- 1Chemical Engineering, School of Engineering, University of KwaZulu-Natal, Durban, South Africa
- 2WASH R&D Centre, School of Engineering, University of KwaZulu-Natal, Durban, South Africa
Forward osmosis with an ammonium bicarbonate draw solution was investigated as a low energy non-sewered sanitation solution, to recover nutrients and water from source separated urine. Stored urine collected from Urine Diversion Dry Toilets in the eThekwini Municipality (Durban), South Africa was used as the feed solution. Water recoveries of up to 45.9% with water fluxes up to 6.0 L m−2 h−1 were achieved using undiluted stored urine over an 8-h operating period with a 2.5 M draw solution. Rejections of up to 95% for phosphates, 85% for nitrogen and chlorides, and 75% for potassium and sodium were achieved. Low fouling of the membrane was observed after multiple runs and cleaning the membrane by circulation of deionized water or by osmotic backwash was sufficient to recover >95% of the original water flux. Little irreversible fouling was detected, assumed to be caused by carbonate calcium scaling from SEM-EDX analysis. This study suggests that forward osmosis with an ammonium bicarbonate solution could be integrated as a closed loop nutrient recycling technology for source separated urine with the prospect of clean water and draw solution recovery that could use waste heat from, for example, fecal sludge combustion.
1 Introduction
The United Nations estimates that 46% of the global population lack safely managed sanitation (United Nations, 2020), due to the economic unfeasibility of a sewered wastewater treatment infrastructure in low-income countries where sewerage accounts for up to 84% of capital costs (Jung et al., 2018). In this circumstance, non-sewered sanitation alternatives exist, which act as containment for feces, urine and wash water. Eventually, this waste requires additional costly services such as emptying, transport and treatment for safe disposal and hence only 22% has been reported to be safely managed in the urban setting (Blackett et al., 2014). Such waste is rich in nutrients, organics and water, which provides direct opportunity for fertilizer, energy or water recovery, which could thereby present local prospects for an affordable sanitation chain (Onabanjo et al., 2016; Eshetu Moges et al., 2018; Larsen et al., 2021a).
With a world population estimated to reach 9.8 billion by 2030 (United Nations, 2017), demand for resources will increase proportionally. Particularly, fertilizers containing the macronutrients nitrogen, phosphorus and potassium (N, P, K respectively), will need to increase to ensure food security (Xie et al., 2016). Urine is a source for these nutrients and provides an alternative to synthesized nitrogenous fertilizer compounds from the Haber-Bosch process, potash from rock salt deposits geographically constrained to the Northern Hemisphere and phosphates from finite rock deposits of increasingly lower grade (Skorina and Allanore, 2015; Randall and Naidoo, 2018). Urine contains 80% and 50% of the polluting nutrients, nitrogen and phosphorus, in domestic wastewater, while contributing only 1% of volumetric flow (Larsen and Gujer, 1996). By containing urine’s abundant source of nutrients at 10–12 g/L nitrogen, 0.1–0.5 g/L phosphorous and 1.0–2.0 g/L potassium (Patel et al., 2020) through passive separation from feces at the toilet interface (source separation), the nutrient polluting potential of wastewater is reduced and contained in a smaller volume for potential recovery opportunities. In addition, solid-liquid separation is achieved which reduces dewatering requirements in the solids fraction and immediate fecal separation mitigates pathogenic risk for water recovery in the liquid fraction. As such, source separated urine has been identified to contribute to multiple Sustainable Development Goals (SDGs), including zero hunger (SDG 2), water and sanitation for all (SDG 6), and nutrient pollution mitigation (SDG 14) (Larsen et al., 2021a).
The recovery of nutrients from urine has been demonstrated by a range of physical, chemical, and biological processes (Larsen et al., 2021b). Although effective, such processes either possess are limited selectivity to one or two nutrients (precipitation, adsorption, ammonia stripping, membrane distillation, microbial fuel cells, microbial electrolysis cells); or are energetically demanding (reverse osmosis, nanofiltration); require a large footprint (algal growth); are limited by concentration factor (electrodialysis) or do not provide prospects for water recovery (ion exchange, heat drying, freeze drying) (Patel et al., 2020; Larsen et al., 2021b).
An alternative technology for concentrating multiple nutrients in urine is forward osmosis (FO) membrane filtration, which also provides prospects for water recovery, using analogous semipermeable reverse osmosis membranes, applied for potable water reuse from wastewater (Pype et al., 2016; Davey, Thomas and McAdam., 2022). The osmotic pressure differential between the feed solution (FS) and draw solution (DS) provides the driving force for separation, thus reducing energy requirements when compared to reverse osmosis. This also allows for a more fouling resilient process, as water transport occurs by diffusion instead of advection (Siddiqui et al., 2018).
Previous studies have investigated the use of FO for urine volume reduction, to decrease transport costs and protect nutrient sensitive environments such as caves during exploration (Nikiema et al., 2017; Engelhardt et al., 2020), or to recover nutrients such as ammonium (Engelhardt et al., 2019; Ray et al., 2020), urea (Engelhardt et al., 2019), nitrogen (Zhang et al., 2014; Nikiema et al., 2017; Volpin et al., 2018; Volpin et al., 2019a), phosphorus (Zhang et al., 2014; Volpin et al., 2018; Volpin et al., 2019b), and potassium (Zhang et al., 2014). Despite promising nutrient concentration and water flux results, previous studies have employed a DS where permeated water separation from the DS is challenging for clean water recovery (i.e. sodium chloride, glucose, fertilizer blend or brine). Instead, researchers have investigated the prospect of FO integrated with thermally driven MD, whereby the MD process reconcentrates the DS and generates clean water from low grade heat (Liu et al., 2016; Volpin et al., 2019a; Ray et al., 2019). Ammoniacal nitrogen co-transport from urine can however be a challenge for MD permeate quality as ammonia is highly volatile (vapour pressure of 7,500 mmHg compared to 24 mmHg of water at 25°C), and therefore at reduced permeate vapour pressures and high feed temperatures used for thermally driven processes, its transport is encouraged over water (El-Bourawi et al., 2007). Additional measures such as feed acidification to convert ammonia to non-volatile ammonium, thicker membranes and feed dilutions are then required to mitigate its transport (Volpin et al., 2019a; Ray et al., 2019). Ammonium bicarbonate as a DS could represent an alternative water recovery method without the need of a downstream membrane process, as it can be decomposed into ammonia and carbon dioxide by moderate heating (<60°C). Hence, a scenario could be depicted where urine is treated for nutrient and water recovery by FO and the ammonium bicarbonate DS is subsequently decomposed and recaptured to regenerate the DS for further urine filtration This is advantageous over conventional distillation and evaporation/alkaline dehydration with condensation processes as: 1) the FO membrane provides a selective barrier for water recovery, able to reject urine micropollutants and achieve compliance in high strength wastewaters (e.g., blackwater) (Valladares Linares et al., 2011; Davey, Thomas and McAdam, 2022) and 2) Heating is targeted at volatile gas decomposition, rather than water vapour recovery through evaporation and therefore requires less heat energy than the latent heat of vaporization. However, FO could also be integrated with such volume reducing thermal processes as a pre-treatment, to enhance water quality. Ammonium bicarbonate provides a cheaper solute than sodium chloride at a similar osmotic pressure (Johnson et al., 2018) and has been explored for seawater desalination (Feng et al., 2018), acid mine drainage treatment (Vital et al., 2018), textile wastewater treatment (Wang et al., 2020), among other possible applications. However, to the best of the authors’ knowledge the use of ammonium bicarbonate as a DS has not been reported for urine treatment.
South Africa provides an ideal case study for trialing FO with an ammonium bicarbonate DS. Firstly, source separation has been implemented as Urine Diversion Dry Toilets (UDDTs) in the communities without sewered sanitation access (Durban municipality). Secondly, during the storage of urine in UDDTs, urea hydrolysis occurs Eq (1) facilitated by the urease enzyme (Hellström et al., 1999). This results in ammonium being formed Eq (2) and a pH increase to > pH 8, thus converting ammonium to volatile free ammonia (pKa = 9.24).
Although nutrient concentrations are slightly lower in hydrolyzed urine compared to fresh (Larsen et al., 2021b), the removal of urea and free ammonia is beneficial for FO rejection capabilities. Both compounds are low molecular weight uncharged compounds which bypass the electrostatic repulsion mechanism of the membrane (Lee and Lueptow, 2001). Thirdly, South Africa persistently experiences low crop yields (i.e., maize) due to geographic constraints of nutrient limited soils and water scarcity (Choruma et al., 2021).
This study aims to assess the suitability of ammonium bicarbonate as an alternative FO DS for the recovery of water and nutrients from source separated urine from UDDTs in South Africa with the specific objectives: 1) to identify the optimum DS and FS concentrations for water flux; 2) to evaluate the rejection of key macronutrients (P, K, N) and 3) to determine fouling propensity and reversibility.
2 Materials and methods
2.1 Feed and draw solutions
Stored hydrolyzed urine (Table 1) collected from Urine Diverting Dry Toilet (UDDT) in the eThekwini Municipality in Durban, South Africa, was used in this study as the feedstock (ethical clearance: BREC/00002684/2021). Urine was also diluted to simulate typical flushing scenarios from urine diversion toilets where flush volumes range between 0.5 and 2 L (von Münch and Winker, 2011). Assuming a urine volume of ∼300 ml (Haylen et al., 1989), dilution factors of 3 and 6 were prepared accordingly using deionized water.
Ammonium bicarbonate DS were prepared for each test run using distilled water and analytical grade ammonium bicarbonate. Draw solution concentrations of 0.6, 1.0, 2.0, 2.2, and 2.5 M ammonium bicarbonate solution were used in this study.
2.2 Forward osmosis experimental setup
Thin film composite (TFC) membranes (OsMemTM TFC-ES, Hydration Technology Innovations) comprised of a polyamide active layer placed on the top of a thick microporous polysulfone support layer were employed in this study, due to their reported higher water fluxes and lower reverse solute flux (Kedwell et al., 2018; Almoalimi and Liu, 2022). The membrane supports a maximum operating temperature of 71°C and a maximum transmembrane pressure of 70 kPa. The pH of the solutions should be in the range of 2–11 and this high pH tolerance justified use of the TFC membrane for stored urine. Membrane sheets were immersed in deionized water before experiments as recommended by the membrane manufacturer. The membrane was supported in a SEPA crossflow membrane cell which allowed for tangential flow of both solutions (draw and feed) across the membrane. The cell was characterized by an effective filtration area of 140 cm2, and a channel width and depth of 95.25 mm and 1.91 mm, respectively.
The membranes were operated in the AL-FS (Active Layer facing Feed Solution) orientation as this configuration minimized reverse solute flux in comparison to AL-DS (Active Layer facing Draw Solution), according to a baseline test using a 0.36 M sodium chloride solution FS with a 2 M ammonium bicarbonate DS over a period of 4 h. The measurements indicated a reverse solute flux of 4 ± 0.3 and 7.1 ± 0.1 g of ammonium bicarbonate per L of permeated water across the membrane in the AL-FS and AL-DS mode, respectively.
Feed and DS (1 L starting volume) were circulated at a cross flow velocity of 0.05 m s−1 (Figure 1). The solutions were maintained at 22°C ± 0.1°C using a Grant TC120 thermostatic bath and continuously mixed with magnetic stirrers whilst sealed to ensure no loss of NH4-N as NH3 gas. The pH and conductivity of the FS as well as the pH of the DS were continuously measured through a probe Hach MM150, whilst the mass of both the feed and DS were measured on a Kern precision balance connected to a computer to log the data every two hours. Samples (5 ml) were taken every two hours during 8 h runs for chemical analysis. All FO runs were carried out in triplicate with new membranes for each run to evaluate the reproducibility of the results.
2.3 Chemical analysis
The following parameters were tracked during the FO runs: sodium (Na+), calcium (Ca2+), magnesium (Mg2+), potassium (K+), chloride (Cl−), sulfates (SO42-) total nitrogen (TN) and total phosphates (TP). Sodium and chloride concentrations allowed for the initial characterization of the membrane rejections during the baseline runs. Potassium, total phosphates and total nitrogen concentrations were monitored due to their significance as agricultural supplements, while calcium, magnesium and sulfates gave an indication of precipitation that would have occurred during hydrolysis of urine. The feed and draw solutions were analyzed by concentrations and volume over time to calculate a mass balance and evaluate membrane rejections. The ions concentration (Ca+, Na+, Mg2+, K+) were determined using an Agilent 4,200 Microwave-Plasma Atomic Emission Spectroscopy, whilst the total phosphates total nitrogen, ammonical nitrogen and sulfates were determined using a Merck Pharo 300 Spectroquant. Chloride analysis was carried out using a Sherwood Scientific 926 chloride analyzer.
2.4 Water flux, solute rejection, and osmotic pressure calculations
Water flux was calculated from the mass change of the FS over time, considering the density of water and area of the membrane Eq. (3):
Where Jw represents the water flux (L·m−2·h−1), ΔW is the mass change in FS (kg), ρ is the water density (kg·L−1), A is the membrane area (m2) and Δt is the time interval between measurements (h). Overall permeate recovery R to the DS was calculated as a volume percentage at the end of each run according to Eq (4):
Where Vp is the permeate volume (L) and Vf is the initial FS volume (L). Solute rejections were determined through Eq (5) based on the chemical analysis of the samples taken every two hours from the feed and DS.
Where R(ti) represents solute rejection at the time ti (-),
Where
Osmotic pressure of the feed and DS were determined from the measured concentrations of ionic species using PHREEQC™ software, which is a program based on the calculation of equilibrium chemistry of aqueous solutions.
2.5 Membrane cleaning and fouling evaluation
Comparison of the water flux through the virgin membrane, fouled membrane (after several FO runs with undiluted stored urine accumulating to 48 h and a processed flux of 108.6 L m−2) and cleaned membrane can be used to evaluate the fouling and flux recovery through cleaning. Two cleaning methods were evaluated to test the fouling reversibility of the membranes after a run: the circulation of deionized water at higher flow rates, and the utilization of an osmotic backwash.
The first method involved cleaning the used membrane by circulation of deionized water on both sides of the FO rig for 30 min. Osmotic backwashing was also conducted on fouled membranes, whereby ammonium bicarbonate was circulated on FS side and distilled water on the DS side. This configuration leads to the inverse of the osmotic pressure gradient across the membrane, leading to a water flow in opposite direction compared to the forward osmosis runs. This is expected to remove the foulant material deposited on the membrane surface. Osmotic backwashes were conducted with 1 and 2 M ammonium bicarbonate solutions for 30 and 60 min in each case (Kim et al., 2012). Prior to osmotic backwashing, all the feed and DS trapped within the system were drained to minimize the influence of residual salts on the osmotic pressure differential across the membrane. Care was taken to not disturb the membrane prior to the backwashing. On completion of the osmotic backwashing, the flux recovery was measured, to have an indication of the flux recovered and to compare with deionized water operation.
Virgin and cleaned membranes were observed using scanning electron microscopy (SEM) with a maximum resolution of 50 nm, to determine the deposition of foulants on the membrane after cleaning. This analysis was coupled with energy-dispersive X-ray spectroscopy (EDX) to perform chemical analyses on selected areas from the virgin and cleaned used membranes. Prior to the SEM observations, the membranes were dried at room temperature and then coated with a thin layer of platinum using a sputter coater (SC7620, Emitech, United Kingdom).
3 Results and discussion
3.1 Impact of draw and feed solutions on water flux
Feed and DS concentrations were investigated to identify conditions which improved water flux, for increased FS volume reduction and water recovery prospects. Ammonium bicarbonate DS ranging from 0.6 M to 2.5 M resulted in increasing water fluxes from 1.4 to 4.7 L m−2 h−1 (Figure 2), driven by a greater osmotic gradient between the feed and DS. It would be expected that this relationship increases linearly, however at 2 M the line gradient decreases from 2 to 0.7. This suggests that at DS concentration gradients greater than ∼2 M, the onset of internal dilutive concentration polarization (ICP) occurs, whereby water accumulates within the support layer of an asymmetric membrane which in turn reduces the draw solute concentration at the membrane (Johnson et al., 2018). Several authors have reported this phenomenon in literature (McCutcheon et al., 2006; Le and Nunes, 2016). Therefore, 2 M was considered as the optimum DS concentration to be taken forward for further assessment. This concentration is also similar to Liu et al. (2016) who reported a water flux of 5.2 L m−2 h−1 using 2 M sodium chloride compared to 4.6 L m−2 h−1 with ammonium bicarbonate in this study.
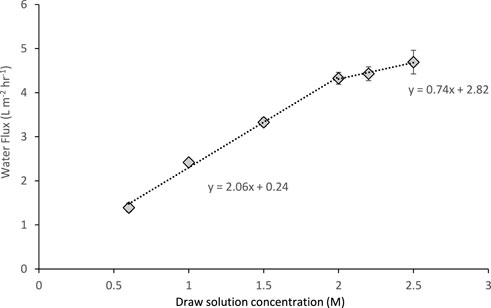
FIGURE 2. Influence of ammonium bicarbonate draw solution concentration on water flux using stored urine as a feed solution. Operated for 2 h at 22°C and a 0.05 m s−1 crossflow velocity. Error bars represent the standard deviation of a triplicated experiment.
As the experiments were operated in batch mode using 1 L draw and FS, water transport into the DS impacted the temporal water flux, due to the decline in osmotic gradient over time. Over an 8-h run using 2 M ammonium bicarbonate and stored urine, the transmembrane osmotic pressure decreased from 51.41 to 9.61 bar, which corresponded to water fluxes of 4.6 to 2.1 L m−2 h−1 (Figure 3). This decrease in the osmotic pressure gradient followed a logarithmic pattern, illustrating the declining rate in driving force and consequent water transport which is to be expected as the solutions verge towards equilibrium. Increasing the initial DS volume would prolong the solutions reaching equilibrium, thereby maintaining a higher osmotic pressure gradient/water flux and concentrating the FS at a faster rate. This attenuation of water flux decline by mitigating DS dilution was demonstrated by Xue et al. (2015), using a 2:1 DS:FS volume ratio with seawater and wastewater, achieving a 93% water reduction and a 10 fold concentration factor.
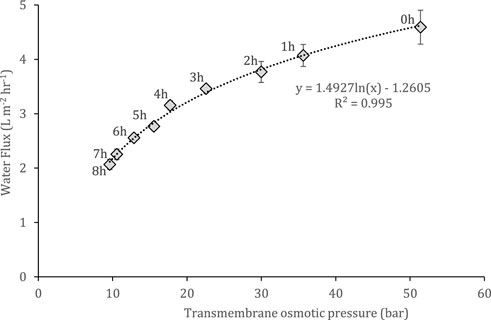
FIGURE 3. Temporal change in transmembrane osmotic pressure and its influence on water flux. Operated for 8 h at 22°C and a 0.05 m s−1 crossflow velocity. Feed and draw solutions are stored urine and 2 M ammonium bicarbonate respectively. Error bars represent the standard deviation of a triplicated experiment.
The implications of urine FS dilution with flush water were assessed in Figure 4. Greater dilution factors (DF) decrease urine osmolality, leading to an increase in transmembrane osmotic pressure. This results in a greater water flux where a DF of 6 reached 12.8 L m−2 h−1 compared to 4.6 L m−2 h−1 of undiluted urine, at the start of the run. Temporally, the water flux declines faster with increasing DF factors (linear gradients of −0.91, −0.51 and −0.31 for DF 6, DF 3 and DF 1 respectively, Figure 4). These results suggest that flush water is advantageous for FO favoring water recovery, however limits volume reduction and nutrient concentration due to the increased operational times required (Volpin et al., 2019b). In addition, a higher amount of energy will be required in the regeneration step of the draw solution. Indeed, a higher volume of water on the draw solution side will need more thermal energy for its heating to the temperatures where the ammonium bicarbonate decomposes into carbon dioxide and ammonium (<60°C).
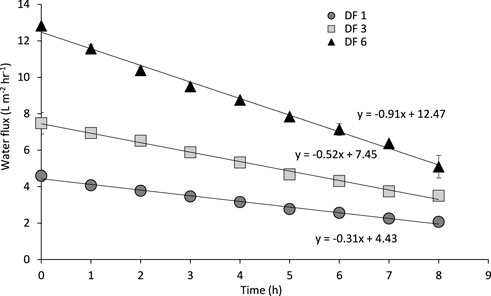
FIGURE 4. Impact of urine feed dilution on water flux over time. Urine was either undiluted (DF 1) or diluted by a factor of 3 (DF 3) or 6 (DF 6). Operated for 8 h at 22°C and a 0.05 m s−1 crossflow velocity. Feed and draw solutions are stored urine and 2 M ammonium bicarbonate respectively. Error bars represent the standard deviation of a triplicated experiment.
3.2 Rejection of key nutrients
The impact of water flux on key nutrient rejection was assessed on undiluted urine to understand the potential of combined water and nutrient recovery (Figure 5). Within an 8-h operating period, the membrane demonstrated high rejections of total phosphates (TP, 84%–94%), total nitrogen (TN, 72%–85%) and chloride (Cl−, 70%–85%), followed by potassium (K+, 39%–71%) and sodium (Na+, 32%–68 %). It is also important to note that the less critical ions Ca2+ and SO42+ and CO32- were completely rejected during the run. The observed high rejections for TP and Cl− could be attributed to their ability to retard the reverse permeation of the negatively charged bicarbonate ions from the DS, resulting in electrostatic repulsion as also reported by Zhang et al. (2014), Liu et al. (2016), and Volpin et al. (2018). The PO43− ion was particularly retained due to its larger size (molecular weight of 95 g mol-1 compared to 35 g mol-1 of Cl−) aiding to steric hindrance associated to reduced membrane permeability (Zhang et al., 2014).
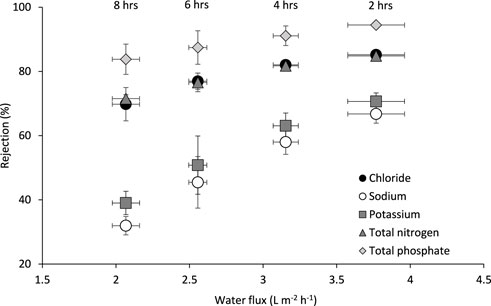
FIGURE 5. Relationship between water flux and nutrient rejection using stored urine and 2 M ammonium bicarbonate as the feed and draw solutions respectively. Operated for 8 h at 22°C and a 0.05 m s−1 crossflow velocity. Error bars represent the standard deviation of a triplicated experiment.
The membrane exhibited lower rejections of Na+ and K+, compared to Cl−, TN, and TP. A possible explanation for the low rejections of Na+ and K+ might be the fact that the membrane might have assumed a more negative charge as the pH of the urine feed stock increased during the FO process. The increase in pH (pH 7.5–8.4) could be attributed to the back diffusion of the ammonium bicarbonate draw solutes. The baseline tests (0.36 M NaCl FS and 2 M NH4HCO3 DS) indicated an average reverse solute flux of 4 ± 0.3 g of ammonium bicarbonate per litre of permeated water. The assumed negative charge would have attracted more positive ions towards the membrane (higher partitioning) resulting in higher permeation rates towards the DS as also observed by Zhang et al. (2014). Potassium also has a high permeability due to a smaller hydrated radius than PO43−, which has prevented high rejection in literature (Zhang et al., 2014; Ray et al., 2020). For the same reason, the small solute size of sodium has proven to be problematic as a NaCl DS, resulting in large reverse solute fluxes (Johnson et al., 2018).
Total nitrogen exists predominantly as ammonium ions in the stored hydrolyzed urine as the mean pH of the hydrolysed urine was pH 8 (Table 1), leading to only 4.39% ammonia because of a pKa of 9.34 at 22°C (Emerson et al., 1975). Therefore, following the explanations for the observed high rejections for negative ions and low rejections for positive ions, coupled with being the smallest ion (molecular weight = 18 g mol−1), we could expect the TN rejections to be low. However, the rejections are higher than that of K+ and Na+. This could be attributed to the reduced mass transfer gradient that exists between the feed and DS with respect to the ammonium. The DS (ammonium bicarbonate) contains more ammonium ions than the stored urine, resulting in a reduced mass transfer force for ammonium diffusion from the feed to the DS, hence the observed higher rejections compared to the other positively charged ions. This phenomenon explains why Na+, Cl− and K+ rejections are higher in other studies using NaCl and KH₂PO₄ as respective DS (Ray et al., 2020). Back diffusion could also be contributing to the enhanced TN FS concentrations (4 ± 0.3 g of ammonium bicarbonate per litre of permeated water). Therefore, the use of ammonium bicarbonate as a DS is particularly advantageous as a method for enhanced TN nutrient recovery, where TN is usually problematic to retain (Patel et al., 2020).
Generally, the rejections of nutrients declined with the water flux which decreased over time. This can be accounted for by the classical solution-diffusion theory, whereby the solute concentration gradient governs mass transfer. In the case of AL-FS FO, an increased co-transport of water dilutes the concentration of nutrients at the membrane interface (active layer and porous support) which results in a higher nutrient rejection (Zhang et al., 2014). The Na+ and K+ rejections decline the fastest with decreasing the water flux, which could be linked to the effect of their positive charge enhancing solute permeability compared to the negatively charged solutes.
3.3 Membrane fouling characterization and mitigation
Two in situ cleaning methods were evaluated for cleaning membranes to restore water flux after the same membrane sample was exposed to several forward osmosis runs with undiluted stored urine accumulating to 48 h and a processed flux of 108.6 L m−2: deionized water circulation (Figure 6) and osmotic backwashing (Figure 7). The fluxes were determined over 2 h with deionized water and 2 M ammonium bicarbonate as the FS and DS respectively. After circulating deionized water for 30 min, a fouled membrane with a flux reduced to ∼83% could be recovered to ∼95% of the flux using a virgin membrane (Figure 6).
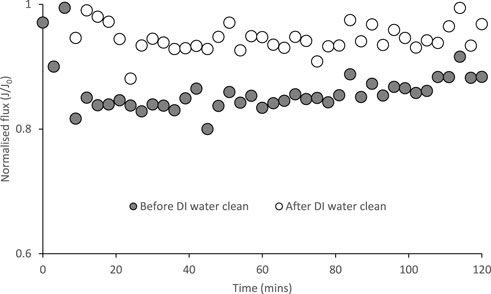
FIGURE 6. Normalised water flux with a virgin membrane (J0) with respect to a used membrane (J) before and after membrane cleaning with deionized water. Operated for 2 h at 22°C and a 0.05 m s−1 crossflow velocity. Feed and draw solutions are deionized water and 2 M ammonium bicarbonate respectively.
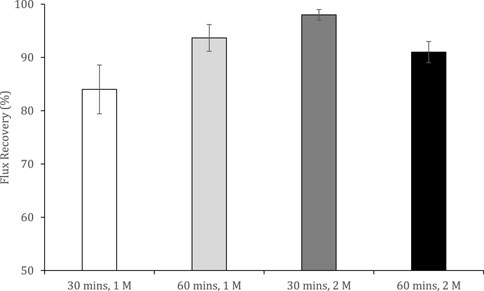
FIGURE 7. Flux recoveries observed after osmotic backwashing for 30 and 60 min, with 1 and 2 M ammonium bicarbonate as the feed solution and deionized water as the draw solution. Operated for 2 h at 22°C and a 0.05 m s−1 crossflow velocity. Error bars represent the standard deviation of a triplicated experiment.
For the osmotic backwash cleaning method which is particularly effective in controlling particulate and organic fouling (Kim et al., 2012), the duration and DS concentration were evaluated. The flux recovery improved by increasing the osmotic gradient between the feed and DS (i.e., by increasing ammonium bicarbonate concentration), and by increasing the duration of the osmotic backwash (only at 1 M DS concentration). An increase in the osmotic gradient results in a greater reverse water flux that removes foulants from the membrane which achieved ∼98% flux recovery at 2 M for 30 min. However, an unexpected decline in flux recovery during the fourth osmotic backwash after increasing the backwashing time to 60 min and ammonium bicarbonate concentration to 2 M was observed. This limit was also documented by Daly and Semiao (2020), who reported adhesive forces acting between the fouling layer and DS at high concentrations.
The foulants which could not be removed from DI water circulation and osmotic backwashing cleaning methods were examined using SEM and EDX analysis (Figure 8). The virgin membrane (Figures 8A,B) exhibited peaks of carbon, sulphur, and oxygen, which are the constituent elements of the membrane. Carbon has the highest content as it forms the backbone of the membrane structure. The membrane is composed of a TFC polyamide on polysulfone with an embedded polyester screen, which explains the O and S peaks. Through SEM observations, it was noted that some particulates remained linked to the membrane surface after cleaning (Figure 8C). Achilli et al. (2010), emphasized that the probability of mineral scale occurring on the membrane surface is enhanced when the FS is concentrated above the solubility limits of various water-soluble minerals. The FS for this work contained scale precursors such as magnesium, calcium, sulfate and bicarbonate ions. EDX analysis of the fouled membrane (Figure 8D) exhibited prevalent peaks of calcium, suggesting irreversible fouling by the deposition of bicarbonate on the membrane. In addition, sulfate fouling could occur, as this compound was completely rejected by the membrane and therefore prone to exceeding the solubility limits, causing scaling. However, the impact of irreversible foulants on the membrane was negligible for flux reduction during this study (3%–5% irreversible decline in flux), illustrating the low fouling propensity of the FO membranes and the ease of in situ membrane cleaning, which can significantly reduce the operating costs of the process.
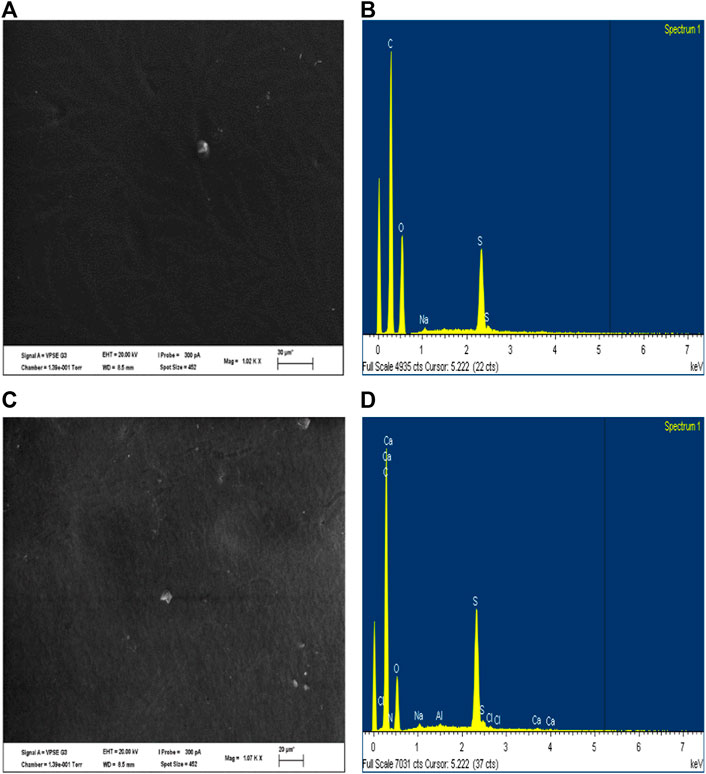
FIGURE 8. SEM observation and EDX analysis of the (A,B) virgin membrane and (C,D) fouled membrane after cleaning.
4 Outlook—Prospects of draw solution regeneration and water recovery
The decomposition of ammonium bicarbonate solution upon moderate heating (<60°C) to yield ammonia and carbon dioxide gases and a water product can be summarized by the following equation:
The use of a distillation column to initiate volatile compound separation and the diluted DS to reabsorb the gases downstream is a simple and proven method for the removal and recovery of volatile DS for seawater desalination applications. (Kim et al., 2015). Pilot scale results from a desalination plant that utilized ammonium bicarbonate as a DS indicated that 265–300 kWh of thermal energy was needed to produce 1000 L of water (McGinnis et al., 2013; Kim et al., 2015). In the case of urine treatment, a low-grade heat source will be therefore enough to provide the thermal energy required to decompose ammonium bicarbonate in the draw solution. Different low-cost energy source options could provide the energy for the draw solution regeneration. One alternative could be using the faecal sludge from UDDTs (solid fraction) as biofuel.
Based on an individual’s average daily defecation and urination (Rose et al., 2015), the faecal sludge and urine production rates in a UDDT would be 128 g wet solid user−1 day −1 (or 32 g dry solid user−1 day−1 considering the average moisture content of faeces of 75% and 1.4 L user−1 day−1, respectively (Rose et al., 2015). Assuming that the UDDT serves one individual, the annual faecal sludge and urine production will be around 12 kg dry solid and 500 L, respectively. Considering that the urine will be treated by a forward osmosis process with a water recovery of 50% (as observed after an 8-h run with the 2.5 M ammonium bicarbonate draw solution), an energy amount of 240–270 MJ would be required to regenerate the draw solution (based on the estimated thermal energy required to produce 1,000 L of water). Considering a calorific value of around 15 MJ kg−1 dry solids (Getahun, et al., 2020), combusting the UDDT sludge generated will liberate 175 MJ, which could cover part of the energy required for the regeneration of the draw solution.
Therefore, the solid fraction from source-separated toilets can be utilized as a fuel to decompose ammonium bicarbonate and ensure a closed loop in the recycling of the DS, which allows for an economically feasible process. However, additional energy may be required to fully regenerate the ammonium bicarbonate, which could be brought by adding other types of biomass or organic wastes to the sludge or by solar thermal energy. For example, co-incinerating the faecal sludge with 4 kg of food waste with a calorific value of approximately 23 MJ/kg (Ouadi et al., 2019), or adding a 0.1 m2 solar water heater (typically yielding 450 kWh/m2 of thermal energy (Zukowski et al., 2021)), to the system could suffice to provide the energy to decompose the ammonium carbonate in the draw solution and generate 250 L of reuse water per year and per person in the studied scenario.
When comparing forward osmosis with an ammonium bicarbonate DS to other urine volume reducing processes, the energy consumption of the forward osmosis is lower than direct urine evaporation. For example, the evaporation of 250 L of water from urine will require a theoretical energy consumption of 565 MJ, which is distinctly higher than the energy required to recover the same amount of water from the forward osmosis process. FO also provides an additional passive selective barrier process for enhanced water quality. Reverse osmosis has a notably lower specific energy consumption for seawater desalination (2.5–4.0 kWh per 1000 L of produced water (Kim et al., 2019)) than forward osmosis. Nonetheless, reverse osmosis consumes energy in the form of electricity, requiring grid connection or a renewable power source (photovoltaic cells, wind turbines). In contrast, most of the energy consumption in the case of a forward osmosis setup would be in the form of heat, with a minimal need for electricity to run low-pressure pumps. The thermal energy requirements in a forward osmosis process could be easily supplied by the incineration of waste, allowing for the integration of a waste management component to urine treatment, and by solar thermal collectors, which are simpler to implement and lower cost than photovoltaic systems. Another advantage of forward osmosis is its robustness to fouling, as it would not necessitate urine pre-treatment and can handle higher salinity levels. Therefore, forward osmosis could be more suitable than reverse osmosis (particularly in South African where electricity interruptions are frequent), which is currently the desalination gold standard process.
With regards to water quality for recovered water (Table 2), the permeate already meets compliance to key chemical parameters (TP, TN and pH) stated in the ISO 30500 standard for non-sewered sanitation systems (International Organization for Standardization, 2018). Although not measured in this study, it can also be assumed that organics and faecal coliforms (also included in the standard), would be sufficiently rejected by FO due to the proven rejection of urine organics in literature (>97% rejection of total organic carbon, Liu et al., 2016), and dense membrane providing an absolute barrier to pathogens in a naturally sterile liquid.
5 Conclusion
This study evaluated the use of a novel volatile DS (ammonium bicarbonate) for the sustainable treatment of source separated urine with prospects of nutrient, water and DS regeneration. Ammonium bicarbonate as a DS for FO evidenced comparable water fluxes to literature using conventional NaCl (Liu et al., 2016). Up to 6 L m−2 h−1 was achieved with a FS of stored urine and a DS of 2.5 M ammonium bicarbonate, representing water recoveries of up to 46% by volume after 8 h. This allows for a significant volume reduction in the treated urine and the potential for recovery of reuse water through further processing of the DS. The water flux increased with urine DF or increased DS concentrations, due to greater osmotic pressure gradients. Internal concentration polarization was observed at DS concentrations >2 M, therefore operation using 2 M is advised to maximize water recovery efficiency.
Rejections of up to 95% of total phosphates, 85% of total nitrogen and chloride, and 70% of potassium and sodium were achieved. This would allow generating a concentrated FS rich in nutrients that could be used as fertilizer. The rejections were influenced by temporally reducing water fluxes. However, this could be controlled through increasing the DS volume in batch mode, thereby prolonging the osmotic gradient from reaching equilibrium. Ammonium in the DS also acted to retard the mass transfer of ammonium in the urine feed by reversing the concentration gradient, thereby enhancing rejection of the typically problematic nitrogenous compounds.
Forward osmosis exhibited a low fouling propensity with a water flux decrease of approximately 20% after a few uses. In situ membrane cleaning through circulation of deionized water on both the feed and draw sides could restore up to 95 % of the water flux for a fouled membrane, whilst osmotic backwashing of the membrane could achieve up to 98% flux recovery, with calcium, bicarbonate and sulfate detected as the causes of irreversible fouling.
This study has evidenced that an ammonium bicarbonate DS provides similar results to other well researched DS, for the treatment of stored urine using FO. However, its use provides additional benefits such as enhanced TN rejection and the prospect of closed loop nutrient recovery, water recovery and DS regeneration using low grade heat from fecal sludge combustion. Further research is required to develop a closed loop system which assesses recovered water quality against ISO 30500 (International Organization for Standardization, 2018) and integrates fecal combustion for DS regeneration. Long term trials are also warranted to assess the impacts of the organic and inorganic fractions of urine.
Data availability statement
The raw data supporting the conclusions of this article will be made available by the authors, without undue reservation.
Ethics statement
The studies involving human participants were reviewed and approved by Biomedical Research Ethics Committee: BREC/00002684/2021. The patients/participants provided their written informed consent to participate in this study.
Author contributions
PJ, VK, SS and BA contributed to conception and design of the study. MA conducted the experiments. MA and ME performed data analysis. MA wrote the first draft of the manuscript. PJ, ME, VK, SS, and BA wrote sections of the manuscript. All authors contributed to manuscript revision, read, and approved the submitted version.
Funding
This publication is based on research funded by the Bill & Melinda Gates Foundation (Grant number: OPP1069575) which includes open access publication fees.
Conflict of interest
The authors declare that the research was conducted in the absence of any commercial or financial relationships that could be construed as a potential conflict of interest.
Publisher’s note
All claims expressed in this article are solely those of the authors and do not necessarily represent those of their affiliated organizations, or those of the publisher, the editors and the reviewers. Any product that may be evaluated in this article, or claim that may be made by its manufacturer, is not guaranteed or endorsed by the publisher.
References
Abousnina, R. M., and Nghiem, L. D. (2014). Removal of dissolved organics from produced water by forward osmosis. Desalination Water Treat. 52 (4–6), 570–579. doi:10.1080/19443994.2013.827292
Achilli, A., Cath, T. Y., and Childress, A. E. (2010). Selection of inorganic-based draw solutions for forward osmosis applications. J. Memb. Sci. 364 (1), 233–241. doi:10.1016/j.memsci.2010.08.010
Almoalimi, K., and Liu, Y. Q. (2022). Enhancing ammonium rejection in forward osmosis for wastewater treatment by minimizing cation exchange. J. Memb. Sci. 648, 120365. doi:10.1016/J.MEMSCI.2022.120365
Blackett, I., Hawkins, P., and Heymans, C. (2014). The missing link in sanitation service delivery: a review of faecal sludge management in 12 cities. Washington D.C: World Bank Group.
Choruma, D. J., Balkovic, J., Pietsch, S. A., and Odume, O. N. (2021). Using EPIC to simulate the effects of different irrigation and fertilizer levels on maize yield in the Eastern Cape, South Africa. Agric. Water Manag. 254, 106974. doi:10.1016/j.agwat.2021.106974
Daly, S., and Semiao, A. J. C. (2020). Mechanisms involved in osmotic backwashing of fouled forward osmosis (FO) membranes. J. Membr. Sci. Res. 6 (2), 158–167. doi:10.22079/jmsr.2020.118843.1315
Davey, C. J., Thomas, N., and McAdam, E. J. (2022). Downscaling reverse osmosis for single-household wastewater reuse: towards low-cost decentralised sanitation through a batch open-loop configuration. J. Water Reuse Desalination 12, 191–205. doi:10.2166/wrd.2022.084
El-Bourawi, M. S., Khayet, M., Ma, R., Ding, Z., Li, Z., Zhang, X., et al. (2007). Application of vacuum membrane distillation for ammonia removal. J. Membr. Sci. 301 (1–2), 200–209. doi:10.1016/j.memsci.2007.06.021
Emerson, K., Russo, R. C., Lund, R. E., and Thurston, R. V. (1975). Aqueous ammonia equilibrium calculations: effect of pH and temperature. J. Fish. Res. Bd. Can. 32 (12), 2379–2383. doi:10.1139/f75-274
Engelhardt, S., Bender, K., Vogel, J., Duirk, S., Moore, F., and Barton, H. (2020). Urine volume reduction during long-duration cave exploration by a light-weight and portable forward osmosis system. Int. J. Speleol. 49, 229–234. doi:10.5038/1827-806X.49.3.2336
Engelhardt, S., Vogel, J., Duirk, S. E., Moore, F. B., and Barton, H. A. (2019). Urea and ammonium rejection by an aquaporin-based hollow fiber membrane. J. Water Process Eng. 32, 100903. doi:10.1016/j.jwpe.2019.100903
Eshetu Moges, M., Todt, D., and Heistad, A. (2018). Treatment of source-separated blackwater: a decentralized strategy for nutrient recovery towards a circular economy. Water 104, 463. doi:10.3390/w10040463
Feng, L., Xie, L., Suo, G., Shao, X., and Dong, T. (2018). Influence of temperature on the performance of forward osmosis using ammonium bicarbonate as draw solute. Trans. Tianjin Univ. 24 (6), 571–579. doi:10.1007/s12209-018-0159-1
Getahun, S., Septien, S., Mata, J., Somorin, T., Mabbett, I., Buckley, C., et al. (2020). Drying characteristics of faecal sludge from different on-site sanitation facilities. J. Environ. Manage. 261, 110267. doi:10.1016/j.jenvman.2020.110267
Haylen, B. T., Ashby, D., Sutherst, J. R., Frazer, M. I., and West, C. R. (1989). Maximum and average urine flow rates in normal male and female populations-the liverposl nomograms. Br. J. Urol. 64, 30–38. doi:10.1111/j.1464-410x.1989.tb05518.x
Hellström, D., Johansson, E., and Grennberg, K. (1999). Storage of human urine: acidification as a method to inhibit decomposition of urea. Ecol. Eng. 12 (3), 253–269. doi:10.1016/S0925-8574(98)00074-3
International Organization for Standardization (2018). Non‒sewered sanitation systems–Prefabricated integrated treatment units–General safety and performance requirements for design and testing (ISO 30500: 2018 (E)). Available at: https://www.iso.org/standard/72523.html.
Johnson, D. J., Suwaileh, W. A., Mohammed, A. W., and Hilal, N. (2018). Osmotic’s potential: an overview of draw solutes for forward osmosis. Desalination 434, 100–120. doi:10.1016/j.desal.2017.09.017
Jung, Y. T., Narayanan, N. C., and Cheng, Y.-L. (2018). Cost comparison of centralized and decentralized wastewater management systems using optimization model. J. Environ. Manage. 213, 90–97. doi:10.1016/j.jenvman.2018.01.081
Kedwell, K. C., Quist-Jensen, C., Giannakikis, G., and Christensen, M. L. (2018). Forward osmosis with high-performing TFC membranes for concentration of digester centrate prior to phosphorus recovery. Sep. Purif. Technol. 197, 449–456. doi:10.1016/j.seppur.2018.01.034
Kim, C., Lee, S., and Hong, S. (2012). Application of osmotic backwashing in forward osmosis: mechanisms and factors involved. Desalination Water Treat. 43 (1–3), 314–322. doi:10.1080/19443994.2012.672215
Kim, J., Park, K., Yang, D. R., and Hong, S. (2019). A comprehensive review of energy consumption of seawater reverse osmosis desalination plants. Appl. Energy 254, 113652. doi:10.1016/j.apenergy.2019.113652
Kim, Y., Lee, J. H., Kim, Y. C., Lee, K. H., Park, I. S., Park, S.-J., et al. (2015). Operation and simulation of pilot-scale forward osmosis desalination with ammonium bicarbonate. Chem. Eng. Res. Des. 94, 390–395. doi:10.1016/j.cherd.2014.08.015
Larsen, T. A., Gruendl, H., and Binz, C. (2021a). The potential contribution of urine source separation to the SDG agenda – A review of the progress so far and future development options. Environ. Sci. Water Res. Technol. 7 (7), 1161–1176. doi:10.1039/D0EW01064B
Larsen, T. A., Riechmann, M. E., and Udert, K. M. (2021b). State of the art of urine treatment technologies: a critical review. Water Res. X 13, 100114. doi:10.1016/j.wroa.2021.100114
Larsen, T., and Gujer, W. (1996). Separate management of anthropogenic nutrient solutions (human urine). Water Sci. Technol. 34 (3–4), 87–94. doi:10.2166/wst.1996.0420
Le, N. L., and Nunes, S. P. (2016). Materials and membrane technologies for water and energy sustainability. Sustain. Mater. Technol. 7, 1–28. doi:10.1016/j.susmat.2016.02.001
Lee, S., and Lueptow, R. M. (2001). Membrane rejection of nitrogen compounds. Environ. Sci. Technol. 35, 3008–3018. doi:10.1021/es0018724
Liu, Q., Liu, C., Zhao, L., Ma, W., Liu, H., Ma, J., et al. (2016). Integrated forward osmosis-membrane distillation process for human urine treatment. Water Res. 91, 45–54. doi:10.1016/j.watres.2015.12.045
McCutcheon, J. R., McGinnis, R. L., and Elimelech, M. (2006). Desalination by ammonia–carbon dioxide forward osmosis: influence of draw and feed solution concentrations on process performance. J. Memb. Sci. 278 (1), 114–123. doi:10.1016/j.memsci.2005.10.048
McGinnis, R. L., Hancock, N. T., Nowosielski-Slepowron, M. S., and Mcgurgan, G. (2013). Pilot demonstration of the NH3/CO2 forward osmosis desalination process on high salinity brines. Desalination 312, 67–74. doi:10.1016/j.desal.2012.11.032
Nikiema, B. C. W.-Y., Ito, R., Guizani, M., and Funamizu, N. (2017). Estimation of water flux and solute movement during the concentration process of hydrolysed urine by forward osmosis. J. Water Environ. Technol. 15 (5), 163–173. doi:10.2965/jwet.16-074
Onabanjo, T., Kolios, A. J., Patchigolla, K., Wagland, S. T., Fidalgo, B., Jurado, N., et al. (2016). An experimental investigation of the combustion performance of human faeces. Fuel 184, 780–791. doi:10.1016/j.fuel.2016.07.077
Ouadi, M., Bashir, M. A., Speranza, L. G., Jahangiri, H., and Hornung, A. (2019). Food and market waste–a pathway to sustainable fuels and waste valorization. Energy fuels. 33 (10), 9843–9850. doi:10.1021/acs.energyfuels.9b01650
Patel, A., Mungray, A. A., and Mungray, A. K. (2020). Technologies for the recovery of nutrients, water and energy from human urine: a review. Chemosphere 259, 127372. doi:10.1016/j.chemosphere.2020.127372
Pype, M. L., Lawrence, M. G., Keller, J., and Gernjak, W. (2016). Reverse osmosis integrity monitoring in water reuse: the challenge to verify virus removal – a review. Water Res. 98, 384–395. doi:10.1016/J.WATRES.2016.04.040
Randall, D. G., and Naidoo, V. (2018). Urine: the liquid gold of wastewater. J. Environ. Chem. Eng. 6 (2), 2627–2635. doi:10.1016/j.jece.2018.04.012
Ray, H., Perreault, F., and Boyer, T. H. (2020). Ammonia recovery from hydrolyzed human urine by forward osmosis with acidified draw solution. Environ. Sci. Technol. 54 (18), 11556–11565. doi:10.1021/acs.est.0c02751
Ray, H., Perreault, F., and Boyer, T. H. (2019). Urea recovery from fresh human urine by forward osmosis and membrane distillation (FO–MD). Environ. Sci. Water Res. Technol. 5 (11), 1993–2003. doi:10.1039/C9EW00720B
Rose, C., Parker, A., Jefferson, B., and Cartmell, E. (2015). The characterization of feces and urine: a review of the literature to inform advanced treatment technology. Crit. Rev. Environ. Sci. Technol. 45 (17), 1827–1879. doi:10.1080/10643389.2014.1000761
Siddiqui, F. A., She, Q., Fane, A. G., and Field, R. W. (2018). Exploring the differences between forward osmosis and reverse osmosis fouling. J. Memb. Sci. 565, 241–253. doi:10.1016/j.memsci.2018.08.034
Skorina, T., and Allanore, A. (2015). Aqueous alteration of potassium-bearing aluminosilicate minerals: from mechanism to processing. Green Chem. 17 (4), 2123–2136. doi:10.1039/C4GC02084G
United Nations (2020). SDG 6 overview. Available at: https://sdgs.un.org/goals/goal6 (Accessed November 10, 2021).
United Nations (2017). World population prospects: the 2017 revision, key findings and advance tables. New York: United Nations.
Valladares Linares, R., Yangali-Quintanilla, V., Li, Z., and Amy, G. (2011). Rejection of micropollutants by clean and fouled forward osmosis membrane. Water Res. 45 (20), 6737–6744. doi:10.1016/j.watres.2011.10.037
Vital, B., Bartacek, J., Ortega-Bravo, J. C., and Jeison, D. (2018). Treatment of acid mine drainage by forward osmosis: heavy metal rejection and reverse flux of draw solution constituents. Chem. Eng. J. 332, 85–91. doi:10.1016/j.cej.2017.09.034
Volpin, F., Chekli, L., Phuntsho, S., Cho, J., Ghaffour, N., Vrouwenvelder, J. S., et al. (2018). Simultaneous phosphorous and nitrogen recovery from source-separated urine: a novel application for fertiliser drawn forward osmosis. Chemosphere 203, 482–489. doi:10.1016/j.chemosphere.2018.03.193
Volpin, F., Chekli, L., Phuntsho, S., Ghaffour, N., Vrouwenvelder, J. S., Shon, H. K., et al. (2019a). Optimisation of a forward osmosis and membrane distillation hybrid system for the treatment of source-separated urine. Sep. Purif. Technol. 212, 368–375. doi:10.1016/j.seppur.2018.11.003
Volpin, F., Heo, H., Hasan Johir, M. A., Cho, J., Phuntsho, S., Shon, H. K., et al. (2019b). Techno-economic feasibility of recovering phosphorus, nitrogen and water from dilute human urine via forward osmosis. Water Res. 150, 47–55. doi:10.1016/j.watres.2018.11.056
von Münch, E., and Winker, M. (2011). Technology review of urine diversion components. Editor E. von Münch (Eschborn, Germany: Postfach: Deutsche Gesellschaft für Internationale Zusammenarbeit (GIZ) GmbH). Available at: http://www.susana.org/_resources/documents/default/2-875-giz2011-en-technology-review-urine-diversion.pdf (Accessed June 15, 2022).
Wang, S.-Y., Chen, Y.-F., Zhou, H., Xu, X.-H., and Cheng, L.-H. (2020). Calcium carbonate scaling in forward osmosis for textile reverse osmosis concentrate treatment. J. Water Process Eng. 35, 101181. doi:10.1016/j.jwpe.2020.101181
Xie, M., Shon, H. K., Gray, S. R., and Elimelech, M. (2016). Membrane-based processes for wastewater nutrient recovery: technology, challenges, and future direction. Water Res. 89, 210–221. doi:10.1016/j.watres.2015.11.045
Xue, W., Tobino, T., Nakajima, F., and Yamamoto, K. (2015). Seawater-driven forward osmosis for enriching nitrogen and phosphorous in treated municipal wastewater: effect of membrane properties and feed solution chemistry. Water Res. 69, 120–130. doi:10.1016/j.watres.2014.11.007
Zhang, J., She, Q., Chang, V. W. C., Tang, C. Y., and Webster, R. D. (2014). Mining nutrients (N, K, P) from urban source-separated urine by forward osmosis dewatering. Environ. Sci. Technol. 48 (6), 3386–3394. doi:10.1021/es405266d
Keywords: fouling, membrane, non-sewered sanitation, rejections, resource recovery, water recovery
Citation: Pocock J, Muzhingi A, Mercer E, Velkushnova K, Septien S and Buckley CA (2022) Water and nutrient recovery from stored urine by forward osmosis with an ammonium bicarbonate draw solution. Front. Environ. Sci. 10:937456. doi: 10.3389/fenvs.2022.937456
Received: 06 May 2022; Accepted: 11 July 2022;
Published: 04 August 2022.
Edited by:
Kangning Xu, Beijing Forestry University, ChinaReviewed by:
Prithvi Simha, Swedish University of Agricultural Sciences, SwedenPeizhe Sun, Tianjin University, China
Copyright © 2022 Pocock, Muzhingi, Mercer, Velkushnova, Septien and Buckley. This is an open-access article distributed under the terms of the Creative Commons Attribution License (CC BY). The use, distribution or reproduction in other forums is permitted, provided the original author(s) and the copyright owner(s) are credited and that the original publication in this journal is cited, in accordance with accepted academic practice. No use, distribution or reproduction is permitted which does not comply with these terms.
*Correspondence: S. Septien, c2VwdGllbnNAdWt6bi5hYy56YQ==
†Present address K. Velkushnova, IHE-DELFT Institute for Water Education, Delft, Netherlands