- State Key Laboratory of Soil Erosion and Dryland Farming on the Loess Plateau, Northwest A & F University, Yangling, China
Litter decomposition promotes soil carbon and nitrogen cycling and is driven by litter quality, the soil environment and enzyme activities. The relative importance of these factors may change during the litter decomposition, however, very few studies have emphasized the temporal dynamics of these factors across plantation ecosystem, which limits our understanding of litter decomposition. To evaluate the temporal dynamic of above-mentioned litter decomposition drivers, we collected leaf and fine root litters from four different years of restoration of Robinia pseudoacacia on the Loess plateau of China and placed them on soil from the corresponding sites to incubate for 210 days. We constructed successive litter decomposition stages according to litter mass-loss interval, and we also used partial least squares path modelling (PLSPM) to evaluate the relative importance of these drivers. Our results showed that the C and N losses in leaf litter were significantly higher than those in root litter regardless of stand age. Leaf litter C and N losses increased with restoration duration, while root litter C and N showed an opposing trend with restoration duration, with the lowest levels of losses occurring at older stand ages. The initial litter quality, litter quality and the soil environment regulated leaf and root litter C loss, and enzyme activity also determined root C loss. Litter quality, the soil environment and enzyme activity influence leaf litter N loss, while root N loss was controlled by initial litter quality and the soil environment. Overall, enzyme activities had a relatively weak influence on litter C and N losses, and they impacted litter C and N losses only during the early stages. Therefore, our results revealed substantial differences in different restoration durations and litter types at the different decomposition stages, which has important significance for understanding carbon and nitrogen cycling on the Loess Plateau of China.
1 Introduction
Litter decomposition is a key process in carbon and nutrient cycling in the terrestrial system (Krishna and Mohan, 2017; Prescott and Vesterdal, 2021). A classic theory suggests that litter decomposition is regulated by climate, litter quality and soil organisms (Bradford et al., 2016; Couˆ;teaux et al., 1995), while at the local scale, litter decomposition is driven by litter types, litter quality, soil conditions and enzyme activities (Zhang et al., 2008; Chen et al., 2018; Lin et al., 2020; Tan et al., 2020). Owing to different morphological characteristics, chemical components and decomposition microclimates, the effects of leaf and root litter on decomposition rates may vary (Sun et al., 2018; Jiang et al., 2019). Although many studies have tried to explore litter decomposition and its driving factors (Singer et al., 2014; Veen et al., 2015), they have primarily focused on aboveground litter decomposition. Root litter is estimated to account for 48% of annual plant litter input, which is higher than the proportion accounted for by leaf litter input (Freschet et al., 2013). Within the root, fine root (d < 2 mm) is an active component with a high turnover rate (McCormack et al., 2015); therefore, it is the largest contributor to root litter (Xia et al., 2015). However, a comprehensive understanding of fine-root decomposition relative to aboveground litter is currently lacking.
The Grain for Green Project was implemented in 1999 and has played an important role in vegetation restoration in China (Fu et al., 2017). Vegetation restoration can alter the above factors driving decomposition (Zhang et al., 2019; Xu et al., 2020), thereby influencing litter decomposition and carbon and nitrogen cycling in ecosystems. Previous studies have demonstrated that the litter decomposition rate gradually decreases over the course of vegetation restoration. For example, the increasing of C concentration and C:P ratio in litter had driven the decline of litter decomposition (Zhang et al., 2013). Furthermore, the increase of soil nutrient availability may cause a decrease in microbial C decomposition genes, which could decrease litter decomposition rate (Zhong et al., 2017). However, there are studies showing opposing evidence. One report indicated that improvements in litter substrate quality accelerated leaf litter decomposition rates with vegetation restoration (Zhang et al., 2021), while another showed that leaf litter decomposition is not affected by stand age (Li et al., 2020). The changing patterns and influencing factors of litter decomposition during vegetation restoration are diverse and complex. At present, there is still a knowledge gap about how leaf litter and fine root decomposition respond to R. pseudoacacia plantation restoration, which affects our understanding of nutrient cycle in plantation ecosystems.
The greatest differences in litter decomposition can be explained by litter quality, the soil environment and enzyme activities (Gunina et al., 2019; Lin et al., 2019; Joshi and Garkoti, 2021). Generally, high-quality litter (with higher nitrogen and phosphorous contents and lower lignin/N) tends to degrade (Guo et al., 2021; Eastman et al., 2022); soil nitrogen availability can increase or decrease microbial activities and respiration rates and then influence litter nitrogen release (Ge et al., 2013; Martínez-García et al., 2021). In addition, time changes are vital for litter decomposition. Generally, water soluble components and a small amount of unstable organic components released at the early stage (Liu et al., 2021), and then litter will eventually converge towards a common chemistry (Wickings et al., 2012). Therefore, it is considered that litter decomposition is driven by the metabolism of active components. At the same time, litter quality also plays a pivotal role in litter loss at late decomposition stages due to legacy effect (García-Palacios et al., 2016; Liao et al., 2022). These indicated that litter quality exerts an important effect at the different stages of decomposition. Meanwhile, enzyme activities can be potentially associated with the stages of litter decomposition (Kourtev et al., 2002). The production of soil enzyme is a trade-off in the process of litter decomposition, microbes allocate energy and nutrient by adjusting soil enzyme production (Bai et al., 2021). Previous study showed that N and P acquisition enzyme activities increased at the middle and late stage of decomposition, which indicated microbial nutrient demand increased (Kourtev et al., 2002). However, research on the relative contribution of each driver to the decomposition process is relatively lacking.
Here, we collected leaf and fine root litter of Robinia pseudoacacia in four different restoration durations and incubated the litter in the corresponding soil. The decomposition incubation experiment was conducted in the climate chamber for 210 days to validate the following hypotheses: 1) leaf litter has a higher decomposition rate than fine-root decomposition, which may be related to the higher initial litter nutrient content and soil nutrient availability; 2) the decomposition of different types of litter is regulated by trait, soil conditions, and decomposer; hence, leaf and root litter decomposition may not be coordinated across vegetation restoration; and 3) initial litter quality plays an important role in the early stages, while litter quality, the soil environment, and enzyme activities play crucial roles in the late stages. We evaluated the direct and indirect influences of initial litter quality, litter quality, the soil environment and enzyme activities on leaf and root litter decomposition and assessed the relative importance of these factors in different decomposition stages.
2 Materials and methods
2.1 Study site
The sample site was located at Huaiping Forest Farm in Yongshou County, Shaanxi Province, Northwest China, which is located in the southern Loess Plateau (34°12′12″-34°50′50″N, 108°05′7″-108°05′11″E) (Figure 1). The climate in this area is continental warm-temperature monsoon. The mean annual temperature is approximately 10.8°C, with a mean of −2.9°C in January and 23.7°C in July. The mean annual rainfall is approximately 605 mm, which places this area in the subhumid climate zone. The altitude ranges from 1,123 m to 1,464 m. All surveyed soils were derived from the same parent materials but differed in planting time. Since 1951, large areas of cropland have been converted into Robinia pseudoacacia and Pinus tabulaeformis plantations to reduce water loss and soil erosion (Liu et al., 2020). We then investigated the sites. The understorey vegetation had recovered well, and the dominant herb species were Rubus parvifolius, Aster tataricus, Humulus scandens, Leonurus japonicus, Carpesium abrotanoides, Artemisia argyi, Chenopodium album and Artemisia selengensis.
2.2 Sample collection
In this study, space instead of time was used to study the vegetation restoration sequence. The different periods of agricultural abandonment permitted the formation of a relatively comprehensive chronosequence for R. pseudoacacia. Four R. pseudoacacia plantations with different restoration durations (15 years–45 years) were determined by reviewing the relevant literature and talking to local elders. A global positioning system (GPS) was used to measure the longitude and latitude, altitude, slope and aspect. Site information for each different stand age of Robinia pseudoacacia plantations is listed in Table 1.
Three 20 m × 20 m plots were randomly established at each stand age. We collected fresh senesced leaves, fine roots, and soil samples from stands of R. pseudoacacia of different ages. New, naturally fallen leaves were collected and mixed with leaf litter from the same plots. We dug through the topsoil to collect the quantity of fine roots needed for the study. We distinguished the roots of R. pseudoacacia from other herbaceous roots based on the colour, smell and nodules. Vernier callipers were used to measure root diameter; those with diameters less than 2 mm were chosen. Similarly, fine root samples from the same plot were mixed. Finally, all leaf and root litters were brought to the laboratory, cleaned in distilled water, and dried to a constant weight at 60°C. One portion of each litter was used for measuring basic properties, and the other portion was stored at an appropriate temperature for subsequent incubation.
In addition to collecting plant samples, we also collected the corresponding soil samples for each stand age. We drilled the topsoil to a depth of 0–20 cm (by removing the surface litter and humus in each plot). Nine soil samples were evenly pooled in each plot to generate 12 soil sample mixtures. All 12 samples were sieved through 2 mm sieves to remove roots and other debris. A proportion of the fresh soil sample was stored at 4°C to measure soil enzyme activities, and the remaining soil sample was used for performing fine-root decomposition experiments. Other air-dried soil was used for further laboratory analysis.
2.3 Incubation experimental design
We performed a 210-day incubation experiment in microcosms (10 cm in height and 9 cm in diameter) with small pots, where litter from each stand age was incubated in the corresponding soil. Mesh nylon cloth was laid flat in the bottom of each pot to prevent soil loss. Each plot was equipped with fresh soil equivalent to 300 g of oven-dried soil. Then, we constructed 10 × 10 cm litterbags for leaf litter decomposition, and each litterbag was filled with 3 g of oven-dried foliar litter. In addition, 150-µm nylon mesh litterbags (7.5 cm *7.5 cm) were filled with 2 g of oven-dried root litter. The size of the litterbags allowed microorganisms to pass through while preventing litter loss during decomposition. The leaf litter was placed on the surface soil, and the root litter was buried in the soil at a depth of 4–5 cm. In total, 144 litterbags (4 stand ages × 2 litter types × 3 replicates × 6 sampling events) were placed across the plots in 2020. Every 2 days, the pots were weighed to maintain 60%–70% field capacity. Litter decomposition incubation was conducted in a dark, closed, and humid climate chamber at 25°C. On days 15, 30, 70, 112, 154, and 210 of the experiment, three pots were removed from each stand age, and a total of 24 nylon bag samples were obtained for each sampling period. Then, the litter was dried in an oven to a constant weight at 60°C to measure the elemental content, and the corresponding soil samples were collected to measure enzyme activities and other basic physical and chemical properties.
2.4 Litter, soil properties and enzyme activities analyses
The carbon contents of leaf and root litters were digested in K2Cr2O4–H2SO4, heated in an oil bath, and titrated with FeSO4 solution. Using H2SO4-H2O2 digestion, the litter total nitrogen content was measured by a Kjeldahl analyser (Kjeltec 2300 Analyser Unit, Foss Tecator, Hoganas, Sweden), and the litter total phosphorus content was measured with a spectrophotometer (U-2800 spectrophotometer, China, Shanghai). The cellulose, hemicellulose and lignin components of the litters were extracted in a series of neutral and acid detergents and analysed in accordance with the National Energy Laboratory Procedure (NREL) (Rowland and Roberts, 1994). All leaf and root litter chemical properties are shown in Supplementary Table S1.
Soil particles were analysed with a laser particle size analyser (Malvern Instruments, Malvern, England). The soil pH was measured with pH metres with a specific soil/water ratio (1:2.5; w/v), the soil water content (SWC) was measured by the drying method in an oven, and the soil bulk density (BD) was determined by the soil core method. The soil organic carbon content (SOC) was assayed by the K2Cr2O4 oxidation process. The soil total nitrogen content (STN) was determined by the Kjeldahl method, and the soil phosphorus content (STP) was analysed by the HCLO4-H2SO4 method. The soil soluble carbon (DOC) and nitrogen (DON) levels were measured by a total organic carbon (TOC) analysis instrument (Vario TOC, German Elementar Company), and the ammonium nitrogen (NH4+-N) and nitrate nitrogen (NO3−-N) contents were measured by using a continuous flow analysis system (Autoanalyzer 3, Bran and Luebbe, Germany). The soil available phosphorus (SAP) content was extracted by 0.5 M NaHCO3 and then assayed by spectrophotometry (Bao, 2000). Soil potassium permanganate-oxidizable C (KMnO4-C) was determined according to Blair et al. (1995). Briefly, soil samples containing approximately 15 mg of carbon were weighed in centrifuge tubes, then 25 ml of 333 mM KMnO4 solution was added; they were shaken for 1 hour, and centrifugation was applied for 5 min. The samples were then diluted with deionized water. Finally, the absorbance of the supernatants was assayed using a spectrophotometer at 565 nm. The changes indicated that the equivalent of 1.0 mmol L−1 of MnO4− was needed to consume 0.75 mmol L−1 (9.0 mg) of oxidized carbon. All soil physical and chemical properties are shown in Supplementary Table S2.
The potential hydrolytic and oxidative enzyme activities were assayed according to modified methods (Saiya-Cork K R, 2002; DeForest, 2009). The activities of four hydrolase enzymes, β-1,4-glucosidase (BG), cellobiohydrolase (CBH), L-leucine aminopeptidase (LAP) and β-1,4-N-acetylglucosaminidase (NAG), were determined by microplate fluorescence. The activities of peroxidase (PERX) and phenol oxidase (PPO) were analysed with a microplate spectrophotometer. The specific determination methods were as follows: all soil enzyme activities were measured in a 96-well plate. Buffers, samples, reference standards, and enzyme substrates were added according to a strict sequence and position on the 96-well plate. The standard substance of BG, CBH, and NAG was 4-methylumbelliferone (4-MUB), and the standard substance of LAP was 7-amino-4-methylcoumarin. The substrates were 4-MUB-β-D-glucopyranoside, 4-MUB-β-D-cellobioside, 4-MUB-N-acetyl-β-D-glucosaminide, and L-leucine-7-amino-4-methylcoumarin. The substrate of oxidase was L-3,4-dihydroxyphenylalanine (L-DOPA). 1 g fresh soil was dissolved in 50 ml of buffer solution, and a magnetic mixer was used to evenly rotate the soil slurry for 1 min. The sample well contained 150 µL of soil suspension and 50 µL of 10umol L−1 substrate solution, and the blank well contained 150 µL of soil suspension and 50 µL of buffer. The negative control contained 150 µL of buffer and 50 µL of enzyme substrate solution, quench wells contained 50 µL of reference standard and 150 µL of suspension, and the reference standard wells contained 50 µL of reference substance and 150 µL of buffer. The incubation times were 2 h, 4 h, 4 h, and 2 h, respectively. The reaction was stopped with 10 µL of 1 mol L−1 NaOH. Fluorescence was measured using a microplate fluorometer (Spectra Max M2, Molecular Device, California, US) with 365 nm excitation and 450 nm emission filters. Oxidative activity was quantified by measuring absorbance at 450 nm using a microplate spectrophotometer. All enzyme activities were expressed as nmol h−1 g−1.
2.5 Calculation
According to García-Palacios et al. (2016), Liao et al. (2022) and Yue et al. (2018), litter C and N losses rather than litter quality loss rate or litter decay rate constant (k) were applied because the factors regulating the temporal dynamics of litter decay can differ for C or N losses, and litter decomposition represent the largest carbon flux and act as primary controls on N cycling (Parton et al., 2007). The litter C or N losses (i.e., the percentage of initial dry mass) during decomposition were calculated as follows:
where Ls represents the litter C or N loss (%), M0 is the initial leaf litter dry mass (3 g) or root litter dry mass (2 g), C0 is the initial leaf or root litter C or N initial content (% of litter dry mass), Mt is the leaf litter or root litter dry mass at the six different sampling times, and Ct is the leaf or root litter C or N content (% of litter dry mass) at the six different sampling times.
2.6 Statistical analyses
All analyses were conducted with SPSS 20.0 (SPSS Inc. Chicago, IL, United States). The Shapiro-Wilk test was used to conduct normal distribution test. One-way ANOVA was used to compare the leaf and root litter initial traits and soil physical and chemical properties under different stand ages. When ANOVA results were significant at p < 0.05, differences among the different stand ages were tested using Duncan tests. Two-way ANOVA was used to test the effect of stand ages, litter types and their interaction on initial litter quality. In addition, the influence of stand age, litter type and time on litter C and N losses, soil properties and enzyme activities during the decomposition process were tested with multiway ANOVA. All graphs were drawn with Origin 2017 (Origin Lab Corporation).
We used partial least squares path modelling (PLSPM) to evaluate whether the relative importance of litter quality, the soil environment, and soil enzyme activities on litter C and N losses differed under different decomposition stages. We proposed a path graph (Figure 2) to represent an a priori model of the hypothesized relationships, and then we used the diagram to perform comparisons across the consecutive decomposition stages. We compared leaves and roots separately since the relative importance of regulating factors may differ between litter types (García-Palacios et al., 2016). Following Parsons et al. (2014) and García-Palacios et al. (2016), a smoothing approach was chosen to allow for the determination of consecutive stages along the litter decomposition. Smoothing was acquired by rounding the mass loss value of each litterbag to the next 10%; 40% mass loss smoothing intervals (i.e., 0%–40% and 10%–50%, 20%–60% and 30%–70% quality loss smoothing intervals) were then chosen for the four groups. The 40% smoothing interval was chosen because it contained enough sample sizes for us to conduct multigroup comparison analysis for each decomposition stage. We then tested the models of carbon and nitrogen losses in litters. The relative importance of regulatory factors to decomposition stages may vary between C or N elements (Liao et al., 2022).
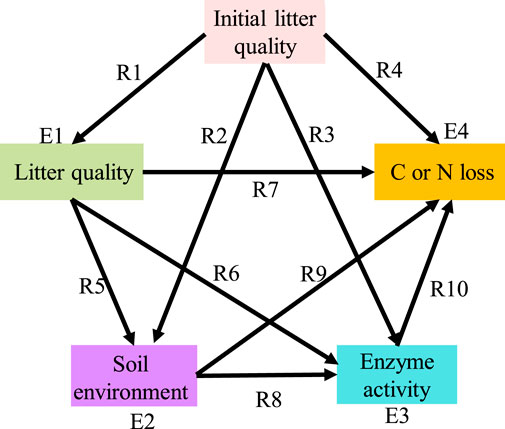
FIGURE 2. The priori model of the hypothesized relationships among initial litter quality, litter quality, the soil environment and soil enzyme activities for litter C or N loss. All measured variables (except for “initial litter quality”) represent temporal dynamics during decomposition. The model was applied to conduct multigroup comparisons among different decomposition stages, and the smoothing groups were selected using 40% mass intervals. The single-headed arrows represent a hypothesized relationship of one variable on another. R is the path coefficient, and E is the error variance of a variable.
We employed the same a priori model for both leaf and root litter decomposition in our study. In the predetermined model, “initial litter quality” was the major driving factor for decomposition, and enzyme activity was the final factor. The “initial litter quality” included total C, total N, total P, cellulose, hemicellulose, lignin, C:N, C:P, and lignin:N. The “litter quality” represented the temporal dynamics of litter C, N, and P contents and stoichiometry (C:N, C:P, and N:P) during decomposition. “Soil environment” represented the temporal measurements of various soil properties (see Table 3), and “enzyme activities” represented the dynamic changes in hydrolytic and oxidative enzyme activities during decomposition (see Table 3). We first used correlation analysis to screen the indexes that were significantly related to litter C and N losses. For leaf litter, the initial litter quality included the total N, cellulose, lignin, C:N and lignin:N. Litter quality included C, N, P, C:N, C:P, and N:P. The soil environment included SOC, STN, NO3−-N, NH4+-N, DON, and pH. Enzyme activities included BG, NAG, LAP, PERX, and PO. For root litter, the initial litter quality included the total C, total N and C:N. Litter quality included C and C:N. The soil environment included STP, NO3−-N, NH4+-N, DOC and KMnO4-C. Enzyme activities included CBH, LAP, and PERX. Then, the selected indexes were used to conduct PLSPM analysis. The goodness-of-fit of each model is listed in Supplementary Table S3; the related statistical analyses were carried out with SPSS 20.0 and the R package “PLSPM”.
3 Results
3.1 Variations in leaf and root litter C and N losses during decomposition
The temporal dynamics of litter C and N losses were influenced by litter type and restoration duration (Figure 3; Table 2). Generally, leaf litter C loss (70.02%) was significantly higher than that of root litter (46.74%) (Figures 3A,B). Similarly, leaf litter N loss (70.72%) was faster than that of root litter (32.39%) (Figures 3C,D). Restoration duration had a significant effect on leaf and root litter C and N losses (Table 2). The leaf litter C and N losses increased with the restoration duration, with higher C and N losses in the older stands (45Y) (Figures 3A,C). However, the root litter C loss at 45 Y (37.74%) was lower than those at 35 Y (47.53%), 25 Y (49.59%), and 15 Y (52.09%) (Figure 3B), and the root litter N loss at 45 Y was also lower than those at the other three restoration durations (Figure 3D).
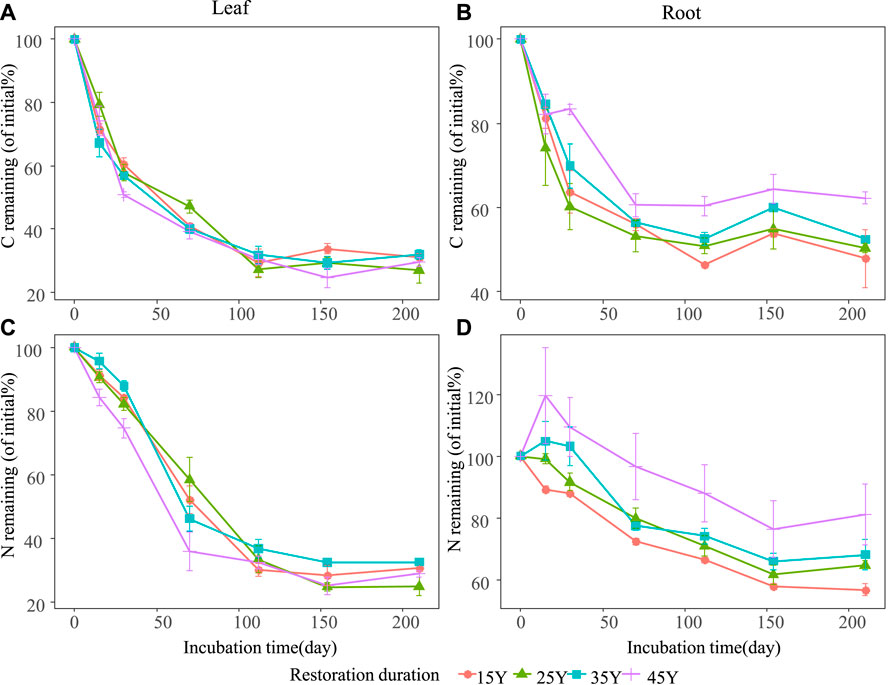
FIGURE 3. Temporal dynamics of litter and root C (A,B) and N (C,D) remaining in four different restoration durations of Robinia pseudoacacia stands. Values are means ± standard error.
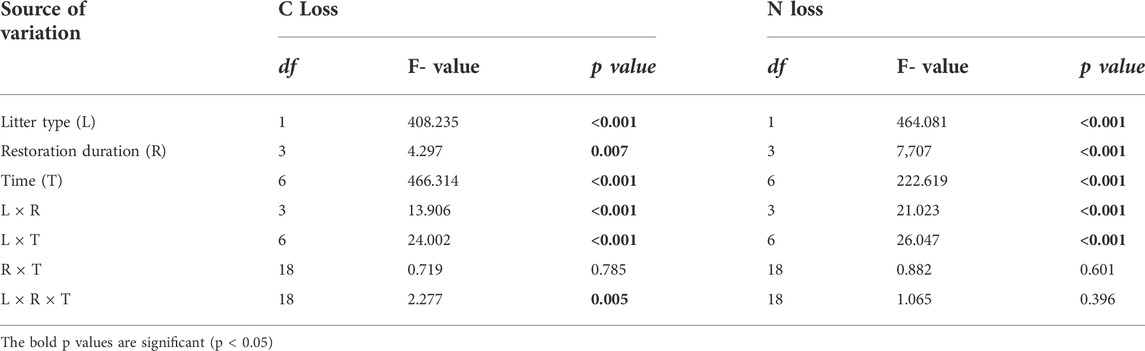
TABLE 2. Significance of the effects of litter type (L), restoration duration (R), decomposition time (T) and their interactions on litter C and N losses based on multiway ANOVA.
3.2 Variations in the soil environment and enzyme activities under decomposition
The soil environment and enzyme activities were significantly influenced by restoration duration, decomposition time and their interactions (Table 3). Litter type had a significant effect on nitrogen-related soil properties (such as TN, NO3−-N, NH4+-N, DON) (Figures 4A–H) and pH (Figure 4K–L), whereas there was no significant effect on soil enzyme activities (Figure 5A; Table 3). For both leaf and root litter, the SOC, STN, STP, NO3−-N, NH4+-N, and DON contents increased during the late stages of decomposition, while enzyme activities fluctuated during the decomposition processes (Figure 5A–L).
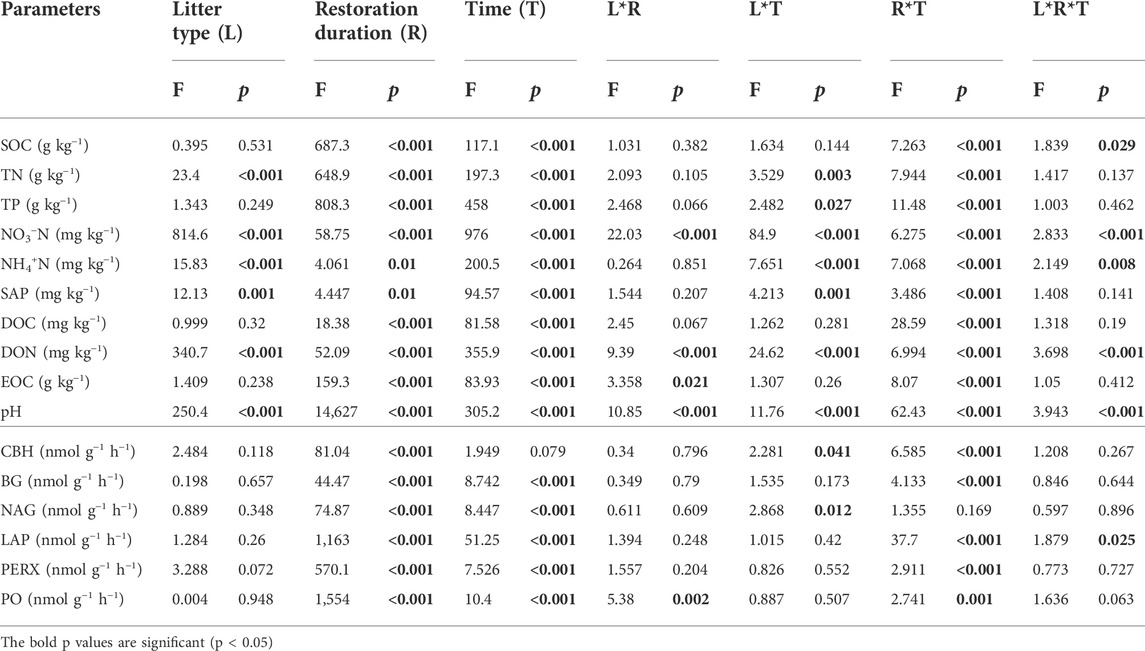
TABLE 3. Significance of the effects of litter type (L), restoration duration (R), decomposition time (T) and their interactions on soil properties and enzyme activities based on multiway ANOVA.
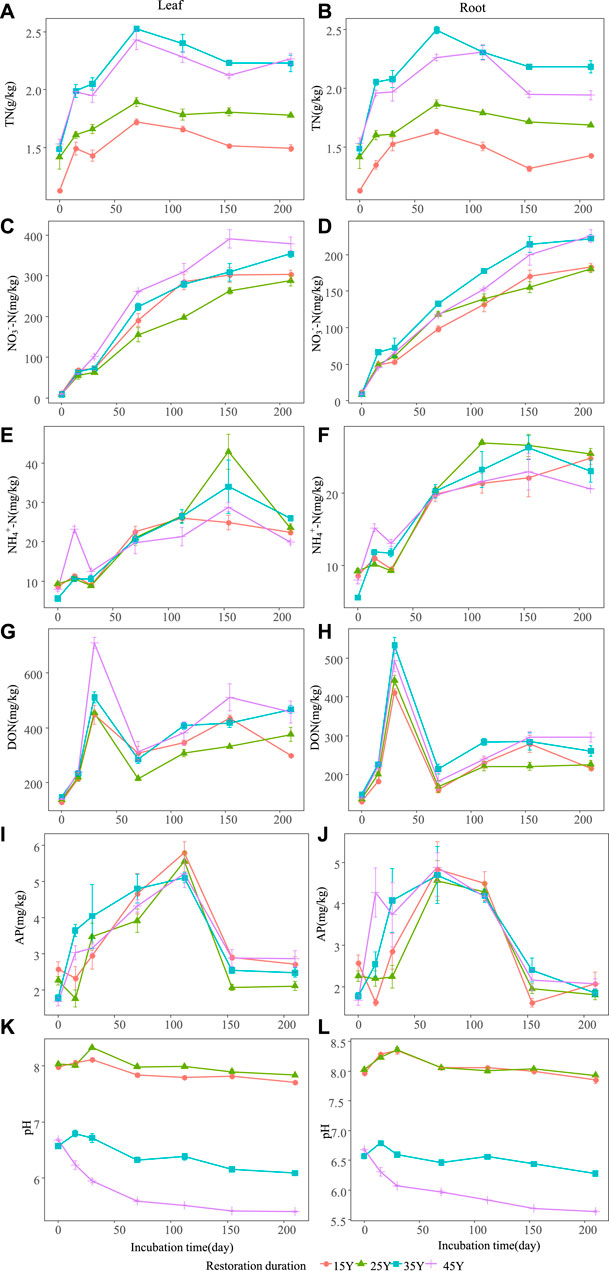
FIGURE 4. Temporal dynamics of soil properties after the leaf and root litter addition of four different restoration durations of Robinia pseudoacacia stands. Values are means ± standard error. The dynamic changes of soil total nitrogen when leaf litter and root addition (A–B); the dynamic changes of soil nitrate nitrogen when leaf litter and root addition (C–D); the dynamic changes of soil ammonia nitrogen when leaf litter and root addition (E–F); the dynamic changes of soil dissolved organic nitrogen when leaf litter and root addition (G–H); the dynamic changes of soil available phosphorus when leaf litter and root addition (I–J); the dynamic changes of soil pH when leaf litter and root addition (K–L).
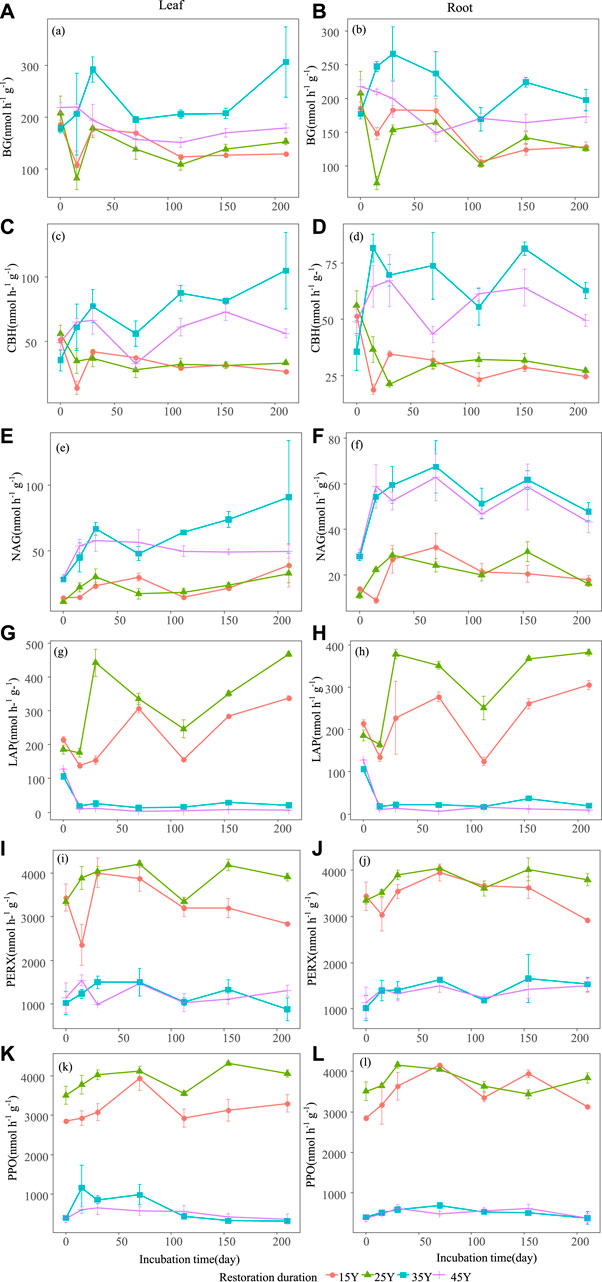
FIGURE 5. Temporal dynamics of soil enzyme activities after the leaf and root litter addition in four different restoration durations of Robinia pseudoacacia stands. Values are means ± standard error. The dynamic changes of soil β-1,4-glucosidase (BG) activity when leaf litter and root addition (A–B); the dynamic changes of soil cellobiohydrolase (CBH) activity when leaf litter and root addition (C–D); the dynamic changes of β-1,4-N-acetylglucosaminidase (NAG) activity when leaf litter and root addition (E–F); the dynamic changes of soil L-leucine aminopeptidase (LAP) activity when leaf litter and root addition (G–H); the dynamic changes of soilperoxidase (PERX) activity when leaf litter and root addition (I–J); the dynamic changes of soil phenol oxidase (PPO) activity when leaf litter and root addition (K–L).
3.3 Effect of litter quality, the soil environment, and enzyme activities on litter C and N losses under decomposition
The path diagram shows the direct and indirect effects of initial litter quality, litter quality, the soil environment and enzyme activities on litter C and N losses (Figure 2). The PLSPM shows that the effects of all regulating factors on litter C and N losses differed in various decomposition stages (Figures 6, 7).
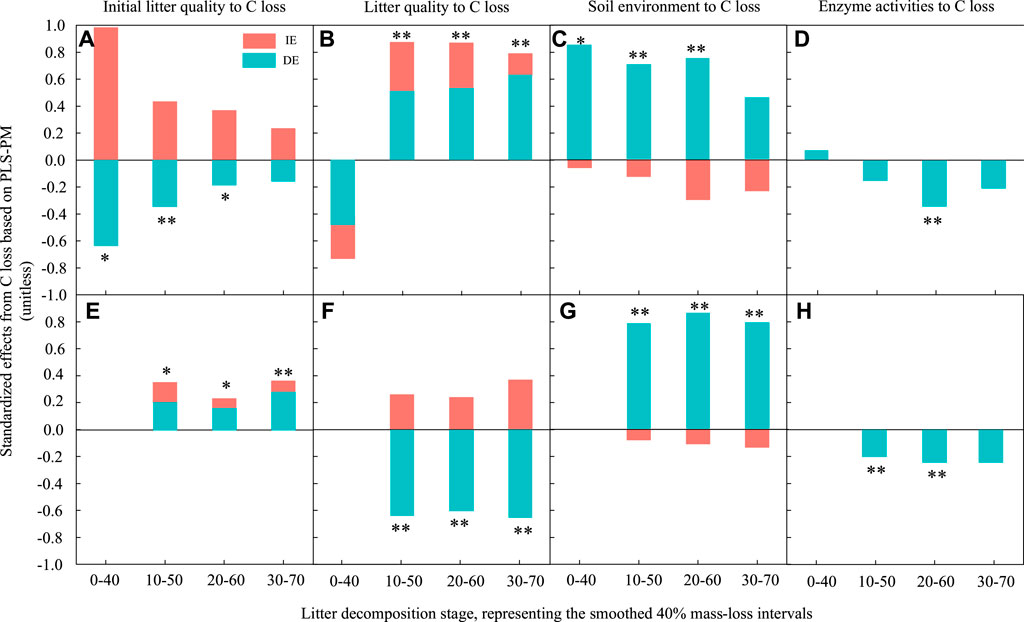
FIGURE 6. The standardized direct and indirect effects derived from partial least squares path modelling (PLSPM) to assess the effects of initial litter quality, litter quality, the soil environment and enzyme activities on leaf and root litter C loss during decomposition. (A) and (E) represent the effect of initial litter quality on C loss for leaf and root litter, respectively. (B) and (F) represent the effect of litter quality on C loss for leaf and root litters, respectively. (C) and (G) represent the effect of the soil environment on C loss for leaf and root litters, respectively. (D) and (H) represent the effect of enzyme activities on C loss for leaf and root litters, respectively. DE, standardized direct effect; IE, standardized indirect effect. **p < 0.01, *p < 0.05.
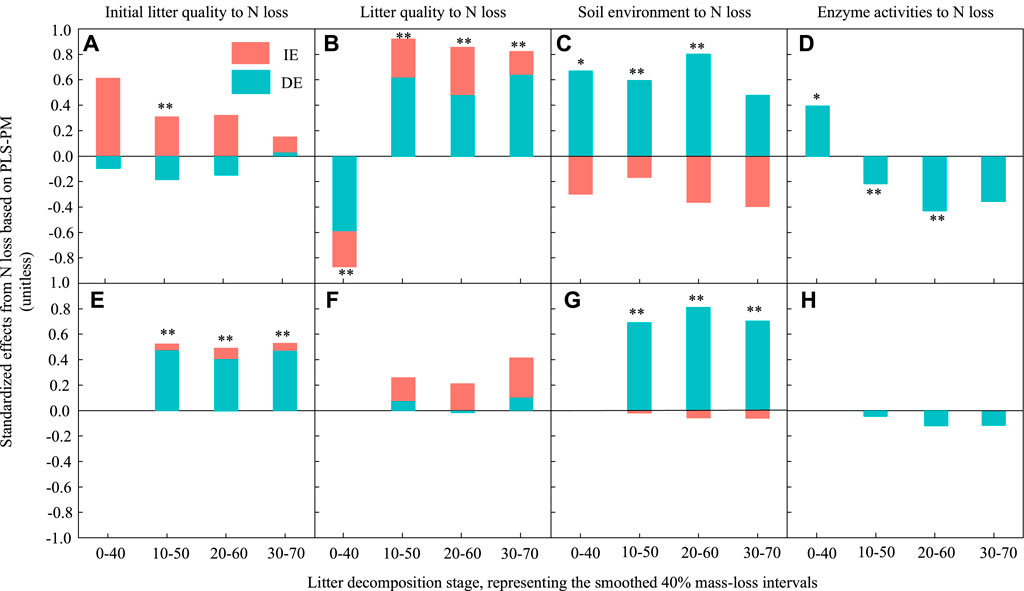
FIGURE 7. Standardized direct and indirect effects derived from partial least squares path modelling (PLSPM) to assess the effects of initial litter quality, litter quality, the soil environment and enzyme activities on leaf and root litter N losses during decomposition. (A) and (E) represent the effect of initial litter quality on N loss for leaf and root litters, respectively. (B) and (F) represent the effect of litter quality on N loss for leaf and root litters, respectively. (C) and (G) represent the effect of the soil environment on N loss for leaf and root litters, respectively. (D) and (H) represent the effect of enzyme activities on N loss for leaf and root litters, respectively. DE, standardized direct effect; IE, standardized indirect effect. **p < 0.01, *p < 0.05.
For C loss, during the early and middle stages, the initial leaf litter quality had a significantly negative direct effect and a positive indirect effect. There was no significant effect at the late stage (Figure 6A). However, it had a significant positive effect on root C loss (Figure 6E). The effect of initial litter quality on root N loss was similar to that on litter C loss in the 10%–50% decomposition stage (Figure 7A), and it had a significant positive effect on root N loss in all stages (Figure 7E).
Litter quality had a significant positive effect on leaf litter C and N losses during the middle and late decomposition processes (Figures 6B, Figure 7B). It had a significantly negative direct effect and positive indirect effect on root C loss (Figure 6F), but there was no significant effect on root N loss.
The soil environment had a significant positive direct effect on leaf litter C and N losses during the early and middle stages; however, there was no significant effect in the late decomposition process (Figures 6C, Figure 7C). Similarly, the soil environment had a significant positive effect on root C and N losses in all decay stages.
Enzyme activities had a weaker influence on litter decomposition. They had a significant negative direct effect on leaf litter C loss in the middle stages; their effect on leaf litter N loss changed from a positive direct effect in the early stage to a negative direct effect in the middle and late stages. However, the effect of enzyme activities on root C loss was negative and direct during the early and middle decomposition stages, but there was no significant effect on root N loss.
4 Discussion
Our results provide a comparative assessment of the temporal dynamics of leaf and root litter decomposition and quantify the relationships among litter quality, the soil environment and enzyme activities, which support a comprehensive understanding of the effect of litter decomposition of Robinia pseudoacacia on carbon and nitrogen cycling on the Loess Plateau of China (Figure 8). We found that the C or N losses in leaf litter were significantly higher than those in root litter regardless of stand age (Figure 3; Table 2). The carbon and nitrogen losses in leaf litter increased over the course of the restoration duration, whereas there was an opposite trend in root litter carbon and nitrogen losses, which had the lowest losses in the older stand ages (45 Y). The carbon and nitrogen loss patterns were driven by litter quality, the soil environment and enzyme activities (Figures 6, 7). More importantly, our results demonstrated that the effects of litter quality and the soil environment on litter decomposition were stronger than those of enzyme activities, and the effects differed with decomposition stage.
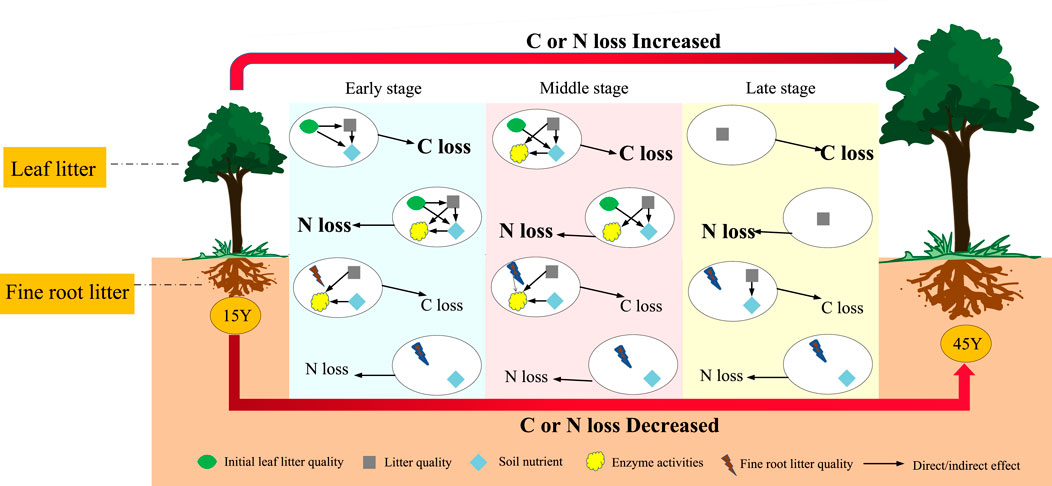
FIGURE 8. Schematic diagram of carbon and nitrogen losses of Robinia pseudoacacia leaf and fine root litter in different restoration durations. 15 Y to 45 Y represent stand ages from 15 years to 45 years. The early stage, middle stage and late stage represent three different decomposition stages. All factors in the ovals (initial litter quality, litter quality, the soil environment and enzyme activities) changed with decomposition stage, which showed the difference in relative importance. The C and N losses in leaf litter are in bold, which indicated that the C or N losses in leaf litter were significantly higher than those in root litter.
4.1 Increased leaf litter carbon and nitrogen losses compared with root litter
In agreement with our first hypothesis, the C and N losses in leaf litter were higher than those in root litter. First, there were higher initial N and P contents and lower C:N ratios in the leaf litter than in the root litter (Supplementary Tables S1,S3). The chemical traits of N, P and lignin content had different effects on leaf and root decomposition, and litters with higher nitrogen and phosphorus contents and lower lignin content generally decomposed faster (Canessa et al., 2020; Cornwell et al., 2008; Guo et al., 2021; See et al., 2019). The C:N ratio represented the ratio of carbohydrates to protein (Zhang et al., 2018). High-quality litters (low C:N) were easily degraded by decomposers; they may have lacked the complex structures needed to endure adverse environments. The complex structure compound means some recalcitrant substrates, such as lignin and cellulose. The presence of this structural defensive compound, which confers toughness on leaf litter, protects the litter from microbial degradation and constitutes waterproofing properties of plant cell walls, slowing down physical abrasion (Zhang et al., 2019). Thus, they were occupied by fungi and microbes and had a faster decomposition rate (García-Palacios et al., 2013; Li et al., 2020). Low-quality litters (high C:N) tended to degrade slowly because they had more recalcitrant substances, and the microbial community needed to mine N from external sources (such as SOM) to degrade. Therefore, high-quality litters tended to degrade more rapidly than low-quality litters (Prieto et al., 2019; Shen et al., 2021). Second, higher leaf litter C and N losses compared with those of root litter may be related to the soil environment. There was a higher mineral N and DON content after litter addition (Figure 4), and litter type had a significant effect on nitrogen-related soil nutrients (Table 3). Litter addition may enhance microbial growth and activities, promote the microbial nutrient utilization rate, and accelerate litter decomposition processes and carbon and nitrogen dynamics (Martínez-García et al., 2021).
Carbon and nitrogen losses from leaf litter increased during vegetation restoration, while those from root litter showed the opposite trend; the older stand ages (45 Y) had the lowest carbon and nitrogen losses, which agrees with our second hypothesis. A previous study demonstrated that the rate of decomposition of R. pseudoacacia plantation litter increased during the revegetation process (Zhang et al., 2021), similar to our results. Litter N and P contents increased with restoration duration (Supplementary Table S1), which may have been beneficial for rapidly growing microbes and accelerating carbon and nitrogen losses (Xiao et al., 2019), as the effect of restoration duration on litter decomposition was more pronounced during the early stage. There have been relatively few studies on the fine-root decomposition of different stand ages; possible explanations for the difference in fine-root decomposition are as follows. Compared with other young stand ages, the older stand age (45 Y) experienced decreases in soil pH after litter addition; thus, soil acidification inhibited litter decomposition (Shen et al., 2021). In addition, a less complex but more specialized microbial network resulted in faster fine-root decomposition in young stands of R. pseudoacacia (data not shown). Different types of litter decomposition showed a contrasting trend with increasing restoration duration, which reflected inconsistencies in leaf and root litter carbon and nitrogen losses over the course of vegetation restoration. Further validation is needed in combination with field experiments.
4.2 Relative importance of influencing factor on litter C and N losses
The relative importance of initial litter quality differs between leaf and root litters during different decomposition stages, which suggests that leaf and root litter decomposition is decoupled with decomposition duration (Ma et al., 2016). In our studies, the initial litter quality had a significant influence on leaf litter carbon and nitrogen losses at the early stages, while it also had a significant impact on root litter carbon and nitrogen losses at the late stages. Previous studies have demonstrated that litter quality is primarily important in the early stage of decomposition (Canessa et al., 2020; Zheng et al., 2020). Litter nitrogen rapid mineralization may have been conducive to lignin degradation during early decomposition, and lignin degradation may have led to carbohydrate decay, supplying a carbon source and energy to microbial decomposers (He et al., 2019). While the mineralization of root litter was lower than that of leaf litter (Figures 3B,D), the legacy effect of initial litter quality may have played a crucial role in root litter decomposition in the late stages (García-Palacios et al., 2016; Yue et al., 2018). The difference in leaf and root litters in the late decomposition stages may have resulted in the slow decomposition of root litter. Initial litter quality represented the variation in litter chemical and morphological composition and was the most important determinant of litter decomposition (Wang et al., 2021a; Wang et al., 2021b; Fu et al., 2021). Moreover, initial litter quality also indirectly regulated litter carbon and nitrogen losses by influencing litter quality (nutrient content and stoichiometry) (Wang et al., 2021b; Liao et al., 2022).
The variation in litter quality with decomposition duration is an important part of litter decomposition. Previous studies have shown that litter quality and stoichiometry directly and indirectly regulate soluble carbon and phosphorus release (Wang et al., 2021a). Similarly, our results demonstrated that the chemical component changes in leaf litter directly and indirectly regulated litter carbon and nitrogen losses, with mainly a direct impact (Figures 6, 7). Litter nutrient contents and C:N:P stoichiometry reflected metabolic limitation for decomposers. During different stages of litter decomposition, differences in litter quality and microbial available carbon influenced microbial community structure and activities (Zheng et al., 2018). Litter quality significantly influenced fine-root carbon loss throughout the decomposition process, while it had no significant effect on fine-root N loss, suggesting that root litter carbon loss was more sensitive to litter quality changes. Taken together, these results show that litter quality had a positive influence on leaf litter carbon and nitrogen losses but a negative influence on fine-root N loss. These different patterns for litter carbon and nitrogen losses indicated that there was a high uncertainty for leaf and root litter decomposition (Sun et al., 2018). The relative importance of litter quality on litter carbon and nitrogen losses depended on whether basic energy could satisfy the demand of microbes (Hättenschwiler and Jørgensen, 2010).
The soil environment is an important factor that affects the litter decomposition rate. Soil not only supplies necessary nutrients for decomposers to maintain life activities but also alters the microclimate or nutrient conditions for litter decomposition (Ge et al., 2013). Therefore, changes in the soil environment significantly influence leaf litter and fine-root carbon and nitrogen losses. This influence mainly had a significant positive direct effect regardless of decomposition stage and litter type (Figures 6, 7). Previous studies have indicated that soil nitrogen availability can influence litter nitrogen release (Ge et al., 2013; Martínez-García et al., 2021). The main soil chemical properties, such as pH and nutrient content, are limiting factors for litter decomposition (Zhang et al., 2018). Soil pH declined during the decomposition process, which had a negative effect on microbial process that are difficult to breakdown (Li et al., 2022). es (Tanikawa et al., 2018; Shen et al., 2021), and thus litter carbon and nitrogen losses decreased during the late period. There are two mechanisms underlying nutrient availability in the decomposition process: microbial and chemical mechanisms (Jiang et al., 2018). Litter carbon and nitrogen are rapidly released during the early stages, and thus, the nutrient supply ratio matches the microbial requirement, so litter has a high decomposition rate (stoichiometric theory). Recalcitrant carbon gradually plays an important role in decomposition after the rapid release of labile carbon. Nutrient availability enhancement (NO3−-N, NH4+-N, DON) may ease microbial demand for nutrients based on “N mining theory”, and litter carbon and nitrogen losses gradually decrease (Fanin et al., 2020). The chemical mechanism is related to initial litter chemical properties, as acid unhydrolyzed residue in litter can absorb inorganic nitrogen ions to create new compounds.
Enzyme activities significantly varied during the decomposition of leaf litter and fine roots (Table 3; Figure 5). Previous studies have demonstrated that enzyme activities mediate the litter decomposition process (Fu et al., 2021; Li et al., 2021; Zhang et al., 2021; Li et al., 2022). Enzyme activities often have a positive effect on litter decomposition at the local scale (Zhong et al., 2017; Zheng et al., 2021). However, our analyses showed that enzyme activities had a negative effect on litter carbon and nitrogen losses during early decomposition, which is inconsistent with our third hypothesis. Litter carbon and nitrogen have a rapid release at the early stage of litter decomposition (Figure 3), which may satisfy the nutrient demand of microorganism and then inhibit the mining of corresponding nutrients by extracellular enzymes (Kourtev et al., 2002). Though enzyme exists in soil, the efficiency was largely decreased (Allison and Vitousek, 2004). Overall, the production of soil enzymes involves a trade-off to maximize carbon, nitrogen and phosphorus production in response to nutrient resources and substrate changes (Bai et al., 2021). As reported by Song et al. (2021), the effects of C, N and P-related enzyme activities on litter carbon and nitrogen losses were weaker than those on litter quality. Indeed, our study demonstrated that the effects of enzyme activities on litter carbon and nitrogen losses were weaker than those of other driving factors. We speculated that the enzyme may be a product secreted by microorganisms, so the relative importance of biotic factors on carbon and nitrogen losses in litters should be further evaluated in combination with specific microorganism groups.
5 Conclusion
Our results demonstrate that the relative importance of litter quality, the soil environment and enzyme activities on litter C and N losses changes with the litter decomposition process. Initial litter quality, litter quality and the soil environment determined leaf and root litter C loss, while enzyme activities has a minor effect on litter C loss. Litter quality, the soil environment and enzyme activities directly influenced leaf litter N loss, while initial litter quality and the soil environment affected fine-root N loss. The effect of enzyme activities on litter C and N loss was weaker than substrate and soil environment. These results indicated that leaf and fine root litter decomposition decoupled during the decomposition process. Litter type and decomposition stages should be comprehensively considered when assessing litter decomposition to better understand the relationships between litter decomposition and carbon and nitrogen cycling in ecosystems.
Data availability statement
The original contributions presented in the study are included in the article/Supplementary Material, further inquiries can be directed to the corresponding author.
Author contributions
ZS contributed to conception and design of the study. XZ conducted experiment analysis. YW and SM performed the statistical analysis. ZS wrote the first draft of the manuscript. ZS reviewed and edited the manuscript. All authors contributed to manuscript revision, read, and approved the submitted version.
Funding
This work was supported by the Science and Technology Innovation Program of the Shaanxi Academy of Forestry (SXLK2022-02-03), and the National Natural Science Foundation of China (42077452).
Conflict of interest
The authors declare that the research was conducted in the absence of any commercial or financial relationships that could be construed as a potential conflict of interest.
Publisher’s note
All claims expressed in this article are solely those of the authors and do not necessarily represent those of their affiliated organizations, or those of the publisher, the editors and the reviewers. Any product that may be evaluated in this article, or claim that may be made by its manufacturer, is not guaranteed or endorsed by the publisher.
Supplementary material
The Supplementary Material for this article can be found online at: https://www.frontiersin.org/articles/10.3389/fenvs.2022.956309/full#supplementary-material
References
Allison, S. D., and Vitousek, P. M. (2004). Extracellular enzyme activities and carbon chemistry as drivers of tropical plant litter decomposition. Biotropica 36 (3), 285–296. doi:10.1111/j.1744-7429.2004.tb00321.x
Bai, X., Dippold, M. A., An, S., Wang, B., Zhang, H., and Loeppmann, S. (2021). Extracellular enzyme activity and stoichiometry: The effect of soil microbial element limitation during leaf litter decomposition. Ecol. Indic. 121, 107200. doi:10.1016/j.ecolind.2020.107200
Bradford, M. A., Berg, B., Maynard, D. S., Wieder, W. R., Wood, S. A., and Cornwell, W. (2016). Understanding the dominant controls on litter decomposition. J. Ecol. 104 (1), 229–238. doi:10.1111/1365-2745.12507
Canessa, R., Brink, L., Saldaña, A., Rios, R. S., Hättenschwiler, S., Mueller, C. W., et al. (2020). Relative effects of climate and litter traits on decomposition change with time, climate and trait variability. J. Ecol. 109 (1), 447–458. doi:10.1111/1365-2745.13516
Chen, Y., Liu, Y., Zhang, J., Yang, W., He, R., and Deng, C. (2018). Microclimate exerts greater control over litter decomposition and enzyme activity than litter quality in an alpine forest-tundra ecotone. Sci. Rep. 8 (1), 14998–15013. doi:10.1038/s41598-018-33186-4
Cornwell, W. K., Cornelissen, J. H. C., Amatangelo, K., Dorrepaal, E., Eviner, V. T., Godoy, O., et al. (2008). Plant species traits are the predominant control on litter decomposition rates within biomes worldwide. Ecol. Lett. 11 (10), 1065–1071. doi:10.1111/j.1461-0248.2008.01219.x
Couˆteaux, M.-M., Bottner, P., and Berg, B. (1995). Litter decomposition, climate and liter quality. Trends Ecol. Evol. 10 (2), 63–66. doi:10.1016/s0169-5347(00)88978-8
DeForest, J. L. (2009). The influence of time, storage temperature, and substrate age on potential soil enzyme activity in acidic forest soils using MUB-linked substrates and L-DOPA. Soil Biol. Biochem. 41 (6), 1180–1186. doi:10.1016/j.soilbio.2009.02.029
Eastman, B. A., Adams, M. B., and Peterjohn, W. T. (2022). The path less taken: Long-term N additions slow leaf litter decomposition and favor the physical transfer pathway of soil organic matter formation. Soil Biol. Biochem. 166, 108567. doi:10.1016/j.soilbio.2022.108567
Fanin, N., Alavoine, G., and Bertrand, I. (2020). Temporal dynamics of litter quality, soil properties and microbial strategies as main drivers of the priming effect. Geoderma 377, 114576. doi:10.1016/j.geoderma.2020.114576
Freschet, G. T., Cornwell, W. K., Wardle, D. A., Elumeeva, T. G., Liu, W., Jackson, B. G., et al. (2013). Linking litter decomposition of above‐and below‐ground organs to plant–soil feedbacks worldwide. J. Ecol. 101 (4), 943–952. doi:10.1111/1365-2745.12092
Fu, B., Wang, S., Liu, Y., Liu, J., Liang, W., and Miao, C. (2017). Hydrogeomorphic ecosystem responses to natural and anthropogenic changes in the Loess Plateau of China. Annu. Rev. Earth Planet. Sci. 45, 223–243. doi:10.1146/annurev-earth-063016-020552
Fu, Y., Feng, F., Zhang, X., and Qi, D. (2021). Changes in fine root decomposition of primary Pinus koraiensis forest after clear cutting and restoration succession into secondary broad-leaved forest. Appl. Soil Ecol. 158, 103785. doi:10.1016/j.apsoil.2020.103785
García-Palacios, P., Maestre, F. T., Kattge, J., Wall, D. H., and Klironomos, J. (2013). Climate and litter quality differently modulate the effects of soil fauna on litter decomposition across biomes. Ecol. Lett. 16 (8), 1045–1053. doi:10.1111/ele.12137
García-Palacios, P., Shaw, E. A., Wall, D. H., Hättenschwiler, S., and Knops, J. M. H. (2016). Temporal dynamics of biotic and abiotic drivers of litter decomposition. Ecol. Lett. 19 (5), 554–563. doi:10.1111/ele.12590
Ge, X., Zeng, L., Xiao, W., Huang, Z., Geng, X., and Tan, B. (2013). Effect of litter substrate quality and soil nutrients on forest litter decomposition: A review. Acta Ecol. Sin. 33 (2), 102–108. doi:10.1016/j.chnaes.2013.01.006
Gunina, A., Vormstein, S., Kaiser, M., and Ludwig, B. (2019). Decomposition of beech leaves and roots in the topsoil and subsoil of a sandy Cambisol: Results of a one-year incubation. Eur. J. Soil Biol. 95, 103136. doi:10.1016/j.ejsobi.2019.103136
Guo, L., Deng, M., Yang, S., Liu, W., Wang, X., Wang, J., et al. (2021). The coordination between leaf and fine root litter decomposition and the difference in their controlling factors. Glob. Ecol. Biogeogr. 30 (11), 2286–2296. doi:10.1111/geb.13384
Hättenschwiler, S., and Jørgensen, H. B. (2010). Carbon quality rather than stoichiometry controls litter decomposition in a tropical rain forest. J. Ecol. 98 (4), 754–763. doi:10.1111/j.1365-2745.2010.01671.x
He, M., Zhao, R., Tian, Q., Huang, L., Wang, X., and Liu, F. (2019). Predominant effects of litter chemistry on lignin degradation in the early stage of leaf litter decomposition. Plant Soil 442 (1), 453–469. doi:10.1007/s11104-019-04207-6
Jiang, L., Kou, L., and Li, S. (2018). Alterations of early-stage decomposition of leaves and absorptive roots by deposition of nitrogen and phosphorus have contrasting mechanisms. Soil Biol. Biochem. 127, 213–222. doi:10.1016/j.soilbio.2018.09.037
Jiang, L., Kou, L., and Li, S. (2019). Decomposition of leaf mixtures and absorptive-root mixtures synchronously changes with deposition of nitrogen and phosphorus. Soil Biol. Biochem. 138, 107602. doi:10.1016/j.soilbio.2019.107602
Joshi, R. K., and Garkoti, S. C. (2021). Influence of Nepalese alder on soil physico-chemical properties and fine root dynamics in white oak forests in the central Himalaya, India. Catena 200, 105140. doi:10.1016/j.catena.2020.105140
Kourtev, P., Ehrenfeld, J., and Huang, W. (2002). Enzyme activities during litter decomposition of two exotic and two native plant species in hardwood forests of New Jersey. Soil Biol. Biochem. 34 (9), 1207–1218. doi:10.1016/s0038-0717(02)00057-3
Krishna, M., and Mohan, M. (2017). Litter decomposition in forest ecosystems: A review. Energy Ecol. Environ. 2 (4), 236–249. doi:10.1007/s40974-017-0064-9
Li, Q., Zhang, M., Geng, Q., Jin, C., Zhu, J., Ruan, H., et al. (2020). The roles of initial litter traits in regulating litter decomposition: A “common plot” experiment in a subtropical evergreen broadleaf forest. Plant Soil 452 (1-2), 207–216. doi:10.1007/s11104-020-04563-8
Li, R., Yang, Q., Guan, X., Chen, L., Wang, Q., Wang, S., et al. (2022a). High quality litters with faster initial decomposition produce more stable residue remaining in a subtropical forest ecosystem. Catena 213, 106134. doi:10.1016/j.catena.2022.106134
Li, Y., Gong, J., Zhang, Z., Shi, J., Zhang, W., and Song, L. (2022b). Grazing directly or indirectly affect shoot and root litter decomposition in different decomposition stage by changing soil properties. Catena 209, 105803. doi:10.1016/j.catena.2021.105803
Li, Z., Wang, F., Su, F., Wang, P., Li, S., Bai, T., et al. (2021). Climate change drivers alter root controls over litter decomposition in a semi-arid grassland. Soil Biol. Biochem. 158, 108278. doi:10.1016/j.soilbio.2021.108278
Liao, C., Long, C., Zhang, Q., and Cheng, X. (2022). Stronger effect of litter quality than micro‐organisms on leaf and root litter C and N loss at different decomposition stages following a subtropical land use change. Funct. Ecol. 36 (4), 896–907. doi:10.1111/1365-2435.13999
Lin, C., Lin, W., Chen, S., Peng, J., Guo, J., and Yang, Y. (2019). Phosphorus addition accelerates fine root decomposition by stimulating extracellular enzyme activity in a subtropical natural evergreen broad-leaved forest. Eur. J. For. Res. 138 (6), 917–928. doi:10.1007/s10342-019-01211-4
Lin, D., Dou, P., Yang, G., Qian, S., Wang, H., Zhao, L., et al. (2020). Home‐field advantage of litter decomposition differs between leaves and fine roots. New Phytol. 227 (4), 995–1000. doi:10.1111/nph.16517
Liu, Y., Fang, Y., and An, S. (2020). How C:N:P stoichiometry in soils and plants responds to succession in Robinia pseudoacacia forests on the Loess Plateau, China. For. Ecol. Manag. 475, 118394. doi:10.1016/j.foreco.2020.118394
Liu, Y., Shangguan, Z., and Deng, L. (2021). Vegetation type and soil moisture drive variations in leaf litter decomposition following secondary forest succession. Forests 12 (9), 1195. doi:10.3390/f12091195
Ma, C., Xiong, Y., Li, L., and Guo, D. (2016). Root and leaf decomposition become decoupled over time: Implications for below‐and above‐ground relationships. Funct. Ecol. 30 (7), 1239–1246. doi:10.1111/1365-2435.12619
Martínez-García, L. B., Korthals, G. W., Brussaard, L., Mainardi, G., and De Deyn, G. B. (2021). Litter quality drives nitrogen release, and agricultural management (organic vs. conventional) drives carbon loss during litter decomposition in agro-ecosystems. Soil Biol. Biochem. 153, 108115. doi:10.1016/j.soilbio.2020.108115
McCormack, M. L., Dickie, I. A., Eissenstat, D. M., Fahey, T. J., Fernandez, C. W., Guo, D., et al. (2015). Redefining fine roots improves understanding of below‐ground contributions to terrestrial biosphere processes. New Phytol. 207 (3), 505–518. doi:10.1111/nph.13363
Parsons, S. A., Congdon, R. A., and Lawler, I. R. (2014). Determinants of the pathways of litter chemical decomposition in a tropical region. New Phytol. 203 (3), 873–882. doi:10.1111/nph.12852
Parton, W., Silver, W. L., Burke, I. C., Grassens, L., Harmon, M. E., Currie, W. S., et al. (2007). Global-scale similarities in nitrogen release patterns during long-term decomposition. Science 315 (5810), 361–364. doi:10.1126/science.1134853
Prescott, C. E., and Vesterdal, L. (2021). Decomposition and transformations along the continuum from litter to soil organic matter in forest soils. For. Ecol. Manag. 498, 119522. doi:10.1016/j.foreco.2021.119522
Prieto, I., Almagro, M., Bastida, F., Querejeta, J. I., and Kardol, P. (2019). Altered leaf litter quality exacerbates the negative impact of climate change on decomposition. J. Ecol. 107 (5), 2364–2382. doi:10.1111/1365-2745.13168
Rowland, A., and Roberts, J. (1994). Lignin and cellulose fractionation in decomposition studies using acid‐detergent fibre methods. Commun. Soil Sci. Plant Analysis 25 (3-4), 269–277. doi:10.1080/00103629409369035
Saiya-Cork, K. R, S. R. L., and Zak, D. R. (2002). The effects of long term nitrogen deposition on extracellular enzyme activity in an Acer saccharum forest soil. Soil Biol. Biochem. 34 (9), 1309–1315. doi:10.1016/s0038-0717(02)00074-3
See, C. R., Luke McCormack, M., Hobbie, S. E., Flores‐Moreno, H., Silver, W. L., Kennedy, P. G., et al. (2019). Global patterns in fine root decomposition: Climate, chemistry, mycorrhizal association and woodiness. Ecol. Lett. 22 (6), 946–953.
Shen, Y., Tian, D., Hou, J., Wang, J., Zhang, R., Li, Z., et al. (2021). Forest soil acidification consistently reduces litter decomposition irrespective of nutrient availability and litter type. Funct. Ecol. 35 (12), 2753–2762. doi:10.1111/1365-2435.13925
Singer, A. C., Moorhead, D., Lashermes, G., Recous, S., and Bertrand, I. (2014). Interacting microbe and litter quality controls on litter decomposition: A modeling analysis. PLoS ONE 9 (9), e108769. doi:10.1371/journal.pone.0108769
Song, S., Hu, X., Zhu, J., Zheng, T., Zhang, F., Ji, C., et al. (2021). The decomposition rates of leaf litter and fine root and their temperature sensitivities are influenced differently by biotic factors. Plant Soil 461 (1-2), 603–616. doi:10.1007/s11104-021-04855-7
Sun, T., Hobbie, S. E., Berg, B., Zhang, H., Wang, Q., Wang, Z., et al. (2018). Contrasting dynamics and trait controls in first-order root compared with leaf litter decomposition. Proc. Natl. Acad. Sci. U. S. A. 115 (41), 10392–10397. doi:10.1073/pnas.1716595115
Tan, X., Machmuller, M. B., Huang, F., He, J., Chen, J., Cotrufo, M. F., et al. (2020). Temperature sensitivity of ecoenzyme kinetics driving litter decomposition: The effects of nitrogen enrichment, litter chemistry, and decomposer community. Soil Biol. Biochem. 148, 107878. doi:10.1016/j.soilbio.2020.107878
Tanikawa, T., Fujii, S., Sun, L., Hirano, Y., Matsuda, Y., Miyatani, K., et al. (2018). Leachate from fine root litter is more acidic than leaf litter leachate: A 2.5-year laboratory incubation. Sci. Total Environ. 645, 179–191. doi:10.1016/j.scitotenv.2018.07.038
Veen, G. F. C., Freschet, G. T., Ordonez, A., and Wardle, D. A. (2015). Litter quality and environmental controls of home-field advantage effects on litter decomposition. Oikos 124 (2), 187–195. doi:10.1111/oik.01374
Wang, L., Chen, Y., Zhou, Y., Xu, Z., Tan, B., You, C., et al. (2021a). Environmental conditions and litter nutrients are key determinants of soluble C, N, and P release during litter mixture decomposition. Soil Tillage Res. 209, 104928. doi:10.1016/j.still.2020.104928
Wang, L., Chen, Y., Zhou, Y., Zheng, H., Xu, Z., Tan, B., et al. (2021b). Litter chemical traits strongly drove the carbon fractions loss during decomposition across an alpine treeline ecotone. Sci. Total Environ. 753, 142287. doi:10.1016/j.scitotenv.2020.142287
Wickings, K., Grandy, A. S., Reed, S. C., Cleveland, C. C., and Johnson, N. (2012). The origin of litter chemical complexity during decomposition. Ecol. Lett. 15 (10), 1180–1188. doi:10.1111/j.1461-0248.2012.01837.x
Xia, M., Talhelm, A. F., and Pregitzer, K. S. (2015). Fine roots are the dominant source of recalcitrant plant litter in sugar maple-dominated northern hardwood forests. New Phytol. 208 (3), 715–726. doi:10.1111/nph.13494
Xiao, W., Chen, H. Y., Kumar, P., Chen, C., and Guan, Q. (2019). Multiple interactions between tree composition and diversity and microbial diversity underly litter decomposition. Geoderma 341, 161–171. doi:10.1016/j.geoderma.2019.01.045
Xu, M., Lu, X., Xu, Y., Zhong, Z., Zhang, W., Ren, C., et al. (2020). Dynamics of bacterial community in litter and soil along a chronosequence of Robinia pseudoacacia plantations. Sci. Total Environ. 703, 135613. doi:10.1016/j.scitotenv.2019.135613
Yue, K., García‐Palacios, P., Parsons, S. A., Yang, W., Peng, Y., Tan, B., et al. (2018). Assessing the temporal dynamics of aquatic and terrestrial litter decomposition in an alpine forest. Funct. Ecol. 32 (10), 2464–2475. doi:10.1111/1365-2435.13143
Zhang, D., Hui, D., Luo, Y., and Zhou, G. (2008). Rates of litter decomposition in terrestrial ecosystems: Global patterns and controlling factors. J. Plant Ecol. 1 (2), 85–93. doi:10.1093/jpe/rtn002
Zhang, K., Cheng, X., Dang, H., Ye, C., Zhang, Y., and Zhang, Q. (2013). Linking litter production, quality and decomposition to vegetation succession following agricultural abandonment. Soil Biol. Biochem. 57, 803–813. doi:10.1016/j.soilbio.2012.08.005
Zhang, M., Cheng, X., Geng, Q., Shi, Z., Luo, Y., Xu, X., et al. (2019a). Leaf litter traits predominantly control litter decomposition in streams worldwide. Glob. Ecol. Biogeogr. 28 (10), 1469–1486. doi:10.1111/geb.12966
Zhang, T. a., Luo, Y., Chen, H. Y. H., and Ruan, H. (2018a). Responses of litter decomposition and nutrient release to N addition: A meta-analysis of terrestrial ecosystems. Appl. Soil Ecol. 128, 35–42. doi:10.1016/j.apsoil.2018.04.004
Zhang, W., Gao, D., Chen, Z., Li, H., Deng, J., Qiao, W., et al. (2018b). Substrate quality and soil environmental conditions predict litter decomposition and drive soil nutrient dynamics following afforestation on the Loess Plateau of China. Geoderma 325, 152–161. doi:10.1016/j.geoderma.2018.03.027
Zhang, W., Liu, W., Xu, M., Deng, J., Han, X., Yang, G., et al. (2019b). Response of forest growth to C:N:P stoichiometry in plants and soils during Robinia pseudoacacia afforestation on the Loess Plateau, China. Geoderma 337, 280–289. doi:10.1016/j.geoderma.2018.09.042
Zhang, X., Wang, L., Zhou, W., Hu, W., Hu, J., and Hu, M. (2021). Changes in litter traits induced by vegetation restoration accelerate litter decomposition in Robinia pseudoacacia plantations. Land Degrad. Dev. 33 (1), 179–192. doi:10.1002/ldr.4136
Zheng, H., Chen, Y., Liu, Y., Heděnec, P., Peng, Y., Xu, Z., et al. (2020). Effects of litter quality diminish and effects of vegetation type develop during litter decomposition of two shrub species in an alpine treeline ecotone. Ecosystems 24 (1), 197–210. doi:10.1007/s10021-020-00512-9
Zheng, H., Chen, Y., Liu, Y., Zhang, J., Yang, W., Yang, L., et al. (2018). Litter quality drives the differentiation of microbial communities in the litter horizon across an alpine treeline ecotone in the eastern Tibetan Plateau. Sci. Rep. 8 (1), 10029–10111. doi:10.1038/s41598-018-28150-1
Zheng, H., Yang, T., Bao, Y., He, P., Yang, K., Mei, X., et al. (2021). Network analysis and subsequent culturing reveal keystone taxa involved in microbial litter decomposition dynamics. Soil Biol. Biochem. 157, 108230. doi:10.1016/j.soilbio.2021.108230
Keywords: litter decomposition, litter quality, soil environment, enzyme activity, robinia pseudoacacia
Citation: Su Z, Zhu X, Wang Y, Mao S and Shangguan Z (2022) Litter C and N losses at different decomposition stages of Robinia pseudoacacia: The weaker effects of soil enzyme activities compared with those of litter quality and the soil environment. Front. Environ. Sci. 10:956309. doi: 10.3389/fenvs.2022.956309
Received: 10 June 2022; Accepted: 17 October 2022;
Published: 03 November 2022.
Edited by:
Riccardo Scalenghe, University of Palermo, ItalyReviewed by:
Juying Huang, Ningxia University, ChinaZhenhong Hu, Institute of Soil and Water Conservation (CAS), China
Copyright © 2022 Su, Zhu, Wang, Mao and Shangguan. This is an open-access article distributed under the terms of the Creative Commons Attribution License (CC BY). The use, distribution or reproduction in other forums is permitted, provided the original author(s) and the copyright owner(s) are credited and that the original publication in this journal is cited, in accordance with accepted academic practice. No use, distribution or reproduction is permitted which does not comply with these terms.
*Correspondence: Zhouping Shangguan, U2hhbmdndWFuQG1zLmlzd2MuYWMuY24=