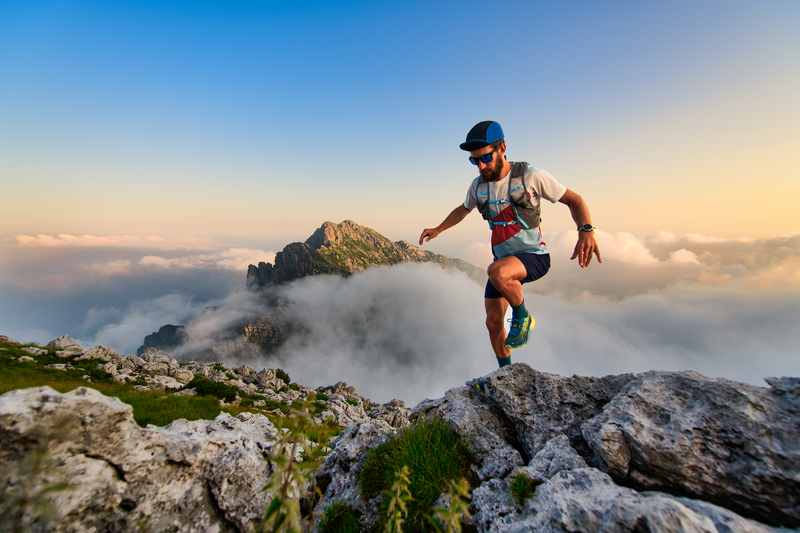
95% of researchers rate our articles as excellent or good
Learn more about the work of our research integrity team to safeguard the quality of each article we publish.
Find out more
ORIGINAL RESEARCH article
Front. Environ. Sci. , 05 September 2022
Sec. Biogeochemical Dynamics
Volume 10 - 2022 | https://doi.org/10.3389/fenvs.2022.930052
This article is part of the Research Topic The Cold Regions in Transition: Impacts on Soil and Groundwater Biogeochemistry View all 8 articles
The ongoing climate warming is likely to increase the frequency of freeze-thaw cycles (FTCs) in cold-temperate peatland regions. Despite the importance of soil hydro-physical properties in water and carbon cycling in peatlands, the impacts of FTCs on peat properties as well as carbon sequestration and release remain poorly understood. In this study, we collected undisturbed topsoil samples from two drained lowland fen peatlands to investigate the impact of FTCs on hydro-physical properties as well as dissolved organic carbon (DOC) fluxes from peat. The soil samples were subject to five freeze-thaw treatments, including a zero, one, three, five, ten cycles (FTC0, FTC1, FTC3, FTC5, and FTC10, respectively). Each FTC was composed of 24 h of freezing (−5°C) and 24 h of thawing (5°C) and the soil moisture content during the freeze-thaw experiment was adjusted to field capacity. The results showed that the FTCs substantially altered the saturated hydraulic conductivity (Ks) of peat. For peat samples with low initial Ks values (e.g., < 0.2 × 10−5 m s−1), Ks increased after FTCs. In contrast, the Ks of peat decreased after freeze-thaw, if the initial Ks was comparably high (e.g., > 0.8 × 10−5 m s−1). Overall, the average Ks values of peatlands decreased after FTCs. The reduction in Ks values can be explained by the changes in macroporosity. The DOC experiment results revealed that the FTCs could increase DOC concentrations in leachate, but the DOC fluxes decreased mainly because of a reduction in water flow rate as well as Ks. In conclusion, soil hydraulic properties of peat (e.g., Ks) are affected by freezing and thawing. The dynamics of soil hydraulic properties need to be explicitly addressed in the quantification and modelling of the water flux and DOC release from peatlands.
Peatlands cover approximately 3% of the terrestrial surface of the Earth (Bragazza et al., 2013) but store approximately 21% of the global soil carbon pool (Leifeld and Menichetti, 2018). Nowadays, around 10–15% of the world’s peatlands are artificially drained mainly for agriculture (Kreyling et al., 2021). Peatland drainage lowers the water table depth, leading to land subsidence (Hooijer et al., 2012; Liu et al., 2020), water storage loss (Liu et al., 2022), greenhouse gases emissions (e.g., carbon dioxide, CO2; Leifeld and Menichetti, 2018), and dissolved organic carbon (DOC) release to groundwater and streams in peatland-dominated catchments (Evans, 2015). Carbon loss from peatlands could accelerate global warming, which in turn impacts carbon storage in peatlands (Hanson et al., 2020).
The ongoing winter climate warming is expected to increase the frequency of freeze-thaw cycles (FTCs) in cold-temperate regions (Kreyling et al., 2008), which may substantially alter the biogeochemical processes (e.g., carbon and nitrogen turnover; Azizi-Rad et al., 2022; Byun et al., 2021; Henry, 2007; Matzner and Borken, 2008). A substantial amount of greenhouse gases (e.g., carbon dioxide, nitrous oxide) are emitted from thawing soils during and/or following winters (Dörsch et al., 2004; Matzner and Borken, 2008). The changes in soil structure, microbial biomass, and root turnover during FTCs are reported to be the main reasons for the increased carbon and nitrogen concentrations (Tierney et al., 2001; Matzner and Borken, 2008).
Dissolved organic carbon is one of the major carbon loss pathways in peat soils and also leads to water quality problems in downstream surface and groundwater (Evans et al., 2016; Xu et al., 2020). Increasing DOC concentrations in streams have been recorded across Europe and North America (Monteith et al., 2007). A high DOC concentration can, likewise, cause color, odor, and taste problems in drinking water (Ritson et al., 2017). There have been several studies that have addressed the impact of FTCs on DOC concentrations from peatland, however, the results are divergent. It has been observed that the FTCs may increase water-extracted DOC concentrations of permafrost fen peat (Wang et al., 2014) and pore water DOC concentrations of natural wetlands soils (Yu et al., 2011). However, some other studies observed that the FTCs have a limited role in DOC concentrations of temperate bogs and permafrost bogs (Heinz and Zak, 2018; Pokrovsky et al., 2018; Payandi-Rolland et al., 2021). It should be noted that the DOC flux to streams is controlled by DOC pore water concentrations (Freeman et al., 2001) and the hydrological processes and DOC transport mechanisms (Evans et al., 2006; Holden et al., 2012; Olefeldt and Roulet, 2014). However, the latter conditions as well as DOC fluxes under FTCs have not been addressed in previous studies.
Soil hydraulic properties (e.g., saturated hydraulic conductivity, Ks) and the soil pore structure (e.g., macropore) play critical roles in water flow and contaminants export (Baird, 1997; Holden, 2009; Hribljan et al., 2014). It has been reported that the hydraulic properties and the soil structure could be substantially altered by FTCs (Starkloff et al., 2017; Ding et al., 2019; Liu et al., 2021). For mineral soils, it has been reported that the FTCs can cause the fragmentation of soil coarse particles and aggregation of soil fine particles (Zhang et al., 2016), thereby changing the soil structure. However, the impact of FTCs on soil structure is found to be quite divergent, depending on the soil texture (Zhao et al., 2021). From computed tomography scanning and image analysis, Starkloff et al. (2017) observed that the macroporosity in silty clay loam and loamy sand decreased after FTCs. However, some other studies found that the macroporosity in clay and black soils increased significantly after several FTCs (Fan et al., 2021; Liu et al., 2021). Due to the changes in soil structure, the soil water permeability also changes after several FTCs. It has been reported that the saturated hydraulic conductivity (Ks) of compacted clay increased after several FTCs (Othman and Benson, 1993). However, Starkloff et al. (2017) found that the consecutive FTCs can reduce the water permeability of loose soils. Compared with mineral soils, peat soils have some unique properties, such as high organic matter content (as high as 99 wt%, percent by weight; Liu and Lennartz, 2019) and low bulk density (as low as 0.01 g cm−3; Boelter, 1968). The Ks values of pristine peat range from 10−2 to 10−5 m s−1 (Letts et al., 2000; Rezanezhad et al., 2016). After peatland drainage, Ks values generally decrease ranging from 10−4 to 10−8 m s−1 (Wang M. et al., 2021). Although previous studies have proved that the Ks of peat plays an important role in DOC export from peatland (Hribljan et al., 2014; Strackloff et al., 2017), we know very little about the dynamics of Ks and DOC fluxes under winter warming conditions with frequent FTCs. In this study, we hypothesize that FTCs may change the hydro-physical properties (e.g., macroporosity, Ks) of peat, which possibly influences the DOC fluxes from peat. The main research objectives were to investigate: 1) the impact of FTCs on Ks and macroporosity of peat, and 2) the impact of FTCs on DOC concentrations and fluxes from peat.
The study sites are located in the state of Mecklenburg-Pomerania, northeastern Germany. The climate of Mecklenburg-Pomerania is characterized by an average annual precipitation of 620 mm and the average air temperature in winters (reference period 1882–2010) generally fluctuates between –5°C and 5°C (data is from Germany’s national meteorological service; Supplementary Figure S1). In Mecklenburg-Pomerania, peatlands cover an area of 2,800 km2 and approximately 98% of peatlands are groundwater fed and thus are called fens (Zeitz et al., 2011). Over the last century, more than 95% of peatlands in Mecklenburg-Pomerania have been drained for agriculture (Tanneberger et al., 2021). In this study, two drained fen peatlands (Site 1, 54°00'22.6" N, 12°06'56.3" E; Site 2, 53°59'57" N, 12°13'56" E) were selected as they represent the majority of the peatlands in north-eastern Germany (Liu et al., 2016). The topsoils (0–20 cm) in the two drained peatlands were highly degraded due to the organic matter mineralization caused by land drainage. The organic matter contents of the top soils are 30% and 80% for sites 1 and 2, respectively (Liu et al., 2016; Wang M. et al., 2021), indicating that the soils at site 1 are more decomposed and degraded (Liu and Lennartz, 2019). The parent plant materials that form peat soils are Carex peat with some Phragmites (Liu et al., 2016). For both sites, the water table depth is approximately 0.8–1.2 m below the ground surface in summers and 0.2–0.6 m below the ground surface during winters.
Forty-five undisturbed peat core samples (7.2 cm in diameter and 6.1 cm in length) were collected randomly over an area of 8 m × 8 m at each study site in November 2020. All undisturbed peat samples were taken by cutting soil with steel cylinders and a sharp knife (Wang et al., 2020). After soil sampling, all peat cores were refrigerated and transported to the laboratory at Rostock University, Germany, for freeze-thaw experiments.
Before starting the freeze-thaw experiments, all the peat cores were saturated slowly from the bottom using tap water with an electrical conductivity (EC) of approximately 700 μS cm−1 corresponded to that of the groundwater at the field sites. After saturation, the Ks was determined for all peat cores by a constant-head permeameter (Liu et al., 2016). Thereafter, the samples were classified into five groups (each group, n = 9) with a comparable mean value of Ks. Among the five groups, one group was set as control group (without freeze-thaw treatment; FTC0) and the other four groups, the peat cores experienced 1, 3, 5, and 10 FTCs (FTC1, FTC3, FTC5, FTC10, respectively). The freezing and thawing of the samples were conducted using a freezer with temperature range of –10–20°C and each FTC was composed of freezing at −5°C for 24 h and thawing at 5°C for 24 h. The soil moisture content of peat samples was adjusted to the field capacity by draining the samples at –60 cm H2O pressure head before the start of the FTC experiment. For the control group, the moisture content of the samples was also adjusted to the field capacity after initial Ks measurement. After experimental treatments (freeze-thaw treatment and control treatment), all the peat cores were re-saturated from the bottom and the Ks was measured. Thereafter, the peat cores were drained at –60 cm H2O pressure head again and after the final round oven-dried at 105°C for 24 h to determine the bulk density using dry mass. The soil organic matter content of peat samples was analyzed by using the loss on ignition method [burning samples at a temperature of 550°C for 4 h; ISO (2,247)6–3:2005]. In this study, we define the macropore as pores with an equivalent cylindrical diameter greater than 30 μm (Cameron and Buchan, 2016), which can be estimated as the difference between the total porosity and the volumetric water content at –60 cm H2O pressure head (assuming the soil liquid contact angle of 52° for peat; Gharedaghloo and Price, 2019; Wang M. et al., 2021). The gravimetric soil water content and bulk density were determined to estimate the total porosity and the volumetric water content at –60 cm H2O pressure head.
In order to determine the impact of FTCs on DOC flux, additional eight peat core samples were collected from each field site. The samples were separated into two groups (control group without freeze-thaw treatment and freeze-thaw group) with a comparable mean value and range of Ks. For the freeze-thaw group, the samples were saturated with artificial water (NaCl solution with EC of 700 μS cm−1, corresponded to that of the groundwater at the field sites) and then they were flushed with rainwater alike (NaCl solution, EC = 50 μS cm−1) at a constant water hydraulic head of 1.5 cm (Supplementary Figure S2). After 1-h of flushing, the peat samples were drained at –60 cm H2O pressure head and subjected to one FTC. Thereafter, the soil samples were re-saturated and re-flushed following the same procedure. The effluent water samples were collected after the peat samples experienced a cumulative of 0, 1, 3, 5, and 10 FTCs. The volume of the effluent was also recorded during the flushing experiment. For the control group, the peat samples were saturated, flushed, and drained 5 times on the same dates as treatment group. The samples of the control group were kept in a refrigerator at 5°C between leaching experiments and each sample of the freeze-thaw group was covered by a plastic sheet (Profissimo, dm-drogerie markt, Karlsruhe, Germany; not directly contact with the peat soils) during freezing-thawing treatment to prevent water loss by evaporation. The effluent water samples were collected and filtered through a pre-washed 0.45 µm mixed cellulose ester (MCE) syringe filter to determine the DOC concentrations using DIMATOC® 2000 (Dimatec Analysentechnik GmbH, Essen, Germany). The DOC flux (
Where
The Ks values followed a lognormal distribution; therefore, the data were transformed to common logarithms (log10) before a further statistical analysis. A t-test was used to investigate the differences in bulk density, macroporosity, and log10Ks between the two study sites. A paired t-test was performed on each group to evaluate the mean difference of log10Ks before and after FTC treatment. A one-way analysis of variance (ANOVA) was carried out to analyze whether the log10Ks differed significantly among the groups after freeze-thaw treatments. The Shapiro-Wilk tests indicate that the changes in flow rate, DOC concentrations as well as DOC fluxes were normally distributed. Therefore, a t-test was used to investigate whether the changes in DOC concentrations and fluxes as well as flow rates differed significantly between the freeze-thaw group and control group after each freeze-thaw treatment. A paired t-test was applied to the freeze-thaw group to evaluate the mean difference of DOC concentrations before and after FTCs. All the statistical analyses were conducted using R (R Core Team, 2020).
The organic matter contents of peat samples from the study sites 1 and 2 were 35.7 ± 0.3 wt% and 76.5 ± 0.4 wt% (by weight; mean ± 1 standard error), respectively (Supplementary Table S1). The average soil bulk density value was 0.48 g cm−3 for site 1, which was higher than the value of site 2 (0.41 g cm−3; p < 0.001). The average macroporosity differed likewise between sites (site 1 = 0.12 ± 0.01 cm3 cm−3, site 2 = 0.10 ± 0.01 cm3 cm−3; mean ± 1 standard error). The average field capacity at sites 1 and 2 were 0.61 ± 0.02 and 0.67 ± 0.01 cm3 cm−3, respectively (mean ± standard error). The Ks of fen peat at site 1 ranged from 1.9 × 10−6 m s−1 to 1.0 × 10−4 m s−1, while the according values of site 2 varied from 3.0 × 10−7 m s−1 to 4.5 × 10−4 m s−1. For Ks, the coefficient of variation (CV) was 99% for site 1 and 138% for site 2. There was no significant difference in log10Ks between the two study sites (p = 0.082). A strong positive linear relationship was found between log10Ks and macroporosity (Supplementary Figure S3; R2 = 0.68; p < 0.001).
The paired t-test suggested that there was no significant difference between the average log10Ks before and after zero FTC (FTC0) treatment in the control group (without freeze-thaw treatment; p = 0.92; Supplementary Tables S2, S3). Figure 1 shows that the log10Ks values before and after zero freeze-thaw treatment are distributed along the 1:1 ratio line. For the control groups, the differences between the first and second measurements of Ks was generally within 10% of its initial value. The differences of Ks values in the two measurements possibly resulted from measurement errors or occurrence of gas bubbles during the saturation or re-saturation procedure.
FIGURE 1. The saturated hydraulic conductivity (Ks, m s−1) of peat in the control group before (log10Ks, before) and after (log10Ks, after) zero freeze-thaw treatment (FTC0; without FTCs).
For the other four groups (FTC1, FTC3, FTC5, and FTC10), the average log10Ks values generally decreased after temperature treatment (Table 1). The results from the paired t-test suggested that significant differences in log10Ks before (without freeze-thaw treatment) and after FTCs were observed after five cycles for site 1 samples (p = 0.028) and after three cycles for site 2 samples (p = 0.033; Supplementary Tables S2, S3). The results from the one-way ANOVA suggested that there was no significant difference in Ks between the four groups and after FTCs within one site (p > 0.05). For site 1, the geometric mean value of Ks decreased from 2.0 × 10−5 m s−1 (log10Ks,—4.7) to 1.1 × 10−5 m s−1 (log10Ks,—4.9) after five FTCs. For site 2, the geometric mean value of Ks decreased by 25% from 3.6 × 10−6 m s−1 to 2.7 × 10−6 m s−1 (log10Ks from—5.45 to—5.57) after three FTCs (Table 1).
TABLE 1. Mean (standard error) of saturated hydraulic conductivity, log10 (Ks, m s−1) before and after different freeze-thaw cycles (FTC1, FTC3, FTC5, and FTC 10).
For individual peat samples, the FTCs substantially altered the Ks values. After FTCs, the Ks value was generally 30%–270% of its initial value. The changes in Ks differed between the two study sites but highly relied on the initial Ks of peat before FTCs (Figure 2). A strong negative correlation was observed between the differences in log10Ks before and after FTCs and the initial log10Ks values before FTCs (R2 > 0.6; Figure 2). For peat soils with low Ks, the Ks increased after FTC. In contrast, the Ks of peat decreased after FTC, if the initial Ks was high. It seems that the threshold value, above and below this value soil Ks responded differently with FTCs, differed between the two study sites (0.8 × 10−5 m s−1 for site 1 and 0.2 × 10−5 m s−1 for site 2) and the threshold values are much smaller than the average Ks values of the peatlands.
FIGURE 2. Scatter plot of the differences between log10Ks before and after different FTCs against log10Ks of peat before FTCs.
There was no significant difference in average flow rate between the freeze-thaw group and the control group at both sites. For the control groups, the flow rates generally changed slightly over the entire experiment (Figure 3). In contrast, the changes of the flow rates in freeze-thaw group were much greater than in the control group. The average flow rates of the freeze-thaw group at sites 1 and 2 decreased by approximately 40% (0.08–0.14 L h−1; Figure 3) until three to five FTCs and then increased slightly after ten FTCs.
FIGURE 3. Changes in flow rates, DOC concentrations, and DOC fluxes as observed from samples of the freeze-thaw group and control group for site 1 (left) and site 2 (right) in comparison to the initial values before FTCs. The bars denote the standard error within one group. The asterisk represents a significant difference at p < 0.05.
Before any FTCs, the effluent DOC concentrations of the samples at site 1 varied from 19.9 to 49.3 mg l−1, which were lower than those values from the samples at site 2 (69.4–128.7 mg l−1). No significant difference was observed in DOC concentrations between the freeze-thaw group and control group at both sites. For the control groups, the DOC concentrations were kept almost constant in the beginning and then decreased by approximately 50% (14.4 ± 6.5 mg L−1 for site 1 and 60.5 ± 6.6 mg L−1 for site 2) at a later stage of the experiment (Figure 3). In contrast, for the freeze-thaw group at site 1, the average effluent DOC concentration increased by 23% (7.8 ± 3.6 mg L−1) after one FTC and then decreased and increased again thereafter. At site 2, the average effluent DOC concentrations increased by 37% (35.4 ± 12.6 mg L−1) after one FTC and then decreased with further increase in FTCs (Figure 3). At both sites, the highest average DOC concentration was observed after one FTC. After ten FTCs, the changes in DOC concentrations were significantly greater for control group than the freeze-thaw group for site 2 (Figure 3).
The average DOC fluxes (standard error) at sites 1 and 2 were 0.27 ± 0.03 and 0.31 ± 0.07 mg cm−2 h−1, respectively. For the control group, the average DOC flux varied slightly at the beginning and then decreased by approximately 40% at a later stage of the experiment (Figure 3). In contrast, the DOC fluxes of the freeze-thaw groups decreased by approximately 50% (0.12 ± 0.02 mg cm−2 h−1 for site 1 and 0.18 ± 0.08 mg cm−2 h−1 for site 2) until three to five FTCs, and then increased after ten FTCs. After ten FTCs, the changes in DOC fluxes are comparable between the freeze-thaw group and control group (Figure 3).
Peat soils are highly heterogeneous with complex dual-porosity media (Rezanezhad et al., 2016). The Ks values generally differ by two to three orders of magnitude for one peat horizon (Beckwith et al., 2003; Cunliffe et al., 2013; Liu et al., 2016). The Ks values of fen peat of the two study sites varies from 10−7 to 10−4 m s−1, which is within the range reported for degraded fens (10–8 to 10–4 m s−1; Kechavarzi et al., 2010; Schwärzel et al., 2006; Wang M. et al., 2021). The CV values for Ks at site 1 and site 2 (99% and 138%, respectively) are higher than the value reported for a fen peat in the United Kingdom (98%; Baird, 1997) but within the range of 70%–150% reported by Wang M. et al. (2021). In our experiments, the CV for Ks decreased after a few successive FTCs (Supplementary Table S4) for both sites with approximately 40% decrease after five FTCs. This finding indicates that the spatial heterogeneity of peat may be reduced under winter warming conditions with frequent FTCs.
The Ks of peat is mainly influenced by the peat-forming plant residues (Sphagnum or Carex; Päivänen, 1973), peat decomposition and degradation stages (Letts et al., 2000; Rezanezhad et al., 2016; Liu and Lennartz, 2019; Morris et al., 2019), microhabitat (Morris et al., 2015), and soil pore water chemistry (Kettridge and Binley, 2010; McCarter et al., 2019, 2020; Sirianni and Comas, 2020). Branham and Strack (2014) observed that the Ks of Sphagnum peat (pristine peat) was strongly positively correlated with the macropore occurrence (pore diameter of 2000 μm; Spearman correlation > 0.8). It should be noted that the definition of macropore differs in previous studies from minimum pore diameter of 30 μm–3,000 μm (Beven and Germann, 1982; Holden et al., 2001; Weber et al., 2017; Wallor et al., 2018). The peat samples used in this study are highly degraded fen peat, for which the macroporosity with a pore diameter of > 300 μm is quite small (<1 vol%) and difficult to quantify (Liu et al., 2020). Therefore, we here defined the macroporosity as pores having a diameter of greater than 30 μm. The relation between macroporosity and log10Ks is supported by a recent study from Wang M. et al. (2021), who observed a moderate correlation between macroporosity and log10Ks for various kinds of peat types (Pearson’s correlation coefficient > 0.55). We here found that the function between macroporosity and log10Ks is not affected by the FTCs (Figure 4), indicating that the macroporosity is the main factor controlling the Ks of peat at a centimeter scale.
FIGURE 4. The relationship between macroporosity and saturated hydraulic conductivity (log10Ks) of peat before (orange circles) and after (blue circles) freeze-thaw cycles (FTCs).
Our results showed that the FTCs did not change the average macroporosity of peat soils (site 1 = 0.12 ± 0.01 cm3 cm−3, site 2 = 0.10 ± 0.01 cm3 cm−3; mean ± 1 standard error). But the FTCs alter the individual macroporosity of peat soils where the changes ranged from –5 vol% to 4 vol%, depending on the initial macroporosity. A moderate negative relationship was found between the initial macroporosity and the changes in macroporosity after FTCs (Pearson’s correlation coefficient of –0.58, p < 0.01). For peat soils, to our knowledge, no information on the temperature-affected macroporosity dynamic is available. For mineral soils, the changes in soil structure depend on the texture and it has been reported that the macroporosity (> 140 µm pore diameter) of looser sand decreased up to 2 vol% after six FTCs (Starkloff et al., 2017). Leuther and Schlüter (2021) observed that the macroporosity (> 48 µm pore diameter) of repacked loose silt clay decreased by 8 vol% after 10 FTCs. For clay soils opposite results were obtained and the macroporosity increased by 30%–60% upon freezing and thawing (Fan et al., 2021).
A moderate positive linear relation was found between the differences of log10Ks and the differences of macroporosity for before and after FTCs (R2 = 0.41, p < 0.001; Figure 5). This finding suggests that for peat soils with low Ks values, the macroporosity generally increases after FTCs, thereby increasing the hydraulic conductivity. In contrast, the FTCs decrease the macroporosity and thereby the Ks, if the hydraulic conductivity of peat is comparably high. A similar relationship has been observed for mineral soils. For sand, the Ks values decreased from 2.0 × 10−5 to 6.0 × 10−6 m s−1 after six FTCs (Wang Y. B. et al., 2021). For mineral soils with low initial Ks values (e.g., saline–alkali soils or black soils; 10−7 m s−1), the FTCs increase the macroporosity and the Ks increased by 2–20 times after a few FTCs (Ma et al., 2019; Xu et al., 2021). In our study, the soil samples were drained at—60 cm H2O pressure head before FTCs. Thus, the macropores (pore diameter > 30 μm) are dewatered and the micropores (pore diameter < 30 μm) were water saturated. We assume that ice lenses form in the microporous domain during the freezing period causing cracks, which increase the Ks of soils with low initial Ks values upon thawing (Othman and Benson, 1993). However, for soils with high initial Ks values (high macroporosity), the ice lenses formation may cause a breakdown of large-size aggregates and block the original macropores (Hayashi, 2013; Starkloff et al., 2017), thereby reducing the soil permeability. Considering all the peat samples, the average Ks values of peat decreased as the average Ks values are much higher than the threshold values (0.8 × 10−5 m s−1 for site 1 and 0.2 × 10−5 m s−1 for site 2). It has been reported that the response of hydraulic conductivity to freeze-thaw cycles is related to the initial soil moisture content (Roy et al., 2021). The soil organic matter (76.5 ± 0.4%) and the soil moisture content (0.67 ± 0.01 cm3 cm−3) before freezing of site 2 samples were significantly higher than for site 1 samples (p < 0.01), which may be the reasons for the different threshold values.
FIGURE 5. The differences between saturated hydraulic conductivity of peat (log10Ks) before and after different freeze-thaw cycles (FTC1, FTC3, FTC5, FTC10) against the difference between macroporosity before and after different FTCs.
The flow rate depends on the Ks of the soil core when the hydraulic gradient is constant (Hillel, 1998). The Ks values of the samples from the freeze-thaw group for DOC experiment were generally greater than 0.48 × 10−5 m s−1. The temperature treatment decreased the Ks values consistently and thereby lowered the flow rates. Only one soil core from site 2 had a low initial Ks value (0.15 × 10−5 m s−1) and the flow rate increased slightly after FTCs. However, in general, the average Ks values and flow rates decreased after FTCs.
The effluent DOC concentrations increased after one FTC consistently for both sites, showing the possible effect of the FTCs on DOC concentration in peat. This finding can be supported by previous studies that FTCs may increase the DOC pore water concentrations due to the physical breakdown of macroaggregates, death of microbial cells, and root turnover during the freezing period (Matzner and Borken, 2008; Yu et al., 2011; Wang et al., 2014). However, some other studies reported that the FTCs have little impact on DOC concentrations of permafrost peat soils (e.g., Payandi-Rolland et al., 2021). One possible reason is that in the permafrost studied by Payandi-Rolland et al. (2021), the microbial communities are resistant to FTCs. Previous studies reported that the microbes in a variety of soil types are highly susceptible to the first freeze-thaw cycle, and then less affected by subsequent freeze-thaw cycles (Koponen et al., 2006; Song et al., 2017; Patel et al., 2021; Rooney et al., 2022). The different climate regions and peatland types (temperate fen grasslands vs. permafrost bogs) may be responsible for the different changes in DOC concentrations after FTCs.
The highest effluent DOC concentrations were observed after one FTC and the impact of freezing and thawing on DOC concentrations varies with the number of FTCs. Comparing with the initial DOC concentrations, an increase of DOC concentrations was observed in the first FTC and there was no change in DOC concentrations after three FTCs. For soils that experienced five or ten FTCs, the DOC concentrations decreased but the reduction in DOC concentrations was lower than the control group. This finding is consistent with the studies from Yu et al. (2011) and Yang et al. (2022), in which an increase of DOC concentration generally occurred in the first two FTCs. Similarly, Wang et al. (2014) reported that the FTCs significantly increased the water-extracted organic carbon concentration of peat by 22%–36% and the highest values occur after five FTCs.
The DOC flux calculations explicitly account for water flow. The leached water volume differed after FTCs because the hydraulic conductivity changed, while the hydraulic head was kept constant. In contrast to the control group, where the DOC fluxes changed only slightly, the DOC fluxes of the freeze-thaw group decreased significantly within three FTCs mainly due to the reduction of flow rate (Figure 3). Thus, the DOC fluxes decreased though DOC concentrations may increase after FTCs. It should be noted that the FTCs may also change the soil carbon to nitrogen ratio as well as DOC sorption processes (Yu et al., 2010; Song et al., 2017), which may further impact the DOC fluxes from soils. The mineral particles in peat (e.g., clay) may also affect the soil response to FTCs, which needs to be further investigated. The physical disruption of macro-aggregates during FTCs might expose previously inaccessible substrate and induce a faster carbon and nitrogen mineralization rate (Matzner and Borken, 2008; King et al., 2021), the importance of soil pore structure on water and carbon fluxes has rarely been addressed. The findings of this study indicate that the soil hydraulic properties (e.g., Ks) control the flushing and retention of pore water (Hribljan et al., 2014) and thus play a vital role in compound fluxes (e.g., DOC). In view of the entire experiment, we can conclude that although winter warming in high latitude regions could increase DOC pore water concentrations in lowland drained fen peat, the DOC fluxes may decrease after three FTCs because of the changes in soil hydraulic properties (Figure 3). It should be noted that the impact of FTCs on hydro-physical properties depends on the freezing and thawing temperatures, soil water content, and the duration of freezing and thawing (Henry, 2007; Wei et al., 2018; Feng et al., 2020; Azizi-Rad et al., 2022). Under global warming conditions, the amplitude of FTCs may shift, and the frequency of freeze-thaw will probably increase in cold-temperate regions. It would, therefore, be helpful to investigate various freeze-thaw conditions in a systematic manner in future studies.
Ongoing global warming in cold-temperate regions will increase the frequency of freeze-thawing processes, which may alter the water and carbon cycles in boreal and temperate peatlands. Until now, the dynamics of peat properties under global warming conditions have not been sufficiently investigated and considered in hydrological models, which may result in an over- or underestimation of water and matter fluxes from peatlands to adjacent water bodies. This study demonstrated that FTCs could alter the macroporosity, hydraulic conductivity, and DOC concentrations of peat. The average Ks values as well as the flow rates decreased after FTCs, which may decrease the DOC fluxes and, thus, the export of DOC from peatlands, although the DOC concentrations increase after FTCs. To our knowledge, this is the first study quantifying the dynamics of hydraulic properties of drained peat soils and their linkage to DOC fluxes under freeze-thaw conditions. The results reveal that the dynamics of soil physical properties might be as important as the pore water concentrations when evaluating the export of compounds from peat in winter conditions. At landscape scale, the results imply that the hydrological processes (e.g., infiltration, runoff) may change under freeze-thaw conditions due to the dynamics of Ks. We argue that the dynamics of peat hydraulic properties should be considered in modelling the water flow and contaminant transport from peatlands. We also recommend investigating severity, duration and frequency of FTCs in more detail in future studies to fully understand the consequences of intensified temperature amplitudes for high latitude peatlands.
The original contributions presented in the study are included in the article/Supplementary Materials, further inquiries can be directed to the corresponding author.
HL: Conceptualization, Investigation, Methodology, Writing—original draft, Writing—review and editing. FR: Methodology, Writing—review and editing. DZ: Methodology, Writing—review and editing. XL: Investigation, Writing—review and editing. BL: Conceptualization, Writing—review and editing.
The authors are grateful for the support by the Research Training Group Baltic TRANSCOAST program, funded by the DFG (Deutsche Forschungsgemeinschaft) under grant number DFG-GRK 2000/2.
We thank Stefan Köhler (University of Rostock, Landscape Ecology) for the DOC measurements.
The authors declare that the research was conducted in the absence of any commercial or financial relationships that could be construed as a potential conflict of interest.
All claims expressed in this article are solely those of the authors and do not necessarily represent those of their affiliated organizations, or those of the publisher, the editors and the reviewers. Any product that may be evaluated in this article, or claim that may be made by its manufacturer, is not guaranteed or endorsed by the publisher.
The Supplementary Material for this article can be found online at: https://www.frontiersin.org/articles/10.3389/fenvs.2022.930052/full#supplementary-material
Azizi-Rad, M., Guggenberger, G., Ma, Y., and Sierra, C. A. (2022). Sensitivity of soil respiration rate with respect to temperature, moisture and oxygen under freezing and thawing. Soil Biol. Biochem. 165, 108488. doi:10.1016/j.soilbio.2021.108488
Baird, A. J. (1997). Field estimation of macropore functioning and surface hydraulic conductivity in a fen peat. Hydrol. Process. 11, 287–295. doi:10.1002/(SICI)1099-1085(19970315)11:3<287::AID-HYP443>3.0.CO;2-L
Beckwith, C. W., Baird, A. J., and Heathwaite, A. L. (2003). Anisotropy and depth related heterogeneity of hydraulic conductivity in a bog peat: I. Laboratory measurements. Hydrol. Process. 17, 89–101. doi:10.1002/hyp.1116
Beven, K., and Germann, P. (1982). Macropores and water flow in soils. Water Resour. Res. 18, 1311–1325. doi:10.1029/WR018i005p01311
Boelter, D. H. (1968). “Important physical properties of peat materials,” in Proceedings of the third international peat congress, August 18-23 (Ottawa, Canada: Dep. of Energy, Mines and Resources), 150–154.
Bragazza, L., Parisod, J., Buttler, A., and Bardgett, R. D. (2013). Biogeochemical plant–soil microbe feedback in response to climate warming in peatlands. Nat. Clim. Change 3, 273–277. doi:10.1038/nclimate1781
Branham, J. E., and Strack, M. (2014). Saturated hydraulic conductivity in Sphagnum dominated peatlands: Do microforms matter? Hydrol. Process. 28, 4352–4362. doi:10.1002/hyp.10228
Byun, E., Rezanezhad, F., Fairbairn, L., Slowinski, S., Basiliko, N., Price, J. S., et al. (2021). Temperature, moisture and freeze–thaw controls on CO2 production in soil incubations from northern peatlands. Sci. Rep. 11, 23219. doi:10.1038/s41598-021-02606-3
Cameron, K. C., and Buchan, G. D. (2016). “Porosity: Pore size distribution,” in Encyclopedia of soil science. Editor R. Lal. third ed (Boca Raton, Florida: CRC Press).
Cunliffe, A. M., Baird, A. J., and Holden, J. (2013). Hydrological hotspots in blanket peatlands: Spatial variation in peat permeability around a natural soil pipe. Water Resour. Res. 49, 5342–5354. doi:10.1002/wrcr.20435
Ding, B., Rezanezhad, F., Gharedaghloo, B., Van Cappellen, P., and Passeport, E. (2019). Bioretention cells under cold climate conditions: Effects of freezing and thawing on water infiltration, soil structure, and nutrient removal. Sci. Total Environ. 649, 749–759. doi:10.1016/j.scitotenv.2018.08.366
Dörsch, P., Palojärvi, A., and Mommertz, S. (2004). Overwinter greenhouse gas fluxes in two contrasting agricultural habitats. Nutr. Cycl. Agroecosyst. 70, 117–133. doi:10.1023/B:FRES.0000048473.11362.63
Evans, C. D., Chapman, P. J., Clark, J. M., Monteith, D. T., and Cresser, M. S. (2006). Alternative explanations for rising dissolved organic carbon export from organic soils. Glob. Chang. Biol. 12 (11), 2044–2053. doi:10.1111/j.1365-2486.2006.01241.x
Evans, C. D., Renou-Wilson, F., and Strack, M. (2016). The role of waterborne carbon in the greenhouse gas balance of drained and re-wetted peatlands. Aquat. Sci. 78, 573–590. doi:10.1007/s00027-015-0447-y
Fan, W., Yang, P., and Yang, Z. J. (2021). Freeze-thaw impact on macropore structure of clay by 3D X-ray computed tomography. Eng. Geol. 280, 105921. doi:10.1016/j.enggeo.2020.105921
Feng, Z., Li, Z., Li, P., and Xiao, L. (2020). Effects of freeze-thaw cycles and soil moisture content on soil available micronutrients on aggregate scale in natural grassland and Chinese pine forestland on the Loess Plateau, China. J. Soils Sediments 20, 4023–4033. doi:10.1007/s11368-020-02706-z
Freeman, C., Evans, C. D., Monteith, D. T., Reynolds, B., and Fenner, N. (2001). Export of organic carbon from peat soils. Nature 412, 785. doi:10.1038/35090628
Gharedaghloo, B., and Price, J. S. (2019). Characterizing the immiscible transport properties of diesel and water in peat soil. J. Contam. Hydrol. 221, 11–25. doi:10.1016/j.jconhyd.2018.12.005
Hanson, P. J., Griffiths, N. A., Iversen, C. M., Norby, R. J., Sebestyen, S. D., Phillips, J. R., et al. (2020). Rapid net carbon loss from a whole‐ecosystem warmed Peatland. AGU Adv. 1, e2020AV000163. doi:10.1029/2020AV000163
Hayashi, M. (2013). The cold vadose zone: Hydrological and ecological significance of frozen-soil processes. Vadose Zone J. 12, 1–8. doi:10.2136/vzj2013.03.0064
Heinz, M., and Zak, D. (2018). Storage effects on quantity and composition of dissolved organic carbon and nitrogen of lake water, leaf leachate and peat soil water. Water Res. 130, 98–104. doi:10.1016/j.watres.2017.11.053
Henry, H. A. (2007). Soil freeze–thaw cycle experiments: Trends, methodological weaknesses and suggested improvements. Soil Biol. Biochem. 39, 977–986. doi:10.1016/j.soilbio.2006.11.017
Holden, J., Burt, T. P., and Cox, N. J. (2001). Macroporosity and infiltration in blanket peat: The implications of tension disc infiltrometer measurements. Hydrol. Process. 15, 289–303. doi:10.1002/hyp.93
Holden, J. (2009). Flow through macropores of different size classes in blanket peat. J. Hydrol. X. 364, 342–348. doi:10.1016/j.jhydrol.2008.11.010
Holden, J., Smart, R. P., Dinsmore, K. J., Baird, A. J., Billett, M. F., and Chapman, P. J. (2012). Natural pipes in blanket peatlands: Major point sources for the release of carbon to the aquatic system. Glob. Chang. Biol. 18, 3568–3580. doi:10.1111/gcb.12004
Hooijer, A., Page, S., Jauhiainen, J., Lee, W. A., Lu, X. X., Idris, A., et al. (2012). Subsidence and carbon loss in drained tropical peatlands. Biogeosciences 9, 1053–1071. doi:10.5194/bg-9-1053-2012
Hribljan, J. A., Kane, E. S., Pypker, T. G., and Chimner, R. A. (2014). The effect of long-term water table manipulations on dissolved organic carbon dynamics in a poor fen peatland. J. Geophys. Res. Biogeosci. 119, 577–595. doi:10.1002/2013JG002527
Kechavarzi, C., Dawson, Q., and Leeds-Harrison, P. (2010). Physical properties of low-lying agricultural peat soils in England. Geoderma 154, 196–202. doi:10.1016/j.geoderma.2009.08.018
Kettridge, N., and Binley, A. (2010). Evaluating the effect of using artificial pore water on the quality of laboratory hydraulic conductivity measurements of peat. Hydrol. Process. 24, 2629–2640. doi:10.1002/hyp.7693
King, E., Rezanezhad, F., and Wagner-Riddle, C. (2021). Evidence for microbial rather than aggregate origin of substrates fueling freeze-thaw induced N2O emissions. Soil Biol. Biochem. 160, 108352. doi:10.1016/j.soilbio.2021.108352
Koponen, H. T., Jaakkola, T., Keinänen-Toivola, M. M., Kaipainen, S., Tuomainen, J., Servomaa, K., et al. (2006). Microbial communities, biomass, and activities in soils as affected by freeze thaw cycles. Soil Biol. Biochem. 38, 1861–1871. doi:10.1016/j.soilbio.2005.12.010
Kreyling, J., Beierkuhnlein, C., Pritsch, K., Schloter, M., and Jentsch, A. (2008). Recurrent soil freeze-thaw cycles enhance grassland productivity. New Phytol. 177, 938–945. doi:10.1111/j.1469-8137.2007.02309.x
Kreyling, J., Tanneberger, F., Jansen, F., van der Linden, S., Aggenbach, C., Blüml, V., et al. (2021). Rewetting does not return drained fen peatlands to their old selves. Nat. Commun. 12, 5693. doi:10.1038/s41467-021-25619-y
Leifeld, J., and Menichetti, L. (2018). The underappreciated potential of peatlands in global climate change mitigation strategies. Nat. Commun. 9, 1071. doi:10.1038/s41467-018-03406-6
Letts, M. G., Roulet, N. T., Comer, N. T., Skarupa, M. R., and Verseghy, D. L. (2000). Parametrization of peatland hydraulic properties for the Canadian land surface scheme. Atmosphere-Ocean 38, 141–160. doi:10.1080/07055900.2000.9649643
Leuther, F., and Schlüter, S. (2021). Impact of freeze–thaw cycles on soil structure and soil hydraulic properties. Soil 7, 179–191. doi:10.5194/soil-7-179-2021
Liu, B., Ma, R., and Fan, H. (2021). Evaluation of the impact of freeze-thaw cycles on pore structure characteristics of black soil using X-ray computed tomography. Soil Tillage Res. 206, 104810. doi:10.1016/j.still.2020.104810
Liu, H., Janssen, M., and Lennartz, B. (2016). Changes in flow and transport patterns in fen peat following soil degradation. Eur. J. Soil Sci. 67, 763–772. doi:10.1111/ejss.12380
Liu, H., and Lennartz, B. (2019). Hydraulic properties of peat soils along a bulk density gradient—a meta study. Hydrol. Process. 33, 101–114. doi:10.1002/hyp.13314
Liu, H., Price, J., Rezanezhad, F., and Lennartz, B. (2020). Centennial‐scale shifts in hydrophysical properties of peat induced by drainage. Water Resour. Res. 56, e2020WR027538. doi:10.1029/2020WR027538
Liu, H., Rezanezhad, F., and Lennartz, B. (2022). Impact of land management on available water capacity and water storage of peatlands. Geoderma 406115521, 115521. doi:10.1016/j.geoderma.2021.115521
Ma, Q., Zhang, K., Jabro, J. D., Ren, L., and Liu, H. (2019). Freeze–thaw cycles effects on soil physical properties under different degraded conditions in Northeast China. Environ. Earth Sci. 78, 321. doi:10.1007/s12665-019-8323-z
Matzner, E., and Borken, W. (2008). Do freeze–thaw events enhance C and N losses from soils of different ecosystems? A review. Eur. J. Soil Sci. 59, 274–284. doi:10.1111/j.1365-2389.2007.00992.x
McCarter, C., Rezanezhad, F., Gharedaghloo, B., Price, J., and Van Cappellen, P. (2019). Transport of chloride and deuterated water in peat: The role of anion exclusion, diffusion, and anion adsorption in a dual porosity organic media. J. Contam. Hydrol. 225, 103497. doi:10.1016/j.jconhyd.2019.103497
McCarter, C., Rezanezhad, F., Quinton, W. L., Gharedaghloo, B., Lennartz, B., Price, J., et al. (2020). Pore‐scale controls on hydrological and geochemical processes in peat: Implications on interacting processes. Earth. Sci. Rev. 207, 103227. doi:10.1016/j.earscirev.2020.103227
Monteith, D., Stoddard, J., Evans, C., de Wit, H. A., Forsius, M., Hogasen, T., et al. (2007). Dissolved organic carbon trends resulting from changes in atmospheric deposition chemistry. Nature 450, 537–540. doi:10.1038/nature06316
Morris, P. J., Baird, A. J., and Belyea, L. R. (2015). Bridging the gap between models and measurements of peat hydraulic conductivity. Water Resour. Res. 51, 5353–5364. doi:10.1002/2015WR017264
Morris, P. J., Baird, A. J., Eades, P. A., and Surridge, B. W. J. (2019). Controls on near-surface hydraulic conductivity in a raised bog. Water Resour. Res. 55, 1531–1543. doi:10.1029/2018WR024566
Olefeldt, D., and Roulet, N. (2014). Permafrost conditions in peatlands regulate magnitude, timing, and chemical composition of catchment dissolved organic carbon export. Glob. Change Biol. 20, 3122–3136. doi:10.1111/gcb.12607
Othman, M. A., and Benson, C. H. (1993). Effect of freeze–thaw on the hydraulic conductivity and morphology of compacted clay. Can. Geotech. J. 30, 236–246. doi:10.1139/t93-020
Päivänen, J. (1973). Hydraulic conductivity and water retention in peat soils. Acta For. Fenn. 129, 1–70. doi:10.14214/aff.7563
Patel, K. F., Tatariw, C., MacRae, J. D., Ohno, T., Nelson, S. J., and Fernandez, I. J. (2021). Repeated freeze–thaw cycles increase extractable, but not total, carbon and nitrogen in a Maine coniferous soil. Geoderma 402, 115353. doi:10.1016/j.geoderma.2021.115353
Payandi-Rolland, D., Shirokova, L. S., Labonne, F., Bénézeth, P., and Pokrovsky, O. S. (2021). Impact of freeze-thaw cycles on organic carbon and metals in waters of permafrost peatlands. Chemosphere 279, 130510. doi:10.1016/j.chemosphere.2021.130510
Pokrovsky, O. S., Karlsson, J., and Giesler, R. (2018). Freeze-thaw cycles of Arctic thaw ponds remove colloidal metals and generate low-molecular-weight organic matter. Biogeochemistry 137, 321–336. doi:10.1007/s10533-018-0421-6
R Core Team (2020). R: A language and environment for statistical computing. Vienna, Austria: R Foundation for Statistical Computing.
Rezanezhad, F., Price, J. S., Quinton, W. L., Lennartz, B., Milojevic, T., and van Cappellen, P. (2016). Structure of peat soils and implications for water storage, flow and solute transport: A review update for geochemists. Chem. Geol. 429, 75–84. doi:10.1016/j.chemgeo.2016.03.010
Ritson, J. P., Brazier, R. E., Graham, N. J. D., Freeman, C., Templeton, M. R., and Clark, J. M. (2017). The effect of drought on dissolved organic carbon (DOC) release from peatland soil and vegetation sources. Biogeosciences 14, 2891–2902. doi:10.5194/bg-14-2891-2017
Rooney, E. C., Bailey, V. L., Patel, K. F., Possinger, A. R., Gallo, A. C., Bergmann, M., et al. (2022). The impact of freeze-thaw history on soil carbon response to experimental freeze-thaw cycles. JGR. Biogeosciences 127, e2022JG006889. doi:10.1029/2022JG006889
Roy, D., Jia, X., Chu, X., and Jacobs, J. M. (2021). Hydraulic conductivity measurement for three frozen and unfrozen soils in the red river of the north basin. Trans. ASABE 64 (3), 761–770. doi:10.13031/trans.14224
Schwärzel, K., Šimůnek, J., Stoffregen, H., Wessolek, G., and van Genuchten, M. T. (2006). Estimation of the unsaturated hydraulic conductivity of peat soils. Laboratory versus field data. Vadose Zone J. 5 (2), 628–640. doi:10.2136/vzj2005.0061
Sirianni, M., and Comas, X. (2020). Changes in physical properties of everglades peat soils induced by increased salinity at the laboratory scale: Implications for changes in biogenic gas dynamics. Water Resour. Res. 56, e2019WR026144. doi:10.1029/2019WR026144
Song, Y., Zou, Y., Wang, G., and Yu, X. (2017). Altered soil carbon and nitrogen cycles due to the freeze-thaw effect: A meta-analysis. Soil Biol. Biochem. 109, 35–49. doi:10.1016/j.soilbio.2017.01.020
Starkloff, T., Larsbo, M., Stolte, J., Hessel, R., and Ritsema, C. (2017). Quantifying the impact of a succession of freezing-thawing cycles on the pore network of a silty clay loam and a loamy sand topsoil using X-ray tomography. Catena 156, 365–374. doi:10.1016/j.catena.2017.04.026
Tanneberger, F., Appulo, L., Ewert, S., Lakner, S., Brolchain, N. O., Peters, J., et al. (2021). The power of nature‐based solutions: How peatlands can help us to achieve key EU sustainability objectives. Adv. Sustain. Syst. 5, 2000146. doi:10.1002/adsu.202000146
Tierney, G., Fahey, T. J., Groffman, P. M., Hardy, J. P., Fitzhugh, R. D., and Driscoll, C. T. (2001). Soil freezing alters fine root dynamics in a northern hardwood forest. Biogeochemistry 56, 175–190. doi:10.1023/A:1013072519889
Wallor, E., Rosskopf, N., and Zeitz, J. (2018). Hydraulic properties of drained and cultivated fen soils part I - Horizon-based evaluation of van Genuchten parameters considering the state of moorsh-forming process. Geoderma 313 (1), 69–81. doi:10.1016/j.geoderma.2017.10.026
Wang, J. Y., Song, C. C., Hou, A. X., Miao, Y. Q., Yang, G. S., and Zhang, J. (2014). Effects of freezing thawing cycle on peatland active organic carbon fractions and enzyme activities in the Da Xing'anling Mountains, Northeast China. Environ. Earth Sci. 72, 1853–1860. doi:10.1007/s12665-014-3094-z
Wang, M., Liu, H., and Lennartz, B. (2021). Small-scale spatial variability of hydro-physical properties of natural and degraded peat soils. Geoderma 399, 115123. doi:10.1016/j.geoderma.2021.115123
Wang, M., Liu, H., Zak, D., and Lennartz, B. (2020). Effect of anisotropy on solute transport in degraded fen peat soils. Hydrol. Process. 34, 2128–2138. doi:10.1002/hyp.13717
Wang, Y. B., Huang, Z., Qian, J. X., Li, T., Luo, J., Li, Z., et al. (2021). Freeze-thaw cycles aggravated the negative effects of moss-biocrusts on hydraulic conductivity in sandy land. Catena 207, 105638. doi:10.1016/j.catena.2021.105638
Weber, T. K. D., Iden, S. C., and Durner, W. (2017). Unsaturated hydraulic properties of Sphagnum moss and peat reveal trimodal pore-size distributions. Water Resour. Res. 53, 415–434. doi:10.1002/2016WR019707
Wei, X., Huang, C., Wei, N., Zhao, H., He, Y., and Wu, X. (2018). The impact of freeze-thaw cycles and soil moisture content at freezing on runoff and soil loss. Land Degrad. Dev. 30, 515–523. doi:10.1002/ldr.3243
Xu, J., Morris, P. J., Liu, J., Ledesma, J. L. J., and Holden, J. (2020). Increased dissolved organic carbon concentrations in peat‐fed UK water supplies under future climate and sulfate deposition scenarios. Water Resour. Res. 56, e2019WR025592. doi:10.1029/2019WR025592
Xu, W., Li, K., Chen, L., Kong, W., and Liu, C. (2021). The impacts of freeze–thaw cycles on saturated hydraulic conductivity and microstructure of saline–alkali soils. Sci. Rep. 11 (1), 18655. doi:10.1038/s41598-021-98208-0
Yang, Z., Zhu, D., Liu, L., Liu, X., and Chen, H. (2022). The effects of freeze–thaw cycles on methane emissions from peat soils of a high-altitude peatland. Front. Earth Sci. 10, 850220. doi:10.3389/feart.2022.850220
Yu, X., Zhang, Y., Zhao, H., Lu, X., and Wang, G. (2010). Freeze-thaw effects on sorption/desorption of dissolved organic carbon in wetland soils. Chin. Geogr. Sci. 20, 209–217. doi:10.1007/s11769-010-0209-7
Yu, X., Zou, Y., Jiang, M., Lu, X., and Wang, G. (2011). Response of soil constituents to freeze–thaw cycles in wetland soil solution. Soil Biol. Biochem. 43, 1308–1320. doi:10.1016/j.soilbio.2011.03.002
Zeitz, J., Fell, H., and Rosskopf, N. (2011). Peatland soils in Mecklenburg-Vorpommern: Quantity, quality and their soil functions. Telma 4, 107–132.
Zhang, Z., Ma, W., Feng, W., Xiao, D., and Hou, X. (2016). Reconstruction of soil particle composition during freeze-thaw cycling: A review. Pedosphere 26, 167–179. doi:10.1016/S1002-0160(15)60033-9
Keywords: freeze-thaw cycles, peat soils, dissolved organic carbon flux, saturated hydraulic conductivity, macroporosity, drained peatland
Citation: Liu H, Rezanezhad F, Zak D, Li X and Lennartz B (2022) Freeze-thaw cycles alter soil hydro-physical properties and dissolved organic carbon release from peat. Front. Environ. Sci. 10:930052. doi: 10.3389/fenvs.2022.930052
Received: 27 April 2022; Accepted: 19 July 2022;
Published: 05 September 2022.
Edited by:
Laodong Guo, University of Wisconsin–Milwaukee, United StatesReviewed by:
Erin Rooney, Oregon State University, United StatesCopyright © 2022 Liu, Rezanezhad, Zak, Li and Lennartz. This is an open-access article distributed under the terms of the Creative Commons Attribution License (CC BY). The use, distribution or reproduction in other forums is permitted, provided the original author(s) and the copyright owner(s) are credited and that the original publication in this journal is cited, in accordance with accepted academic practice. No use, distribution or reproduction is permitted which does not comply with these terms.
*Correspondence: Haojie Liu, aGFvamllLmxpdUB1bmktcm9zdG9jay5kZQ==
Disclaimer: All claims expressed in this article are solely those of the authors and do not necessarily represent those of their affiliated organizations, or those of the publisher, the editors and the reviewers. Any product that may be evaluated in this article or claim that may be made by its manufacturer is not guaranteed or endorsed by the publisher.
Research integrity at Frontiers
Learn more about the work of our research integrity team to safeguard the quality of each article we publish.