- 1Water Research Group, Unit for Environmental Sciences and Management, North-West University, Potchefstroom, South Africa
- 2Department of Zoology and Entomology, Qwaqwa Campus, University of the Free State, Bloemfontein, South Africa
- 3Laboratory of Systemic, Physiological and Ecotoxicological Research, Department of Biology, University of Antwerp, Antwerp, Belgium
Compared to aquatic ecosystems, limited information exists on organochlorine accumulation in terrestrial ecosystems, and this is specifically true for terrestrial carnivores that have received limited attention in terms of studies on pollutant bioaccumulation. The African Leopard, Panthera pardus pardus (Linnaeus, 1758), is a popular focal species for research by ethologists and ecologists, but a noticeable knowledge gap exists with regards to toxicological aspects. To address this gap, the aim of this study was to determine baseline organochlorine pesticide (OCP) concentrations in blood of live wild and captive leopards in South Africa, and to explore the relationship between OCP levels and different conservation management strategies. Peripheral blood samples of seven captive and seven wild leopards, representing regular and melanistic individuals within the captive population, were collected while under sedation. The ΣOCP concentrations in blood serum were detected by means of GC-ECD. Statistical relationships among pesticide content in leopards from three geographical areas, different sexes, age groups and conservation status were examined. Captive leopards from this study had a slightly higher mean ΣOCP concentration (901 pg/ml) than wild leopards (768 pg/ml), and captive females had lower mean levels of ΣOCPs (797 pg/ml) than males (1,058 pg/ml). OCPs accumulated in the following order DDTs (27%) > HCHs (21%) > Heptachlors (15%) > CHLs (15%) > Drins (14%) > HCB (8%). Differences in OCP composition profiles of resampled captive individuals were also found. The sources of OCPs in the leopards can be attributed to the historic and current use of, e.g., DDT and HCHs, for malaria vector control and in insecticides/avicides in South Africa. For the captive leopards in this study the main source is possibly contaminated chicken that formed the major portion of their diet. This is the first report on OCP concentrations from leopards in Africa and highlights the need for this parameter to be considered in terms of the conservation management of healthy populations.
Introduction
Persistent Organic Pollutants (POPs), including organochlorine pesticides (OCPs), are resistant to biological, physical and chemical breakdown and as a result they have been banned world-wide through the Stockholm Convention in 2004 (Bouwman, 2004). Despite their banning, OCPs are still widely distributed in African ecosystems (Bouwman et al., 2014; Volschenk et al., 2019; Gerber et al., 2021). South Africa, a known user of OCPs in the agricultural sector (Wepener and Chapman, 2012), is the largest pesticide user south of the Sahara (Dabrowski et al., 2014). According to Dabrownski et al. (2014) pesticides are widely used for veterinary and crop protection purposes in South Africa, which helps to promote food security. Coupled with the increasing development in rural malaria-endemic areas, increased reliance on insecticides has become the trend in South Africa (Wepener and Chapman, 2012). South Africa has used DDT since the late 1940s as a means to control malaria (Bouwman, 2004) and residues have been measured in human breast milk (Bouwman et al., 1990) and blood (Gaspar et al., 2015) of residents of malaria endemic areas. The semi-volatile nature of OCPs results in their long-distance distribution to regions where there has been no direct use of these chemicals, e.g., ending up in coastal regions and remote river headwaters (Viljoen et al., 2016; Verhaert et al., 2017; Erasmus et al., 2020; Rose et al., 2020). This has led to a recent increase in studies addressing levels of OCPs in terrestrial and aquatic environments in South Africa (Thompson et al., 2017a; Volschenck et al., 2019; Gerber et al., 2021; Wolmarans et al., 2021). Due to trophic migration of compounds along the food web, the highest concentrations are usually reached at the top trophic level of a chain (Skaare et al., 2000), as seen in the study on trophic biomagnification of perfluorinated compounds in lichen, caribou and wolves (Müller et al., 2011). This results in the highest OCP concentrations usually ending up in apex predators and therefore the study of biomagnification is particularly relevant in species that are at the top of the food web.
In contrast to aquatic apex predators, reports on the incidence of POPs in terrestrial apex predators are surprisingly limited and Rodríguez-Jorquera et al. (2017) found that there is biased coverage of this subject, with most literature originating from Europe and North America (see Supplementary Table S1). Most work done to date has been on marine mammals, concentrated in Asia, western Europe and North America. The majority of studies on mammalian carnivores focused on polar bears (Ursus maritimus) (e.g., Bentzen et al., 2008a), otters (e.g., Carpenter et al., 2014) and mink (e.g., Romanić et al., 2015), which are all apex predators in aquatic systems. Very few reports on mammalian apex predators from Africa exist. Cockroft et al. (1989) determined the concentrations of PCBs, dichlorodiphenyltrichloroethanes (DDTs) and dieldrin in blubber samples of bottlenose dolphins (Tursiops aduncus) along the east coast of South Africa and recently Malarvannan et al. (2020) published the first record of POPs in serum of lion (Panthera leo), hyena (Crocuta crocuta) and cheetah (Acinonyx jubatus) from conservation areas in South Africa and the Antwerp Zoological Gardens in Belgium, while Leighton et al. (2022) recorded organochlorines in plasma and adipose tissue of caracal (Caracal caracal) from peri-urban areas in the Western Cape peninsula.
Notwithstanding their important ecological role, the distribution of some of the most wide-ranging African predators has diminished by more than 76% (Ray et al., 2005). The African leopard (Panthera pardus pardus) is the least studied of all big cats (Bailey, 1993; Maheshwari, 2006). They are highly adapted to live in diverse habitats, can persist regardless of various pressures and utilize food resources not favoured by other large carnivores (Norton et al., 1986; Bailey, 1993; Spong et al., 2000). These cats are generalists and will eat whatever food is easiest to obtain, ranging from beetles to large ungulate species (Bailey, 1993; Hayward et al., 2006). Leopards have the ability to live in human-modified habitats whereas other large felids such as lions and tigers cannot (Ray et al., 2005; Athreya and Belsare, 2007), with the largest part of their population occurring outside formally protected areas (Daly et al., 2005). This puts them more frequently in contact with anthropogenic factors than any other big cats in Africa. Nationally protected areas where leopards are still known to exist in viable populations include the Kgalagadi Transfrontier Park, Kruger National Park and Addo Elephant National Park (Daly et al., 2005). The constraints in capturing and working with large, dangerous apex predators make it problematic to investigate the occurrence and effects of toxic chemicals in these animals. As Rodríguez-Jorquera et al. (2017) pointed out, the most convenient way of obtaining data in this subject area is to process carcasses and rely on post-mortem results, which does not necessarily provide information on the effects of sub-lethal concentrations in live animals.
The aims of this study were to 1) establish baseline concentrations of OCPs in African leopards in South Africa; 2) compare differences between captive and wild leopards; and 3) do a preliminary assessment of individual and group category differences to account for anticipated life history effects of sex, age class, phenotypes and conservation management approaches.
Materials and methods
Study area
Fourteen leopards were sampled at six localities in central and northern South Africa (Figure 1) over a period of 3 years (2013–2015), and a total of 16 samples were analyzed as two of the captive leopards were sampled twice (Table 1). It is acknowledged that the sample size may be construed as being relatively small, but this does represent the largest single sampling of live African leopards to date. The limited number of individuals available for this study again shows the difficulty in obtaining material from live African leopards, even from captive animals since of the 11 facilities throughout South Africa, only two (one in the Free State and one in Mpumalanga) agreed to the sampling of their animals. For this study, leopards were classified as “captive” if they were born or raised in captivity from an early age (<1 month-old). Leopards were classified as “wild” when born or caught at an adult stage in the wild or when held at rehabilitation centres for less than 6 months with the aim to relocate. Captive-bred leopards were sampled at two facilities in Bloemfontein, Free State Province ((29.1121° S, 26.2063° E and (29.0667° S, 26.1555° E)) and one facility in the Mpumalanga Province (25.2259° S, 30.1496° E). Wild leopards were sampled in the Mpumalanga (25.2259° S, 30.1496° E) and northern Limpopo provinces (24.5659° S, 31.0377° E and 24.5151° S, 30.9029° E). No leopards were sampled from the southern distribution areas.
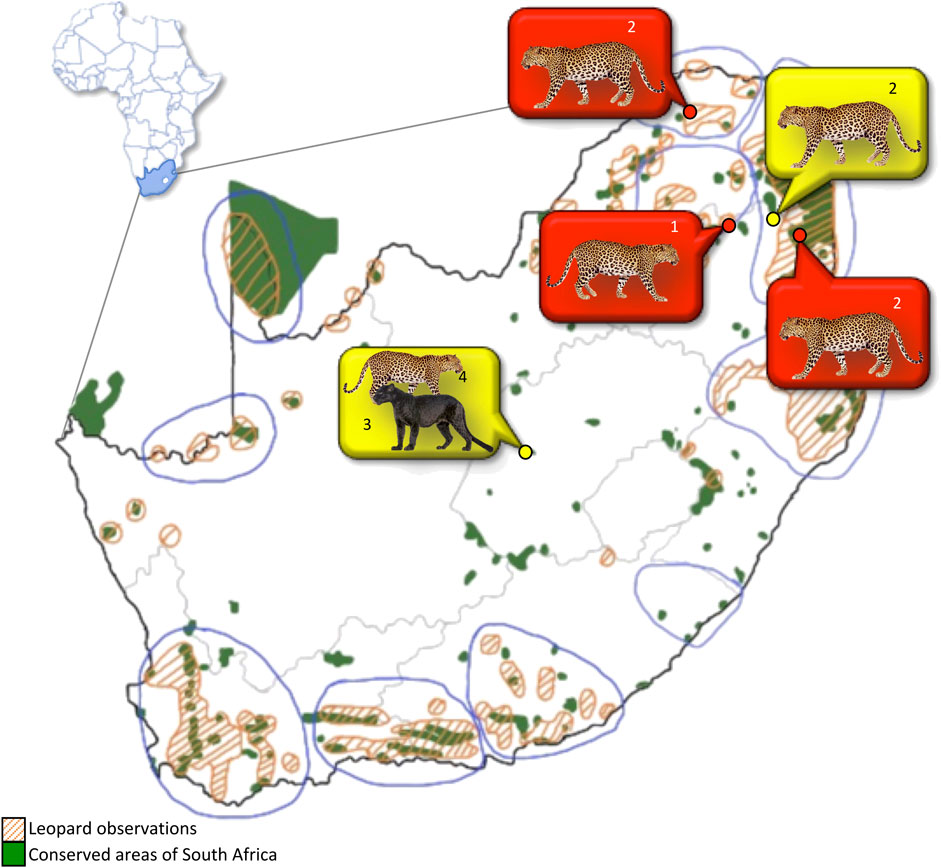
FIGURE 1. Ten core South African leopard populations (blue lined areas) in relation to formally conserved areas. Sampling locations indicated by yellow and red dots. Orange striped polygons: areas of leopard observations; Green polygons: conserved areas; Yellow bubble: captive leopards; Red bubble: wild leopards. Numbers indicate the number of individual leopards sampled at each location.
Sample collection and blood preparation
All leopards were sedated and sampled by a qualified veterinarian surgeon following standard procedures as prescribed and approved by AnimCare, a national registered Animal Ethics committee (North-West University ethics approval no. NWU-00255-17-A5). Wild leopards were sampled at night and were caught by means of baiting, as well as free darting. Captive leopards were sampled during the daytime hours and were sedated in their enclosures by resident veterinarians familiar to these animals. All leopards were sampled while under sedation and under the supervision of the registered veterinarian of that specific region. The pharmaceuticals used to induce sedation was unique for each veterinarian throughout the study and mostly constituted the use of a solution of ketamine (Anaket-V; Centaur labs) (20–25 mg) and Domitor® (Zoetis; South Africa) (dose 80–90 μg/kg), diluted with saline. All leopards were handled with sterile gloves and it took an average of 20 min to collect samples. Following sampling, leopards were revived by the veterinarian through injection with Antisedan® (Zoetis; South Africa). All leopards were carefully monitored until they were fully awake and alert.
Peripheral blood was usually collected from the jugular or the cephalic vein by venipuncture with the use of a sterile Vacutainer system, in BD Vacutainer® (Franklin Lakes, United States) CAT (Clot Activator Tubes). Samples were left overnight in a cooler box with an ice pack to keep temperatures stable, allowing blood components to separate. Early the following morning the separated serum was aliquoted into smaller Eppendorf vials (approximately 1 ml each), labelled and stored at -20°C and transported to the analytical laboratories of the North-West University, South Africa for further analyses. Blood samples were obtained from representative leopards of three core wild populations as identified by Daly et al. (2005). Samples from captive leopards represented two colour variations, six had the regular colour variation and three leopards were melanistic. Four captive males and five females were used for this study. Although two age classes, as defined by Fattebert et al. (2015), were noted, the effect of age was not analysed due to the small sample size (n = 3) for the subadults. Two captive leopards, a regular female and a melanistic male, from the same facility, were sampled twice throughout the study, with at least 1 year between the first and second sampling.
Organochlorine analysis
Levels of α-HCH, β-HCH, γ-HCH, δ-HCH, HCB, aldrin, dieldrin, endrin, heptachlor, oxy-chlordane, cis-heptachlor-epoxide, trans-heptachlor-epoxide, trans-chlordane, trans-nonachlor, cis-chlordane, cis-nonachlor, o,p’-DDE, p,p’-DDE, o,p’-DDD, o,p’-DDT, p,p’-DDD and p,p’-DDT in serum were determined using adaptations of a method by Keller et al. (2004). A known volume of serum (approximately 1 ml) of serum from each leopard was used for extraction. Prior to extraction 100 μl of 100 μg/l chlorinatedbiphenyl (PCB #143) and 100 μl of 100 μg/l epsilon-hexachlorocyclohexane (ε-HCH) was added to each sample as internal standard. PCB #143 was used as an internal standard for the organochlorine pesticides as it has similar functional groups, polarity, and molecular size to the larger OCPs but is not expected in environmental samples. Unfortunately, no suitable OCP was found with no probability of environmental presence to serve as the perfect Internal standard for larger OCPs in this analysis. The use of certain PCBs as internal standards for OCPs is a long-standing accepted practice for this reason (see Covaci et al., 2001; Covaci 2006; López et al., 2007). Sample extraction consisted of liquid-liquid extraction using 2 ml formic acid (>88%) and 4 ml of amethyl tert-butyl ether (MTBE) and hexane mix (MTBE:hexane = 1:1 v/v) in 15 ml polypropylene tubes. Samples were briefly vortexed (Vortex-Genie 2; Scientific Industries, Inc.) to ensure solvent interaction, followed by sonication for 20 min in an ultrasonic bath (Scientech) at 25°C. Following sonication samples were centrifuged for 5 min at 2,000 rpm in a Centrifuge 5,430 (Eppendorf), and the organic phase collected. The extraction process was repeated three times in total, with the second and third extraction performed only with 4 ml MTBE:hexane (1:1 v/v). The collective organic extract sample volume was reduced to <10 ml at 36°C in a Turbo Vap ® Classic (Biotage) evaporator. Sample clean-up consisted of elution through a 40 cm glass column tightly packed with 4 g Florisil (5% deactivated), topped with (≈1 cm) sodium-sulfate, and prepared with hexane. The sample was eluted with 35 ml of dichloromethane:hexane (3:7 v/v) after which it was evaporated to near dryness (≈5 μl) under a gentle N2 stream at 36°C. The sample was reconstituted in 100 μl n-decane containing tetrachloro-m-xylene (100 μg/l) as surrogate marker and analysed using gas chromatography (Hewlett Packard ®6890) coupled with a63Ni micro-electroncapture detector (GC-μECD).
The GC-ECD analysis was performed using a splitless injection (1 μl) at inlet temperature of 225°C. This was specifically lowered from initial methods of 260°C during the method development phase to prevent thermal degradation of DDT during chromatographic analysis, which was confirmed to have no significant impact on analysis repeatability at 225°C through the method validation process. The oven program initiated at 100°C held for 1 min, followed by a ramp of 20°C/min to 200°C, then changing to a ramp of 6°C/min until 260°C held for 4 min. Separation of compounds was achieved using a 30 m length × 0.25 mm I.D. × 0.25 μm film HT8-MS (SGE) column and H2 carrier gas at a constant flow of 1.5 ml/min. The detector temperature was set at 310°C with N2 as the make-up gas (60 ml/min). Calibration of the 22 OCP peaks was performed using a 7-point calibration curve of the Ehrenstorfer® pesticide mix 1,023 (LGC Standards) ranging between 5 and 1,000 μg/L with a linear regression R2 > 0.998 for all analytes. The instrumental limit of detection (LOD) and limit of quantification (LOQ) was calculated based on 3 times and 10 times (respectively) the standard deviation of the slope of the calibration curve of each compound The instrumental LODs were as follows: α-HCH = 1 μg/L, HCB = 10 μg/L, β-HCH = 5 μg/L, γ-HCH = 3 μg/L, δ-HCH = 0.8 μg/l, heptacLlor = 12 μg/L, aldrin = 9 μg/L, oxy-chlordane = 5 μg/L, cis-heptachlor-epoxide = 6 μg/L, trans-heptachlor-epoxide = 8 μg/L, o,p’-DDE = 11 μg/L, trans-chlordane = 11 μg/L, trans-nonachlor = 11 μg/L, cis-chlordane = 14 μg/L, p,p’-DDE = 6 μg/L, dieldrin = 14 μg/L, o,p’-DDD = 17 μg/L, endrin = 13 μg/L, o,p’-DDT = 26 μg/L, cis-nonachlor = 5 μg/L, p,p’-DDD = 30 μg/L, p,p’-DDT = 34 μg/L. The mean internal standard recovery was >80% for ε-HCH and slightly lower and more variable, 66 ± 20%, for PCB#143.
The extraction and GC analysis method was validated using Bovine blood as a matrix substitute spiked with the same pesticide mix used for calibration. Spiked samples were left to equilibrate for 24 h at 4°C prior to extraction. No matrix interference was observed within the retention time range of interest for any of the pesticides (tested through Blank Bovine blood analysis). The spiked bovine blood method validation samples had an average recovery of 78.8 ± 17% for all pesticides in 15 samples extracted and analysed in 3 batches over several days.
The analytical batches contained a blank after every 2 samples to assess the potential for carry-over. A quality control (QC) check for system stability was analysed with every 10th sample. Quality control standards had RSD <10% for all pesticides except p,p-DDT that showed an RSD of 11.8%.
Statistical analysis
All OCP concentrations are expressed as pg/ml and OCP profiles represent the relative percentage contribution of the detected compounds to the ΣOCP concentrations. To allow for statistical analysis, all concentrations that were below the LOQ were transformed to a value by multiplying the detection frequency with the LOQ (Wepener et al., 2012). The respective frequencies of detection for detected compounds were as follows: α-HCH 100%, HCB 91.7%, β-HCH 8.3%, γ-HCH 75%, δ-HCH 66.7%, heptachlor 100%, aldrin 58.3%, cis-heptachlor-epoxide 16.7%, trans-heptachlor-epoxide 25%, o,p’-DDE 33.3%, trans-chlordane 66.7%, trans-nonachlor 8.3%, cis-chlordane 33.3%, p,p’-DDE 50%, dieldrin 0%, o,p’-DDD 83.3%, endrin 100%, o,p’-DDT 33.3%, cis-nonachlor 58.3%, p,p’-DDD 100%, and p,p’-DDT 25%.
Data were analyzed with GraphPad Prism version 7 for Windows (GraphPad Software, La Jolla California United States, www.graphpad.com). All data were checked for normality and homogeneity of variance prior to analyses using the D’Augustino-Pearson omnibus normality test and Levene’s test, respectively. If data met the normality and homogeneity of variance assumptions, differences between the OCP levels in the selected cases were determined using the unpaired t test with Welch correction. Means of data that did not meet the normality and homogeneity of variance assumption were compared using a Mann-Whitney U test. Significant differences were regarded as p < 0.05.
Results
Organochlorine pesticide contamination in South African leopards
To our knowledge these are the first data on OCPs in wild and captive African leopards. Varying concentrations of OCPs were detected in all leopards from this study. All the OCPs tested for, with the exception of cis- and trans-heptachlor-epoxide, o,p’-DDT, p,p’-DDT and o,p’-DDE were recorded in leopard serum samples during the present study (Table 2). The average total OCP concentrations in the blood serum of leopards measured were 770 ± 230 (range 434–1,383) pg/ml. The OCP contamination pattern was in the decreasing order of DDTs (27%) > HCHs (21%) > heptachlors (15%) > CHLs (15%) > drins (14%) > HCB (8%) (Figure 2). Concentrations of α-HCH, HCB, endrin, heptachlor and p,p’-DDD were present in all fourteen leopards, at varying concentrations.
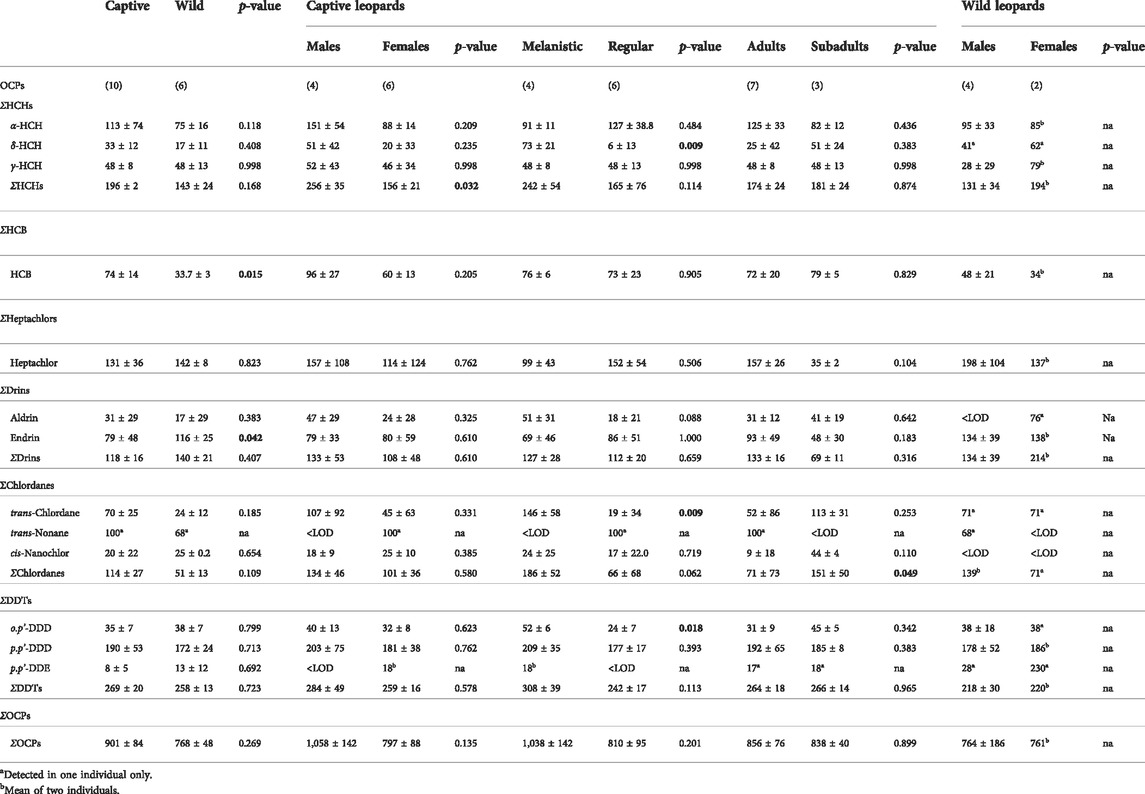
TABLE 2. Organochlorine concentrations (pg/ml) in serum samples from wild and captive leopards. The sample size (n) is presented in brackets. Bold p-values indicate a significant difference p < 0.05. <LOD, below limit of detection. Na, not applicable.
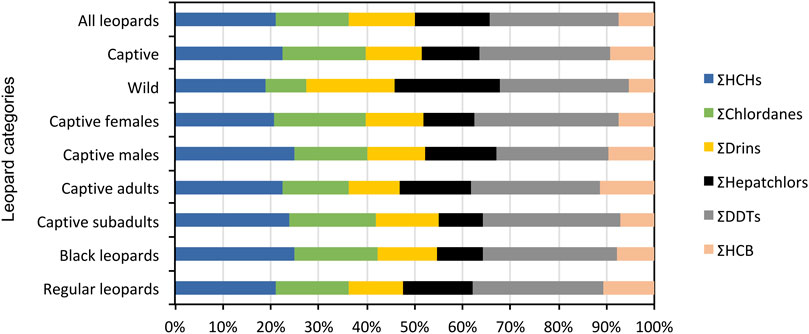
FIGURE 2. The relative contribution of main compound groups (ΣHCHs; ΣChlordanes; ΣDrins; ΣHeptachlors; ΣDDTs; ΣHCB) to ΣOCP concentrations as analyzed in all captive and wild leopards.
The OCP profiles of captive and wild leopards differed in that the contribution of heptachlors and drins were greater in wild leopards (Figure 2). Although the total OCP concentrations in wild leopards were lower than captive leopards it was not significantly different (Table 2). There were no significant differences in DDTs between wild and captive leopards with p,p’-DDD making up the highest concentration of the isomers (Table 2). Similarly, the HCHs did not differ significantly between wild and captive leopards with α-HCH being the predominant isomer. The heptachlors and chlordanes also did not differ significantly between wild and captive leopards. Only endrin was significantly higher in wild leopards (t = 2.3, df = 9, p = 0.042), while HCB were significantly higher in captive leopards (t = 2.9, df = 9, p = 0.015) (Table 2).
It was not possible to statistically compare the wild female and male OCP levels due to the small sample size for females (n = 2) (Table 2). However, based on the mean concentrations, total OCP concentrations appeared to be similar in wild males and females. Wild males had higher concentrations of HCB and heptachlors, while wild females had higher concentrations of HCHs and drins. Small insignificant differences between concentrations of chlordanes and DDTs were found.
Organochloride pesticide contamination in captive leopards
We acknowledge that sample sizes are too small to obtain meaningful inter- and intra-group comparisons, but in some cases we conducted statistical analyses to obtain preliminary insights into e.g., differences between life stages (sub-adult vs. adult) and colouration (normal vs. melanistic) in captive leopards. In captive leopards only the HCHs were significantly higher in males than females (t = 2.6, df = 8, p = 0.032) (Table 2). Notable, although not significant, was that all the OCP compounds/isomers were higher in captive males than in females (Table 2). Captive adult leopards had higher mean OCP serum concentrations than subadults, albeit not significant. Adults also had higher mean concentrations of hepatchlor and drins, while subadults had higher mean concentrations of HCHs, HCB and significantly higher concentration of chlordanes (U = 8, df = 11, p = 0.049) (Table 2). Except for heptachlor, the concentrations of OCP compounds/isomers were higher in captive melanistic leopards compared to the regular coloration leopards (Table 2).
Two captive leopards from the same facility in the Free State were sampled twice with at least 2 years between the sampling events. Total OCP concentrations in serum of the regular female decreased by 35% from 666 to 434 pg/ml between the two sampling periods. Notable changes in her OCP contamination profile were observed between samplings (Figure 3), which showed that heptachlors were higher during the second sampling but both the HCB and chlordanes decreased to below LOD. Total OCP concentrations in the melanistic male serum also decreased (39%) between the two sampling periods from 1,383 to 842 pg/ml. A notable change in the male’s OCP contamination profile was decreased chlordane and heptachlor concentrations from the second sampling event. The HCHs were the predominant compound in the male’s 2015 sample compared to DDTs in the 2013 sample (Figure 3). The only compounds that increased between sampling was heptachlors in the female (156%) and HCB in the male (34%). Unlike the female, no compounds in the male decreased to below LOD between samplings.
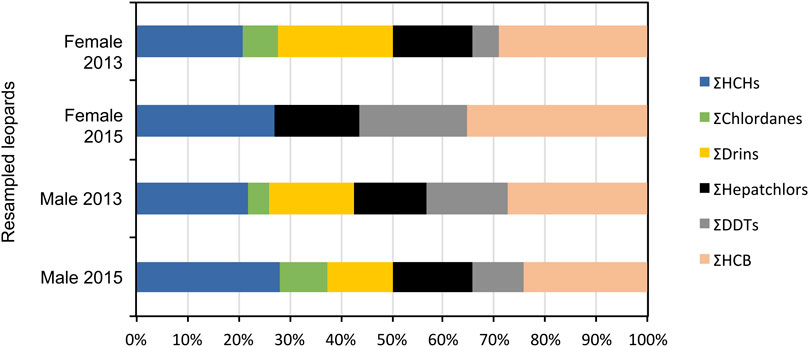
FIGURE 3. The relative contribution of main compound groups (ΣHCHs; ΣChlordanes; ΣDrins; ΣHeptachlors; ΣDDTs; ΣHCB) to ΣOCP concentrations as analyzed in the two resampled captive leopards.
Discussion
There are clear gaps in knowledge with regards to toxicological work on apex predators. The constraints in capturing and working with large, dangerous carnivores make it problematic to investigate the occurrence and effects of toxic chemicals in these animals. According to Rodríguez-Jorquera et al. (2017) it is convenient to obtain tissue from carcasses for chemical residue analysis, but it does not necessarily provide information on sub-lethal concentrations in live animals. In contrast, the advantages of using blood serum samples include the possibility of resampling individuals in future studies, and stress inducement on study subjects are less due to its lower invasiveness. Few studies report on OCP concentrations from blood or plasma samples of apex terrestrial carnivores (Supplementary Table S1), and these authors only report on a few OCP compounds. Whilst it is acknowledged that the sample size of this study was relatively small and precludes from undertaking meaningful statistical analyses, it is still worthwhile commenting on some of the findings. In addition, we do acknowledge that for some compounds the recovery rates were below 80% and for these the reported concentrations were adapted correspondingly. Despite the levels of OCPs being low in the serum of leopards, we are confident that the data are relatively accurate since they are all within the same order of magnitude as OCPs reported in blood/plasma/serum of mammalian carnivores elsewhere in the literature (see Supplementary Table S1).
Contextualising organochlorines in leopards
This study found limited significant differences in ΣOCPs, individual compounds and isomers among many of the groups tested. The OCP contamination pattern found in wild leopards were similar to that found in wild lions, spotted hyenas (Malarvannan et al., 2020) and caracal (Leighton et al., 2022) in South Africa. The only group in which ΣDDTs was not the main contributing compound was in captive males, where like reports from brown bears and grey wolves in Croatia (Romanić et al., 2015), ΣHCHs were the main compounds. ΣDDTs concentrations from leopards (0.22 ng/ml) were lower than that of domestic sled dogs (29 ng/ml) from Greenland (Sonne, 2010), wild spotted hyenas (1.6 ng/ml) from South Africa (Malarvannan et al., 2020) and wild caracal (2.5 ng/ml) from South Africa (Leighton et al., 2022). The ΣDDTs concentrations in leopards were however similar to that of wild lions (0.27 ng/ml) from South Africa (Malarvannan et al., 2020). Other mammals in which ΣDDTs was the main contributing compound in plasma or blood samples include red foxes and wolverines from Sweden (Polder et al., 2009), Eurasian lynxes from Norway (Polder et al., 2009), masked palm civets from Japan (Yamamoto et al., 2012), wild lions and hyenas from South Africa (Malarvannan et al., 2020) and caracal from South Africa (Leighton et al., 2022).
Traces of p,p’-DDE, o,p’-DDD and p,p’-DDD were detected in wild and captive leopards from this study. Comparatively higher concentrations of p,p’-DDE have been reported from the blood of other terrestrial mammalian carnivores such as red foxes, wolverines and Eurasian and Iberian lynxes (Polder et al., 2009; Mateo et al., 2012), lions, spotted hyenas and cheetahs (Malarvannan et al., 2020) and caracal (Leighton et al., 2022). Leighton et al. (2022) and Malarvannan et al. (2020) also found traces of p,p’-DDT in wild lions, spotted hyenas and caracal from South Africa, respectively and captive lions and cheetahs in Belgium. Reports on o,p’-DDD and p,p’-DDD from other tissues such as liver and muscle of mammalian terrestrial carnivores are more frequent (see Supplementary Table S1), but not comparable to the results of this study due to the lipophilic nature of the tissues reported on. There is an anomaly in our data that we cannot explain where the concentrations of p,p’-DDD in wild leopards from our study, were much higher than the concentrations reported from other studies in the northern hemisphere [i.e., red foxes in Sweden, Norway Polder et al. (2009) and Spain (Mateo et al., 2012), Polar bears in Norway (Skaare et al., 2001), Wolverines in Norway (Polder et al., 2009), Iberian lynxes in Spain (Mateo et al., 2012) and Eurasian lynxes in Sweden (Polder et al., 2009). The chromatograms were re-evaluated, and we are confident that the peaks that were recorded were not due to any cross-contamination and due to the elution of p,p’-DDD. This is an aspect that requires further investigation. The p,p’-DDE in wild and captive leopards were similar to levels reported elsewhere in the literature and is usually the predominant metabolite of DDT encountered in terrestrial mammals (Bernhoft et al., 1997; Hoshi et al., 1998; Mateo et al., 2012; Romanić et al., 2015; Malarvannan et al., 2020). Its prevalence is indicative of historical contamination from DDT. It is therefore likely that the DDT contamination in leopards from this study can be attributed to the historic use of DDT in South Africa.
To the best knowledge of the authors, the detection of HCH-isomers from blood or plasma samples are rarely reported (see Supplementary Table S1). Unlike other studies, specifically the study on wild lions and spotted hyenas by Malarvannan et al. (2020) in South Africa, our study did not find traces of β-HCH in any leopards. This is surprising, as Willett et al. (1998), Bernhoft et al. (1997) and Bentzen et al. (2008b) suggested that β-HCH is far more persistent in mammals than α–HCH and γ-HCH isomers. In our study α–HCH was the largest of the HCHs, with concentrations higher in captive than in wild leopards. Interestingly, this contrasts with the results from Malarvannan et al. (2020) on lions and hyenas, who found that γ-HCH was the most prevalent HCH isomer. These authors suggested that this result might reflect a preferential usage of lindane, which consists of purified γ-HCH, in the regions their animals were sampled. The predominance of α–HCH in leopards from our study corresponds to the suggestion of Tomza-Marciniak et al. (2014), who reported that this is usually highest in animals near agricultural areas where mixtures of HCHs were recently used. This may suggest that leopards from our study are either continuously exposed to these mixtures of HCHs in agricultural areas, or that the leopard metabolism is better at metabolizing HCHs than that of other terrestrial mammalian carnivores. Contrary to the information generated in our study, no records of blood/plasma concentrations of α–HCH could be found in literature, even though studies by Romanić et al. (2015) suggest that these isomers contribute substantially to the OCP profiles of carnivores such as brown bears and grey wolves.
The comparison of OCP contamination between species and intraspecific groups must always be approached with caution (Skaare et al., 2000). Numerous factors such as age, sex, seasonality, feeding habits and reproductive status can influence levels of concentration within individuals. Differences in diet can result in variation within and between species (see Hop et al., 2002; Borgå et al., 2004; McKinney et al., 2009; 2013).
Diet and organochlorine accumulation
Although no necropsies and actual stomach content identifications were undertaken during our study, dietary regimes were obtained for captive leopards via interviews with their ex-situ managers and general information on the generalist diet of leopards and their feeding ecology is relatively common (Norton et al., 1986; Bailey, 1993; Hayward et al., 2006). So even though the home ranges of wild leopards from our study included agricultural areas, where pesticides are used on a regular basis, the captive leopards had higher ΣOCP concentrations in their blood. Data collected during visits to ex-situ facilities showed that captive leopards are regularly treated for ecto- and endoparasites with products such as Frontline. The portion of chicken meat in the diet of captive leopards, obtained from commercial broilers, is higher than that of red meat. It has been reported that commercial chicken meat in South Africa is contaminated with OCPs such as Chlordanes and DDTs (Quinn et al., 2011; Thompson et al., 2017a; 2017b). Keeping in mind that wild leopards may not have regular access to chicken meat but would rely more on whatever food source they can find, chicken meat may therefore be a contributing factor to increased OCP exposure among captive leopards in South Africa.
The only significant differences between captive and wild leopards were in their burdens of HCB and endrin. Captive leopards (0.07 ng/ml) had higher HCB levels in their blood serum than wild leopards (0.03 ng/ml), while wild leopards (0.12 ng/ml) had almost twice as much endrin in their blood than captive leopards (0.08 ng/ml). This is indicative of the differences in exposure routes and concentrations of these two groups. Wild leopards in South Africa are known to roam agricultural areas, where insecticides/avicides are regularly used for pest management. Reports on OCP contamination in livers of ground birds such as guinea fowl (e.g., p,p' -DDD–57 ng/g) and francolins (e.g., p,p' -DDD–345 ng/g) from these agricultural areas (Barnhoorn et al., 2009) may already be cause for concern as these birds are known to constitute quite a large part of the leopard diet. Contrary to our expectations, captive leopards had slightly higher concentrations of ΣOCPs, ΣHCHs, ΣHCB, ΣChlordanes and ΣDDTs than wild leopards. However, this result does correspond with levels reported by Malarvannan et al. (2020) from wild and captive lions. This is supported by the findings of Leighton et al. (2022) who attributed higher OCP bioaccumulation in caracal drawn to urbanised areas to the consumption of “exotic” prey (i.e., prey that would not form part of their natural diet in the wild). This results in a co-incidental increase in exposure to OCPs.
Life history and organochlorine accumulation
All leopards from this study had unique OCP profiles. Captive males had significantly higher ΣHCHs and HCH isomer (α–, β- and γ-HCH) concentrations than females, with all concentrations higher than that reported from the plasma of polar bears in Norway (Skaare et al., 2001) and lions and spotted hyena (Malarvannan et al., 2020). Apart from cis-nonachlor and endrin, captive males had higher OCP contamination of every compound and isomer tested, and an overall higher ΣOCP concentration than females. However, this was not the case for all mammalian carnivores, as can be seen in the study on Alaskan Arctic foxes (Harley et al., 2016) and British Eurasian otters (Mason et al., 1986), where the OCP burdens are higher in the blood of females than males. On the other hand, Polder et al. (2009) and Mateo et al. (2012) reported that sex may not be a factor in OCP contamination in Iberian lynx and Red foxes from Europe, and similar findings were documented by Shore et al. (2001) in Russian wolves. These findings coincide with the conclusion of Romanić et al. (2015), indicating that the effect of gender on OCP contamination may be species specific and that little is understood about how felids accumulate and metabolize OCPs. From our results it appears that in the case of leopards that gender differences in OCP contamination exist and it is most probably due to the unloading effect that pregnancies and lactation might have on females’ OCP burdens (also see below).
This study is the first to report on intraspecific differences in ΣChlordane concentrations among captive adult and subadult carnivores. Captive adult leopards had significantly lower ΣChlordane contamination than captive subadults. ΣChlordane concentrations from our study (0.13 ng/ml) was much lower than that reported from plasma of domestic sled dogs (770 ng/ml) from Greenland (Sonne, 2010), but much higher than that reported from the blood of caracal (0.03 ng/ml) (Leighton et al., 2022). Our findings concur with that of Bytingsvik et al. (2012), who reported that polar bear cubs had higher OCP concentrations in their blood plasma than their mothers. On the other hand, Mateo et al. (2012) found that age does not influence OCP concentrations in wild terrestrial carnivores such as the Iberian Lynx and red foxes. Our results suggest that leopards may be born with OCP contamination obtained during the foetal stage from the female or has been exposed to contamination during suckling (Bernhoft et al., 1997; Skaare et al., 2000; Greig et al., 2007; Jaspers et al., 2010).
There were some interesting observations related to OCP accumulation in captive melanistic and regular leopards that are worth mentioning. In general, most of the OCPs measured during this study were higher in the melanistic leopards when compared to the regular, e.g., leopards. Notably the δ-HCH were 14 times higher, trans-chlordane were seven times higher and the o,p’-DDD concentrations were double than regular leopards. This is while both groups are fed similar diets and have similar parasite treatment regimes. These data may indicate that black leopards are not able to metabolize these isomers as efficiently as their regular counterparts. It is, therefore, interesting to speculate that these findings may be attributed to the physiological effects accompanying melanism in mammals since it is known that melanin-producing cells have been reported to be effective at isolating potentially toxic metal ions (Sarna et al., 2022). Since the overall ΣChlordane concentrations are very similar between captive males and females from this study, it may be deducted that melanism in leopard males may influence the accumulation of ΣChlordanes, especially trans-chlordane. However, more sampling is needed to come to a definitive conclusion.
Organochlorine accumulation in resampled leopards
The OCP composition profiles of both resampled individuals changed between samples and ΣOCP concentrations decreased in both leopards. However, the ΣHeptachlor concentrations increased in the female and the ΣChlordanes levels increased in the male. Since this female had a litter of cubs between sampling, the female seemed to have either completely metabolized HCB, trans-nonane, cis-nonachlor, aldrin and o,p’-DDD from her blood or transferred it to her cubs through lactation, while there was a build-up of heptachlor and endrin, with very little change in the concentration of p,p’-DDD. These findings further support the phenomenon of maternal transfer of OCPs from the female to cubs, as discussed previously. It would thus stand to reason that the decrease in the abovementioned OCP compounds and isomers may be attributed to a number of factors: that the body is able to metabolize these compounds over time; levels decrease due to maternal transfer directly to the foetus and later lactation, or that exposure to these compounds diminish as the diet of leopards are changed as they get older.
Management implications
Concurring with Hall et al. (2006), the consideration of environmental pollutants should be a highly important factor in conservation and management concerns. There is growing literature that shows that despite the banning of many OCPs there is still a considerable amount that enter South African conservation areas (Volschenk et al., 2019; Gerber et al., 2021; Wolmarans et al., 2021). Currently, no specific guidelines for managing ex-situ leopard populations exist in South Africa. A successful conservation strategy promotes the awareness of the importance of leopard conservation, which includes an increased emphasis on ex-situ conservation. Effective conservation efforts should take a holistic approach, with all-inclusive evaluation of the occurrence of contaminants and the possible threats posed to apex predators.
Conclusion
The global leopard population is increasingly exposed to intensifying pressures due to habitat loss and impacts of chemical pollution on apex predator populations are seldom considered. Reports on OCP contamination in living terrestrial apex predators, with the possibility of resampling, are rare. As the first report establishing important base-line data for OCP contamination in South African leopards, coupled with our results show similar OCP serum concentrations compared to decade-old data reported for lion and hyena by Malarvannan et al. (2020) and more recently for caracal (Leighton et al., 2022). This study found that dietary factors have a greater influence on OCP accumulation than other intraspecific factors such as age and sex. Attention should be paid to implications for population growth, especially during harsh periods such as droughts when leopards may expand their home ranges, subsequently increasing their presence in agricultural areas where pesticides are used. Our study shows the need for future, continuous monitoring of POPs in terrestrial predators, especially in countries that are known users of OCPs in their agricultural sector and combating malaria, such as South Africa.
Data availability statement
The raw data supporting the conclusions of this article will be made available by the authors, without undue reservation.
Ethics statement
The animal study was reviewed and approved by the AnimCare, a national registered Animal Ethics committee of North-West University with ethics approval no. NWU-00255-17-A5.
Author contributions
MV: Conceptualization, investigation, formal analysis, writing—original draft and review and editing, visualization, project administration. NS: Conceptualization, resources, writing—review and editing, supervision, funding acquisition. NW: methodology, validation, writing—review and editing. VW: Conceptualization, formal analysis, resources, writing—review and editing, supervision.
Funding
This work was funded by the South African National Research Foundation (NRF) (NRF UID Grant: 109352, NS, PI). Conclusions derived to and opinions expressed are those of the authors and are not necessarily to be attributed to the National Research Foundation.
Acknowledgments
We want to thank the North-West University’s (NWU) Unit for Environmental Sciences and Management (UESM), for the use of their facilities, equipment and funding of this project. In addition, we would like to thank all the facilities that allowed us access to sites for sample collection. This is publication number 556 of the NWU Water Research Group.
Conflict of interest
The authors declare that the research was conducted in the absence of any commercial or financial relationships that could be construed as a potential conflict of interest.
Publisher’s note
All claims expressed in this article are solely those of the authors and do not necessarily represent those of their affiliated organizations, or those of the publisher, the editors and the reviewers. Any product that may be evaluated in this article, or claim that may be made by its manufacturer, is not guaranteed or endorsed by the publisher.
Supplementary material
The Supplementary Material for this article can be found online at: https://www.frontiersin.org/articles/10.3389/fenvs.2022.938453/full#supplementary-material
References
Athreya, V., and Belsare, A. (2007). Human-leopard conflict management guidelines. Pune, India: Kaati Trust.
Bailey, T. N. (1993). The african leopard: Ecology and behavior of a solitary felid. New York: Columbia University Press.
Barnhoorn, I. E. J., Bornman, M. S., Jansen van Rensburg, C., and Bouwman, H. (2009). DDT residues in water, sediment, domestic and indigenous biota from a currently DDT-sprayed area. Chemosphere 77, 1236–1241. doi:10.1016/j.chemosphere.2009.08.045
Bentzen, T. W., Follmann, E. H., Amstrup, S. C., York, G. S., Wooller, M. J., Muir, D. C. G., et al. (2008a). Dietary biomagnification of organochlorine contaminants in Alaskan polar bears. Can. J. Zool. 86, 177–191. doi:10.1139/Z07-124
Bentzen, T. W., Muir, D. C. G., Amstrup, S. C., and O'Hara, T. M. (2008b). Organohalogen concentrations in blood and adipose tissue of Southern Beaufort Sea polar bears. Sci. Total Environ. 406, 352–367. doi:10.1016/j.scitotenv.2008.07.030
Bernhoft, A., Wiig, Ø., and Skaare, J. U. (1997). Organochlorines in polar bears (Ursus maritimus) at Svalbard. Environ. Pollut. 95, 159–175. doi:10.1016/S0269-7491(96)00122-4
Borgå, K., Fisk, A. T., Hoekstra, P. F., and Muir, D. C. G. (2004). Biological and chemical factors of importance in the bioaccumulation and trophic transfer of persistent organochlorine contaminants in arctic marine food webs. Environ. Toxicol. Chem. 23, 2367. doi:10.1897/03-518
Bouwman, H., Reinecke, A. J., Cooppan, R. M., and Becker, P. J. (1990). Factors affecting levels of DDT and metabolites in human breast milk from KwaZulu. J. Toxicol. Environ. Health 31, 93–115. doi:10.1080/15287399009531440
Bouwman, H. (2004). South Africa and the Stockholm convention on persistent organic pollutants. S. A. J. Sci. 100, 323–328.
Bytingsvik, J., Lie, E., Aars, J., Derocher, A. E., Wiig, Ø., Jenssen, B. M., et al. (2012). PCBs and OH-PCBs in polar bear mother-cub pairs: A comparative study based on plasma levels in 1998 and 2008. Sci. Total Environ. 417-418, 117–128. doi:10.1016/j.scitotenv.2011.12.033
Carpenter, S. K., Mateus-Pinilla, N. E., Singh, K., Lehner, A., Satterthwaite-Phillips, D., Bluett, R. D., et al. (2014). River otters as biomonitors for organochlorine pesticides, PCBs, and PBDEs in Illinois. Ecotoxicol. Environ. Saf. 100, 99–104. doi:10.1016/j.ecoenv.2013.07.028
Cockroft, V. G., de Kock, A. C., Lord, A. D., and Ross, G. J. B. (1989). Organochlorines in bottlenose dolphins Tursiops truncatus from the East Coast of South Africa. South Afr. J. Mar. Sci. 8, 207–217. doi:10.2989/02577618909504562
Covaci, A. (2006). “Application of solid-phase disk extraction combined with gas chromatographic techniques for determination of organochlorine pesticides in human body fluids,” in Pesticide protocols. Methods in biotechnology. Editors J. L. Martínez Vidal, and A. G. Frenich (New Jersey: Humana Press), Vol. 19. doi:10.1385/1-59259-929-X:049
Covaci, A., Hura, C., and Schepens, P. (2001). Selected persistent organochlorine pollutants in Romania. Sci. Total. Environ. 280, 143–152. doi:10.1016/S0048-9697(01)00820-8r
Dabrowski, J. M., Shadung, J. M., and Wepener, V. (2014). Prioritizing agricultural pesticides used in South Africa based on their environmental mobility and potential human health effects. Environ. Int. 62, 31–40. doi:10.1016/j.envint.2013.10.001
B. Daly, J. Power, G. Camacho, K. Traylor-Holzer, S. Barber, S. Catterallet al. (Editors) (2005). “Leopard (Panthera pardus) PHVA. Workshop report,” Conservation breeding specialist group (SSC/IUCN)/CBSG South Africa (Hoedspruit: Endangered Wildlife Trust).
Erasmus, A., Ikenaka, Y., Nakayama, S. M. M., Ishizuka, M., Smit, N. J., Wepener, V., et al. (2020). Trophic transfer of pollutants within two intertidal rocky shore ecosystems in different biogeographic regions of South Africa. Mar. Pollut. Bull. 157, 111309. doi:10.1016/j.marpolbul.2020.111309
Fattebert, J., Balme, G., Dickerson, T., Slotow, R., and Hunter, L. (2015). Density-dependent natal dispersal patterns in a leopard population recovering from over-harvest. PloS One 10, e0122355. doi:10.1371/journal.pone.0122355
Gaspar, F. W., Chevrier, J., Bornman, R., Crause, M., Obida, M., Barr, D. B., et al. (2015). Undisturbed dust as a metric of long-term indoor insecticide exposure: Residential DDT contamination from indoor residual spraying and its association with serum levels in the VHEMBE cohort. Environ. Int. 85, 163–167. doi:10.1016/j.envint.2015.09.014
Gerber, R., Bouwman, H., Govender, D., Ishizuka, M., Ikenaka, Y., Yohannes, Y. B., et al. (2021). Levels of DDTs and other organochlorine pesticides in healthy wild Nile crocodiles (Crocodylus niloticus) from a flagship conservation area. Chemosphere 264, 128368. doi:10.1016/j.chemosphere.2020.128368
Greig, D. J., Ylitalo, G. M., Hall, A. J., Fauquier, D. A., and Gulland, F. M. D. (2007). Transplacental transfer of organochlorines in California sea lions (Zalophus californianus). Environ. Toxicol. Chem. 26, 37. doi:10.1897/05-609R.1
Hall, A. J., McConnell, B. J., Rowles, T. K., Aguilar, A., Borrell, A., Schwacke, L., et al. (2006). Individual-based model framework to assess population consequences of polychlorinated biphenyl exposure in bottlenose dolphins. Environ. Health Perspect. 114 (1), 60–64. doi:10.1289/ehp.8053
Harley, J. R., Bammler, T. K., Farin, F. M., Beyer, R. P., Kavanagh, T. J., Dunlap, K. L., et al. (2016). Using domestic and free-ranging arctic canid models for environmental molecular toxicology research. Environ. Sci. Technol. 50, 1990–1999. doi:10.1021/acs.est.5b04396
Hayward, M. W., Henschel, P., O’Brien, J., Hofmeyr, M., Balme, G., Kerley, G. I. H., et al. (2006). Prey preferences of the leopard (Panthera pardus). J. Zoology 270, 298–313. doi:10.1111/j.1469-7998.2006.00139.x
Hop, H., Borgå, K., Gabrielsen, G. W., Kleivane, L., and Skaare, J. U. (2002). Food web magnification of persistent organic pollutants in poikilotherms and homeotherms from the Barents Sea. Environ. Sci. Technol. 36, 2589–2597. doi:10.1021/es010231l
Hoshi, H., Minamoto, N., Iwata, H., Shiraki, K., Tatsukawa, R., Tanabe, S., et al. (1998). Organochlorine pesticides and polychlorinated biphenyl congeners in wild terrestrial mammals and birds from Chubu region, Japan: Interspecies comparison of the residue levels and compositions. Chemosphere 36, 3211–3221. doi:10.1016/S0045-6535(98)00011-3
Jaspers, V. L. B., Dietz, R., Sonne, C., Letcher, R. J., Eens, M., Neels, H., et al. (2010). A screening of persistent organohalogenated contaminants in hair of East Greenland polar bears. Sci. Total Environ. 408, 5613–5618. doi:10.1016/j.scitotenv.2010.07.059
Keller, J. M., Kucklick, J. R., Harms, C. A., and McClellan-Green, P. D. (2004). Organochlorine contaminants in sea turtles: Correlations between whole blood and fat. Environ. Toxicol. Chem. 23, 726. doi:10.1897/03-254
Leighton, G. R. M., Bishop, J. M., Camarero, P. R., Mateo, R., O'Riain, M. J., Serieys, L. E. K., et al. (2022). Poisoned chalice: Use of transformed landscapes associated with increased persistent organic pollutant concentrations and potential immune effects for an adaptable carnivore. Sci. Total Environ. 822, 153581. doi:10.1016/j.scitotenv.2022.153581
López, R., Goñi, F., Etxandia, A., and Millán, E. (2007). Determination of organochlorine pesticides and polychlorinated biphenyls in human serum using headspace solid-phase microextraction and gas chromatography-electron capture detection. J. Chromatogr. B 846, 298–305. doi:10.1016/j.jchromb.2006.09.009
Maheshwari, A. (2006). Food habits and prey abundance of leopard (Panthera pardus fusca) in gir national Park and wildlife sanctuary. Aligarh, India: Aligarh Muslim University.
Malarvannan, G., Poma, G., and Covaci, A. (2020). Interspecies comparison of the residue levels and profiles of persistent organic pollutants in terrestrial top predators. Environ. Res. 183, 109187. doi:10.1016/j.envres.2020.109187
Mason, C. F., Ford, T. C., and Last, N. I. (1986). Organochlorine residues in British otters. Bull. Environ. Contam. Toxicol. 36, 656–661. doi:10.1007/bf01623565
Mateo, R., Millán, J., Rodríguez-Estival, J., Camarero, P. R., Palomares, F., Ortiz-Santaliestra, M. E., et al. (2012). Levels of organochlorine pesticides and polychlorinated biphenyls in the critically endangered Iberian lynx and other sympatric carnivores in Spain. Chemosphere 86, 691–700. doi:10.1016/j.chemosphere.2011.10.037
McKinney, M. A., Iverson, S. J., Fisk, A. T., Sonne, C., Rigét, F. F., Letcher, R. J., et al. (2013). Global change effects on the long-term feeding ecology and contaminant exposures of East Greenland polar bears. Glob. Chang. Biol. 19, 2360–2372. doi:10.1111/gcb.12241
McKinney, M. A., Peacock, E., and Letcher, R. J. (2009). Sea ice-associated diet change increases the levels of chlorinated and brominated contaminants in polar bears. Environ. Sci. Technol. 43, 4334–4339. doi:10.1021/es900471g
Müller, C. E., De Silva, O. A., Small, J., Williamson, M., Wang, X., Morris, A., et al. (2011). Biomagnification of perfluorinated compounds in a remote terrestrial food chain: Lichen-Caribou-Wolf. Environ. Sci. Technol. 45, 8665–8673. doi:10.1021/es201353v
Norton, P. M., Lawson, A. B., Henley, S. R., and Avaery, G. (1986). Prey of leopards in four mountainous areas of the south Western Cape Province. S. Afr. J. Wildl. Res. 16, 47–52.
Polder, A., Skåre, J. U., Tryland, M., Ropstad, E., Gabrielsen, G. W., Vikøren, T., et al. (2009). Screening of halogenated organic compounds (HOCs) in wild living terrestrial mammals in Svalbard, Norway and Northern Sweden. Statlig program for forurensningsovervåking SPFO-report 1064/2009. Oslo, Norway: Norwegian School of Veterinary Science.
Quinn, L., Polder, A., Roos, C., Kylin, H., Løken, K. B., Skaare, J. U., et al. (2011). Levels and implications of persistent organic pollutants and other contaminants in South Africa: Results from the “LIPOPSA” project. DiVA 73, 1–4.
Ray, J. C., Hunter, L., and Zigouris, J. (2005). Setting conservation and research priorities for larger African carnivores. New York: Wildlife Conservation Society.
Rodríguez-Jorquera, I. A., Vitale, N., Garner, L., Perez-Venegas, D. J., Galbán-Malagón, C. J., Duque-Wilkens, N., et al. (2017). Contamination of the upper class: Occurrence and Effects of chemical pollutants in terrestrial top predators. Curr. Pollut. Rep. 3, 206–219. doi:10.1007/s40726-017-0061-9
Romanić, S. H., Klinčić, D., Kljaković-Gašpić, Z., Kusak, J., Reljić, S., Huber, D., et al. (2015). Organochlorine pesticides and polychlorinated biphenyl congeners in wild terrestrial mammals from Croatia: Interspecies comparison of residue levels and compositions. Chemosphere 137, 52–58. doi:10.1016/j.chemosphere.2015.05.026
Rose, N. L., Milner, A. M., Fitchett, J. M., Langerman, K. E., Yang, H., Turner, S. D., et al. (2020). Natural archives of long-range transported contamination at the remote lake Letšeng-la Letsie, Maloti Mountains, Lesotho. Sci. Total Environ. 737, 139642. doi:10.1016/j.scitotenv.2020.139642
Sarna, T., Swartz, H. M., and Zadlo, A. (2022). Interaction of melanin with metal ions modulates their cytotoxic potential. Appl. Magn. Reson. 53, 105–121. doi:10.1007/s00723-021-01386-3
Shore, R. F., Casulli, A., Bologov, V., Wienburg, C. L., Afsar, A., Toyne, P., et al. (2001). Organochlorine pesticide, polychlorinated biphenyl and heavy metal concentrations in wolves (Canis lupus L. 1758) from north-west Russia. Sci. Total Environ. 280, 45–54. doi:10.1016/S0048-9697(01)00802-6
Skaare, J. U., Bernhoft, A., Derocher, A., Gabrielsen, G. W., Goksøyr, A., Henriksen, E., et al. (2000). Organochlorines in top predators at Svalbard - occurrence, levels and effects. Toxicol. Lett. 112-113, 103–109. doi:10.1016/S0378-4274(99)00256-8
Skaare, J. U., Bernhoft, A., Wiig, Ø., Norum, K. R., Haug, E., Eide, D. M., et al. (2001). Relationships between plasma levels of organochlorines, retinol and thyroid hormones from polar bears (Ursus maritimus) at Svalbard. J. Toxicol. Environ. Health A 62, 227–241. doi:10.1080/009841001459397
Sonne, C. (2010). Health effects from long-range transported contaminants in Arctic top predators: An integrated review based on studies of polar bears and relevant model species. Environ. Int. 36, 461–491. doi:10.1016/j.envint.2010.03.002
Spong, G., Johansson, M., and Björklund, M. (2000). High genetic variation in leopards indicates large and long-term stable effective population size. Mol. Ecol. 9, 1773–1782. doi:10.1046/j.1365-294x.2000.01067.x
Thompson, L. A., Darwish, W. S., Ikenaka, Y., Nakayama, S. M. M., Mizukawa, H., Ishizuka, M., et al. (2017b). Organochlorine pesticide contamination of foods in Africa: Incidence and public health significance. J. Vet. Med. Sci. 79, 751–764. doi:10.1292/jvms.16-0214
Thompson, L. A., Ikenaka, Y., Yohannes, Y. B., van Vuren, J. J., Wepener, V., Smit, N. J., et al. (2017a). Concentrations and human health risk assessment of DDT and its metabolites in free-range and commercial chicken products from KwaZulu-Natal, South Africa. Food Addit. Contam. Part A 34, 1959–1969. doi:10.1080/19440049.2017.1357209
Tomza-Marciniak, A., Marciniak, A., Pilarczyk, B., Prokulewicx, A., and Bąkowska, M. (2014). Interspecies comparison of chlorinated contaminant concentrations and profiles in wild terrestrial mammals from Northwest Poland. Arch. Environ. Contam. Toxicol. 66, 491–503. doi:10.1007/s00244-013-9989-y
Verhaert, V., Newmark, N., D’Hollander, W., Covaci, A., Vlok, W., Wepener, V., et al. (2017). Persistent organic pollutants in the Olifants River Basin, South Africa: Bioaccumulation and trophic transfer through a subtropical aquatic food web. Sci. Total Environ. 586, 792–806. doi:10.1016/j.scitotenv.2017.02.057
Viljoen, I. M., Bornman, R., and Bouwman, H. (2016). DDT exposure of frogs: A case study from Limpopo province, south Africa. Chemosphere 159, 335–341. doi:10.1016/j.chemosphere.2016.06.023
Volschenk, C. M., Gerber, R., Mkhonto, M. T., Ikenaka, Y., Yohannes, Y. B., Nakayama, S., et al. (2019). Bioaccumulation of persistent organic pollutants and their trophic transfer through the food web: Human health risks to the rural communities reliant on fish from South Africa's largest floodplain. Sci. Total Environ. 685, 1116–1126. doi:10.1016/j.scitotenv.2019.06.144
Wepener, V., and Chapman, P. M. (2012). South African ecotoxicology — Present status and future prognosis. Afr. J. Aquat. Sci. 37, 229–234. doi:10.2989/16085914.2012.717051
Wepener, V., Smit, N. J., Covaci, A., and Bervoets, L. (2012). Seasonal bioaccumulation of organohalogens in tigerfish, Hydrocynus vittatus castelnau, from lake pongolapoort, south Africa. Bull. Environ. Contam. Toxicol. 88, 277–282. doi:10.1007/s00128-011-0439-0
Wolmarans, N. J., Bervoets, L., Gerber, R., Yohannes, Y. B., Nakayama, S. M. M., Ikenaka, Y., et al. (2021). Bioaccumulation of DDT and other organochlorine pesticides in amphibians from two conservation areas within malaria risk regions of South Africa. Chemosphere 274, 129956. doi:10.1016/j.chemosphere.2021.129956
Yamamoto, M., Isobe, T., Hayashi, T., Yachimori, S., Nomiyama, K., and Tanabe, S. (2012). “Contamination status and accumulation features of organohalogen compounds in raccoon dog and masked palm civet,” in Interdisciplinary studies on environmental chemistry—environmental pollution and ecotoxicology. Editors M. Kawaguchi, K. Misaki, H. Sato, T. Yokokawa, and T. Itai. (Tokyo: Terrapub).
Keywords: persistent organic pollutants, DDT, HCH, wildlife ecotoxicology, leopard conservation
Citation: van As M, Smit NJ, Wolmarans NJ and Wepener V (2022) First record of organochlorine pesticides in blood of wild and captive African leopards, Panthera pardus pardus (Linnaeus, 1758). Front. Environ. Sci. 10:938453. doi: 10.3389/fenvs.2022.938453
Received: 07 May 2022; Accepted: 15 July 2022;
Published: 25 August 2022.
Edited by:
Guijin Su, Research Center for Eco-environmental Sciences (CAS), ChinaReviewed by:
Mengjing Wang, City University of Hong Kong, Hong Kong SAR, ChinaJuan Muñoz-Arnanz, Spanish National Research Council (CSIC), Spain
Copyright © 2022 van As, Smit, Wolmarans and Wepener. This is an open-access article distributed under the terms of the Creative Commons Attribution License (CC BY). The use, distribution or reproduction in other forums is permitted, provided the original author(s) and the copyright owner(s) are credited and that the original publication in this journal is cited, in accordance with accepted academic practice. No use, distribution or reproduction is permitted which does not comply with these terms.
*Correspondence: Nico J. Smit, bmljby5zbWl0QG53dS5hYy56YQ==