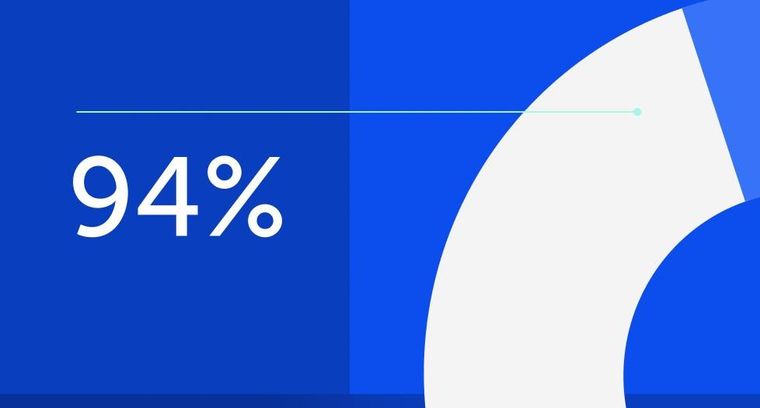
94% of researchers rate our articles as excellent or good
Learn more about the work of our research integrity team to safeguard the quality of each article we publish.
Find out more
ORIGINAL RESEARCH article
Front. Environ. Sci., 01 August 2022
Sec. Freshwater Science
Volume 10 - 2022 | https://doi.org/10.3389/fenvs.2022.950458
This article is part of the Research TopicGulf of Mexico Estuaries: Ecology of the Nearshore and Coastal Ecosystems Impacted by the Deepwater Horizon Oil SpillView all 14 articles
Infaunal sedimentary communities underpin marine ecosystems worldwide. Understanding how disturbances such as oil spills influence infauna is therefore important, especially given that oil can be trapped in sediments for years or even decades. The 2010 Deepwater Horizon (DWH) event was the largest marine oil spill in United States history, impacting habitats throughout the Northern Gulf of Mexico. We investigated infaunal community structure at two shallow sites in the Chandeleur Islands, LA, United States, over a 2-year period from 2015 to 2016 (5–6 years post-spill). One site was moderately contaminated with oil from the DWH spill, while the other was only lightly contaminated. Both sites featured patchy Ruppia seagrass meadows, allowing us to compare infaunal communities between sites for seagrass versus unvegetated sediment. The moderately-oiled site featured a significantly different community than that of the lightly oiled site; these differences were driven by altered abundance of key taxa, with some taxa being less abundant at the moderately oiled site but others more abundant. During our second year of sampling, a crude oil slick moved transiently through the moderately-oiled site, allowing us to directly observe responses to an acute re-oiling event. Virtually every taxonomic and community-level metric declined during the re-oiling, with effects more pronounced in seagrass beds than in unvegetated sediment. The sole exception was the snail, Neritina usnea, which we found exclusively at the more-oiled site. Our observations suggest that oil responses are driven more by key taxa than by entire guilds responding together. By identifying the families and genera that showed the largest signal at this pair of sites, we can begin laying groundwork for understanding which benthic taxa are most likely to be impacted by oil spills, both in the immediate aftermath of a spill and through longer-term contamination. While more studies will certainly be needed, this contribution is a step towards developing clear a priori hypotheses that can inform future oil-spill work. Such hypotheses would help to focus future sampling efforts, allowing resources to be directed towards those taxa that are most likely to be responding, and which are potential bio-indicators of oil exposure.
Oil spills, like climate change and habitat loss, are a hallmark of post-industrial human activities (Kennish, 2002; Jernelöv, 2010; Farrington, 2013; Barron et al., 2020; Rowe et al., 2020). Spills have occurred in every ocean of the world and can be surprisingly frequent (Eckle et al., 2012) –nearly one million gallons of oil are released in US waters annually (US Dept of Transportation, 2017). Oil can remain in habitats for decades or longer (Bejarano and Michel, 2010; Pashaei et al., 2015; Bejarano and Michel, 2016), yet its impacts are most often assessed in the immediate aftermath of the largest spills. Understanding how marine communities are influenced by oil contamination is important, not only for assessing long-term consequences of major disasters, but also for understanding how systems experiencing pervasive oil exposure might be affected.
Sedimentary infaunal communities are the foundation of marine ecosystems worldwide. Infauna contribute secondary productivity to marine food webs, and their activities drive bioturbation and sediment oxygenation, thereby influencing biogeochemical cycling at global scales (Meysman et al., 2006; Mermillod-Blondin, 2011; Solan et al., 2019). Infaunal communities often respond to oiling (Montagna and Harper, 1996; Joydas et al., 2012; Zabbey and Uyi, 2014; Washburn et al., 2016; Reuscher et al., 2017; Reuscher et al., 2020). However, more research has focused on immediate post-spill responses than on longer-term effects (for exceptions, see (Montagna and Harper, 1996; Zerebecki et al., 2021). While responses are usually negative, oiling can facilitate some taxa, most notably the polychaete family Capitellidae and perhaps other deposit-feeding organisms (Daan et al., 1994; Montagna and Harper, 1996). This has been interpreted as a trophic response: if oil provides organic enrichment, it may fuel microbial growth and thereby increase food availability for deposit feeders (Montagna and Harper, 1996).
The Deepwater Horizon (DWH) event was the third largest oil spill of all time, and the single largest marine spill in US history (Beyer et al., 2016). Between April and July 2010, roughly five million barrels of Macondo oil spewed from a broken riser pipe into the Gulf of Mexico. The resulting oil slick, covering nearly 65,000 km2, was visible from space, yet most oil released was likely entrained at depth (Beyer et al., 2016). In shallow coastal habitats, oil can be transported into marine sediments by infaunal bioturbation and by sediment deposition. Even 5 years after the spill, DWH hydrocarbons were detectable in shallow-water sediments at sites in the Northern Gulf of Mexico (NGOM; Robertson and Baltzer, 2017; Duan et al., 2018; Tatariw et al., 2018, Robertson et al. in prep), making long-term impacts likely.
Assessing infaunal responses to oiling presents many challenges. Perhaps most significant is a broad lack of baseline data that would permit comparison between pre- and post-spill communities. Post-spill assessments must primarily rely on comparisons among sites with variable degrees of oiling, which in turn raises issues of how to distinguish oiling effects from other between-site differences. Ideally, studies would sample a large number of sites for a range of oiling intensities, allowing oil effects to be statistically disambiguated from other sources of variance. However, sampling on this scale is often not feasible due to logistical and funding constraints. Furthermore, every oil spill is chemically distinct, and the fractions of oil moving in different portions of the water column at different points of time during the spill can be chemically different. True replication may therefore be elusive for field studies. Another challenge is that taxonomically identifying infaunal samples requires extensive time and expertise. Better a priori hypotheses for which taxa were most likely to respond to oiling would allow future studies to target limited resources on examining the taxa most likely to be impacted.
Here we describe infaunal communities from a pair of sites located in the Chandeleur Islands, LA. One site was moderately contaminated with DWH oil (as determined by Shoreline Cleanup and Assessment Technique maps and published chemical analyses), the other was only lightly oiled but is otherwise physically similar. This pairing permits a focused assessment of taxonomic differences at sites that differ primarily in their oiling history. We sampled in summer and fall of 2015 and 2016, five and 6 years after the DWH spill. The more-oiled site experienced a reoiling event in summer of 2016, in which a tar slick moved transiently through the site. This affords a unique opportunity to document acute community responses to an oiling event, with comparisons to a control site where no known re-oiling occurred. Previous work has suggested that infaunal responses to oil can be mediated by both toxicity and organic enrichment (Daan et al., 1994; Montagna and Harper, 1996; Pashaei et al., 2015). If community-level effects of oiling are dominated by toxicity, then we would predict reduced abundance and diversity at oiled sites. Alternatively, if organic enrichment facilitates deposit feeders, then we might expect to see increased abundances for deposit feeding taxa (Daan et al., 1994; Montagna and Harper, 1996), with possible indirect effects at higher trophic levels. These impacts may affect entire functional groups in similar ways, or species within functional groups may show distinct responses. Species may share inherited traits making them more susceptible to oil disturbance, in which case larger taxonomic groups or evolutionary history may be useful in assessing impacts of oil on communities (addressed in Kiskaddon et al., this issue). By comprehensively evaluating the benthic communities at these sites, we provide insight into different strategies of assessing benthic community recovery, thus advancing a priori hypotheses to inform future work.
The Chandeleur Islands are a chain of low-lying uninhabited barrier islands in the NGOM. Following the DWH spill, no cleanup activities were conducted at the Chandeleurs, making them an ideal location to study oiling effects. The distribution of oil across the barrier island chain was patchy, allowing us to select oiled-unoiled pairs of sites within close proximity. Sites were chosen in collaboration with the ACER consortium (Alabama Center for Ecological Resilience, (Tatariw et al., 2018; Zerebecki et al., 2021). Oiled sites were identified first, then matched to nearby less-oiled but physically similar sites to serve as controls. Both oiled and control sites were initially identified using National Oceanic and Atmospheric Administration (NOAA) Shoreline Cleanup and Assessment Technique (SCAT) maps (Michel et al., 2013), then confirmed by chemical analysis of sediment cores (Robertson and Baltzer, 2017; Tatariw et al., 2018). Here we present data from a single oiled/control pair of sites designated A and B; site A is the less-oiled “control”, while site B is more heavily oiled (Figure 1; Table 1). Both sites are shallow-water (30–50 cm depth) sandy mud flats fringing Chandeleur Sound with large Ruppia seagrass beds and areas of unvegetated sediment (Table 2). The seagrass Thalassia testudinum is also sporadically present. The sites are separated by only 3.7 km, and experience similar salinity, temperature, and dissolved oxygen concentrations (Supplementary Figure S1). Both sites experienced oiling during the DWH spill, but SCAT maps showed more spatially extensive oil at site B (see Zerebecki et al., 2021 Figure 1). SCAT maps are useful but are only one snapshot in time; sediment polycyclic aromatic hydrocarbon (PAH) levels provide more robust evidence of oiling history. Tatariw et al. (2018) assessed oil residues from marsh sediments near both sites and found that Site A (their ‘Site 1’) was only lightly oiled while Site B (their ‘Site 2’) was moderately to heavily oiled. These findings are congruent with data showing higher Benzo(a)pyrene concentrations at Site B in marsh samples of summer 2015 (Robertson and Baltzer, 2017) and in intertidal saltmarsh, subtidal Ruppia seagrass (most likely R. maritina, hereafter referred to as Ruppia), and subtidal unvegetated mud samples of fall 2015 (A. Robertson ms in preparation and pers. Comm; Benzo(a)pyrene is commonly used as an indicator of long-term organic polyaromatic hydrocarbon pollution (Vane et al., 2020). Taken together, these data support the designation of site A as a “control” site suitable for comparison to the more-heavily oiled Site B.
FIGURE 1. Map of the Northern Gulf of Mexico, showing the study sites in Louisiana’s Chandeleur Islands (inset). Site A (29°51′36″N 88°50′24″W) showed low to no evidence of hydrocarbon contamination, while site B (29°53′24″N 88°49′48″W) showed elevated hydrocarbon concentrations throughout the upper 15 cm of sediment. The sites are 3.7 km apart and show similar physical characteristics.
TABLE 1. Sampling periods, dates, and sample sizes for Ruppia and unvegetated (‘open’) habitats at Site A (unoiled) and Site B (oiled).
TABLE 2. Summary of environmental data for each site. Temperature and DO (means ± SD) were measured from HOBO oxygen sensors being used to measure sediment metabolism at each site (Dorgan et al. in preparation).
Sampling was carried out during four time periods in the summer and fall of 2015 and of 2016 (Table 1). Within each site, we sampled Ruppia seagrass habitats as well as unvegetated mud (n = 8 to 18 per season/site/habitat, Table 1). Each sample consisted of either a 14.5 cm diameter x 15 cm deep sediment core or a 9.5 cm diam x 15 cm deep core, collected from mud submerged in 15–40 cm water depth. The larger cores were tied to a sediment metabolism study being conducted concurrently, which required larger cores to accommodate sensors (Dorgan et al. ms in preparation); the smaller cores were added to increase our sample size with less processing time. Abundances were standardized to the larger core using the formula (Aobserved ⋅ 0.0071 m−2) ⋅ 2.324 = Aadjusted ⋅ 0.0165 m−2. Each core was sieved in the field; organisms retained on a 500 μm mesh were transferred to 95% ethanol with Rose Bengal stain (0.05 g L−1). Organisms were later sorted, identified to the lowest taxonomic level possible, and counted.
In summer 2016, we discovered a large slick of crude oil at the oiled site, Site B. The slick measured roughly 50 cm wide by 5 + m long and was caught in vegetation at the edge of the marsh, immediately below the water line (Robertson et al. in preparation), and was closer to the Ruppia seagrass beds than it was to unvegetated mud. The slick may have been transported into the site at some point after our fall 2015 sampling, perhaps during a storm. Alternatively, it may have been buried below sediment surfaces and then exposed by scour during a storm. Either way, we interpret this as a reoiling event. The slick was no longer evident when we returned to the site in fall 2016, although oil was detected nearby (∼650 m away) in Nov. 2016 and Feb. 2017 (Tatariw et al., 2018).
It is important to recognize that samples from a single pair of sites cannot statistically enumerate an “oil effect”—such estimation would require replicate random sampling from a theoretical population of “oiled” sites in comparison to a population of unoiled sites. Rather, this study provides an in-depth comparison of two sites that are known to differ primarily in their oiling history. Furthermore, the re-oiling event that we document is a truly unique opportunity to observe immediate responses to oiling in a single place and time.
Non-metric Multidimensional Scaling: To compare community structure at oiled versus unoiled sites, we conducted a non-metric multidimensional scaling (NMDS) analysis using the R package ‘vegan’ (Oksanen et al., 2013). We used ‘isoMDS’ (k = 2) with a Bray-Curtis dissimilarity matrix obtained via ‘vegdist’. Differences were assessed using permutational multivariate analysis of variance (PERMANOVA, 999 permutations) using the function ‘adonis2’, and assumptions of homogeneity of dispersions were tested using the function ‘betadisper’. A three-way model for site × habitat × sampling period suffered from significant heterogeneity of dispersions, primarily associated with higher dispersion following the summer 2016 reoiling event. Unlike univariate ANOVA, there is no alternative to PERMANOVA when assumptions are violated (Anderson, 2014; Anderson et al., 2017). It is well known that infaunal communities are highly variable across seasons and years, making temporal variability the least biologically interesting model term. Thus, to focus more deeply on patterns for sites and habitats, we rejected the three-way model and focused instead on two-way PERMANOVAs (again evaluated for homogeneity of dispersion) for site × habitat effects within each sampling period.
Other Diversity Metrics: We assessed differences in standard diversity metrics (Shannon, Simpson, and Inverse Simpson diversity) plus total abundance, taxon richness, and functional richness. Taxon richness was estimated at the family level for taxa that could be reliably identified to family or lower; but at the level of ‘order’ when lower identification would have been prohibitively time-consuming (see Table 1). Statistical methods for these univariate metrics are detailed below.
To evaluate which taxa contribute most to differences revealed by NMDS, we examined patterns in abundance for the most abundant taxa observed. We also comprehensively explored the data for all individual taxa and for other levels of aggregation (i.e., genus, functional groups, feeding guilds) to ensure that we were not missing important patterns, but no consistent patterns emerged. Analyses presented here were conducted at the family level, but we have verified that aggregating taxa at the genus level does not change any interpretations.
We examined community and taxon-level responses to oiling and habitat type using ANOVA. Abundance and diversity data were first evaluated for normality and square-root transformed if needed. We then examined a three-way model for site × habitat × sampling period, and examined residuals for heteroscedasticity. In many cases, residual patterning suggested a poor model fit. Rather than attempt three-way non-parametric tests, we employed separate two-way ANOVAs for site × habitat within each sampling period. This approach is reasonable because seasonal and inter-annual variability is expected in benthic habitats, and we were not primarily interested in estimating year or season effects. Non-significant interaction terms were dropped, and if the ‘habitat’ main effect was non-significant it, too, was dropped in favor of a one-way ANOVA for the ‘site’ main effect. All significant ANOVAs were followed by Tukey post-hoc tests (after adjusting for normality and checking residuals for heteroscedasticity).
If residual patterns suggested that two-way ANOVA model assumptions were violated, we instead performed the non-parametric Kruskal–Wallis test. Kruskal–Wallis cannot handle two-way interactions, so we pooled together data from both habitats and assessed the ‘site’ term only (after confirming that separate Kruskal–Wallis tests for each habitat would not have altered our interpretation, not shown).
Ecological data can be explored at many taxonomic or functional levels, leading to multiple comparisons in most community studies. This can compound the risk of Type I error–falsely rejecting a true null hypothesis. In the ecological literature, there is little consensus about when and how to offset this risk (reviewed Cabin and Mitchell, 2000; Moran, 2003; García, 2004); many published studies ignore the issue entirely. In this study, we were primarily interested in evaluating differences between sites. Because we may or may not expect to see an oil effect, this is where the greatest risk of false discovery lies. We also evaluated ‘habitat’ effects, because infaunal communities are usually different in seagrass versus unvegetated areas (Bostrom et al., 2006; Rodil et al., 2021). Given this strong a priori knowledge, we see false discovery in the ‘habitat’ term as being unlikely. We have therefore applied the Benjamini–Hochberg (B-H) method to adjust p-value interpretations for the ‘site’ main effect for all ANOVA and Kruskal–Wallis analyses. We report raw p-values throughout the main text; the B-H correction did not alter interpretation of most tests, and the few exceptions will be clearly indicated. A more detailed explanation of false discovery rate and our rationale is in Supplementary Appendix S1; results of the B-H procedure are in Supplementary Table S2.
We used NMDS and PERMANOVA to evaluate community composition for Ruppia vs unvegetated habitats at oiled vs unoiled sites in summer and fall of 2015 and 2016. Oiled and unoiled sites cluster separately, as do Ruppia and unvegetated habitats within each site (Figure 2). Site, habitat, and site × habitat interactions were highly significant in all four sampling periods (Table 3; full statistics in Supplementary Appendix S2). However, site (oiled vs. unoiled) had the greatest explanatory power for both summer sampling periods (2015 R2 = 0.25; 2016 R2 = 0.21). For fall samples, the site and habitat terms captured similar variance in 2015 (site R2 = 0.17; habitat R2 = 0.19), but the habitat term captured more variance in fall 2016 (R2 = 0.28) (Table 3 and Supplementary Appendix S2). Notably, there was no overlap between the cluster of samples from the Ruppia habitat at the oiled site in Summer 2016 and samples from other habitats and sampling times (Figure 2).
FIGURE 2. Nonmetric Multidimensional Scaling for infaunal communities in cores from unvegetated or Ruppia habitat at oiled vs. unoiled sites in each of the four sampling periods (A: Summer 2015; B: Fall 2015; C: Summer 2016; D: Fall 2016). A single ordination was performed using family-level abundance data from all four sampling periods (stress = 0.205). Each plot highlights samples from a single year/season. The convex hulls enclose all samples from a given site, while habitats are differentiated using color. In each sampling period, both Site and Habitat were significantly different by PERMANOVA (Table 2, Supplementary Appendix S2). The Summer 2016 panel is highlighted to emphasize the reoiling event observed at that time.
TABLE 3. Summary of p-values for community-level analyses. PERMANOVA results from PERMANOVA apply to the NMDS analysis shown in Figure 2. ANOVA results accompany Figure 3. Full statistical tables are in Supplementary Appendix S2. When a p < 0.05 is marked (ns), this indicates that the p-value should not be considered significant after applying the Benjamini–Hochberg procedure (Supplementary Table S2).
To explore community-level patterns more deeply, we examined total abundance, taxonomic richness, and Shannon diversity as a function of site and habitat within each sampling period. Total abundance was 2-fold–6-fold lower at the oiled site following the reoiling event in summer 2016 (Figure 3A; Table 3, Supplementary Appendix S2; site F1,46 = 48.3, p << 0.0001; habitat F1,46 = 0.52, ns; site x habitat F1,46 = 11.04, p << 0.0001). However, neither ‘site’ main effects nor interaction terms were significant in other sampling periods (Table 3 and Supplementary Appendix S2). Unvegetated habitats had lower abundances than Ruppia habitats during both fall sampling periods, but not in summer samples (Table 3 and Supplementary Appendix S2).
FIGURE 3. Diversity and abundance metrics for oiled vs. unoiled sites in unvegetated vs. Ruppia habitats for each sampling period. The Summer 2016 panels are highlighted to emphasize the reoiling event observed at that time. Letters indicate Tukey groupings; full ANOVA results are given in Table ANOVA. (A) Total abundance per core; (B) Taxonomic richness (taxa defined at the family level, or higher if ID’ing to family was not possible). (C) Shannon diversity index; other diversity indices showed similar patterns.
Taxonomic richness was 2.6-fold lower at the oiled Ruppia site following the summer 2016 reoiling event (Figure 3B; Table 3; Supplementary Appendix S2; site F1,46 = 39.81, p << 0.0001), but showed no site differences in other sampling periods. (Supplementary Appendix S2). Note that the ‘site’ term yielded a p-value of 0.03 for Summer 2015, but this was not significant after applying the B-H procedure (Supplementary Table S2). Overall, richness was higher in Ruppia than in unvegetated habitats for all four sampling periods, particularly at the less oiled site (Figure 3B, Supplementary Appendix S2).
Shannon diversity was 28% lower at oiled Ruppia habitats following the summer 2016 reoiling event (Figure 3C; Table 3, Supplementary Appendix S2 site F1,138 = 10.2, p = 0.002). Overall, however, Shannon diversity patterns were inconsistent across sites and habitats, driving significant interaction terms (Table 3 and Supplementary Appendix S2). Results for the Simpson and Inverse Simpson indices were broadly similar to the Shannon index (not shown).
To understand which taxa contributed most to observed differences at the oiled site, we first identified the most abundant taxa overall. After examining a rank-abundance plot, we identified a breakpoint after the top ten taxa, after which abundances remain low and shallowly decreasing (Supplementary Figure S2). Of the top ten taxa, one was a catch-all group of minute gastropods that we could not identify to family; these were consequently not analyzed further. Another was the annelid family Maldanidae, which we also excluded because it was only abundant in 2015, and almost entirely absent from both sites in 2016. The eight remaining most abundant taxa were the annelid families Capitellidae, Spionidae, Nereididae, Paraonidae, and Ampharetidae; the gastropod Neritina usnea (the only neritid in our samples); the bivalve family Tellinidae; and the crustacean order Amphipoda (excluding the tube-building family Ampeliscidae, which was enumerated separately and was less common). In addition to these eight taxa, we examined patterns for the annelid Arenicola cristata (the only arenicolid in this region), given a priori knowledge about its ecologically important effects on sediment structure and surrounding communities (reviewed Berke, 2010; Volkenborn et al., 2010). Some of these focal taxa showed generally negative responses to oiling. Others showed generally positive responses, while a third group showed mixed or no response. Notably, however, all taxa except N. usnea declined in abundance following the summer 2016 reoiling event. Most of these appeared to rebound by fall 2016.
Negative Responses to Oiling: The annelid families Capitellidae, Spionidae, and Arenicolidae generally showed lower abundance at the oiled site (with some exceptions) (Figure 4). This pattern was strongest for Ruppia samples–indeed, all three taxa were entirely absent from oiled Ruppia following the 2016 reoiling event (S16 capitellid ‘site’ F1,46 = 392.4, p << 0.0001; spionid F1,46 = 23.78, p << 0.0001; arenicolid K-W
FIGURE 4. Abundance data for families that showed negative responses to oiling (all here are in phylum Annelida). The Summer 2016 panels are highlighted to emphasize the reoiling event observed at that time. For panels (A,B), boxes with different letters are different by Tukey post-hoc tests. Arenicolid data (C) was heavily dominated by 0 values, making boxplots inappropriate visualizations–instead, we are showing raw data (circles) overlaid with means (horizontal bars) and 95% confidence intervals for the mean (shaded rectangles; in cases where no 95%CI is visible, all abundances were ‘0’).
TABLE 4. Summary of ANOVA p-values for square-root transformed abundances of individual taxa (Figures 4–6). Full ANOVA tables are in Supplementary Appendix S3; statistics for taxa with non-normal abundances are in Table 5. In this table, all p < 0.05 remain significant after applying the Benjamini–Hochberg procedure (Supplementary Table S2).
For capitellids, summertime abundance was consistently lower at the oiled site. In fall samples, however, patterns were mixed. The largest effects were observed in Ruppia beds. At the oiled site, capitellid abundance in Ruppia was 7-to-8-fold lower than at the unoiled site sampling period except fall 2016. In unvegetated muds, capitellids were generally 2-to-6-fold less abundant at the oiled site in every sampling period except fall 2015 (Figure 4; Table 4, Supplementary Appendix S3).
Spionids were 5-fold less abundant in oiled Ruppia samples in both summer sampling periods, and 3-fold less abundant in oiled unvegetated samples in summer 2015. However, there were no site differences in fall samples (Figure 4; Table 4, Supplementary Appendix S3).
Positive Response to Oiling: The gastropod N. usnea was notably only found at the oiled site, being entirely absent from the control site. (Figure 5; Table 5, Supplementary Appendix S3). N. usnea was also primarily confined to Ruppia beds, with abundances ranging from 0.63 ± 1.41 to 18.25 ± 11.49 ind · 0.0165 m−2.
TABLE 5. Summary of Kruskal–Wallis p-values for site differences for individual taxon abundances that could not be adjusted for normality. Full Kruskal–Wallis tables are in Supplementary Appendix S3. When a p < 0.05 is marked (ns), this indicates that the p-value should not be considered significant after applying the Benjamini–Hochberg procedure (Supplementary Table S2).
FIGURE 5. Abundance data for families that showed positive responses to oiling (A) Tellinidae (Mollusca: Bivalvia). (B) Nereididae (Annelida). (C) Neritidae (Mollusca:Gastropoda), here represented by Neritina usnea. The Summer 2016 panels are highlighted to emphasize the reoiling event observed at that time. Boxes with different letters are different by Tukey post-hoc tests.
The families Tellinidae (Bivalvia) and Nereididae (Annelida) were either more abundant at the oiled site or showed no pattern. The important exception, however, was that both taxa were less abundant in Ruppia beds at the oiled site following the summer 2016 reoiling event, when nereids were nearly 5-fold less abundant at the oiled site (ctrl 2.03 ± 1.8 vs. oiled 0.43 ± 0.85 ind · 0.0165 m−2) and tellinids nearly 20-fold less abundant (ctrl 5.4 ± 4.5 vs. oiled 0.29 ± 0.60 ind · 0.0165 m−2). Otherwise, Nereids were 3-fold more abundant at the oiled Ruppia site in both fall 2015 and 2016, and in unvegetated mud in 2015. Tellinids were at least 3-fold more abundant at the oiled site (both habitats) in summer 2015, but showed no ‘site’ response in fall samples.
Mixed Response to Oiling: The remaining most abundant taxa—Crustacean amphipods, and the annelid families Paraonidae and Ampharetidae—all showed mixed responses to oiling in general, but nonetheless declined in Ruppia after the 2016 reoiling event (Figure 6). For ampharetids, this pattern continued into fall 2016. In contrast, however, both ampharetids and paraonids were more abundant at the oiled site throughout 2015. Amphipods showed no clear patterns outside of the reoiling event (Figure 6; Tables 4-5, Supplementary Appendix S3).
FIGURE 6. Abundance data for abundant taxa that showed mixed responses to oiling (A) Amphipoda (Arthropoda:Crustacea). (B) Paraonidae (Annelida). (C) Ampharetidae (Annelida). The Summer 2016 panels are highlighted to emphasize the reoiling event observed at that time. Boxes with different letters are different by Tukey post-hoc tests.
While community structure clearly differed between sites in every sampling period, our single most consistent finding is widespread negative responses to the reoiling event of summer 2016. The slick we observed was caught in marsh grass near the shoreline and was closest to the site’s Ruppia habitats. It is therefore unsurprising that the strongest responses were observed in Ruppia–indeed, all univariate metrics, at both the community and taxon level, declined in Ruppia at the oiled site in summer 2016. The sole exception was Neritina usnea abundance, discussed more below. Unvegetated samples qualitatively showed a similar trend, and ‘site’ main effects were pervasively significant for summer 2016. These acute responses, however, appear to have been transient: the heavily oiled site looked generally similar to the less-oiled site in fall 2016 samples. We cannot know how long the slick remained at the site; we do know that it was gone during the fall 2016 sampling (for that matter, we cannot know that the control site never experienced re-oiling; we do know that we never observed such an event there, and oil contamination in sediments from the control site were lower.) This type of transient reoiling was also noted by Beyer et al. (2016), who reviewed information about the changing toxicity profiles of tar balls and other weathered oil. Understanding and predicting oil slick movement has long been a focus of oceanographic research, but most work has focused on short-term modeling in the cleanup phase of an oil spill (Kim et al., 2014; Lee et al., 2020). Understanding how small slicks move around marine habitats over scales of years and decades has, to our knowledge, received less attention; slicks like the one we observed are probably too small to detect in satellite imagery, which could make them difficult to track and model. Evaluating how smaller oil slicks move in shallow marine environments, and how communities respond to their presence, would be an interesting target for further research.
The observational nature of this study makes it impossible to fully rule out the possibility that site differences were caused by some unknown factor(s), unrelated to oil. However, given the sites’ similarity in terms of temperature, salinity, oxygen saturation, sediment type, and vegetation, we see oiling history as the most likely explanation. Quantitatively estimating what proportion of between-site variability is attributable to oil would naturally require a much larger study.
Shifts in community structure appear to have been driven by strong responses in select taxa, some negative but others positive. The two most abundant taxa, polychaete families Capitellidae and Spionidae, clearly appear sensitive to oil at these sites. For capitellids this is surprising, given that Capitella capitata is a well-known indicator species with high tolerance for environmental pollutants (Dean, 2008). C. capitata can metabolize at least some PAHs (Forbes et al., 2001), and has dominated oil-contaminated muds in the Niger river delta (Zabbey and Uyi, 2014)and the Netherlands (Daan et al., 1994). Unfortunately, capitellids are one of the more challenging groups to identify beyond family, and genus-level identification would have been prohibitively time-consuming given the large scope of taxonomic work for this study. However, reexamining a subset of our samples showed that Capitella cf. Capitata is certainly present at these sites, comprising ≥50% of the individuals in Capitellidae overall. Interestingly, all capitellids at the oiled Ruppia site in fall 2016 were C. cf. Capitata, whereas the unoiled Ruppia site had a mix of capitellid species (Supplementary Figure S3). Together with the total absence of capitellids at the reoiled Ruppia site in summer 2016, this suggests that oil toxicity overwhelmed C. cf. Capitata during the reoiling event, but the species recruited opportunistically once the oil slick had moved away.
The lugworm Arenicola cristata also declined in samples from the oiled site. While not highly abundant, it did occur commonly (ranging from 0 to 3 worms per core), and is by far the largest polychaete we observed (most individuals 3–4 cm in length). Lugworms are well-known ecosystem engineers, building deep burrows of compacted sediment that they actively irrigate (Wells, 1966). The hydraulic forces generated by irrigation and defecation events drive porewater movement laterally at distances of 10–20 cm from the burrow (Volkenborn et al., 2010). Lugworms also have a unique funnel-feeding mechanism in which they pull surface sediment down into the burrow for feeding then later defecate on the surface. Together, their feeding and burrowing activities contribute to vertical mixing in the upper 10–20 cm of sediment (Volkenborn and Reise, 2007). Even modest changes to lugworm density may therefore have substantial changes on sediment microbial communities and nutrient cycling, with possible cascading effects to other infauna. Experimentally excluding lugworms can increase polychaete diversity (Volkenborn and Reise, 2007); it is possible that tellinids and nereids increased at the oiled site in part due to the near-total absence of bioturbation and disturbance by lugworms. That said, baseline lugworm density is much lower here than that reported by Volkenborn and Reise (2007), so lugworm impacts may not be as extensive.
While several taxa increased in abundance at the oiled site, these effects were inconsistent over time, making interpretation difficult. However, the pattern is unequivocal, however, for Neritina usnea, which we observed only at the oiled site, even after the reoiling event. Moreover, while previous information on N. usnea in seagrass beds is scarce, densities at the oiled site greatly exceed published abundances for other N. usnea populations in Halodule wrightii beds from the northern Gulf of Mexico (Cebrian et al., 2013). This suggests that attention should be directed at evaluating the mechanisms driving this snail’s positive response. N. usnea differs from other taxa surveyed in being comparatively large-bodied (∼2–5 mm shell length) and entirely epibenthic, found primarily on seagrass blades. We considered the possibility that N. usnea larvae cannot access the control site, but N. usnea is similar to other taxa from these samples in having planktonic larvae (Heard and Lutz, 1982). An explanation invoking larval supply would thus need to explain why N. usnea larvae are uniquely unable to get to this site when all other pelagic dispersers are unhindered. A post-settlement explanation seems more likely; we could speculate that these snails may be exploiting a predation refuge at the oiled site (i.e. if oil-contaminated sediments are less attractive to mobile crustacean and fish predators, then predation risk could be lower at the oiled site). Our sampling methods would not capture mobile epibenthic fauna, but future work exploring epibenthic communities in oiled seagrass beds would be highly valuable.
Most studies of infaunal responses to oil spills have found evidence that oil toxicity generally reduces abundance/diversity (Montagna et al., 2013; Reuscher et al., 2020), with notable exceptions for some more tolerant opportunistic species (Daan et al., 1994; Bejarano and Michel, 2010; McCall and Pennings, 2012; Pashaei et al., 2015). Long term effects may be more complex. While high marsh fauna in the Chandeleurs were less abundant at oiled sites more than 5 years after the DWH (Zerebecki et al., 2021), more nuanced trophic cascades can also drive longer-term patterns. Montagna and Harper (1996) suggested a paradigm for long-term effects in which organic enrichment from oil can boost deposit-feeding taxa, even as toxicity continues to reduce abundance of more sensitive taxa. In contrast, the numerically most abundant subsurface deposit feeder in our study–Capitellidae–was less abundant at the oiled site. However, this did not extend to other deposit-feeding groups such as Paraonidae (or Orbiniidae, not shown), and organic enrichment could contribute to positive responses in Tellinidae (which switch between surface deposit and suspension feeding) and the omnivorous Nereididae. More broadly, we examined data for each of the major infaunal feeding modes, but found no clear patterns. Similarly, we found that for these sites, incorporating evolutionary relatedness by calculating phylogenetic diversity did not add to our understanding of oil impacts on infaunal communities; instead phylogenetic diversity was strongly correlated with taxon richness (Kiskaddon et al., this issue). Rather than grouping by feeding mode, we would recommend that future studies examine (at minimum) tellinid bivalves plus four polychaete families: Capitellidae, Spionidae, Nereididae, and Arenicolidae. A focus on N. usnea merits consideration as well when impacts of oiling is assessed in seagrass beds. These recommendations acknowledge that multiple sampling methodologies may need to be conducted to quantify adequately the targeted benthic taxa.
Given the reoiling event we observed, it is difficult to truly know how often and how recently these communities have experienced acute oil exposure. Long term responses to oiling may be more dynamic than realized, as oil continues to move about habitats. Deeply understanding the long-term effects of oil spills may require more active monitoring over long periods of time, with an eye towards quantifying the continued movement of smaller tar-balls and oil slicks. Outside of reoiling events, it seems clear that oiled sites can exhibit high levels of infaunal diversity. However, stable ‘recovery’ from an oil spill may not be possible as long as mobile oil remains in the habitat. At regional scales, the spatial and temporal extent of reoiling may determine how long it takes to fully return to pre-spill levels of ecosystem functioning.
The datasets presented in this study can be found in online repositories. The names of the repository/repositories and accession number(s) can be found below: https://data.gulfresearchinitiative.org, doi:10.7266/N7CF9NFD.
SKB, KD, and SB planned the study. SKB and KD oversaw all field collection and taxonomic work. SKB, KD, EPK, KG, WC, ELK, and TC participated in field work and taxonomic work. SKB conducted the analyses and wrote the paper. KD, SB, and EPK provided valuable feedback and helped refine the draft.
This research was made possible by a grant from The Gulf of Mexico Research Initiative through the Alabama Center for Ecological Resilience Consortium administered by the Dauphin Island Sea Lab.
We thank Captain Brian Gagnon and the Southern Way crew of Southern Magnolia Charters in Pass Christian, MS, for facilitating access to our field sites. We also thank Alison Robertson, Will Ballentine, Erica Weldin, Shelby Budai and Stephen Hesterberg for assistance with field work and invertebrate identification.
The authors declare that the research was conducted in the absence of any commercial or financial relationships that could be construed as a potential conflict of interest.
All claims expressed in this article are solely those of the authors and do not necessarily represent those of their affiliated organizations, or those of the publisher, the editors and the reviewers. Any product that may be evaluated in this article, or claim that may be made by its manufacturer, is not guaranteed or endorsed by the publisher.
The Supplementary Material for this article can be found online at: https://www.frontiersin.org/articles/10.3389/fenvs.2022.950458/full#supplementary-material
Anderson, M. J. (2014). Permutational multivariate analysis of variance (PERMANOVA). 3rd ed. Chichester, UK: American Cancer Society.
Anderson, M. J., Walsh, D. C. I., Clarke, K. R., Gorley, R. N., and Castro, E. G. (2017). Some solutions to the multivariate Behrens–Fisher problem for dissimilarity-based analyses. Aust. N. Z. J. Stat. 59, 57–79. doi:10.1111/anzs.12176
Barron, M. G., Vivian, D. N., Heintz, R. A., and Yim, U. H. (2020). Long-term ecological impacts from oil spills: comparison of exxon valdez , hebei spirit , and deepwater horizon. Environ. Sci. Technol. 54, 6456–6467. doi:10.1021/acs.est.9b05020
Bejarano, A. C., and Michel, J. (2010). Large-scale risk assessment of polycyclic aromatic hydrocarbons in shoreline sediments from Saudi Arabia: environmental legacy after twelve years of the Gulf war oil spill. Environ. Pollut. 158, 1561–1569. doi:10.1016/j.envpol.2009.12.019
Bejarano, A. C., and Michel, J. (2016). Oil spills and their impacts on sand beach invertebrate communities: a literature review. Environ. Pollut. 218, 709–722. doi:10.1016/j.envpol.2016.07.065
Berke, S. K. (2010). Functional groups of ecosystem engineers: a proposed classification with comments on current issues. Integr. Comp. Biol. 50, 147–157. doi:10.1093/icb/icq077
Beyer, J., Trannum, H. C., Bakke, T., Hodson, P. V., and Collier, T. K. (2016). Environmental effects of the deepwater horizon oil spill: a review. Mar. Pollut. Bull. 110, 28–51. doi:10.1016/j.marpolbul.2016.06.027
Bostrom, C., Jackson, E. L., and Simenstad, C. A. (2006). Seagrass landscapes and their effects on associated fauna: a review. Estuar. Coast. Shelf Sci. 68, 383–403. doi:10.1016/j.ecss.2006.01.026
Cabin, R. J., and Mitchell, R. J. (2000). To bonferroni or not to bonferroni: when and how are the questions. Bull. Ecol. Soc. Am. 81, 246–248. doi:10.2307/20168454
Cebrian, J., Stutes, J., and Christiaen, B. (2013). Effects of grazing and fertilization on epiphyte growth dynamics under moderately eutrophic conditions: Implications for grazing rate estimates. Mar. Ecol. Prog. Ser. 474, 121–133.
Daan, R., Mulder, M., and Van Leeuwen, A. (1994). Differential sensitivity of macrozoobenthic species to discharges of oil-contaminated drill cuttings in the North Sea. Neth. J. Sea Res. 33, 113–127. doi:10.1016/0077-7579(94)90056-6
Dean, H. K. (2008). The use of polychaetes (Annelida) as indicator species of marine pollution: a review. Rev. Biol. Trop. 56, 29.
Duan, J., Liu, W., Zhao, X., Han, Y., O’Reilly, S. E., Zhao, D., et al. (2018). Study of residual oil in Bay Jimmy sediment 5 years after the Deepwater Horizon oil spill: persistence of sediment retained oil hydrocarbons and effect of dispersants on desorption. Sci. Total Environ. 618, 1244–1253. doi:10.1016/j.scitotenv.2017.09.234
Eckle, P., Burgherr, P., and Michaux, E. (2012). Risk of large oil spills: a statistical analysis in the aftermath of deepwater horizon. Environ. Sci. Technol. 46, 13002–13008. doi:10.1021/es3029523
Farrington, J. W. (2013). Oil pollution in the marine environment I: Inputs, big spills, small spills, and dribbles. Environ. Sci. Policy Sustain. Dev. 55, 3–13. doi:10.1080/00139157.2013.843980
Forbes, V. E., Andreassen, M. S. H., and Christensen, L. (2001). Metabolism of the polycyclic aromatic hydrocarbon fluoranthene by the polychaete Capitella capitata species I. Environ. Toxicol. Chem. 20, 1012–1021. doi:10.1002/etc.5620200511
García, L. V. (2004). Escaping the Bonferroni iron claw in ecological studies. Oikos 105, 657–663. doi:10.1111/j.0030-1299.2004.13046.x
Heard, R., and Lutz, L. (1982). Guide to common tidal marsh invertebrates of the northeastern Gulf of Mexico. Ocean Springs, MS: Mississippi Alabama Sea Grant Consortium.
Jernelöv, A. (2010). The threats from oil spills: now, then, and in the future. AMBIO 39, 353–366. doi:10.1007/s13280-010-0085-5
Joydas, T. V., Qurban, M. A., Al-Suwailem, A., Krishnakumar, P. K., Nazeer, Z., Cali, N. A., et al. (2012). Macrobenthic community structure in the northern Saudi waters of the Gulf, 14 years after the 1991 oil spill. Mar. Pollut. Bull. 64, 325–335. doi:10.1016/j.marpolbul.2011.11.007
Kennish, M. J. (2002). Environmental threats and environmental future of estuaries. Environ. Conserv. 29, 78–107. doi:10.1017/s0376892902000061
Kim, T.-H., Yang, C.-S., Oh, J.-H., and Ouchi, K. (2014). Analysis of the contribution of wind drift factor to oil slick movement under strong tidal condition: hebei spirit oil spill case. PLoS One 9, e87393. doi:10.1371/journal.pone.0087393
Lee, K.-H., Kim, T.-G., and Cho, Y.-H. (2020). Influence of tidal current, wind, and wave in Hebei Spirit oil spill modeling. J. Mar. Sci. Eng. 8, 69. doi:10.3390/jmse8020069
McCall, B. D., and Pennings, S. C. (2012). Disturbance and recovery of salt marsh arthropod communities following bp deepwater horizon oil spill. PLoS One 7, e32735. doi:10.1371/journal.pone.0032735
Mermillod-Blondin, F. (2011). The functional significance of bioturbation and biodeposition on biogeochemical processes at the water–sediment interface in freshwater and marine ecosystems. J. North Am. Benthol. Soc. 30, 770–778. doi:10.1899/10-121.1
Meysman, F. J. R., Middelburg, J. J., and Heip, C. H. R. (2006). Bioturbation: a fresh look at Darwin’s last idea. Trends Ecol. Evol. 21, 688–695. doi:10.1016/j.tree.2006.08.002
Michel, J., Owens, E. H., Zengel, S., Graham, A., Nixon, Z., Allard, T., et al. (2013). Extent and degree of shoreline oiling: deepwater Horizon oil spill, Gulf of Mexico, USA. PLoS One 8, e65087. doi:10.1371/journal.pone.0065087
Montagna, P. A., Baguley, J. G., Cooksey, C., Hartwell, I., Hyde, L. J., Hyland, J. L., et al. (2013). Deep-sea benthic footprint of the Deepwater Horizon blowout. PLoS One 8, e70540. doi:10.1371/journal.pone.0070540
Montagna, P. A., and Harper, D. E. (1996). Benthic infaunal long-term response to offshore production platforms in the Gulf of Mexico. Can. J. Fish. Aquat. Sci. 53, 2567–2588. doi:10.1139/f96-215
Moran, M. D. (2003). Arguments for rejecting the sequential Bonferroni in ecological studies. Oikos 100, 403–405. doi:10.1034/j.1600-0706.2003.12010.x
Oksanen, J., Blanchet, F. G., Kindt, R., and Legendre, P. (2013). Vegan: community ecology package. R-package version 2.0-10. Available at: https://github.com/vegandevs/vegan (Accessed July 1, 2021).
Pashaei, R., Gholizadeh, M., and Iran, K. J. (2015). The effects of oil spills on ecosystem at the Persian Gulf. Int. J. Rev. Life. Sci. 5, 82–89. doi:10.13140/RG.2.1.2239.3684
Reuscher, M. G., Baguley, J. G., Conrad-Forrest, N., Cooksey, C., Hyland, J. L., Lewis, C., et al. (2017). Temporal patterns of Deepwater Horizon impacts on the benthic infauna of the northern Gulf of Mexico continental slope. PLoS One 12, e0179923. doi:10.1371/journal.pone.0179923
Reuscher, M. G., Baguley, J. G., and Montagna, P. A. (2020). The expanded footprint of the Deepwater Horizon oil spill in the Gulf of Mexico deep-sea benthos. PLoS One 15, e0235167. doi:10.1371/journal.pone.0235167
Robertson, A., and Baltzer, K. (2017). Benzo(a)Pyrene analysis of sediment from the Chandeleur islands, Louisiana in june 2015. Corpus Christi: Harte Research Institute, Texas A&M University. doi:10.7266/N77942RW
Rodil, I. F., Lohrer, A. M., Attard, K. M., Hewitt, J. E., Thrush, S. F., Norkko, A., et al. (2021). Macrofauna communities across a seascape of seagrass meadows: environmental drivers, biodiversity patterns and conservation implications. Biodivers. Conserv. 30, 3023–3043. doi:10.1007/s10531-021-02234-3
Rowe, G. T., Fernando, H., Elferink, C., Ansari, G. A. S., Sullivan, J., Heathman, T., et al. (2020). Polycyclic aromatic hydrocarbons (PAHs) cycling and fates in Galveston Bay, Texas, USA. PLoS One 15, e0243734. doi:10.1371/journal.pone.0243734
Solan, M., Ward, E. R., White, E. L., Hibberd, E. E., Cassidy, C., Schuster, J. M., et al. (2019). Worldwide measurements of bioturbation intensity, ventilation rate, and the mixing depth of marine sediments. Sci. Data 6, 58. doi:10.1038/s41597-019-0069-7
Tatariw, C., Flournoy, N., Kleinhuizen, A., Tollette, D., Overton, E., Sobecky, P., et al. (2018). Salt marsh denitrification is impacted by oiling intensity six years after the Deepwater Horizon oil spill. Environ. Pollut. 243, 1606–1614. doi:10.1016/j.envpol.2018.09.034
US Dept of Transportation (2017). Petroleum oil spills impacting navigable U.S. Waterways. Table 4-54, bureau of transportation statistics. Available at: https://www.bts.gov/archive/publications/national_transportation_statistics/table_04_54 (Accessed August 11, 2021).
Vane, C. H., Kim, A. W., Moss-Hayes, V., Turner, G., Mills, K., Chenery, S. R., et al. (2020). Organic pollutants, heavy metals and toxicity in oil spill impacted salt marsh sediment cores, Staten Island, New York City, USA. Mar. Pollut. Bull. 151, 110721. doi:10.1016/j.marpolbul.2019.110721
Volkenborn, N., Polerecky, L., Wethey, D. S., and Woodin, S. A. (2010). Oscillatory porewater bioadvection in marine sediments induced by hydraulic activities of Arenicola marina. Limnol. Oceanogr. 55, 1231–1247. doi:10.4319/lo.2010.55.3.1231
Volkenborn, N., and Reise, K. (2007). Effects of Arenicola marina on polychaete functional diversity revealed by large-scale experimental lugworm exclusion. J. Sea Res. 57, 78–88. doi:10.1016/j.seares.2006.08.002
Washburn, T., Rhodes, A. C. E., and Montagna, P. A. (2016). Benthic taxa as potential indicators of a deep-sea oil spill. Ecol. Indic. 71, 587–597. doi:10.1016/j.ecolind.2016.07.045
Wells, G. P. (1966). The lugworm (Arenicola) — a study in adaptation. Neth. J. Sea Res. 3, 294–313. doi:10.1016/0077-7579(66)90016-0
Zabbey, N., and Uyi, H. (2014). Community responses of intertidal soft-bottom macrozoobenthos to oil pollution in a tropical mangrove ecosystem, Niger Delta, Nigeria. Mar. Pollut. Bull. 82, 167–174. doi:10.1016/j.marpolbul.2014.03.002
Keywords: infauna, seagrass, oil spill, polychaeta, neritina, capitellidae, arenicolidae, bio-indicating species
Citation: Berke SK, Dorgan KM, Kiskaddon E, Bell S, Gadeken K, Clemo WC, Keller EL and Caffray T (2022) Shallow infaunal responses to the Deepwater Horizon event: Implications for studying future oil spills. Front. Environ. Sci. 10:950458. doi: 10.3389/fenvs.2022.950458
Received: 22 May 2022; Accepted: 01 July 2022;
Published: 01 August 2022.
Edited by:
Charles William Martin, University of Florida, United StatesReviewed by:
David Hala, Texas A&M University at Galveston, United StatesCopyright © 2022 Berke, Dorgan, Kiskaddon, Bell, Gadeken, Clemo, Keller and Caffray. This is an open-access article distributed under the terms of the Creative Commons Attribution License (CC BY). The use, distribution or reproduction in other forums is permitted, provided the original author(s) and the copyright owner(s) are credited and that the original publication in this journal is cited, in accordance with accepted academic practice. No use, distribution or reproduction is permitted which does not comply with these terms.
*Correspondence: Sarah K. Berke, c2JlcmtlQHNpZW5hLmVkdQ==
Disclaimer: All claims expressed in this article are solely those of the authors and do not necessarily represent those of their affiliated organizations, or those of the publisher, the editors and the reviewers. Any product that may be evaluated in this article or claim that may be made by its manufacturer is not guaranteed or endorsed by the publisher.
Research integrity at Frontiers
Learn more about the work of our research integrity team to safeguard the quality of each article we publish.