, and time steps. Future costs are discounted in the objective function using an average discount rate for national planning. Input parameters include i) technology/process costs and efficiencies, ii) historical capacities (e.g., the maximum production rate), iii) resource availability, and iv) demands. The optimization decision variables are the capacity, operation, storage, and emissions rates of technologies and processes and the types of land-use from the year 2020 to 2050. The decisions include the adaptive responses needed to accommodate cross-sector interactions. For example, a NEST capacity expansion scenario has power plant capacity and fuel required to generate electricity used to pump and treat water in line with projected water demands. Likewise, the NEST considers the land needed for crops and the yield trade-offs associated with reduced irrigation. The NEST has been developed in previous work to examine sustainable development pathways for the Indus River Basin (Vinca et al., 2020b) and is adapted in this study to enable EF allocation policies at the catchment scale. Previous studies include a more technical description of the framework, and an applied use case focused on the transboundary aspects. These did not include aspects on the Indus Delta, the environmental flows, and impacts of flow allocation downstream of the IRB in the upstream. We consider this aspect a key to the sustainable development of the IRB. We also found that there is very little/limited knowledge about the standards of environmental flow allocation within the IRB either by local stakeholders or the academic community.
The NEST delineates a given study region into a combination of administrative and hydrological units. The administrative units refer to country administrative units and sub-national (administrative) boundaries, and the hydrological units refer to sub-basins (tributaries) here to account for flows within the basin and impacts of upstream water withdrawals on downstream water availability. These two spatial units are intersected using the geospatial technique to create the spatial unit for the modeling framework. The future work will include further intersection of units to agro-ecological zones to account for more informed agriculture-related decisions. An example of the delineation of the Indus River Basin is depicted in Figure 1. A reduced-form river and electricity transmission network optimize flows and reservoir releases between the spatially distributed units. Crop yields are aggregated nationally to meet projections of future production quotas. The NEST framework includes a hydrological model (CWatM) (Burek et al., 2019) that is linked to the capacity expansion module (MESSAGEix) (Huppmann et al., 2019). The linkage informs the optimization of spatially distributed water resource availability. Socioeconomic and climate data in a gridded format are also harmonized to a standard scale and used to define water, energy, and crop demands in each delineated management unit.
A high-resolution land surface (hydrological) model, CWatM (Burek et al., 2019), is calibrated to observations that simulate the vertical and horizontal freshwater transfer across terrestrial areas’ daily time scale. Climate-impacted water scenarios consistent with the emissions pathways simulated with the global IAM (integrated assessment modeling) are implemented using downscaled climate forcing variables from global climate models. The sectoral analysis is harmonized between both models to capture the historical period and a future time horizon, translating demand profiles. CWatM is initially run in baseline conditions to inform MESSAGEix of dynamic constraints on water availability, hydropower potential, and irrigation water requirements (Vinca et al., 2020a). The streamflow values obtained from CWatM output are naturalized flows without human activities and water withdrawals since these are obtained from MESSAGEix. CWatM simulates the river streamflow using the kinematic wave routing approach. It can simulate both the naturalized streamflow and streamflow impacted by human activities, i.e., reservoirs. It uses a fixed network depending on spatial resolution, and each grid cell through the network is simulated using kinetic wave equations. The basic equations include the continuity and momentum equations (Burek et al., 2019). Here, naturalized flows represent the flows without the impact of human activities, and these help in understanding the hydrological perspective of the basin from the perspective of EFs. These naturalized flows are also used for environmental assessment, as shown in Figure 2.
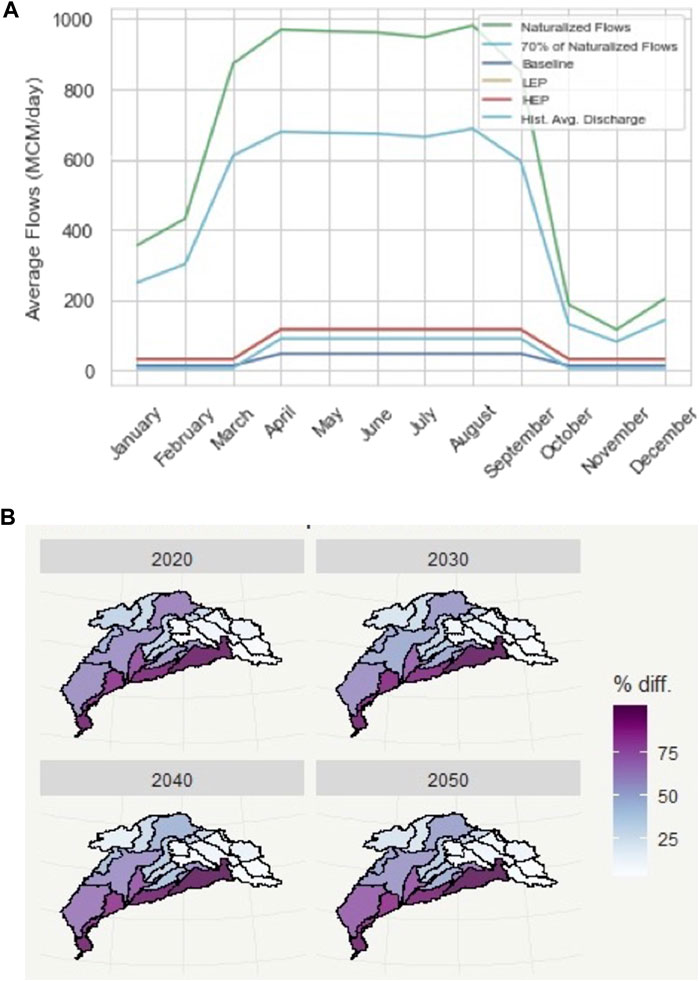
FIGURE 2. (A) Seasonal visualization of average flows per year (MCM/day) incorporated in baseline, historical average discharge downstream for the Kotri Barrage, EF scenarios, and naturalized flows. Scenario layout and description of sensitivity scenarios. (B) Map showing the difference between actual flows of the baseline scenario and naturalized flows computed from CWatM (Community Water Model) to show percentage variation between both flow types.
The resources, technological process, and demands are parameterized using data sources mentioned in Supplementary Table S2. Much of the data mentioned in Supplementary Table S2 can be obtained from globally available databases; however, for some parameters, local data are used to approve the results and calibration of the historical process using stakeholder dialogs. The range of uncertainties in Community Water Model (CWatM) hydrological modeling is covered using multiple climate models and RCP-SSP scenario ensembles. The hydrological model, CWatM, is calibrated at five arcmin resolutions considering the historical monthly flow data from 1995 to 2010 at the Besham station in the upper Indus collected from the local authorities. The station was selected due to the availability of historical data for the historical years. The availability of consistent hydrological observations in the IRB is highly complex due to rapid elevation changes within the basin (Forsythe et al., 2019) and the non-reliability of the observational data in the basin (Akhter, 2017). The calibration was carried out using the streamflow by considering storage within the basin to allow for a stable flow calculation. The calibration results showed that the streamflow was impacted by the ice melt coefficient and the empirical shape parameter of the rainfall and runoff ARNO model for infiltration (Burek et al., 2013). The second calibration searched for optimal values between parameters. The parameter values are mentioned in Supplementary Table S3. The total basin runoff results from the hydrological model match closely with the reported data (Laghari et al., 2012; Yaseen et al., 2020).
System solutions in the NEST include investments in new infrastructure capacity (e.g., power plants, water distribution, irrigation system, and land-use type) to find the least-cost solution by satisfying energy and water demands with supply. It also includes a comparison of different investment types and strategies for water infrastructure technologies, energy systems, and land use, such as constraining a given level of environmental flow in a river which gives us an integrated solution as an output under global change scenarios. The NEST co-optimizes sub-national water, energy, and land system representation over a multi-decadal time horizon. The joint optimization of resource management occurs at the technology level, considering the physical balance between supply and demand from industrial, urban, rural, and agricultural sectors. The basin is disaggregated into 24 sub-national decision-making units representing the intersection between country administrative and sub-basin boundaries (see Figure 1 for node IDs). The hydro-economic representation links techno-economic parameters across units with a reduced form of the river network. For the detailed linkages, mathematical equations, calibration, parameterization, and integration of water–energy–food sources and technologies in the modeling framework, refer to the model description study by Vinca et al. (2020a). The calibration of hydrological and data sources used in the modeling framework is mentioned in Supplementary Sections S2, S3.
2.2 Multi-sector transformation pathways
The NEST constrains the flows in the downstream nodes, i.e., below the Kotri Barrage (node 1; Figure 1) outflowing to the Arabian Sea. However, by constraining the downstream management nodes near the Indus Delta, the model allocates EFs and adjusts different EWL technologies as per the available constraints and investments upstream. As mentioned previously, no suitable standard exists to allocate EFs in the IRB. We gathered the data from local authorities and calculated the average discharge flowing downstream of the Kotri Barrage historically (1971–2018) (Supplementary Table S1) and found that the average flows reaching the Indus Delta have been decreasing continuously. Due to the complexity of the basin, we allocated suitable EFs based on the historical flows, the standard defined by the governments, and the standards in the literature in the lower IRB. The choice of percentages of allocation is made on different model runs and by analyzing the impacts of water availability in the outflow. This approach suits highly modified and regulated rivers like the IRB, where achieving an ecological potential and saving the biodiversity is a target, considering the societal demands for highly modified rivers instead of taking the natural flow regime as a reference (Acreman et al., 2014).
The NEST implementation for the IRB has been developed to examine the interplay between regional cooperation and the achievement of multiple targets consistent with the SDGs (sustainable development goals). The analysis in this paper utilizes the model setup and scenario design to explore enhanced ambition for delta EFs (Table 1). The baseline scenario optimizes water, energy, and land system costs from 2020 to 2050, with future population and GDP assumptions. The sectoral demand profiles are generated from the shared socioeconomic pathways (SSP2). The system costs include investment and operational costs for all sectors for different nodes and times. The baseline scenario incorporates sustainable development goal (SDG) policies that ensure universal access to piped water and wastewater collection and decarbonization and electrification of the energy sector in line with the Paris Agreement. These input assumptions and associated data are further summarized in Table 1.
Transformation pathways incorporating enhanced EF allocation to the Indus Delta are generated by layering an additional set of constraints on the allowable basin outflow. The NEST is used to access the impacts of environmental flow constraints in the lower Indus for future pathways. The target level is defined relative to the historical outflow levels provided by the local policy, as mentioned in Figure 2A, and projected with base-year (2015) data. Evidence from the literature shows that historical constraints in the outflow node have not been scientifically tested. The constraints in the outflow node should be tested on-field for a longer period (∼10–15 years) to test the biophysical and ecological impacts on the basin. For designing environmental flow scenarios, we designed ecological target-based scenarios based on the assumption that constraining more water in the southern nodes can potentially increase delta sustainability and improve socio-economic conditions in the Indus Delta.
Despite the critical role of EFs in Indus Delta sustainability, there is a lack of quantitative analysis linking enhanced EF allocation policies to the adaptation costs borne by upstream sectors. We carried out a parametric model analysis to find the least-cost system transformations that support the enhanced allocation of environmental flows in the downstream nodes (i.e., nodes numbers 1, 2, and 3 in Figure 1). Constraining the river levels to the naturalized flows (modeled flows without human interaction) results in extreme investment costs due to huge difference between naturalized and actual river flows (Figure 2B). The constraint makes the consumption projections infeasible due to the highly modified nature of the IRB, i.e., much of the existing flow has already been allocated, thus making the shift back to naturalized conditions highly unlikely. Therefore, we allocated EFs such that they generate an economic response function based on a range of EF constraints defined relative to the historical outflow. This provides decision-makers with an understanding of the marginal costs for enhancing EFs. We designed a low ecological potential (LEP) scenario and a high ecological potential (HEP) scenario by increasing the existing EF ambition levels by 2.5× and 5×, respectively. A visual representation of the flow levels and their variation across various times during the year can be seen in Figure 2. The increased flows during the April–September months are due to glacier melting and the monsoon season in the region. Sensitivity cases are generated to assess the variation in the model results due to uncertainties in the model parameters and policy design. The choice of sensitivities is made based on the domain of research questions, local socio-political issues, and climate mitigation against vulnerability in the region but does not encompass the full range of uncertainties. The detailed scenario representation and sensitivity details are described in Table 1.
2.3 Marginal costs for EF allocation
The marginal costs of EFs allocation help in accessing costs and opportunities for water, energy, and land systems at a specific instance. The constrained optimization problem solved in the NEST generates corresponding Lagrange multipliers representing the marginal change in the objective function value (total system costs) when varying the constraint value by a small amount. In planning operations, the Lagrange multipliers associated with demand constraints are commonly interpreted as the market-clearing commodity prices for recovering system investments. By solving the NEST across a range of delta EF constraints, the marginal costs are calculated, thus linking the delta EF allocation level to the cost of implementation. This provides an indicator for the required pricing of environmental flows that recovers the costs of the multi-sector transformation. It is of note that the socio-economic improvements from delta replenishment are not included. If these benefits exceed the costs, then implementation of the EF policy is economically net positive. These impacts are complex and highly non-linear. Future works in this area can leverage the marginal costs derived in this paper to compare the economics of implementation. This is like marginal costs used in marginal allocation curves (MACs) for emissions mitigation, where the benefits from avoided adaptation are also excluded. In this case, the marginal cost is, therefore, calculated as follows:
3 Results
3.1 Total system and marginal costs of EF allocation
The analysis is performed using SDG sustainability targets to offset these costs for EF scenario runs. The SDG constraints in the scenario show additional 10 billion USD/year investments overall across multiple sectors (Supplementary Figure S2A). These SDG targets also show additional energy required for water (pumping, desalination, etc.) and lesser water required for energy in SDG targets (Supplementary Figure S2B). These costs are already accounted for in the baseline scenario in the current study. By constraining more water in the enhanced EF scenarios, we see that approximately an additional 28 billion USD (US Dollars) and 103 billion USD per year are required across multi-sectors in the HEP scenario, as compared to the LEP scenario, distributed across sectors. Results show that Pakistan must bear all the costs for enhanced EF allocation in the lower IRB. The costs here refer to the sum of operational and investment costs from a systems perspective (Figure 3A).
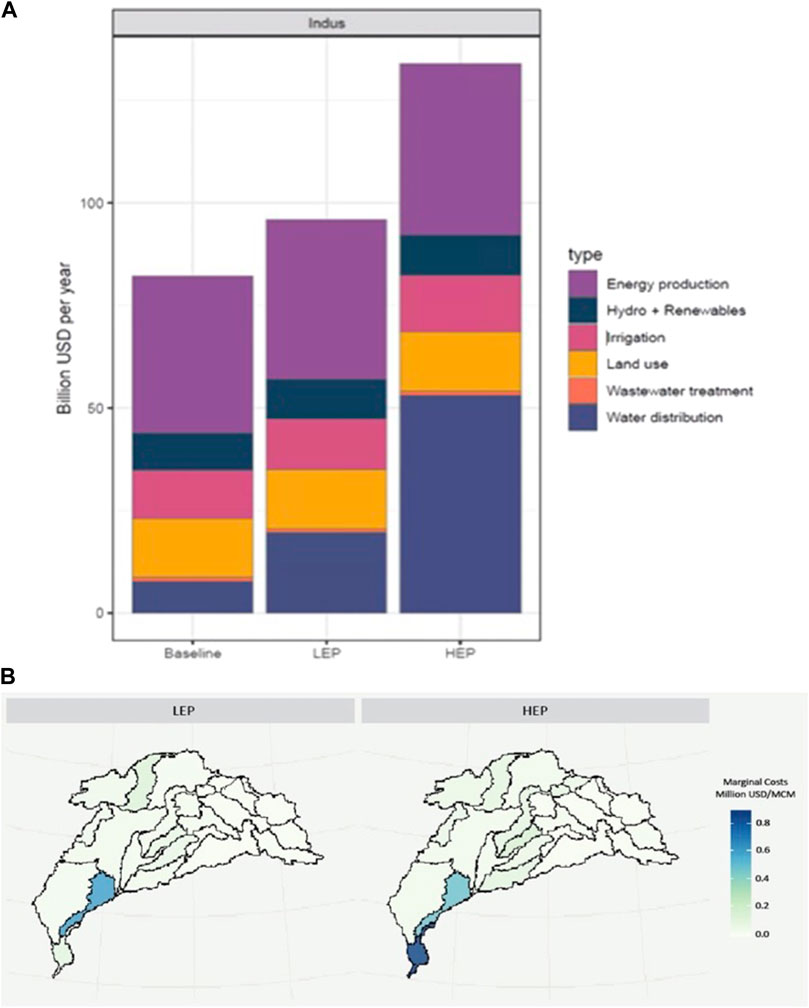
FIGURE 3. Average system costs (investment and operational) per year from the optimization model for (A) EF scenarios and (costs are indicated in USD2010 values) (B) regional marginal costs showing the regions with increase in marginals in the IRB per allocation of the EF in downstream nodes. During the post-processing phase, energy, water, and land technologies are grouped into clusters to display aggregated results. The technological clusters from Figure 3A include coal, oil, electricity import in energy production; river, canal, existing and planned hydropower projects, and renewables (solar and wind) in Hydro + RES; diversion, distribution, wastewater collection, desalination, and canal infrastructure costs in water distribution; irrigation technologies (flood, drip, sprinkler, and canals) in irrigation; and crop production and biomass costs in land use.
Marginal costs for EF allocation (as discussed in Section 2.3) depict the marginal costs (billion USD/MCM) of EF scenarios compared to the baseline scenario. The costs are aggregated costs for all time horizons and all spatial regions, with future cash flows discounted at an average rate of 7%. Nearly 3.78 and 5.44 billion USD/MCM is required in LEP and HEP scenarios for the whole river basin. The lower IRB and regions where most of the storage reservoirs are available in the IRB show comparatively higher numbers and have increased marginal costs than other regions (Figure 3B). This shows that the lower Indus must bear most of the EF allocation-related costs. The regions in the basin, including storage reservoirs, also have higher marginal costs, which show the dependency of EFs on storage reservoirs. The marginal costs are calculated here as the pricing of EF allocation increases rapidly by moving to a higher ecological target for the Indus Delta. The economic implications of this pricing are beyond the scope of this paper and will be studied in future work.
Results show the need for investments in water distribution sectors due to the increased allocation of EFs downstream. Optimizing and improving water distribution is critical for EF allocation. Water distribution includes diversion, canal, desalination, and wastewater treatment. The increased investment in water distribution also shows that proper integrated water management can enhance EF allocation. Wastewater treatment and allocation are critical for the provision of EFs in the river basin. Although a specific wastewater treatment ratio is incorporated as part of the SDG6 targets, results show that water withdrawals in the region require more investment in the wastewater treatment sector. Increased wastewater treatment not only helps increase the flows in the system but also helps ensure the water quality parameters in the river basin, which is also often neglected in the case of IRB studies. To analyze the wastewater aspect further, we analyzed the EF scenarios with an increased wastewater treatment ratio in the sensitivity analysis (see Supplementary Table S1 in supplementary data for cost comparison). The results show that an additional 5–50 billion USD/yr across scenarios is required for improved water quality parameters.
3.2 Least-cost structural changes in the energy–water–land (EWL) sectors
The optimization model shows not only the system costs but also system transformations in terms of additional capacity, shifts in water sources, energy supply, and the interactions between water, energy, and land systems. We see that average water use per year reduces in the EF scenarios from the baseline scenario; however, a major portion of water is being allocated for flood irrigation. Due to the agricultural dependency of the system, floor irrigation has been widely used, which is a highly water-consumptive irrigation type (Figure 4A). The current study motivates further research into exploring shifts to smart irrigation technologies for a sustainable river basin. The share of groundwater in the water source increases rapidly from 43% to 50% in the baseline scenario to 59–63% in the LEP and 64–66% in the HEP scenario. This shows that while allocating environmental flows downstream of the IRB, the groundwater resource is stressed due to high demands and withdrawals. The share of oil in the electricity supply by source (Figure 4B) increases in the higher EF scenario, which is not sustainable in terms of the climate vulnerability of the river basin in the future. However, considering a climate mitigation scenario, i.e., reducing total emissions by 2050 (EMISS), can help address a more sustainable option for climate mitigation along with river sustainability.
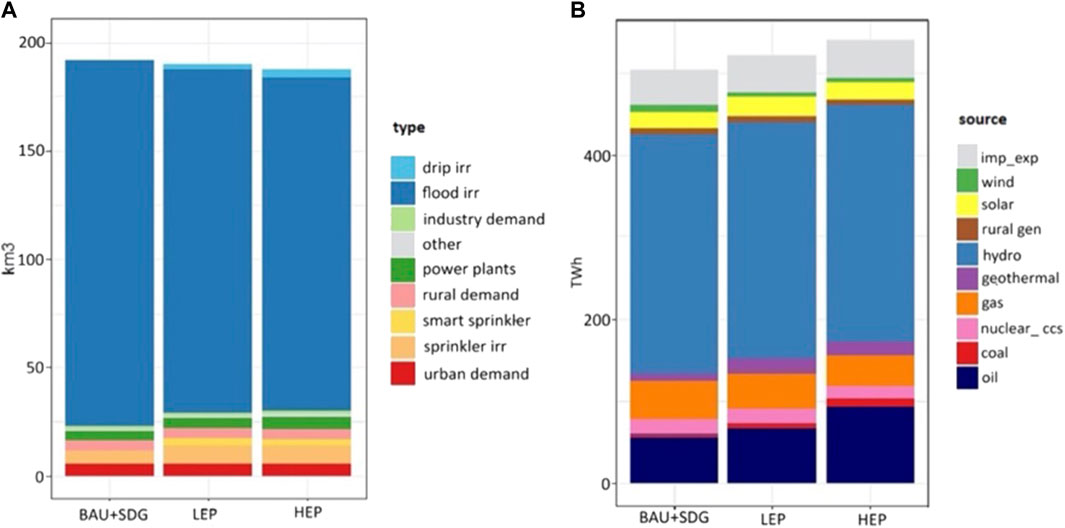
FIGURE 4. (A) Average water use per year for different sectors. (B) Electricity supply by source per year for EF scenarios/water use for different sectors and electricity supply for various sources represent nexus interactions.
The nexus interactions show that due to the increased allocation of water downstream of the IRB, we need more energy for water withdrawals and irrigation, which also increases water use for cooling power plants. This includes investments in desalination plants causing the system to consume more energy than surface runoff. The energy system transforms toward less water-consumptive technologies to save water for EF allocation. We also observed the variation of the share of water through different sources across the EF scenarios. The share of surface water throughout the Indus reduces by approx. 14–18% through the time horizon. The share of wastewater and seawater desalination increases in the HEP scenario to allocate enough water to meet sectoral demands across the IRB (Supplementary Figure S3).
3.3 Sensitivity and uncertainty
Results from sensitivity cases include the variation in agricultural food demand and electricity demands, transboundary cooperation, increased wastewater treatment, and rapid reduction in emissions across the EF scenarios (see Supplementary Table S4 for Supplementary details). Results show that variation in electricity and agricultural demands significantly impact the savings from baseline cases from 2–25%. This represents increased dependency on agricultural systems in the river basin. Since the energy system is also dependent on water in the form of hydro energy potential and cooling water for power plants, the total system costs vary with electricity demand variation in the system. Also, the increase in costs in the irrigation sector in the EMISS scenario depicts that shifts to low water and energy-consumptive irrigation technologies are key for climate mitigation in the future years. The rapid reduction in emissions under the decarbonization policy and shift toward low-carbon technologies results in a cost increase in water distribution, renewables, and irrigation. However, when trade barriers are relaxed in the transboundary cooperation scenario (COOP), the average system costs per year significantly reduce in the LEP and HEP scenarios. This is because there are significant opportunities for irrigation water efficiency in the Indian portion of the river basin, and these investments increase the amount of flow that transfers to Pakistan and drains into the Indus Delta.
Additional uncertainties in the analysis and not covered by the scenario setup include the influence of future climate extremes on water availability and flood management in the IRB. There is also a need to meet EF guidelines in all basin tributaries and for groundwater systems, but these spatially distributed EF constraints were not considered. These aspects create additional management challenges and are expected to increase the cost of securing EFs in the delta region.
4 Discussion
The study accesses the role of freshwater provision downstream of the IRB and its importance for the replenishment of the delta. It uses an engineering economic optimization framework to access the prices, impacts, and structural shifts required within the water, energy, and land systems of the IRB. Based on the historical flows reaching the Indus Delta, the standard defined by the policymakers, and the model responses for upstream water allocation, we identify optimal flows that form a narrative for the environmental flow requirements for the lower IRB and, thus, could potentially help replenish the Indus Delta. From the results, we found out that the delta flows can be improved in the model by 1) investing in water efficiency measures in the irrigation, water treatment, conveyance, and power plant cooling sectors; and 2) investing in alternative supply-side measures, including extraction from groundwater resources, advanced water treatment, and new conveyance canals. The allowable flow constraints are implemented from 2030 onward in the model to reflect the SDG timeline. The flow constraints are varied over a range of ambition levels to provide an indicator of marginal cost implications and the value of delta flows derived from an infrastructure investment perspective.
Allocation of environmental flows to replenish the Indus Delta using a designer approach causes an increase in system costs from 17 to 32% in the LEP to 68–72% in the HEP scenario across multiple sectors from the baseline scenario. We propose that the system cost representation for EF scenarios and sensitivities can help determine the scale of investments across different uncertainties for policymakers and river planners aiming to assess the level at which the allocation of EFs can be achieved in the future. Although investments increase as more water is allocated for EFs downstream, these EFs protect the Indus Delta from serious long-term ecological threats. Thus, some trade-offs need to be considered by regional planners to find the right balance between EF allocation and financial sustainability. This study provides river planners with insights into the scale of costs for water, energy, and land sectors and which technologies in the system can be leveraged together to replenish the delta in a least-cost way.
The linkage between the upper and lower Indus and a better understanding of extreme climate change impacts and impacts on water, energy, and land resources are complex in the IRB (Shrestha et al., 2021). The significant climate variation from the high-altitude snow-capped to the sub-tropical climates in the lower IRB makes the IRB extremely sensitive to climate change variability (Krishnan et al., 2019). EF allocation in the IRB is dependent on the surface water availability from the upper Indus, as mentioned in previous sections, whereas dependence of surface water in the IRB on glacial meltwater and snowmelt poses dire consequences on surface water availability (Lutz et al., 2019). Due to higher glacial melt, the river flow will increase and cause more frequent flooding and droughts, which pose threats to river sustainability in the future.
The findings also demonstrated how the total system costs for hydropower and renewable energy are impacted by the change in the electricity demands among the EF scenarios. The cooperation of the riparian nations enables the basin to lower system-wide operating expenses and provide more water to rehydrate the Indus Delta. Since a large portion of the water in the IRB is supplied by flooded irrigation, the system is extremely sensitive to the demand for agricultural food. Although the EF scenarios that ensure increased water availability in the downstream regions are the main focus of discussion, we propose looking into the sensitivity cases presented in this paper for additional research and policy analysis, such as how improved wastewater treatment can not only help increase water availability but also improve water quality.
5 Conclusion
Evidence from multiple literature studies show that the Indus Delta ecosystem has experienced losses in fisheries, economical gains, and mangroves due to human-induced stressors in the past years. Also, it has been observed in studies that the decrease in freshwater reaching delta and sediments is one of the major causes of the damage to the Indus Delta (Salik et al., 2015; Salik et al., 2016; Kidwai et al., 2016; Kidwai et al., 2019). This study assesses the multi-sectoral impacts of provision of freshwater to the Indus Delta and associated costs in the water, energy, and land sectors. This study also lays out the need for defining the environmental flow requirements within the basin and its importance for replenishing the Indus Delta. A standard for environmental flows is yet to be defined by basin practitioners in the IRB, and a detailed eco-hydrological study is needed to determine the ecosystem response to the EF policies. The delta EF constraints could cause stress on groundwater resources in the river system if groundwater is used to mitigate surface water withdrawals in response to EF policies. EF standards need to consider a sound groundwater withdrawal rate for ecological protection (Gleeson and Richter, 2018; Driver et al., 2020). Moreover, hydropower energy generation decreases when a higher EF target is achieved in the system, which increases energy costs at the user-end. River basins that have the potential for storage expansion might be able to reduce these interactions by modifying the flow pattern. Ruan et al. (2021) mentioned a similar conflict between hydropower projects and impacts on environmental flows using a case study from China. Hydropower expansion plans in the upper IRB raise concerns for downstream water availability and have implications on socio-economic conditions near the Indus Delta. Dhaubanjar et al. (2021) developed a systematic framework for the sustainable assessment of hydropower potential in the IRB. We propose that future studies on environmental flows may explore the synergies and trade-offs between hydropower expansion in the upper IRB and the impacts on hydrological and socioeconomics in the lower IRB. The multi-sector, multi-scale modeling framework leveraged in this paper can support this type of analysis and will be the topic of future research. EF allocation in the IRB is crucial for ecosystem sustainability and deltaic protection. The current policy structure of water allocation lacks scientific evidence for EFs. Considering the competing water uses in agriculture, municipal, industry, energy, and ecosystem services, it is critical for stakeholders to build a consensus on the allocation of water for EFs. This study accesses the EF requirements based on the integrated impacts on EWL sectors using an innovative approach by repetitive model runs to see the impacts of the hydrological and socio-economic conditions in the upper Indus and lower Indus. However, we think that implementing EF in the IRB requires adaptive management techniques, i.e., a learning-by-doing approach. The stakeholders need to negotiate the objectives and outcomes of the EF allocations for the river basin sustainability. Also, the integrated impacts of EF allocation provided critical choices in the nexus, including over infrastructures such as dams, managing abstractions for irrigation, and allocation of water for ecosystems. With this study, we propose that there is a need for more focused and on-site experiments to develop environmental flow standards. These are critical for the vulnerability of the IRB to climate change impacts, and they will help the IRB adapt to the rapidly changing and uncertain climate in the basin. The 1991 Water Apportionment Accord for sharing water among different provinces is ambiguous and requires clear interpretation to allow fair water allocation downstream of the IRB for the socio-economic well-being and replenishment of the delta.
Data availability statement
The datasets presented in this study can be found at: https://github.com/iiasa/NEST.
Author contributions
MA: conceptualization, methodology, software, formal analysis, writing—original draft, writing—review and editing, and visualization. AV: conceptualization, methodology, software, writing—review and editing, and visualization. SP: conceptualization, methodology, and writing—review and editing. MM: supervision and writing—review and editing. EB: conceptualization and methodology. BW: writing—review and editing. AM: writing—review and editing. KR: supervision.
Acknowledgments
The authors acknowledge the Global Environment Facility (GEF) for funding the development of this research as part of the Integrated Solutions for Water, Energy, and Land (ISWEL) project (GEF Contract Agreement: 6993), the support of the United Nations Industrial Development Organization (UNIDO) and NSERC Discovery Grant.
Conflict of interest
The authors declare that the research was conducted in the absence of any commercial or financial relationships that could be construed as a potential conflict of interest.
Publisher’s note
All claims expressed in this article are solely those of the authors and do not necessarily represent those of their affiliated organizations, or those of the publisher, the editors, and the reviewers. Any product that may be evaluated in this article, or claim that may be made by its manufacturer, is not guaranteed or endorsed by the publisher.
Supplementary material
The Supplementary Material for this article can be found online at: https://www.frontiersin.org/articles/10.3389/fenvs.2022.958101/full#supplementary-material
References
Acreman, M., Arthington, A. H., Colloff, M. J., Couch, C., Crossman, N. D., Dyer, F., et al. (2014). Environmental flows for natural, hybrid, and novel riverine ecosystems in a changing world. Front. Ecol. Environ. 12 (8), 466–473. doi:10.1890/130134
Akhter, M. (2017). Desiring the data state in the Indus Basin. Trans. Inst. Br. Geogr. 42 (3), 377–389. doi:10.1111/TRAN.12169
Ali, G., Ashraf, A., Bashir, M. K., and Cui, S. (2017). Exploring environmental kuznets curve (ekc) in relation to green revolution: A case study of Pakistan. Environ. Sci. Policy 77, 166–171. doi:10.1016/j.envsci.2017.08.019
Anwar, A. A., and Bhatti, M. T. (2017). Pakistan’s water apportionment Accord of 1991: 25 Years and beyond. J. Water Resour. Plan. Manag. 144 (1), 05017015. doi:10.1061/(asce)wr.1943-5452.0000831
Anwar, M., Chandio, N., and Bhalli, M. (2014). Economic deprivation of Indus river delta, Sindh, Pakistan: Causes and suggestions. Sci. Int. (Lahore), 885–890. (January 2014).
Arthington, A. H. (2012). Environmental flows: Saving rivers in the third millennium. Univ of California Press.
Burek, P., Satoh, Y., Kahil, T., Tang, T., Greve, P., Smilovic, M., et al. (2019). Development of the Community Water Model (CWatM v1.04) A high-resolution hydrological model for global and regional assessment of integrated water resources management. Geosci. Model Dev. Discuss., 1–49. doi:10.5194/gmd-2019-214
Burek, P., van der Kniff, J., and Roo, A. D. (2013). LISFLOOD, distributed water balance and flood simulation model - publications Office of the EU. Available at: https://op.europa.eu/en/publication-detail/-/publication/e3b3c713-c832-4614-8527-f3ab720192f8/language-en (Accessed: July 4, 2022).
Byers, E., Gidden, M., Leclere, D., Balkovic, J., Burek, P., Ebi, K., et al. (2018). Global exposure and vulnerability to multi-sector development and climate change hotspots. Environ. Res. Lett. 13 (5), 055012. doi:10.1088/1748-9326/aabf45
Cazcarro, I., and Dilekli, N. (2021). Developing the food, water, and energy nexus for food and energy scenarios with the world trade model. Water 1313 (17), 2354. Page 2354. doi:10.3390/W13172354
Day, J., Goodman, R., Chen, Z., Hunter, R., Giosan, L., and Wang, Y. (2021). Deltas in arid environments. Water 13 (12), 1677. Page 1677. doi:10.3390/W13121677
Dhaubanjar, S., Lutz, A. F., Gernaat, D. E., Nepal, S., Smolenaars, W., Pradhananga, S., et al. (2021). A systematic framework for the assessment of sustainable hydropower potential in a river basin – the case of the upper Indus. Sci. Total Environ. 786, 147142. doi:10.1016/j.scitotenv.2021.147142
Driver, L. J., Cartwright, J. M., Knight, R. R., and Wolfe, W. J. (2020). Species-richness responses to water-withdrawal scenarios and minimum flow levels: Evaluating presumptive standards in the Tennessee and cumberland river basins. Water 12 (5), 1334. Page 1334. doi:10.3390/W12051334
Ericson, J. P., Vorosmarty, C., Dingman, S., Ward, L., and Meybeck, M. (2006). Effective sea-level rise and deltas: Causes of change and human dimension implications. Glob. Planet. Change 50 (1–2), 63–82. doi:10.1016/j.gloplacha.2005.07.004
Forsythe, N., Archer, D. R., Pritchard, D., and Fowler, H. (2019). A hydrological perspective on interpretation of available climate projections for the upper Indus Basin. Indus River Basin Water Secur. Sustain. 2019, 159–179. doi:10.1016/B978-0-12-812782-7.00008-4
Gleeson, T., and Richter, B. (2018). How much groundwater can we pump and protect environmental flows through time? Presumptive standards for conjunctive management of aquifers and rivers. River Res. Appl. 34 (1), 83–92. doi:10.1002/rra.3185
González-villareal, F., and Schultz, B. (2005) “Final report of IPOE for review studies on water escapages below Kotri barrage,” in Hydraulic engineering. doi:10.13140/RG.2.2.28670.02885
Hannaford, J. (2015). Climate-driven changes in UK river flows: A review of the evidence. Prog. Phys. Geogr. Earth Environ. 39 (1), 29–48. doi:10.1177/0309133314536755
Hill Clarvis, M., Allan, A., and Hannah, D. M. (2014). Water, resilience and the law: From general concepts and governance design principles to actionable mechanisms. Environ. Sci. Policy 43, 98–110. doi:10.1016/j.envsci.2013.10.005
Hirji, R., and Davis, R. (2009). Environmental flows in water resources policies, plans, and projects. Fidings and recommendations. Environ. Health. doi:10.1016/j.jenvman.2008.06.005
Huppmann, D., Gidden, M., Fricko, O., Kolp, P., Orthofer, C., Pimmer, M., et al. (2019). The MESSAGE Integrated Assessment Model and the ix modeling platform (ixmp): An open framework for integrated and cross-cutting analysis of energy, climate, the environment, and sustainable development. Environ. Model. Softw. 112, 143–156. doi:10.1016/j.envsoft.2018.11.012
IRSA (1991). Water apportionment Accord. Islamabad. Available at: http://pakirsa.gov.pk/WAA.aspx.
Khan, Z., Linares, P., and García-González, J. (2017). Integrating water and energy models for policy driven applications. A review of contemporary work and recommendations for future developments. Renew. Sustain. Energy Rev. 67, 1123–1138. doi:10.1016/j.rser.2016.08.043
Kidwai, S., Waqar, A., Syed, M. T., Jing, Z., Liviu, G., Clift, P. D., et al. (2019). The Indus delta-catchment, river, coast, and people, coasts and estuaries: The future. Elsevier. doi:10.1016/B978-0-12-814003-1.00012-5
Kidwai, S., Fanning, P., Ahmed, W., Tabrez, M., Zhang, J., and Khan, M. W. (2016). Practicality of marine protected areas - can there be solutions for the River Indus delta? Estuar. Coast. Shelf Sci. 183, 349–359. doi:10.1016/J.ECSS.2016.09.016
Krishnan, R., Shrestha, A. B., Ren, G., Rupak, R., Saeed, S., Sanjay, J., et al. (2019). “Unravelling climate change in the Hindu kush himalaya: Rapid warming in the mountains and increasing extremes,” in The Hindu kush himalaya assessment: Mountains, climate change, sustainability and people. Editor P. Wester. (Cham: Springer International Publishing), 57–97. doi:10.1007/978-3-319-92288-1_3
Laghari, A. N., Vanham, D., and Rauch, W. (2012). The Indus basin in the framework of current and future water resources management. Hydrol. Earth Syst. Sci. 16 (4), 1063–1083. doi:10.5194/hess-16-1063-2012
LeRoy Poff, N., Allan, J. D., Bain, M. B., Karr, J. R., Prestegaard, K. L., Richter, B. D., et al. (1997). The natural flow regime. BioScience 47 (11), 769–784. doi:10.2307/1313099
Lutz, A. F., ter Maat, H. W., Wijngaard, R. R., Biemans, H., Syed, A., Shrestha, A. B., et al. (2019). South Asian river basins in a 1.5 °C warmer world. Reg. Environ. Change 19 (3), 833–847. doi:10.1007/s10113-018-1433-4
Memon, J. A., and Thapa, G. B. (2016). Explaining the de facto open access of public property commons: Insights from the Indus Delta mangroves. Environ. Sci. Policy 66, 151–159. doi:10.1016/j.envsci.2016.08.014
O’Neill, B. C., Kriegler, E., Ebi, K. L., Kemp-Benedict, E., Riahi, K., Rothman, D. S., et al. (2017). The roads ahead: Narratives for shared socioeconomic pathways describing world futures in the 21st century. Glob. Environ. Change 42, 169–180. doi:10.1016/j.gloenvcha.2015.01.004
Parkinson, S. C., Makowski, M., Krey, V., Sedraoui, K., Almasoud, A. H., and Djilali, N. (2018). A multi-criteria model analysis framework for assessing integrated water-energy system transformation pathways. Appl. Energy 210, 477–486. doi:10.1016/j.apenergy.2016.12.142
Pastor, A. V., Ludwig, F., Biemans, H., Hoff, H., and Kabat, P. (2014). Accounting for environmental flow requirements in global water assessments. Hydrol. Earth Syst. Sci. 18 (12), 5041–5059. doi:10.5194/hess-18-5041-2014
Rasul, G. (2014) Food, water, and energy security in South Asia: A nexus perspective from the Hindu kush himalayan region. Environ. Sci. Policy 39, 35–48. doi:10.1016/j.envsci.2014.01.010
Richter, B. D. (2009). Re-Thinking environmental flows: From allocations and reserves to sustainability boundaries. River Res. Appl. 26, 1052–1063. doi:10.1002/rra.1320
Richter, B. D., Warner, A. T., Meyer, J. L., and Lutz, K. (2006). A collaborative and adaptive process for developing environmental flow recommendations. River Res. Appl. 22 (3), 297–318. doi:10.1002/rra.892
Ruan, Q., Wang, F., and Cao, W. (2021). Conflicts in implementing environmental flows for small-scale hydropower projects and their potential solutions—a case from fujian province, China. Water 13 (18), 2461. Page 2461. doi:10.3390/W13182461
Salik, K. M., Hashmi, M. Z. U. R., Ishfaq, S., and Zahdi, W. U. Z. (2016). Environmental flow requirements and impacts of climate change-induced river flow changes on ecology of the Indus Delta, Pakistan. Regional Stud. Mar. Sci. 7, 185–195. doi:10.1016/j.rsma.2016.06.008
Salik, K. M., Jahangir, S., Zahdi, W. U. Z., and Hasson, S. U. (2015). Climate change vulnerability and adaptation options for the coastal communities of Pakistan. Ocean Coast. Manag. 112, 61–73. doi:10.1016/J.OCECOAMAN.2015.05.006
Shrestha, A. B., Shukla, D., Pradhan, N. S., Dhungana, S., Azizi, F., Memon, N., et al. (2021). Developing a science-based policy network over the upper Indus Basin. Sci. Total Environ. 784, 147067. doi:10.1016/j.scitotenv.2021.147067
Siddiqi, A., and Wescoat, J. L. (2013). Energy use in large-scale irrigated agriculture in the Punjab province of Pakistan. Water Int. 38 (5), 571–586. doi:10.1080/02508060.2013.828671
Syvitski, J., Day, J., de Sherbinin, A., Giosan, L., and Paola, C. (2009). Battling to save the world’s river deltas. Bull. Atomic Sci. 65 (2), 31–43. doi:10.2968/065002005
Syvitski, J. P. M., Kettner, A. J., Overeem, I., Giosan, L., Brakenridge, G. R., Hannon, M., et al. (2013). Anthropocene metamorphosis of the Indus Delta and lower floodplain. Anthropocene 3, 24–35. doi:10.1016/j.ancene.2014.02.003
Tharme, R. E. (2003). A global perspective on environmental flow assessment: Emerging trends in the development and application of environmental flow methodologies for rivers. River Res. Appl. 19 (5–6), 397–441. doi:10.1002/rra.736
Tilmant, A., Beevers, L., and Muyunda, B. (2010). Restoring a flow regime through the coordinated operation of a multireservoir system: The case of the Zambezi River basin. Water Resour. Res. 46 (7), 1–11. doi:10.1029/2009WR008897
van Vuuren, D. P., Edmonds, J., Kainuma, M., Riahi, K., Thomson, A., Hibbard, K., et al. (2011). The representative concentration pathways: An overview. Clim. Change 109 (1), 5–31. doi:10.1007/s10584-011-0148-z
Vinca, A., Parkinson, S., Byers, E., Burek, P., Khan, Z., Krey, V., et al. (2020a). The NExus Solutions Tool (NEST) v1.0: An open platform for optimizing multi-scale energy-water-land system transformations. Geosci. Model Dev. 13 (3), 1095–1121. doi:10.5194/gmd-13-1095-2020
Vinca, A., Parkinson, S., Riahi, K., Byers, E., Siddiqi, A., Muhammad, A., et al. (2020b). Transboundary cooperation a potential route to sustainable development in the Indus basin. Nat. Sustain. 4, 331–339. doi:10.1038/s41893-020-00654-7
Wu, M., Wu, H., Warner, A. T., Li, H., and Liu, Z. (2021). Informing environmental flow planning through landscape evolution modeling in heavily modified urban rivers in China. Water 1313 (22), 3244. Page 3244. doi:10.3390/W13223244
Yang, Y. C. E., Brown, C. M., Yu, W. H., and Savitsky, A. (2013). An introduction to the IBMR, a hydro-economic model for climate change impact assessment in Pakistan’s Indus River basin. Water Int. 38 (5), 632–650. doi:10.1080/02508060.2013.830691
Yang, Y. C. E., Ringler, C., Brown, C., and Mondal, M. A. H. (2016). Modeling the agricultural water–energy–food nexus in the Indus River basin, Pakistan. J. Water Resour. Plan. Manag. 142 (12), 04016062. doi:10.1061/(asce)wr.1943-5452.0000710
Yarnell, S. M., Petts, G. E., Schmidt, J. C., Whipple, A. A., Beller, E. E., Dahm, C. N., et al. (2015). Functional flows in modified riverscapes: Hydrographs, habitats and opportunities. BioScience 65 (10), 963–972. doi:10.1093/biosci/biv102
Keywords: environmental flows, ecosystem adaptation, water–energy–food nexus, integrated assessment modeling, sustainability
Citation: Awais M, Vinca A, Parkinson S, McPherson M, Byers E, Willaarts B, Muhammad A and Riahi K (2022) Replenishing the Indus Delta through multi-sector transformation. Front. Environ. Sci. 10:958101. doi: 10.3389/fenvs.2022.958101
Received: 31 May 2022; Accepted: 04 November 2022;
Published: 09 December 2022.
Edited by:
Xudong Zhou, The University of Tokyo, JapanReviewed by:
Joao Paulo Moura, University of Trás-os-Montes and Alto Douro, PortugalKe Jack Ding, IB Environmental, United States
Copyright © 2022 Awais, Vinca, Parkinson, McPherson, Byers, Willaarts, Muhammad and Riahi. This is an open-access article distributed under the terms of the Creative Commons Attribution License (CC BY). The use, distribution or reproduction in other forums is permitted, provided the original author(s) and the copyright owner(s) are credited and that the original publication in this journal is cited, in accordance with accepted academic practice. No use, distribution or reproduction is permitted which does not comply with these terms.
*Correspondence: Muhammad Awais, YXdhaXNAaWlhc2EuYWMuYXQ=