- Shandong Institute of Sericulture, Shandong Academy of Agricultural Sciences, Yantai, China
The application of biochar and bio-organic fertilizers (BOFs) is effective for improving soil ecological environments. However, soil physicochemical properties and the microbiome diversity of rhizosphere soil after the application of different-sized particles of biochar together with BOF in saline–alkali land have not been thoroughly described. A field experiment was performed to investigate the effects of different-sized particles of apple shoot biochar (60, 30, and 10 mesh) together with BOF on soil bacteria (using Illumina high-throughput sequencing) and the physicochemical properties of Mesembryanthemum cordifolium L. f. grown on saline–alkali land. Results indicated that the combined application of BOF and 10–60 mesh biochar reduced the volumetric weight of soil by 14%–29%, respectively, and additionally decreased soil electrical conductivity, increased the aerial biomass of the M. cordifolium L. f. by over 30%, and notably improved soil water–holding capacity, with 60 mesh giving the best results; organic carbon (OC), organic matter (OM), total nitrogen (N), available phosphorus, alkaline nitrogen, total potassium (K), and total phosphorus (P) were all significantly increased by the addition of combined biochar and BOF; thereinto, field capacity, N, P, K, OC, and OM were positively correlated with the bacterial community structure of coapplied biochar and BOF. There were no significant differences in the richness of total bacteria among the treatments; Proteobacteria, Actinobacteria, and Chloroflexi accounted for >70% of the total bacteria in each treatment; Norank_f__Geminicoccaceae and Micromonospora were the dominant genera across the treatments. The findings suggested that plant growth, physicochemical properties, and community diversity of rhizosphere bacteria in saline–alkali land were significantly positively influenced by biochar 60 mesh plus BOF, followed by biochar 10 and 30 mesh plus BOF. This conclusion could facilitate the study of the ecological functions of biochar and BOF, as well as their interactions with salt-tolerant plants on saline–alkali soil, which can be used to provide exploration ideas for saline–alkali land improvement.
Introduction
Environmental stress is an area of profound scientific interest because of its detrimental consequences on agricultural productivity. Soil salinity is a global conundrum that severely impacts agricultural productivity and sustainability (Sofy et al., 2020). The inhibitory effect of salt on plants is mainly because of increased osmotic and ionic stress leading to reduced water availability, in addition to excess Na + disrupting cellular physiological and biochemical processes, thus affecting plant growth (Akhtar et al., 2015). High soil salt content, high pH, and poor soil structure not only severely affect plant growth but also affect microorganism community structure and diversity (Shi et al., 2019). More than 800 Mha of the world’s agricultural land is composed of saline soil, either based on salinity (397 Mha) or sodicity (434 Mha) (FAO, 2020), whereas there is approximately 8.11 × 107 ha of saline–alkali soil in China, which accounts for 8%–9% of the total land area (Zhao et al., 2014). The Yellow River delta is one of the three largest river deltas in China and is becoming an important region for agricultural development (Jing et al., 2019); however, crop production is limited by high soil salinity (Yang et al., 2020), and thus far, the utilization of this saline–alkali land has not been effectively managed. The development of strategies to make use of saline–alkali land will be crucial in addressing problems of insufficient cropland and meeting the challenge of providing food security for the projected global population of 9.3 billion people by 2050 (Shabala et al., 2015). Related research studies have shown that applications of biochar and organic fertilizers to saline–alkali soil are considered effective measures for reducing nitrogen (N) loss and improving soil environmental quality (Shi et al., 2019).
Biochar is a stable carbon-rich product obtained from the thermochemical decomposition of organic biomasses in an oxygen-limited condition and has attracted extensive attention because of its role in environmental improvement (Lehmann et al., 2011; Joseph et al., 2015; Kumar et al., 2021). As it generally has excellent properties, including high stability (Duan et al., 2021), large specific surface area (Luo et al., 2019; Shen et al., 2019), and abundant surface functional groups (Akdeniz, 2019; Chen et al., 2019), biochar is now increasingly used as a soil amendment (Bilias et al., 2021). To date, it has been reported to affect various parameters, including N (Borchard et al., 2014), total phosphorus (P) (Zhang et al., 2016), pH (Haque et al., 2021), soil organic matter (OM) content (Laskosky et al., 2020), microorganisms (Ren et al., 2020), organic carbon (OC) (Zhang et al., 2021), alter crop yield (Hossain et al., 2010; Sun et al., 2014; Ye et al., 2019), and water holding capacity (Yu et al., 2013), and alleviate abiotic stress (Lashari et al., 2014; Akhtar et al., 2015; Razzaghi et al., 2019). There is increasing evidence that the application of biochar to saline soils may also have positive effects on the physical, chemical, and biological properties of saline soils (Artiola et al., 2012; Egamberdieva et al., 2021).
Bio-organic fertilizers (BOFs), which combine beneficial functional microbes with a suitable substrate, are more effective than microbes added directly to the soil and are widely accepted as a promising biological tactic for suppressing soil-borne pathogens (Cao et al., 2011; Liu et al., 2015), promoting plant growth (Gopalakrishnan et al., 2013; Naher et al., 2021), and altering the composition of the rhizosphere (Rh) microbial community (Zhang et al., 2014; Zhao et al., 2018; Chen et al., 2021). Limited studies on the combined application of BOFs and other amendments suggest they may be effective strategies for remediating saline–alkaline soil properties, enhancing soil enzymatic and microbial activities closely related to the nutrient cycling and bioavailability, and improving soil productivity in a saline–alkali ecosystem (Liang et al., 2005; Vishnu et al., 2017; Lu et al., 2020). These studies support the incorporation of BOFs as a low-input effective agrotechnological approach to minimizing salt constraints. However, little information is available about the combination of biochar with BOFs on the Rh soil microecological environment in saline–alkali land, especially through field experiments.
Some plants can grow under salinity conditions because of physiological adaptations for salt tolerance; they are known as salt-resisting plants, salt-tolerant plants, or halophytes (Flowers et al., 1986). Salt-tolerant plants represent only 2% of terrestrial plant species, but they represent a wide diversity of plant forms (Glenn et al., 1999). Mesembryanthemum cordifolium L. f. has a physiology that offers relatively strong salt tolerance (Chen, 2017). To explore options for the biological improvement of saline lands, the salt-tolerant plant M. cordifolium, which grows more easily in saline lands, was selected. The objective of the present study was to obtain a broad overview of the combined effects of biochar and BOFs on plant growth, soil physicochemical properties, and the diversity of the bacterial community of the Rh of M. cordifolium. Our results provide new insights into this bacterial community and are a foundation for future studies.
Materials and methods
Biochar and bio-organic fertilizers preparation
BOF was purchased from Yangfeng Agricultural Technology Co., Ltd. (Weifang, China). The product includes humic aid, mushroom residue, corn residue, and soybean meal as the main raw materials, and Bacillus subtilis, Bacillus licheniformis, and Bacillus mucilaginous are added. Biochar was purchased from Taiyu Bioengineering Co., Ltd. (Qixia, China). The biochar sample was produced from apple shoots, and after charring at 450°C for 24 h, the biochar was milled to pass through 10, 30, and 60 mesh sieves, respectively, prior to further use. The pH of the biochar was 7.49,7.36, and 7.45; moreover, electrical conductivity (EC) was 0.357, 0.355, and 0.349 ms/cm for 10, 30, and 60 mesh, respectively.
Field experiment
The field experiments were performed at the Institute of Modern Agriculture on the Yellow River Delta, Shandong Academy of Agricultural Sciences, (118.37°N, 37.17°E), located in Dongying, China. The sampling field had been left fallow in past years, with no fertilizer application, and the soil was classified as silty clay (Zheng et al., 2018). The prepared biochar with different particle sizes (60, 30, and 10 mesh) and the BOF were incorporated into the selected plot at rates of 225 and 150 t/ha, respectively, hereafter referred to as CK (without biochar and BOF), S1 (10 mesh), S2 (30 mesh), and S3 (60 mesh). The biochar and BOF were spread on the field in March, and after the application, soil samples from each plot were taken in April before planting. M. cordifolium was chosen as the test plant in the field experiments, and the seedlings were transplanted into the field at the four-leaf stage in April; after planting, no other field management such as fertilization was performed except watering. Plant growth indicators were investigated in May and June. Three plants with medium growth were selected for each treatment, the aerial parts of the plant were harvested separately, and Rh soil was collected at the same time. Excess soil was removed from the root by shaking, and only tightly adherent soil remained for study (Egamberdieva et al., 2016). The Rh soils of three plants in each treatment from the topsoil (0–20 cm) were mixed evenly; impurities, including animal and plant residues from the soil samples, were removed with sterilized tweezers, and samples were divided into two parts. One part was immediately snap-frozen in liquid nitrogen and sent on dry ice to MajorBio for high-throughput sequencing for microbial diversity determination; the remaining part was air-dried, homogenized, and again sieved ( <2 mm) to remove any residue of silica sand or plant roots to determine the soil physicochemical properties.
Sample analysis
The fresh weight (FW) of the aerial biomass was weighed immediately after sampling, and soil physicochemical properties were analyzed after all soil samples were taken. Volumetric weight (VW) was determined by the ring knife method. Soil pH value according to a soil–water ratio of 1:5 was measured with a Shanghai Lei Magnetic Multi-parameter Water Quality Analyzer DZS-708. EC was measured in the soil at a depth of 10 cm using a FieldScout EC450 m. Maximum moisture capacity, capillary capacity, and field capacity (FC) were measured using the cutting ring method without compaction treatment (Wang et al., 2021). Total phosphate (P) content was measured using ICP-MS after microwave digestion (MARS5, CEM, United States) (0.1 g sample + 6 mL concentrated nitric acid). Available phosphorous (AP; extracted with 0.5 M NaHCO3) was determined using a segmented continuous-flow analyzer (Quaatro, Bran + Luebbe, Germany). Alkaline nitrogen (AN) and N (N) contents in the plants were determined using an elemental analyzer (FLASH-2000, Thermo Scientific, United States). Soil total potassium (K) was extracted with 1 mol/L ammonium acetate (pH 7.0) and determined using an Atomic Absorption Spectrophotometer AA4590. OM was measured using the potassium dichromate oxidation method. OC in soil was determined using an SSM-5000 A (Shimadzu, Japan) carbon analyzer (Zheng et al., 2018).
DNA extraction and PCR amplification
Genomic DNA of the microbial community was extracted from Rh soils using the E. Z.N.A.® soil DNA Kit (Omega Bio-tek, Norcross, GA, United States) according to the standard protocol. The quality of the extracted DNA was checked on a 1% agarose gel, and DNA concentration and purity were determined with a NanoDrop 2000 UV-vis spectrophotometer. The bacterial universal V3–V4 region of the 16S rRNA gene was amplified with the primers 338 F (50-ACTCCTACGGGAGGCAGCAG-30) and 806R (50-GGACTACHVGGGTATCTAAT-30). The PCR mixtures contained 4 μL 5 × TransStartFastPfu buffer, 2 μL of 2.5 mM dNTPs, 0.8 μL each primer (5 μM each), 0.4 μL TransStartFastPfu DNA polymerase, 10 ng template DNA, and ddH2O to 20 μL, and all the reactions were performed in triplicate. PCR cycling conditions comprised an initial denaturation at 95°C for 3 min, 27 cycles of denaturing at 95°C for 30 s, annealing at 55°C for 30 s, and extension at 72°C for 45 s, followed by a single extension at 72°C for 10 min and a continued hold at 4°C. The complete sequences generated in this study are available in the NCBI SRA database under accession number SRA data: PRJNA837444.
Illumina MiSeq and processing of sequencing data
The resulting PCR products were extracted from 2% agarose gels, purified using the AxyPrep DNA Gel Extraction Kit (Axygen Biosciences, Union City, CA, United States), and quantified using a Quantus™ Fluorometer (Promega Corporation, Madison, United States). Purified amplicons were pooled in equimolar ratios and paired-end sequenced by Majorbio Bio-Pharm Technology Co. Ltd. (Shanghai, China) using an Illumina MiSeq PE300 platform (Illumina Inc. San Diego, CA, United States) according to standard protocols. Raw 16S rRNA gene sequencing reads were demultiplexed, quality-filtered, and merged using FLASH v.1.2.7. Operational taxonomic units (OTUs) with 97% similarity cut-off were clustered using UPARSE v.7.1 (Edgar, 2013), and chimeric sequences were identified and removed. The taxonomy of each OTU representative sequence was analyzed by using RDP Classifier v.2.2 against the 16S rRNA database using a confidence threshold of 0.7 (Beckers et al., 2016).
Data analysis
The necessary computations were performed using Microsoft Excel spreadsheets, the figures were created using GraphPad Prism 9.3.1, and the statistical analyses of all parameters were performed using DPS Statistics 18.10 software (http://www.dpsw.cn). All analyses, including bacterial relative abundance, α-diversity, community composition, β-diversity, network structure, and functional analysis, were performed on the Majorbio Cloud Platform (www.majorbio.com). α-diversity including Chao, ACE, and Shannon and Simpson indices were calculated using Mothur software (v.1.30.2). Rarefaction curves were generated based on the observed species richness using Mothur at a 97% identity level. Venn and bar diagrams were generated using R script (v.3.3.1), and Circos was visualized using Circos-0.67-7 (http://circos.ca/). β-diversities were visualized using principal coordinates analysis (PCoA) based on the distance matrix, with Euclidean calculations. The distance-based redundancy analysis (db-RDA) was used to identify the relationships between microbial communities and soil properties. The heatmap was visualized by R (version 3.3.1) (pheatmap package). Spearman’s correlation was used to determine the relationships between gene copy number and soil properties using SPSS statistics 20. All experiments were conducted in triplicate. Data are presented as means with standard deviations. Differences between the means of different treatments were determined using the Duncan test at p < 0.05.
Results
Effects of biochar and bio-organic fertilizers amendment on the growth of Mesembryanthemum cordifolium
The aerial biomass of M. cordifolium was increased with the biochar and BOF amendment significantly (Figure 1). Treatment with biochar in combination with BOF resulted in biomasses that were significantly increased by 59.24% (30 mesh, p < 0.01) and 129.96% (60 mesh, p < 0.01) in May, compared with the control. Meanwhile, the application of 10 mesh biochar coapplied with BOF increased the FW by 28.69% in May, although the difference was not significant, and the biomasses of the three treatments were significantly improved by 38.79%, 74.75%, and 138.12% in June (p < 0.01) (Supplementary Table S1). The biochar and BOF treatment had a higher growth rate in June than in May, indicating that it also had a higher growth rate than the control.
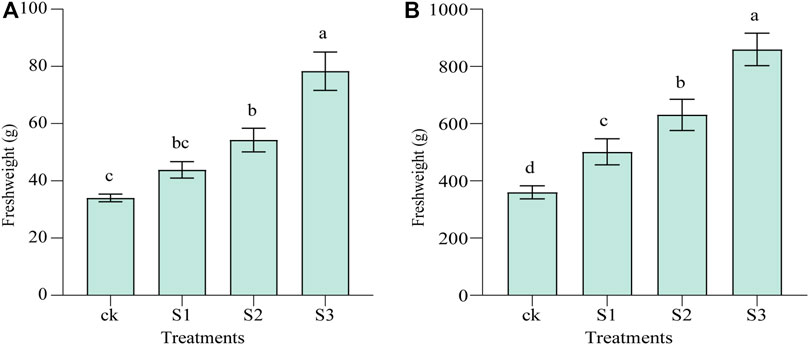
FIGURE 1. CK: soil without amendment; S1: soil amended with 10 mesh biochar and BOF at 10% (w/w), respectively; S2: soil amended with 30 mesh biochar and BOF at 10% (w/w), respectively; S3: soil amended with 60 mesh biochar and BOF at 10% (w/w), respectively; Error bars represent standard errors of the means (n = 3). Different uppercase and lowercase letters indicate significant differences among treatments (p < 0.01) and (p < 0.05), respectively. (A) is in May, and (B) is in June.
Soil physicochemical properties
Figure 2 and Supplementary Table S2 show the effect of biochar and BOF amendment on soil moisture characteristics in the saline–alkali land. The maximum moisture capacity and capillary capacity of the soils of all three treatments were improved significantly by 11.57% (10 mesh, p < 0.05), 23.96% (30 mesh, p < 0.01), and 48.13% (60 mesh, p < 0.01) and 19.6% (10 mesh, p < 0.05), 49.35% (30 mesh, p < 0.01), 87.05% (60 mesh, p < 0.01), respectively, compared with the control. When applying biochar together with BOF, this significantly increased FC by 55.21% (30 mesh, p < 0.01) and 74.86% (60 mesh, p < 0.01), respectively, whereas 10 mesh biochar in combination with BOF increased FC by 28.91%, although the difference was not significant.
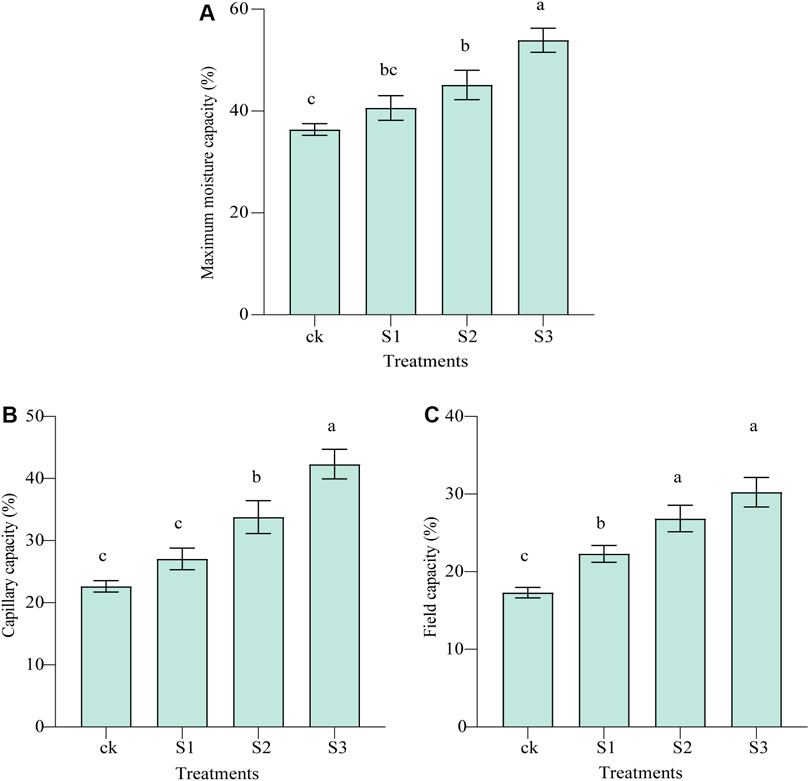
FIGURE 2. Effect of co-application of biochar and bio-organic fertilizers (BOF) on WHC of soil. (A) maximum moisture capacity, (B) capillary capacity, and (C) field capacity.
Table 1 illustrates the effect of biochar and BOF on soil’s physical and chemical properties. Although the effect of the co-application of biochar and BOF, regardless of particle size, was not significant in terms of pH, the EC of biochar in combination with BOF was significantly reduced by 34.57% (30 mesh, p < 0.05) and 42.23% (60 mesh, p < 0.05), respectively, compared with the control. Furthermore, the treatment of 10 mesh biochar and BOF also decreased EC by 24.7%, although this was not significant. Likewise, there was a decreasing trend in VW with increasing biochar particle size; the VWs of all three treatments were significantly decreased by 13.8% (10 mesh), 19.45% (30 mesh), and 29.03% (60 mesh), (p < 0.01) (Supplementary Table S3), compared with the control. There was a similar increase in the content of AP and K in soils, the co-application of 30 mesh biochar and BOF produced the highest content, followed by 10 mesh and 60 mesh (p < 0.01), compared with the CK treatment. The highest AN content was also given by the 30 mesh biochar together with BOF, followed by 10 mesh, and then by 60 mesh; all of them reached a very significant level (p < 0.01). The application of biochar together with BOF significantly promoted the content of TN and OC, with the most improvement being produced by 60 mesh and the least improvement produced by 10 mesh; all treatments reached a very significant level (p < 0.01). The content of OM in soil increased with increasing biochar particle size and was significantly higher than that of the control (p < 0.01).
Sequence data and α-diversity index analysis
A total of 622 127 high-quality sequences remained and were investigated after read-quality filtering. The total number of bases was 257 754 588, and the average read length was 414.34 bp. Rarefaction curves (Supplementary Figure S1), combined with the estimated coverage values (Supplementary Table S4), suggested that the data were sufficiently large to capture most of the bacterial diversity in the samples. The number of OTUs obtained was the highest in the CK treatment, whereas the lowest number of OTUs was found in the 30 mesh biochar coapplied with BOF.
The number of common and unique bacterial OTUs in the different samples is presented in Venn diagrams (Figure 3). A total of 7,431 OTUs were detected across all libraries with 2,408 OTUs common to all samples. The shared bacterial OTUs mainly belonged to the Proteobacteria (622), Actinobacteria (378), Chloroflexi (350), Acidobacteria (159), Gemmatimonadetes (117), Bacteroidetes (109), and Firmicutes (107) at the phylum level. For unique OTUs, the number obtained in CK (1,549) was the largest, and much higher than the other three treatments of biochar and BOF (377 for 10 mesh, 323 for 30 mesh, and 396 for 60 mesh), regardless of particle size.
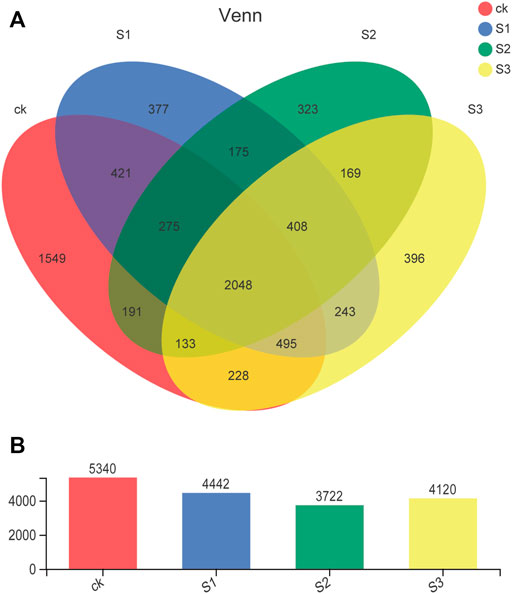
FIGURE 3. Venn diagrams of the number of OTUs obtained in different treatments. Values represent the number of OTUs. (A) Venn diagram and (B) average total OTUs of different treatments.
Microbial taxonomic analysis
The obtained 7,431 OTUs were classified into 37 phyla, 102 classes, 286 orders, 542 families, 1,167 genera, and 2,448 species. The bacterial composition and relative abundances varied across different samples. The diversity of bacterial communities in different samples at the phylum level is shown in Figure 4. The dominant bacterial phyla were Proteobacteria and Actinobacteria across all samples, followed by Chloroflexi, and three phyla occupied over 70% of the total, whereas there was no significant difference between CK and the other treatments, regardless of the biochar particle size. The relative abundance of Acidobacteria and Gemmatimonadetes dropped notably from 44% (CK) to 10% (30 mesh) and from 37% (CK) to 16% (30 mesh), respectively (p < 0.05).
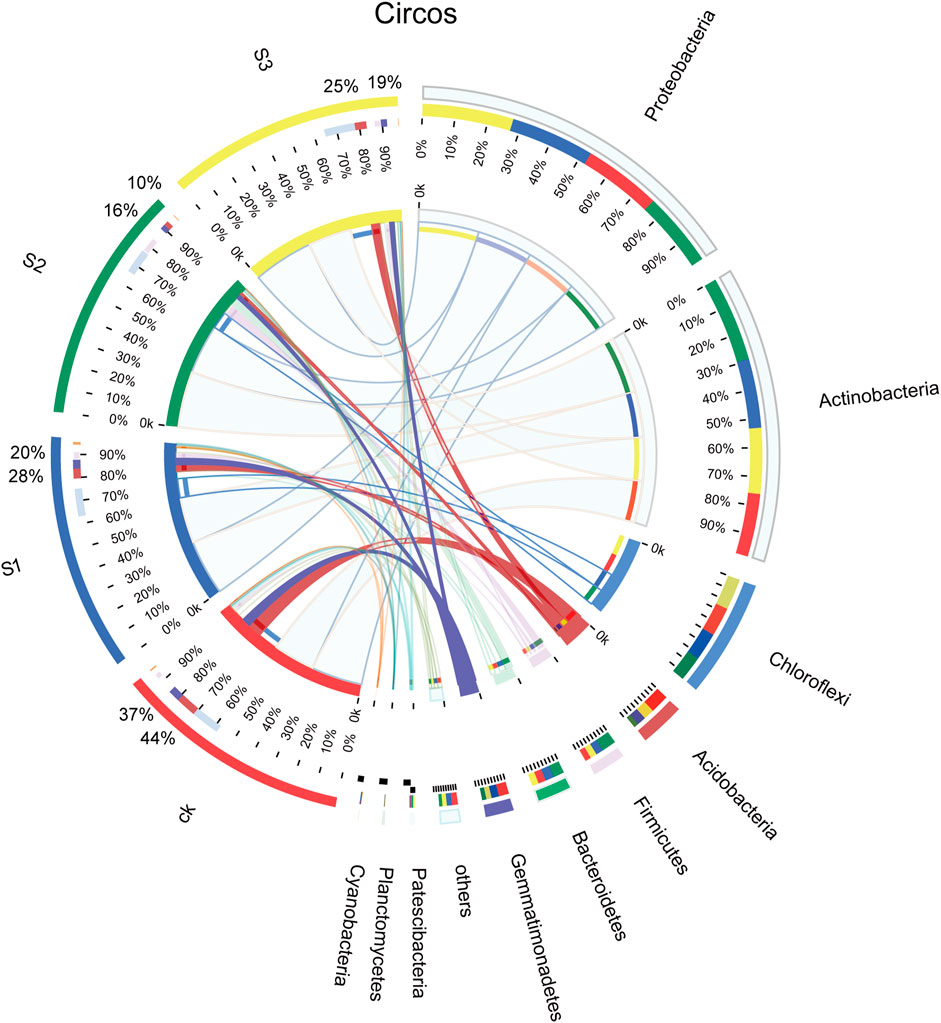
FIGURE 4. Relative abundance of bacteria from different communities at the phylum level. The purple-red band is Acidobacteria, and the blue-purple band is Gemmatimonadetes.
In clustering of the top 40 genera, as shown in Figure 5, the genera distributions differed greatly across different samples. These 40 bacterial genera belonged to seven phyla, namely, Actinobacteria (16 genera), Proteobacteria (11), Chloroflexi (6), Acidobacteria (3), Gemmatimonadetes (2), Bacteroidetes (1), and Firmicutes (1). Norank_f__Geminicoccaceae was the predominant genus in CK, and biochar in combination with BOF (10 mesh and 60 mesh), whereas Micromonospora was the most abundant genus in the 30 mesh biochar co-application of BOF. The co-application of biochar and BOF increased the abundances of Micromonospora, Bacillus, norank_f_A4b, Nocardioides, norank_o_Microtrichales, norank_o_SBR1031, Actinomadura, Marinobacter, norank_c_Gitt-GS-136, Pelagibius, unclassified_c_AlphaProteobacteria, and Rhodococcus, etc. by different degrees, regardless of particle size. However, the abundances of Arthrobacter, norank_c_Subgroup_6, norank_f_JG30-KF-CM45, norank_f_67-14, and norank_o_Gaiellales, etc., decreased compared with CK, regardless of particle size.
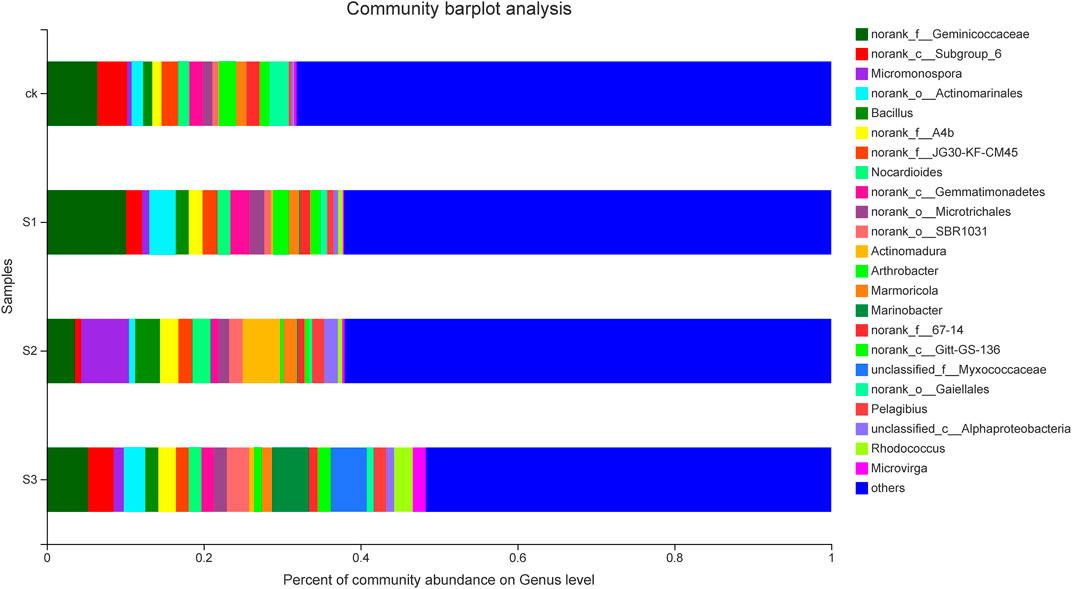
FIGURE 5. Relative abundance of the predominant genera (top 40) in each sample. Taxa with an abundance <0.01 are included in “others.” The x-axis represents the relative abundance of all communities, and the y-axis represents different communities.
β-diversity analysis
To further compare the relationship of bacterial populations among the samples, PCoA based on Euclidean distances with arithmetic mean clustering was conducted using phylum data. This analysis revealed the main variations in bacterial community composition and abundance across the treatments. The PCoA results graphically demonstrated that biochar particles or biochar co-application with BOF were strong factors accounting for the observed variations in the composition of the bacterial community, in which samples of 60 mesh biochar together with BOF were placed at a higher PCoA 1 value (46.3%), whereas samples of 30 mesh biochar together with BOF gave a higher PCoA 2 value (24.04%; Figure 6). PCoA also showed that three biochar treatments together with BOF clustered separately from control treatments. This indicated that the addition of biochar together with BOF shifted the bacteria communities.
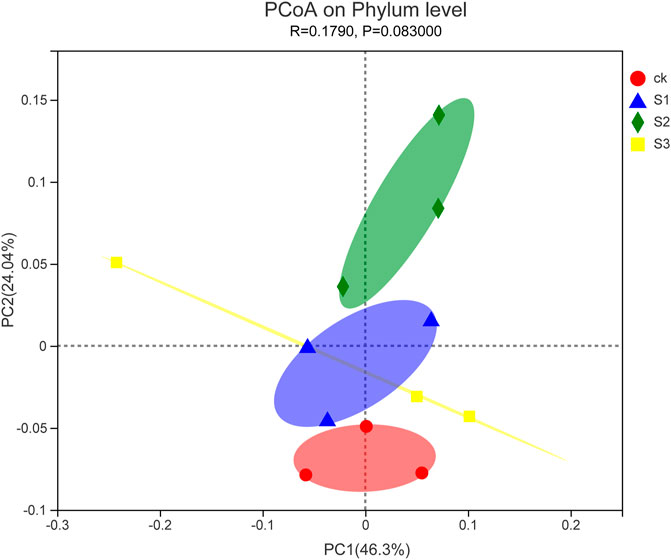
FIGURE 6. PCoA plot of the relationship between samples based on similarity in the community composition of bacterial OTUs. Two first components (PCoA 1 and PCoA 2) were plotted and represent 70.34% of the variation.
Relationship of environmental parameters with microbial communities
The db-RDA results revealed the relationship of bacterial community composition with VW, pH, EC, FC, AP, AN, N, P, K, OC, and OM (Figure 7). G1 was saline–alkali soil without biochar, BOF, and plant. For the microbial community composition, the first axis accounted for 18.57% of overall variations, whereas the second one occupied 15.04%. The RDA tests showed that the bacterial community structure of saline–alkali soil was positively correlated with the VW and EC of soil, and the bacterial community structure of coapplied biochar and BOF were positively correlated with FC, AN, N, P, K, OC, and OM.
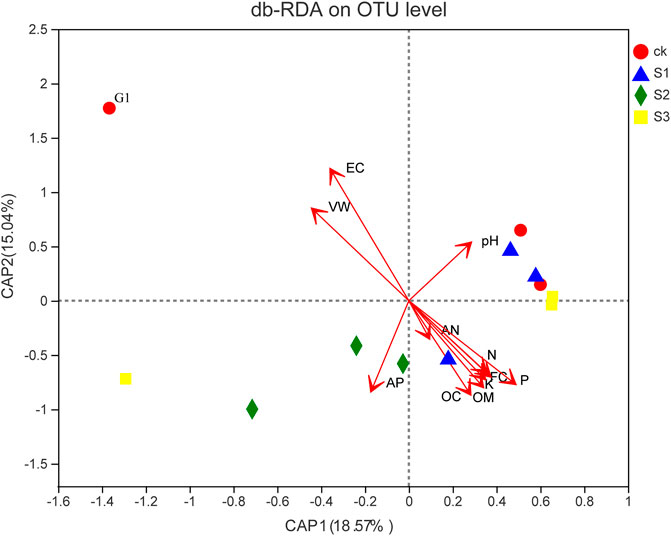
FIGURE 7. db-RDA of the relationship between soil characteristics and soil bacterial community. Two first components (PCoA 1 and PCoA 2) were plotted and represent 23.61% of the variation.
To further compare the relationship of the bacterial community with physicochemical properties, the top 30 most abundant genera and environmental parameters were subject to Spearman’s correlation analysis (Figure 8). The results in Supplementary Table S6 showed 20 genera, which were moderately or strongly correlated with physical and chemical factors. VW was negatively correlated with Rhodococcus and norank_f_D05-2 (p < 0.05); pH rise played an important role in Micromonospora, Bacillus, Marinobacter, and unclassified_c_AlphaProteobacteria (p < 0.05) reduction; EC had a negative impact on the relative abundance of unclassified_c_AlphaProteobacteria (p < 0.05); and FC appeared to be responsible for reduced norank_o_Gaiellales and norank_f_Gemmatimonadaceae abundance (p < 0.05). Norank_o_Gaiellales was negatively correlated with AP (p < 0.01), AN (p < 0.01), N (p < 0.05), K (p < 0.01), and OM (p < 0.05), whereas it was positively correlated with pH (p < 0.05). Norank_f_Gemmatimonadaceae decreased with AP (p < 0.05), N (p < 0.05), and K (p < 0.01) increased; in addition, norank_c_Subgroup_6 tended to decrease with the increase of AP (p < 0.05). Bacillus was significantly positively correlated with AP (p < 0.01), AN (p < 0.01), and K (p < 0.05); however, Micromonospora was positively affected by AP and K (p < 0.05). Marinobacter showed a positive correlation with AP (p < 0.05), and Pelagibius increased with increases in AP, AN, and K (p < 0.05). Rhodococcus showed an increasing trend with increasing AN and OC (p < 0.05), and norank_f_D05-2 was also positively correlated with AN, N, P, and K (p < 0.05).
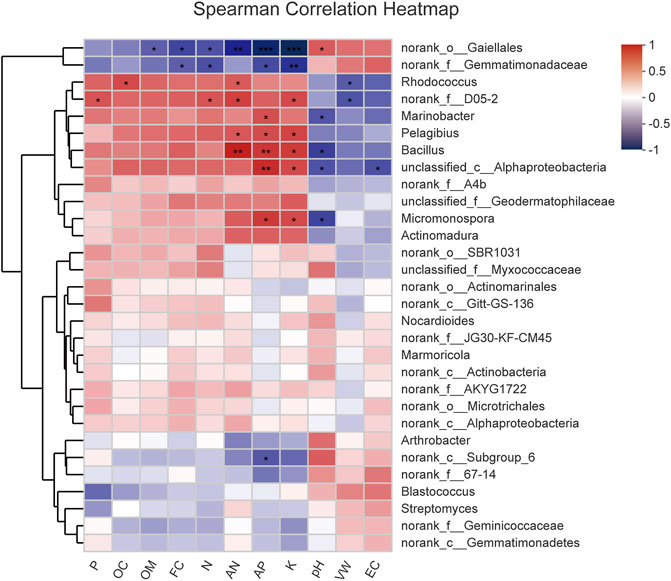
FIGURE 8. Heatmap of correlation of the top 30 abundant genera and environmental parameters. *Correlation is significant at the 0.05 level (two-tailed). **Correlation is significant at the 0.01 level (two-tailed).
Discussion
Owing to the highly heterogeneous properties of biochar, soil conditions, experiment designs, and environmental conditions, the responses of plants to biochar amendment of the soil vary enormously (You et al., 2021). For instance, an economic assessment carried out by Keske et al. (2019) was able to determine that when biochar, created from black spruce, was applied to beets at 10,000 kg/ha, the resultant beet yields increased from 2,900 to 11,004 kg/ha. Another report indicated that the biomasses of ryegrass, Sedum lineare, and cucumber increased by 6.9%–88.9% with the addition of sludge biochar to the natural soil (Chen et al., 2018). Naher et al. (2021) reported that BOF can significantly improve the rice yield in farmers’ fields. Biochar in combination with fertilizer also showed a large effect by increasing the yield of cherry tomato production from 776 to 2,514 g (Hossain et al., 2010); however, Kammann et al. (2015) reported that the addition of 2% (w/w) untreated biochar of Chenopodium quinoa decreased the biomass to 60% of the control and biomass yield increased up to 305% in a sandy-poor soil amended with 2% (w/w) co-composted biochar. Similar to most studies, the results of our study showed that the co-application of biochar with BOF significantly promoted the biomass of aerial parts. By contrast, Schmidt et al. (2014) found no significant effects on vine growth under WBC treatment in alkaline or temperate soils with poor fertility, this may be because of the low application of biochar. We speculate that this might be because of the combined effects of biochar and BOF, which together stimulated plant growth. The high increase in growth rates in this study may be because of the low quality of the saline–alkali soil as the control, resulting in an exaggerated growth rate in the treatments.
It is well known that biochar improves WHC through surface area and porosity; with the addition of 1%–100% biochar, there is an increase from approximately 5% to 1,612.5% WHC (Yu et al., 2013; Razzaghi et al., 2019; Allohverdi et al., 2021). The results of the current study are concurrent with those of Verheijen et al. (2019) and Yu et al. (2013). Biochar can alleviate soil compaction by decreasing bulk density, which increases porosity and accentuates favorable soil processes (Laird et al., 2010; Karhu et al., 2011; Kizito et al., 2019). Liu et al. (2017) reported that there were no significant changes in bulk density for fine, medium, and coarse biochar (2%)-sand mixtures compared with sand. The soil in this study was silty clay and was heavily compacted, so the addition of porous biochar and granular BOF greatly improved soil structure, increased soil permeability, reduced VW, and enhanced WHC, and this may also be an important reason for promoting plant growth. Biochar can also have a variety of effects on soil pH, the degree to which is often dependent on the feedstock and production conditions (Allohverdi et al., 2021). The addition of biochar and BOF in this study did not change the pH of the saline–alkali soil, perhaps because the low temperature used to produce the biochar resulted in the low pH of biochar used in the current study; it could also be related to the already neutral-alkaline pH of the soil, or it might be because of the secretion of organic acids (e.g., citric, oxalic, and malic acids) from the stimulated roots of plants under fertilizer treatment (Hinsinger et al., 2003; You et al., 2021). Many studies have demonstrated that biochar increases soil EC (Hossain et al., 2010; Bilias et al., 2021). On the other hand, Egamberdieva et al. (2021) reported that the application of biochar has little effect on EC when applied alone but produces a notable improvement when applied together with fertilizer (You et al., 2021). However, Lashari et al. (2014) reported a significant reduction in EC by 28% and 41% with the addition of biochar–manure compost in saline soil under maize in field experiments, which agreed with our results. The decrease in EC may be because of the application of organic fertilizers, and different biochar raw materials or preparation methods. The application of biochar can increase soil nutrients (N, P, K, OM, etc.) to varying degrees, because of its ability to directly provide nutrients for plant uptake, to absorb and retain soil nutrients (Borchard et al., 2014; Zhang et al., 2016; Laskosky et al., 2020; Allohverdi et al., 2021), and to improve microbial habitat (Chen et al., 2017). In the current study, BOF was added, so soil nutrients were notably improved; moreover, fine and medium biochar gave significantly better results than coarse biochar, which agrees with Chen et al. (2017); in the meantime, the AN and AP of fine and medium biochar were higher compared with coarse biochar, whereas the N and P were the opposite, which may be because of the conversion of N and P into AN and AP by more microorganisms residing in the fine biochar (Sarfraz et al., 2020; Allohverdi et al., 2021).
Many aspects of biochar affect microbial populations, such as feedstock, pyrolysis conditions, particle size, and soil properties. Through sorption, various organic compounds that are bonded to biochar structures can be used by plants (Joseph et al., 2010), and the addition of OM generally helps microbial species (Vishnu et al., 2017; Lu et al., 2020). There was no significant difference in bacterial richness among the combined treatments of biochar and BOF, but the composition and structure changed. The unique OTUs of CK were much higher than those for the other three treatments of biochar and BOF, as well as the total OTUs, suggesting that the addition of biochar and BOF greatly altered the composition of bacterial communities. The relative abundance of Acidobacteria and Gemmatimonadetes dropped notably compared to CK. Bacteroidetes and Proteobacteria are reported to account for >50% as the predominant phyla in Rh soil of Mesembryanthemum crystallinum L. (You et al., 2021); however, Proteobacteria and Actinobacteria were the predominant phyla in the current study, similar to Sarfraz et al. (2020), although only one of the top ten phyla differed from the results of You et al. (2021). This may be because of the different environments and experimental protocols. RDA is a method used to identify the relationship between environment, taxa, and vegetation. The RDA results showed that bacterial relative abundance after the addition of biochar and BOF was positively correlated with N, P, K, AN, OC, OM, and FC; this result was consistent with many previous studies (Lu et al., 2020; Ren et al., 2020; Gao et al., 2021), and planting salt-tolerant plants also changed the microbial structure.
Biochar amendment increased the relative abundance of Micromonospora, which is known to have plant growth–promoting traits including nitrogen fixation and the inhibition of plant pathogens (Li et al., 2022); our results were in agreement with this study, for being the dominant genus in 30 mesh. Micromonospora not only presented higher relative abundance in biochar and BOF treatments compared with CK but also showed a strong positive correlation with AN, AP, and K. Bacillus spp. are commonly found in Rh soil and have been cited in literature to show plant growth–enhancing effects (Saxena et al., 2013). Compound Bacillus biofertilizer, an atoxic multifunctional fertilizer, could be used in inoculations with functional bacteria to enhance soil fertility and quality and reduce heavy metal toxicity (Vassilev et al., 2015). In the current study, the relative abundances of Bacillus in biochar together with BOF treatments were >40% higher than the control, and there was a significant difference in medium-sized particles (p < 0.05) (Supplementary Table S5, Supplementary Figure S2). This increase was not too great, because BOF itself contains Bacillus, which may be because of the saline–alkali soil environment hindering the reproduction of Bacillus. Nonetheless, Bacillus may still play an important role in improving the properties of saline–alkali soils because of its strong correlation with AN, AP, K, and pH and moderate correlation with other factors. In addition, Rhodococcus is frequently studied because it possesses multiple functions and is useful for its environmental and industrial biotechnology applications (Krivoruchko et al., 2019). Ren et al. (2020) also reported a low abundance of Rhodococcus in the co-application of biochar and plant growth–promoting rhizobacteria. In the current study, the relative abundance of Rhodococcus in co-applications of biochar and BOF was much higher than the control and was moderately correlated with all factors except pH and K; thus, it should also play an important role in improving soil properties. To date, little is known about Ilumatobacter. Zhao et al. (2019) reported that pH, NH4+-N, OM, and OC have strong positive relationships with the abundance of Ilumatobacter and suggested that Ilumatobacter may catalyze the synthesis of polyketides, which are highly effective antibiotics. In the current study, the relative abundances of Ilumatobacter were significantly increased with the co-application of biochar and BOF compared with the control. Furthermore, Ilumatobacter showed a strong positive correlation with AN (p < 0.05) and a moderate positive correlation with FC, AP, P, K, and OM, findings that agree with Zhao et al. (2019), On the other hand, the correlation with pH was negative, unlike that of Zhao et al. (2019). Han et al. (2017) reported that biochar treatment could reduce the relative abundance of Ilumatobacter in cotton soils. These differences may be because of the environments, but this needs further study in the future. The significant negative correlation between Norank_f_norank_o_Gaiellales and K was very strong, which disagrees with Gu et al. (2020), who reported that Norank_f_norank_o_Gaiellales is significantly correlated with K. The different results in the current study may have been caused by the biochar content, mixed matrix, and biochar feedstock. The relative abundances of many functional taxa related to plant growth and soil microecological environment changed, which draws increasing attention; interaction between the Rh and microorganisms in the soil environment deserves more attention in the future.
Although the interest in biochar as a soil amendment increasing greatly, there are limited reports on the effects of biochar particle size on soil and plants. Liu et al. (2017) considered that biochar particle size affects soil water storage through changing interpores and by adding intrapores. Li et al. (2019) speculated that the effect of biochar particle size on soil and water loss might because of the changes to Ksat and aggregate content of biochar. Chen et al. (2017) thought that fine particle biochar may additionally produce a better habitat for microorganisms compared to the other particle sizes. However, little information on field experiments regarding the biochar together with BOF on rhizosphere soil microecological environment in saline–alkali land is available. We aimed to select an optimal application protocol to provide a potentially valuable strategy for saline land reclamation. Papers on biochar particle size confirmed that fine biochar is superior to coarse biochar (Chen et al., 2017; Liu et al., 2017; Li et al., 2019; Alghamdi et al., 2020), which is also confirmed in our study. 60- and 30-mesh biochar together with BOF performed better in saline–alkali land reclamation, whether it is in terms of soil physicochemical properties or microbial composition and structure. This may be because of increased microporosity as a result of the larger internal surfaces and the porous structure of the biochar particles; the increasing interpores and intrapores of fine biochar lead to the flourishing of microbes in the rhizosphere, improve the physical and chemical properties of soil, and then promote the retention and transformation of soil nutrients. However, a long-term study of different biochar particle co-application with BOF in saline–alkali land needs to be pursued further for a more comprehensive understanding.
Conclusion
Amendment with different particle sizes of biochar and BOF significantly improved the aerial biomass of salt-tolerant M. cordifolium in saline–alkali soil, with a particle size of 60 mesh together with BOF giving the best results. In addition, the co-application of biochar and BOF significantly reduced VW and EC, improved soil physicochemical properties, and increased nutrients of saline–alkali soils. Nevertheless, there was no significant difference between CK and biochar–BOF addition in bacterial richness, but the composition and structure changed greatly. The bacterial community structure of coapplied biochar and BOF were positively correlated with FC, N, P, K, OC, and OM. Overall, the combined application of biochar and BOF can improve the rhizosphere soil microecological environment on saline–alkali land; 60-mesh biochar–BOF combined with salt-tolerant plants can be an effective way of reclaiming saline–alkali land. The results of the current study provide crucial information and further scientific guidance regarding the co-application of biochar and BOF to salt-tolerant plants for the sustainable utilization of saline–alkali soils.
Data availability statement
The datasets presented in this study can be found in online repositories. The names of the repository/repositories and accession number(s) can be found at: https://www.ncbi.nlm.nih.gov/sra/PRJNA837444.
Author contributions
Y-YG contributed to the conception of the study and wrote the manuscript; C-JC and ML also contributed to the conception of the study; and H-YZ, X-YL, and RF performed the experiments and data analyses. All authors contributed to the article and approved the submitted version.
Funding
This work was supported by the Sericultural Industry Technical System of Shandong Province (Grant No. SDAIT-18-09).
Acknowledgments
We would like to thank Charlesworth Author Services for English language editing.
Conflict of interest
The authors declare that the research was conducted in the absence of any commercial or financial relationships that could be construed as potential conflicts of interest.
Publisher’s note
All claims expressed in this article are solely those of the authors and do not necessarily represent those of their affiliated organizations or those of the publisher, the editors, and the reviewers. Any product that may be evaluated in this article, or claim that may be made by its manufacturer, is not guaranteed or endorsed by the publisher.
Supplementary material
The Supplementary Material for this article can be found online at: https://www.frontiersin.org/articles/10.3389/fenvs.2022.949190/full#supplementary-material
CK: soil without amendment; S1: soil amended with 10 mesh biochar and BOF at 10% (w/w), respectively; S2: soil amended with 30 mesh biochar and BOF at 10% (w/w), respectively; S3: soil amended with 60 mesh biochar and BOF at 10% (w/w), respectively; Error bars represent standard errors of the means (n = 3). Different uppercase and lowercase letters indicate significant differences among treatments (p < 0.01) and (p < 0.05), respectively. (a) is in May, and (b) is in June.
References
Akdeniz, N. (2019). A systematic review of biochar use in animal waste composting. Waste Manag. 88, 291–300. doi:10.1016/j.wasman.2019.03.054
Akhtar, S. S., Andersen, M. N., and Liu, F. L. (2015). Residual effects of biochar on improving growth, physiology and yield of wheat under salt stress. Agric. Water Manag. 158, 61–68. doi:10.1016/j.agwat.2015.04.010
Allohverdi, T., Mohanty, A. K., Roy, P., and Misra, M. (2021). A review on current status of biochar uses in agriculture. Molecules 26(18), 5584. doi:10.3390/molecules26185584
Artiola, J. F., Rasmussen, C., and Freitas, R. (2012). Effects of a biochar-amended alkaline soil on the growth of romaine lettuce and bermudagrass. Soil Sci. 177 (9), 561–570. doi:10.1097/ss.0b013e31826ba908
Beckers, B., Beeck, M. O. D., Thijs, S., Truyens, S., Weyens, N., Boerjan, W., et al. (2016). Performance of 16s rDNA primer pairs in the study of rhizophere and endosphere bacterial microbiomes in metabarcoding studies. Front. Microbiol. 7, 1–15. doi:10.3389/fmicb.2016.00650
Bilias, F., Nikoli, T., Kalderis, D., and Gasparatos, D. (2021). Towards a soil remediation strategy using biochar: Effects on soil chemical properties and bioavailability of potentially toxic elements. Toxics 9 (184), 184. doi:10.3390/toxics9080184
Borchard, N., Siemens, J., Ladd, B., Moller, A., and Amelung, W. (2014). Application of biochars to sandy and silty soil failed to increase maize yield under common agricultural practice. Soil Tillage Res. 144, 184–194. doi:10.1016/j.still.2014.07.016
Cao, Y., Zhang, Z. H., Ling, N., Yuan, Y. J., Zheng, X. Y., Shen, B., et al. (2011). Bacillus subtilis SQR 9 can control Fusarium wilt in cucumber by colonizing plant roots. Biol. Fertil. Soils 47 (5), 495–506. doi:10.1007/s00374-011-0556-2
Chen, H. J., Zhao, J. M., Jiang, J., Zhao, Z. G., Guan, Z. Y., Chen, S. M., et al. (2021). Effects of inorganic, organic and bio-organic fertilizer on growth, rhizosphere soil microflora and soil function sustainability in chrysanthemum monoculture. Agriculture 11, 1214. doi:10.3390/agriculture11121214
Chen, H. M., Ma, J. Y., Wei, J. X., Gong, X., Yu, X. C., Guo, H., et al. (2018). Biochar increases plant growth and alters microbial communities via regulating the moisture and temperature of green roof substrates. Sci. Total Environ. 635 (SEP.1), 333–342. doi:10.1016/j.scitotenv.2018.04.127
Chen, H. Y., Awasthi, S. K., Liu, T., Duan, Y. M., Ren, X. N., Zhang, Z. Q., et al. (2019). Effects of microbial culture and chicken manure biochar on compost maturity and greenhouse gas emissions during chicken manure composting. J. Hazard. Mat. 389, 121908. doi:10.1016/j.jhazmat.2019.121908
Chen, J. H., Li, S. H., Liang, C. F., Xu, Q. F., Li, Y. C., Qin, H., et al. (2017). Response of microbial community structure and function to short-term biochar amendment in an intensively managed bamboo (Phyllostachys praecox) plantation soil: Effect of particle size and addition rate. Sci. Total Environ. 574, 24–33. doi:10.1016/j.scitotenv.2016.08.190
Chen, L. (2017). Physiological response of salt stress on Mesembryanthemum cordifolium L.f. J. Guangxi Agric. 32 (5), 17–21.
Duan, M. L., Liu, G. H., Zhou, B. B., Chen, X. P., Wang, Q. J., Zhu, H. Y., et al. (2021). Effects of modified biochar on water and salt distribution and water-stable macro-aggregates in saline-alkaline soil. J. Soils Sediments 21 (3), 2192–2202. doi:10.1007/s11368-021-02913-2
Edgar, R. C. (2013). Uparse: Highly accurate OTU sequences from microbial amplicon reads. Nat. Methods 10 (10), 996–998. doi:10.1038/nmeth.2604
Egamberdieva, D., Ma, H., Alaylar, B., Zoghi, Z., Kistaubayeve, A., Wirth, S., et al. (2021). Biochar amendments improve licorice (Glycyrrhiza uralensis fisch.) growth and nutrient uptake under salt stress, Plants (Basel).,. Plants 10. 2135, doi:10.3390/plants10102135
Egamberdieva, D., Wirth, S., Behrendt, U., Abd_Allah, E. F., and Berg, G. (2016). Biochar treatment resulted in a combined effect on soybean growth promotion and a shift in plant growth promoting rhizobacteria. Front. Microbiol. 7 (209), 209. doi:10.3389/fmicb.2016.00209
Faostat, F. A. O. (2020). Available online: http://www.fao.org/faostat/en/#data/QC (accessed on February 20, 2020).
Flowers, T. J., Hajibagheri, M. A., and Clipson, N. J. W. (1986). Q. Rev. Biol. 6, 313–337. doi:10.1086/415032
Gao, W. H., Gao, K., Guo, Z. H., Liu, Y., Jiang, L., Liu, C., et al. (2021). Different responses of soil bacterial and fungal communities to 3 Years of biochar amendment in an alkaline soybean soil. Front. Microbiol. 12, 630418. 3389/fmicb.2021.630418. doi:10.3389/fmicb.2021.630418
Glenn, E. P., Brown, J. J., and Blumwald, E. (1999). Salt tolerance and crop potential of halophytes. CRC. Crit. Rev. Plant Sci. 18, 227–255. doi:10.1080/07352689991309207
Gopalakrishnan, S., Srinivas, V., Vidya, M. S., and Rathore, A. (2013). Plant growth-promoting activities of Streptomyces spp. in sorghum and rice. SpringerPlus 2 (1), 574. doi:10.1186/2193-1801-2-574
Gu, Y. Z., Wang, Y. X., Wang, P. Z., Wang, C. N., Ma, J., Yang, X. F., et al. (2020). Study on the diversity of fungal and bacterial communities in continuous cropping fields of Chinese chives (Allium tuberosum). Biomed. Res. Int. 3589758, 1–14. doi:10.1155/2020/3589758
Han, G. M., Lan, J. Y., Chen, Q. Q., Yu, C., and Bie, S. (2017). Response of soil microbial community to application of biochar in cotton soils with different continuous cropping years. Sci. Rep. 7 (1), 10184. doi:10.1038/s41598-017-10427-6
Haque, A. N. A., Uddin, M. K., Sulaiman, M. F., Amin, A. M., Hossain, M., Solaiman, Z. M., et al. (2021). Biochar with alternate wetting and drying irrigation: A potential technique for paddy soil management. Agriculture 11, 367. doi:10.3390/agriculture11040367
Hinsinger, P., Plassard, C., Tang, C., and Jaillard, B. (2003). Origins of root-mediated pH changes in the rhizosphere and their responses to environmental constraints: A review. Plant Soil 248, 43–59. doi:10.1023/A:1022371130939
Hossain, M. K., Strezov, V., Chan, K. Y., and Nelson, P. F. (2010). Agronomic properties of wastewater sludge biochar and bioavailability of metals in production of cherry tomato (Lycopersicon esculentum). Chemosphere 78 (9), 1167–1171. doi:10.1016/j.chemosphere.2010.01.009
Jing, C. L., Xu, Z. C., Zou, P., Tang, Q., Li, Y. Q., You, X. W., et al. (2019). Coastal halophytes alter properties and microbial community structure of the saline soils in the Yellow River Delta, China. Appl. Soil Ecol. 134, 1–7. doi:10.1016/j.apsoil.2018.10.009
Joseph, S., Anawar, H. M., Storer, P., Blackwell, P., Chia, C., Lin, Y., et al. (2015). Effects of enriched biochars containing magnetic iron nanoparticles on mycorrhizal colonisation, plant growth, nutrient uptake and soil quality improvement. Pedosphere 25 (5), 749–760. doi:10.1016/S1002-0160(15)30056-4
Joseph, S. D., Camps-Arbestain, M., Lin, Y., Munroe, P., Chia, C. H., Hook, J., et al. (2010). An investigation into the reactions of biochar in soil. Soil Res. 48 (7), 501. doi:10.1071/SR10009
Kammann, C. I., Schmidt, H. P., Messerschmidt, N., Linsel, S., Steffens, D., Muller, C., et al. (2015). Plant growth improvement mediated by nitrate capturein co-composted biochar. Sci. Rep. 5, 11080. doi:10.1038/srep11080
Karhu, K., Mattila, T., Bergstrm, I., and Regina, K. (2011). Biochar addition to agricultural soil increased CH4 uptake and water holding capacity- Results from a short-term pilot field study. Agric. Ecosyst. Environ. 140 (1-2), 309–313. doi:10.1016/j.agee.2010.12.005
Keske, C., Godfrey, T., Hoag, D., and Abedin, J. (2019). Economic feasibility of biochar and agriculture coproduction from canadian black spruce forest. Food Energy secur. 9 (4), 188. doi:10.1002/fes3.188
Kizito, S., Luo, H., Lu, J., Bah, H., Dong, R. J., Wu, S. B., et al. (2019). Role of nutrient-enriched biochar as a soil amendment during maize growth: Exploring practical alternatives to recycle agricultural residuals and to reduce chemical fertilizer demand. Sustainability 11, 3211. doi:10.3390/su11113211
Krivoruchko, A., Kuyukina, M., and Ivshina, I. (2019). Advanced Rhodococcus biocatalysts for environmental biotechnologies. Catalysts 9 (3), 236. doi:10.3390/catal9030236
Kumar, A., Singh, E., Singh, L., Kumar, S., and Kumar, R. (2021). Carbon material as a sustainable alternative towards boosting properties of urban soil and foster plant growth. Sci. Total Environ. 751, 141659. doi:10.1016/j.scitotenv.2020.141659
Laird, D. A., Fleming, P., Davis, D. D., Horton, R., Wang, B. Q., Karlen, D. L., et al. (2010). Impact of biochar amendments on the quality of a typical Midwestern agricultural soil. Geoderma 158 (3-4), 443–449. doi:10.1016/j.geoderma.2010.05.013
Lashari, M. S., Ye, Y. X., Ji, H. S., Li, L. Q., Kibue, G. W., Lu, H. F., et al. (2014). Biochar-manure compost in conjunction with pyroligneous solution alleviated salt stress and improved leaf bioactivity of maize in a saline soil from central China: A 2-year field experiment. J. Sci. Food Agric. 95 (6), 1321–1327. doi:10.1002/jsfa.6825
Laskosky, J. D., Mante, A. A., Zvomuya, F., Amarakoon, I., and Leskiw, L. (2020). A bioassay of long-term stockpiled salvaged soil amended with biochar, peat, and humalite. Agrosyst. Geosci. Environ. 3 (1). doi:10.1002/agg2.20068
Lehmann, J., Rillig, M. C., Thies, J., Masiello, C. A., Hockaday, W. C., Crowley, D., et al. (2011). Biochar effects on soil biota-A review. Soil Biol. Biochem. 43 (9), 1812–1836. doi:10.1016/j.soilbio.2011.04.022
Li, J., Chen, Y., Qin, X., Cao, A., and Lu, A. (2022). Impact of biochar on rhizosphere bacterial diversity restoration following chloropicrin fumigation of planted soil. Int. J. Environ. Res. Public Health 19 (4), 2126. doi:10.3390/ijerph19042126
Liang, Y. C., Si, J., Nikolic, M., Peng, Y., Chen, C., Jiang, Y., et al. (2005). Organic manure stimulates biological activity and barley growth in soil subject to secondary salinization. Soil Biol. Biochem. 37 (6), 1185–1195. doi:10.1016/j.soilbio.2004.11.017
Liu, L. J., Sun, C. L., Liu, S. R., Chai, R. S., Huang, W. Q., Liu, X., et al. (2015). Bioorganic fertilizer enhances soil suppressive capacity against bacterial wilt of tomato. PloS One 10 (4), e0121304. 1371/journal.pone.0121304. doi:10.1371/journal.pone.0121304
Liu, Z. L., Dugan, B., Masiello, C. A., and Gonnermann, H. M. (2017). Biochar particle size, shape, and porosity act together to influence soil water properties. PloS One 12 (6), e0179079. 1371/journal.pone.0179079. doi:10.1371/journal.pone.0179079
Lu, P. N., Bainard, L. D., Ma, B., and Liu, J. H. (2020). Bio-fertilizer and rotten straw amendments alter the rhizosphere bacterial community and increase oat productivity in a saline-alkaline environment. Sci. Rep. 10 (1), 19896. doi:10.1038/s41598-020-76978-3
Naher, U. A., Biswas, J. C., Maniruzzaman, M., Khan, F. H., Sarkar, M., Jahan, A., et al. (2021). Bio-organic fertilizer: A green Technology to reduce synthetic N and P fertilizer for rice production. Front. Plant Sci. 12, 602052. 3389/fpls.2021.602052. doi:10.3389/fpls.2021.602052
Razzaghi, F., Obour, P. B., and Arthur, E. (2019). Does biochar improve soil water retention? A systematic review and meta-analysis. Geoderma 361, 114055. doi:10.1016/j.geoderma.2019.114055
Ren, H., Huang, B., Fernández-García, V., Miesel, J., Yan, L., Lv, C. Q., et al. (2020). Biochar and rhizobacteria amendments improve several soil properties and bacterial diversity. Microorganisms 8 (4), 502. doi:10.3390/microorganisms8040502
Sarfraz, R., Yang, W. H., Wang, S. S., Zhou, B. Q., and Xing, S. H. (2020). Short term effects of biochar with different particle sizes on phosphorous availability and microbial communities. Chemosphere 256, 126862. doi:10.1016/j.chemosphere.2020.126862
Saxena, J., Rana, G., and Pandey, M. (2013). Impact of addition of biochar along with Bacillus sp. on growth and yield of French beans. Sci. Hortic. 162, 351–356. doi:10.1016/j.scienta.2013.08.002
Schmidt, H. P., Kammann, C., Niggli, C., Evangelou, M. W. H., Mackie, K. A., Abiven, S., et al. (2014). Biochar and biochar-compost as soil amendmentsto a vineyard soil: Influences on plant growth, nutrient uptake, plant healthand grape quality. Agric. Ecosyst. Environ. 191, 117–123. doi:10.1016/j.agee.2014.04.001
Shabala, S., Wu, H. H., and Bose, J. (2015). Salt stress sensing and early signalling events in plant roots: Current knowledge and hypothesis. Plant Sci. 241, 109–119. doi:10.1016/j.plantsci.2015.10.003
Shen, X. L., Zeng, J. F., Zhang, D. L., Wang, F., Li, Y. J., Yi, W. M., et al. (2019). Effect of pyrolysis temperature on characteristics, chemical speciation and environmental risk of cr, mn, cu, and zn in biochars derived from pig manure. Sci. Total Environ. 704 (20), 135283.1–135283.11. doi:10.1016/j.scitotenv.2019.135283
Shi, Y. L., Liu, X. R., Zhang, Q. W., Gao, P. L., and Ren, J. Q. (2019). Biochar and organic fertilizer changed the ammonia-oxidizing bacteria and archaea community structure of saline-alkali soil in the North China Plain. J. Soils Sediments 20 (3), 12–23. doi:10.1007/s11368-019-02364-w
Sofy, M. R., Elhawat, N., and Alshaal, T. (2020). Glycine betaine counters salinity stress by maintaining high K+/Na+ ratio and antioxidant defense via limiting Na+ uptake in common bean (Phaseolus vulgaris L.). Ecotoxicol. Environ. Saf. 200, 110732. doi:10.1016/j.ecoenv.2020.110732
Sun, Z. C., Bruun, E. W., Arthur, E., Jonge, L. D., Moldrup, P., Hauggaard-Nielsen, H., et al. (2014). Effect of biochar on aerobic processes, enzyme activity, and crop yields in two sandy loam soils. Biol. Fertil. Soils 50 (7), 1087–1097. doi:10.1007/s00374-014-0928-5
Vassilev, N., Vassileva, M., Lopez, A., Martos, V., Reyes, A., Maksimovic, I., et al. (2015). Unexploited potential of some biotechnological techniques for biofertilizer production and formulation. Appl. Microbiol. Biotechnol. 99, 4983–4996. org/10. 1007/s00253- 015- 6656-4. doi:10.1007/s00253-015-6656-4
Verheijen, F. G. A., Zhuravel, A., Silva, F. C., Amaro, A., Ben-Hur, M., Keizer, J. J., et al. (2019). The influence of biochar particle size and concentration on bulk density and maximum water holding capacity of sandy vs sandy loam soil in a column experiment. Geoderma 347, 194–202. doi:10.1016/j.geoderma.2019.03.044
Vishnu, V., Usadadia, V. P., Mawalia, A. K., Patel, M. M., and Patel, V. K. A. (2017). Impact assessment of land configuration and bio-organic on nutrient uptake and quality of chickpea (Cicer arietinum L.) under coastal salt affected soil. Int. J. Pure App. Biosci. 5 (3), 726–734. org/10.18782/2320-7051.2832. doi:10.18782/2320-7051.2832
Wang, T., Xu, Q., Gao, D., Zhang, B., Zuo, H., Jiang, J., et al. (2021). Effects of thinning and understory removal on the soil water-holding capacity in Pinus massoniana plantations. Sci. Rep. 11 (1), 13029. doi:10.1038/s41598-021-92423-5
Yang, Y., Liu, L., Singh, R. P., Meng, C., Ma, S., Jing, C., et al. (2020). Nodule and root zone microbiota of salt-tolerant wild soybean in coastal sand and saline-alkali soil. Front. Microbiol. 11, 2178. 3389/fmicb.2020.523142. doi:10.3389/fmicb.2020.523142
Ye, L., Camps-Arbestain, M., Shen, Q., Lehmann, J., Singh, B., Sabir, M., et al. (2019). Biochar effects on crop yields with and without fertilizer: A meta-analysis of field studies using separate controls. Soil Use Manag. 36 (1), 2–18. doi:10.1111/sum.12546
You, X. W., Yin, S. J., Suo, F. Y., Xu, Z. C., Chu, D. P., and Li, Y. Q. (2021). Biochar and fertilizer improved the growth and quality of the ice plant (Mesembryanthemum crystallinum L.) shoots in a coastal soil of Yellow River Delta, China. Sci. Total Environ. 775 (27), 144893. doi:10.1016/j.scitotenv.2020.144893
Yu, O. Y., Raichle, B., and Sink, S. (2013). Impact of biochar on the water holding capacity of loamy sand soil. Int. J. Energy Environ. Eng. 4 (1), 44. doi:10.1186/2251-6832-4-44
Zhang, H. Z., Chen, C. R., Gray, E. M., Boyd, S. E., Yang, H., Zhang, D. K., et al. (2016). Roles of biochar in improving phosphorus availability in soils: A phosphate adsorbent and a source of available phosphorus. Geoderma 276, 1–6. doi:10.1016/j.geoderma.2016.04.020
Zhang, N., Xin, H. E., Zhang, J., Raza, W., Yang, X. M., Yun-Ze, R., et al. (2014). Suppression of Fusarium wilt of banana with application of bio-organic fertilizers. Pedosphere 24 (5), 613–624. doi:10.1016/S1002-0160(14)60047-3
Zhang, S., Cui, J., Wu, H., Zheng, Q., Song, D. L., and Zhang, S. (2021). Organic carbon, total nitrogen, and microbial community distributions within aggregates of calcareous soil treated with biochar. Agric. Ecosyst. Environ. 314, 107408. doi:10.1016/j.agee.2021.107408
Zhao, F. Y., Zhang, Y. Y., Dong, W. G., Zhang, Y. Q., Zhang, G. X., Yang, L. J., et al. (2019). Vermicompost can suppress Fusarium oxysporum f. sp. lycopersici via generation of beneficial bacteria in a long-term tomato monoculture soil. Plant Soil 440, 491–505. doi:10.1007/s11104-019-04104-y
Zhao, J., Liu, J., Liang, H., Huang, J., Chen, Z., Wang, Y., et al. (2018). Manipulation of the rhizosphere microbial community through application of a new bio-organic fertilizer improves watermelon quality and health. PloS one 13 (2), e0192967. 1371/journal.pone.0192967. doi:10.1371/journal.pone.0192967
Zhao, X. Y., Bian, X. Y., Li, Z. X., Wang, X. W., Yang, C. J., Yang, C. P., et al. (2014). Genetic stability analysis of introduced Betula pendula, Betula kirghisorum, and Betula pubescens families in saline-alkali soil of northeastern China. Scand. J. For. Res. 29 (7), 639–649. doi:10.1080/02827581.2014.960892
Keywords: different biochar particles sizes, bio-organic fertilizer, rhizosphere soil, microecological environment, saline–alkali land
Citation: Gu Y-y, Zhang H-y, Liang X-y, Fu R, Li M and Chen C-j (2022) Effect of different biochar particle sizes together with bio-organic fertilizer on rhizosphere soil microecological environment on saline–alkali land. Front. Environ. Sci. 10:949190. doi: 10.3389/fenvs.2022.949190
Received: 20 May 2022; Accepted: 29 June 2022;
Published: 05 August 2022.
Edited by:
Yuxue Liu, Zhejiang Academy of Agricultural Sciences, ChinaReviewed by:
Wenhai Mi, Yangzhou University, ChinaManhattan Lebrun, Czech University of Life Sciences Prague, Czechia
Copyright © 2022 Gu, Zhang, Liang, Fu, Li and Chen. This is an open-access article distributed under the terms of the Creative Commons Attribution License (CC BY). The use, distribution or reproduction in other forums is permitted, provided the original author(s) and the copyright owner(s) are credited and that the original publication in this journal is cited, in accordance with accepted academic practice. No use, distribution or reproduction is permitted which does not comply with these terms.
*Correspondence: Meng Li, bGltMDMyMEAxMjYuY29t; Chuan-jie Chen, Y2h1YW5qaWU3OUAxNjMuY29t