- Key Laboratory of Cultivated Land Quality Monitoring and Evaluation, Ministry of Agriculture and Rural Affairs, College of Environmental Science and Engineering, Yangzhou University, Yangzhou, China
In this research, a magnetic core-shell composite, consisting of a Fe3O4 core and a silica shell (called Fe3O4@SiO2), was developed and then functionalized via MnO2 grafting at different MnO2 deposition levels (termed Fe3O4@SiO2-MnO2). The resulting materials were characterized by X-ray fluorescence, X-ray diffraction, a vibration sample magnetometer, transmission electron microscopy, N2 adsorption-desorption, zeta-potential studies and X-ray photoelectron spectroscopy. Visualizations showed that Fe3O4@SiO2-MnO2 had a magnetite core with size of 100 nm, overlaid by a rough silica shell and a relatively loose MnO2 deposition. The Pb(II) adsorption onto the composites was also assessed. It was found that MnO2 deposition on the Fe3O4@SiO2 surface enhanced Pb(II) adsorption, and the Pb(II) adsorption amount was highly correlated to the MnO2 deposition level. The adsorption kinetics of Pb(II) followed pseudo-second-order kinetics, and the adsorption rate could be decreased by increasing the initial concentration of Pb(II). A higher pH resulted in enhanced Pb(II) adsorption, which slightly increased with the coexistence of Na+ and Ca2+, along with the presence of dissolved humic acid. The adsorbent could easily be separated and recovered under the action of the external magnetic component and it displayed stable adsorption behaviour over four adsorption-desorption periods. The results emphasize the high potential of Fe3O4@SiO2-MnO2 materials for the adsorptive removal of Pb(II) in water.
Introduction
Lead (Pb(II)) pollutant in municipal and industrial wastewater poses a serious threat to public health and ecological systems (Liu et al., 2018). Different treatment techniques, involving chemical precipitation (Li et al., 2022), ion exchange (Li et al., 2017), adsorption (Hussain et al., 2021), and membrane separation (Hamid et al., 2020), have been used to eliminate Pb(II) pollution, among which adsorption has the highest removal efficiency and ease of operation. Therefore, the design and preparation of effective adsorbents for aqueous Pb(II) removal are being widely researched.
Some metal oxides–such as Fe2O3, Al2O3, and MnO2–have been evaluated for adsorbing Pb(II) from wastewaters, among which MnO2 has exhibited promising potential for the adsorptive removal of Pb(II) (Zhang et al., 2020b; Yadav et al., 2021; Ghaedi et al., 2022). Zhang et al. (2017a) and Li et al. (2021) found that MnO2 presented a strong affinity for Pb(II) under acidic conditions and played a significant role in controlling the geochemical cycle of heavy metal ions owing to its high specific surface area and negatively charged surface over an extensive pH range. However, pure nanosized MnO2 particles tend to aggregate in water. The separation of MnO2 nanoparticles requires energy-intensive filtration, increasing the total cost and leading to filter clogging (Zhu et al., 2015).
Magnetic separation is quicker and more efficient than traditional filtration when used to separate suspended magnetic particles (Xia and Liu, 2021). Fe3O4 and γ-Fe2O3 are common magnetic agents, exhibiting superparamagnetic behaviour and strong magnetization. Combining their magnetic efficacy with that of MnO2 nanoparticles can help mitigate the limitation of MnO2 nanoparticles in terms of their slow separation from water. However, magnetic Fe3O4 and γ-Fe2O3 are not stable under acidic conditions (Bandar et al., 2021). Significant research effort has been devoted to enhancing the stability of magnetic iron oxides by coating them with polymers (Chen et al., 2018), lauric acid (Zhu et al., 2019), carbon (Wang W. et al., 2015), ZrO2 (Gugushe et al., 2021) and SiO2 (Vishnu and Dhandapani, 2020). Notably, SiO2-based coatings are very stable under acidic conditions and highly resistant to oxidants and reductants (Vishnu and Dhandapani, 2020). Hence, they can act as ideal shell composites to shield the inner magnetic cores. Furthermore, SiO2 has a high specific surface area and abundant hydroxyl groups, which facilitates facile functionalization. For instance, Huang et al. (2014) used M-Fe3O4@SiO2/ZnO for the photocatalytic degradation of methyl group, finding that SiO2 incorporation prevented the agglomeration of the Fe3O4 nanoparticles and enhanced the photocatalytic performance. Vishnu and Dhandapani (2020) synthesized an amino-functionalised silica-coated magnetic nanoparticle with high stability under acidic conditions. However, there have been few reports on the incorporation of MnO2 onto the surface of magnetic core-shell nanoparticles. Zhang et al. (2015) prepared a Fe3O4@SiO2@MnO2 composite as an adsorbent for the decolouration of methyl orange, which consisted of a magnetic Fe3O4 core, SiO2 shell–with thickness of 40 nm–and MnO2 nanoparticles adhering to the surface of SiO2 body, with a diameter of about 10 nm. However, the thick SiO2 body and large MnO2 nanoparticles decreased the saturation magnetisation (Ms) of Fe3O4 from 82.47 emu g−1–29.41 emu g−1, which markedly restricted the efficiency of magnetic separation and recovery of the adsorbent. Hence, we hypothesize that a nanocomposite of Fe3O4 core, a thin SiO2 shell and a monolayer of active MnO2 will exhibit a high adsorption ability and separability for Pb(II). However, to the best of our knowledge, no such study has been reported to date.
Magnetic nano-adsorbents were synthesized in this study by grafting MnO2 with various of concentrations onto the surface of Fe3O4@SiO2 core-shell nanoparticles. The characterization of the adsorbents included X-ray fluorescence (XRF), powder X-ray diffraction (XRD), transmission electron microscopy (TEM), vibration sample magnetometry (VSM), N2 adsorption-desorption, X-ray photoelectron spectroscopy (XPS) and zeta-potential tests. The Pb(II) adsorption capabilities of the materials including the adsorption ability, kinetics, the impact of water chemistry on adsorption, and the regeneration of the saturated adsorbents, were investigated to evaluate the performance of the novel adsorptive materials.
Materials and Methods
Adsorbent Preparation
The Fe3O4 nanoparticles were prepared using the procedure described by Huang et al. (2014) and Shi et al. (2021). In brief, 3.0 g of FeCl3·6H2O, 2.0 g of polyvinylpyrrolidone and 7.2 g of sodium acetate were dissolved in 60 ml ethylene glycol at 50°C. This was followed by transferring the compound to a 100 ml stainless steel autoclave lined with Teflon and heating it to 200°C for 8 h. Once the mixture’ temperature dropped to room temperature, the black solid was gathered through magnetic separation, repeatedly washed in ethanol and deionized water, and then dried at 60°C in a vacuum for 12 h.
After dispersing 1.0 g of Fe3O4 nanoparticles in 200 ml of deionized water at 80°C, 20 ml of 1.0 mol L−1 Na2SiO3 solution was added to the suspension with vigorous agitation. The pH was adjusted to 6.0 using 2.0 mol L−1 HCl solution within 1 h, followed by 3 h of vigorous agitation at 80°C. It was necessary to utilize magnetic separation to collect the resulting nanoparticles, which were then thoroughly washed with deionized water, and followed by drying for 12 h in a vacuum at 60°C. The material obtained is referred to as Fe3O4@SiO2.
KMnO4 as the source of manganese was used to prepare MnO2-functionalized Fe3O4@SiO2 nanoparticles. One gram of Fe3O4@SiO2 nanoparticles was suspended in 100 ml of deionized water, and the predicted amount of KMnO4 solution was added. The mixture was then added dropwise to 40 ml of 30% H2O2 solution. Within 0.5 h of mechanical stirring, the pH of this solution was adjusted to 7.0 by adding 1.0 mol L−1 HNO3. Following that, the reaction mixture was agitated at 25°C for 3 h. The final compound was collected through magnetic separation and repeatedly washed with deionized water before drying at 60°C in a vacuum for 12 h. The subsequent components were designated as Fe3O4@SiO2-MnO2(X), where X denotes the concentration of MnO2 (wt. per cent).
Material Characterization
The amount of MnO2 present in the adsorbents was measured using an ARL9800XP X-ray fluorescence (XRF) spectrometer (Thermo Electron, Switzerland). The X-ray diffraction (XRD) patterns of the materials were recorded using a Rigaku D/max-RA powder diffractometer connected to a Cu Kα radiation source over a wide range of angles (10–70°) (Rigaku, Japan). An electron microscopy, the JEM-2100 (JEM-2100, JEOL, Japan), was utilized to analyse the morphology of the adsorbents under investigation. Through the N2 adsorption-desorption operations performed on a Micromeritics ASAP 2020 analyser (Micromeritics Instrument Co., Norcross, GA, United States), we evaluated the Brunauer–Emmett–Teller (BET) surface area at −196°C (77 K). In this study, an XPS examination was carried out using a PHI5000 VersaProbe equipped with a monochromatized Al Kα excitation source (hv = 1,486.6 eV) (ULVAC-PHI, Japan). The binding energy was calibrated using the C 1s peak (284.6 eV). A vibrating sample magnetometer was used to determine the magnetic characteristics of the sample (Lake Shore VSM 7410, United States). A Zeta Potential Analyser was utilized to determine the nanoparticles’ surface Zeta (ζ) potentials (Zeta PALS, Brookhaven Instruments Co., United States). To ensure proper dispersion 24 h before the zeta potential measurement, 40 mg of the material was spread evenly across 400 ml of 1.0 mmol L−1 NaNO3 solution, and various solution pH values were obtained by adding 0.1 mol L−1 HNO3 or NaOH.
Batch adsorption Experiments
We determined the Pb(II) adsorption isotherms by conducting a series of batch adsorption tests. A 40 ml glass vial was filled with 40 ml of Pb(II) solution with various initial concentrations and capped with a screw cap containing 20.0 mg of adsorbent. The pH of the combination was maintained at 4.0 ± 0.2. The samples were then oscillated in an orbital shaker at 25°C for 24 h. Preliminarily kinetic trials (data available upon reasonable request) indicated that the adsorption time (24 h) was sufficient to reach an apparent adsorption equilibrium. Following the equilibrium adsorption, the adsorbent was magnetically isolated from the aqueous solutions. When determining the residual Pb(II) concentrations in aliquots, atomic absorption spectroscopy (AAS) (Hitachi Z-8100, Japan) was utilized. The equilibrium amount (mg g−1) of Pb(II) adsorption was determined as following:
The initial and equilibrium concentrations of Pb(II) are C0 and Ce (mg L−1), respectively. The total volume of Pb(II) solution is V (L) and the adsorbent mass M is measured in grams.
The Pb(II) adsorption data were fitted to the Langmuir and Freundlich models, which were often used to describe the interaction between the adsorbate and the adsorbent. The Langmuir and Freundlich models could be expressed as Eqs. 2, 3, respectively:
The maximum adsorption capacity is represented by qm (mg g−1), and b (L mg−1) is the Langmuir equilibrium constant. Kf (mg g−1) is the Freundlich coefficient, which indicates the adsorbent’s adsorption capacity, and n is the linearity index, which indicates the adsorption intensity.
Adsorption Kinetics
To investigate the Pb(II) adsorption kinetics, 250.0 mg of Fe3O4@SiO2-MnO2(1.54) was put into a round-bottom flask with 500 ml of aqueous solution with different Pb(II) concentrations (10.0 mg L−1 and 25.0 mg L−1) under vigorous stirring. About 5 ml of the solution was sampled using a pipette at preset time separations. The suspended magnetic nanoparticles were fast filtered by a 0.22 μm filter membrane. AAS was used to determine the concentration of Pb(II) in the filtrate. The quantity of Pb(II) adsorption was calculated using the following formula:
An adsorbing quantity, known as “qt” (mg g−1) is dependent on t (min). C0 and Ct are the Pb(II) solution concentration (mg L−1) at initial and time t of (mg L−1). The volume of Pb(II) solution is measured by V (L) and the adsorbent mass M is measured in grams.
Effect of Water Chemistry on Pb(II) adsorption
Using Fe3O4@SiO2-MnO2(1.54) at a temperature of 25°C, the influence of pH on Pb(II) adsorption was investigated. A 25.0 mg L−1 Pb(II) solution with variable pH was added to Teflon-lined, cap-on glass tubes containing 20.0 mg of Fe3O4@SiO2-MnO2(1.54), and the tubes were shaken at 25°C for 48 h. Equation 1 was used to compute the amount of Pb(II) adsorption, and the residual Pb(II) concentration was evaluated by AAS. The effects of co-existing ions on Pb(II) adsorption onto Fe3O4@SiO2-MnO2(1.54) were carried out with the presence of various NaCl or CaCl2 concentrations (0.01–0.20 mol L−1) at pH 4.0 ± 0.2, and at 25°C. The effect of dissolved humic substances on Pb(II) adsorption was studied by comparing the adsorption isotherms of Pb(II) in the presence and absence of dissolved humic acid (Sigma-Aldrich) at a concentration of 10.2 mg L−1.
Desorption and Regeneration of the Saturated Adsorbent
To determine the reusability of the synthesized sorbent, eight successive periods of Pb(II) adsorption and regeneration on Pb(II)-saturated Fe3O4@SiO2-MnO2 (1.54) were conducted. Specifically, 50.0 mg of Fe3O4@SiO2-MnO2(1.54) was suspended in 100 ml of 25.0 mg L−1 Pb(II) solution and maintained at 25°C for 24 h with vigorous stirring. The Pb(II)-saturated adsorbent was then split magnetically, and the residual Pb(II) concentration was measured by methods described previously. The resultant Pb(II)-saturated adsorbent was regenerated by suspending it in 20 ml of 1.0 mol L−1 HCl solution and keeping it for 24 h. Before the next adsorption-desorption period, the regenerated adsorbent was repeatedly cleaned using deionized water until the pH of the supernatant was almost neutral.
Results and Discussion
Characterization of the Adsorbents
The XRD diagrams of Fe3O4, Fe3O4@SiO2 and Fe3O4@SiO2-MnO2 with different MnO2 concentrations are shown in Figure 1. The major diffraction peaks of Fe3O4 were determined with 2θ of 18.3°, 30.1°, 35.5°, 37.1°, 43.1°, 53.5°, 57.1°, and 62.7°, this indicated the existence of magnetite with a cubic spinel framework (Bandar et al., 2021; Panahandeh et al., 2021). For Fe3O4@SiO2, no prominent and new crystalline peak was observed, revealing that SiO2 component in Fe3O4@SiO2 was amorphous (Bandar et al., 2021). Upon MnO2 functionalization, a broad peak at 2θ of 23.7° was detected (except for the diffraction peaks of Fe3O4@SiO2), the intensity of which enhanced with increasing MnO2 concentration, reflecting the presence of amorphous MnO2 moieties on the Fe3O4@SiO2 surface (Yin et al., 2011; Zhang et al., 2017b). Additionally, the few diffraction peak features of crystalline MnO2 reflected the low MnO2 content in the Fe3O4@SiO2-MnO2 adsorbents.
The BET surface areas of the adsorbents identified through N2 adsorption-desorption are summarized in Table 1. These specific surface areas of the Fe3O4@SiO2-MnO2 adsorbents were higher than that of Fe3O4@SiO2, revealing an increase in the surface area upon functionalization. The XRF analysis results of MnO2 loading amounts are listed in Table 1. The MnO2 contents of Fe3O4@SiO2-MnO2 adsorbents were 0.32, 0.62, 0.97, 1.54, and 1.92 (wt%), reflecting the successful grafting of MnO2 onto the Fe3O4@SiO2 surface.
The TEM images of Fe3O4, Fe3O4@SiO2 and Fe3O4@SiO2-MnO2 are displayed in Figure 2. The Fe3O4 image (Figure 2A) showed the aggregation of spherical clusters with an average diameter of 100 nm. In Figure 2B, rough SiO2 with a thickness of approximately 3 nm outside the Fe3O4 core was identified, reflecting that Fe3O4@SiO2 consists of Fe3O4 cores and SiO2 shells. After MnO2 functionalization (see Figures 2A,C,D relatively loose body was obviously observed on the Fe3O4@SiO2 surface, reflecting the successful deposition of MnO2 onto the Fe3O4@SiO2 surface.
The magnetization curves determined for Fe3O4@SiO2 and Fe3O4@SiO2-MnO2(1.54) are presented in Figure 3A. The saturation magnetisation (Ms) of Fe3O4@SiO2 was determined to be 84.1 emu g−1, whereas the Ms of Fe3O4 nanosphere was reported to around 100.3 emu g−1 (Shi et al., 2021). The lower saturation magnetization of Fe3O4@SiO2 than that of Fe3O4 could be attributed to the SiO2 shell. After MnO2 modification, the saturation magnetization of Fe3O4@SiO2-MnO2(1.54) continuously reduced to 56.8 emu g−1, further confirming the successful deposition of MnO2 onto the Fe3O4@SiO2 surface. VSM results also showed that the samples were superparamagnetic with no remanent coercivity (Xu et al., 2018). As evident in Figure 3B, the effective separation and recovery of Fe3O4@SiO2-MnO2(1.54) could be achieved in seconds with the assistance of an external magnetic field. Further, the nanoparticles quickly redispersed while the magnetic field was removed, reflecting the potential for using MnO2-functionalized Fe3O4@SiO2 magnetic nanoparticles as recyclable adsorbent in water and wastewater treatment.
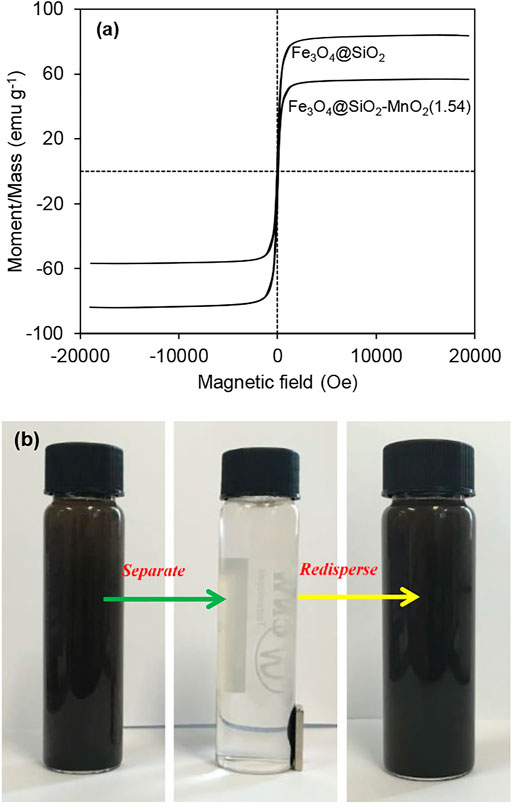
FIGURE 3. (A) Magnetization curves of Fe3O4@SiO2 and Fe3O4@SiO2-MnO2(1.54) and (B) the magnetic separation and redispersion of Fe3O4@SiO2-MnO2(1.54).
The surface zeta potentials of Fe3O4, Fe3O4@SiO2 and Fe3O4@SiO2-MnO2(1.54) are displayed in Figure 4, as a function of pH. The surface potential gradually decreased with increases in the pH for all of the samples, likely due to the deprotonated surface hydroxyl groups. The isoelectric point (IEP) of the Fe3O4 nanoparticles was found to be 5.8, which was in line with the value found in literature (Bandar et al., 2021). The IEP of Fe3O4@SiO2 was found to be 2.4, further confirming the successful amorphous silica coating (Wang et al., 2013). More specifically, the IEP of Fe3O4@SiO2-MnO2(1.54) was found to be approximately 1.4. It is important to note that the IEP of MnO2 has previously been found to lie within the range of 1–2 (McBride, 1997; Huang et al., 2014). Hence, the lower IEP of Fe3O4@SiO2-MnO2(1.54) compared with Fe3O4@SiO2 was attributed to the MnO2 grafting on the Fe3O4@SiO2 surface.
The XPS spectra of Mn 2p and O 1s are presented in Figures 5A,B, respectively. In the spectra of Fe3O4@SiO2-MnO2 adsorbents, Mn 2p peaks were found, reflecting the successful grafting of the MnO2 functional groups onto the Fe3O4@SiO2 surface. The typical Mn 2p XPS spectrum of Fe3O4@SiO2-MnO2 nanoparticles revealed a spin-orbit doublet with Mn 2p1/2 (653.3 eV) and Mn 2p3/2 (641.3 eV), which was separated into a mixed-valent manganese system comprising Mn3+ and Mn4+ (Zhang et al., 2015; Zhang et al., 2017b). Owing to the low MnO2 content and high MnO2 dispersion, the intensity of the Mn 2p1/2 and Mn 2p3/2 peaks of Fe3O4@SiO2-MnO2(0.32) and Fe3O4@SiO2-MnO2(0.62), respectively, were not the typical intensities reported for manganese oxides in literature (Zhang et al., 2020a). In the case of the O 1s spectrum (Figure 5B), two peaks at 529.8 and 532.1 eV appeared in the spectra of the samples, indicative of the lattice oxygen (i.e., Mn-O, Si-O and Fe-O) and surface oxygen (O-H) (Wan et al., 2020; Minale et al., 2021), respectively. Additionally, the area ratio of surface oxygen increased with increasing MnO2 deposition levels, which was ascribed to the abundance of hydroxyl groups on the functionalized manganese oxides (Wang et al., 2013).
Adsorption Isotherms
Figure 6A shows the isotherms of Pb(II) adsorption onto the adsorbents. Very low quantities of Pb(II) adsorption was detected for Fe3O4 and Fe3O4@SiO2, indicating that the Fe3O4 and Fe3O4@SiO2 exhibited a negligible affinity for Pb(II) adsorption. In comparison to Fe3O4 and Fe3O4@SiO2, Fe3O4@SiO2-MnO2 exhibited a significant increase in Pb(II) adsorption, which increased as the MnO2 deposition level rose, indicating that MnO2 was the active species for Pb(II) adsorption.
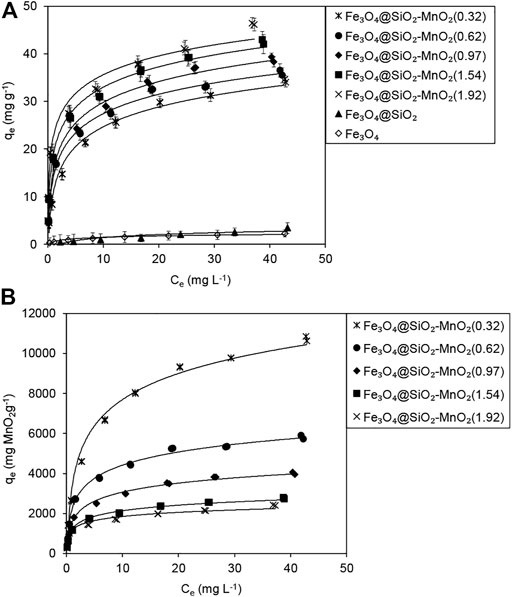
FIGURE 6. (A) Pb(II) adsorption isotherms and (B) MnO2 content-normalized Pb(II) adsorption isotherms of the samples. (Adsorbent dosage = 0.5 g L−1, Temperature = 25°C, pH = 4.0 ± 0.2).
Table 2 summarizes the fitting parameters. For Fe3O4@SiO2-MnO2(0.32), the Adj. R2 value (0.976) of the Freundlich model was slightly higher than that of the Langmuir model (0.965), likely because the Fe3O4@SiO2 surface was not entirely covered by MnO2 at the 0.32% (wt.) deposition level, leading to significant adsorption heterogeneity (Feheem, et al., 2016). In contrast, much higher R2 values (0.990–0.994) of the Langmuir model were obtained when the MnO2 deposition levels increased, indicating homogeneous adsorption sites for Pb(II) adsorption at high MnO2 deposition levels. Additionally, the b and Kf values increased with increasing MnO2 deposition levels, confirming that the active adsorption species was MnO2.
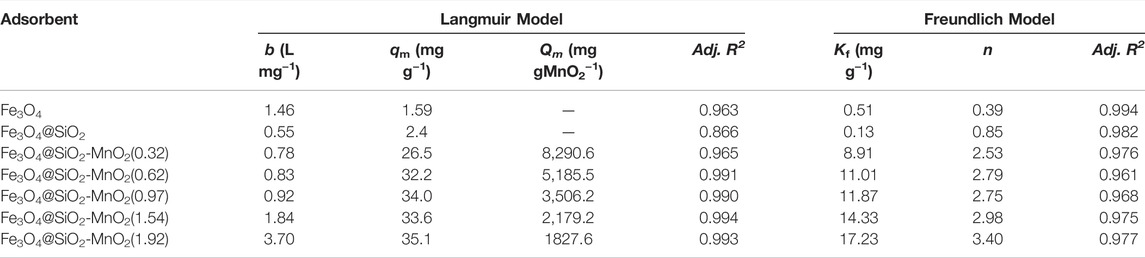
TABLE 2. Fitting parameters of the Langmuir and Freundlich isotherm models for adsorption of Pb(II) onto the adsorbents.
The calculated qm of the sorbents suggested that Pb(II) adsorption was not strongly affected by the MnO2 deposition levels. To obtain deeper insight into the adsorption mechanism, MnO2-content-normalized adsorption isotherms of Fe3O4@SiO2 with various MnO2 deposition levels are compared in Figure 6B. The calculated maximum adsorption capacities Qm upon MnO2 content normalization are listed in Table 2. For Fe3O4@SiO2-MnO2 with various MnO2 contents, the adsorption capacities Qm decreased with increasing MnO2 deposition levels, and the highest Qm was observed for Fe3O4@SiO2-MnO2(0.32). This is likely due to the high dispersion of nanosized MnO2 particles at low deposition levels, and thus, the high utilization of MnO2 as the active site for Pb(II) adsorption. At higher deposition levels, the MnO2 particles may aggregate, leading to the partial inaccessibility of the adsorption sites. Notably, almost identical adsorption isotherms were acquired for MnO2 normalization at deposition levels of 1.54 (wt%) and 1.92 (wt%), reflecting similar structural characteristics (e.g., the content of hydroxyl groups and the particle size) (Zhang et al., 2020a). The nearly identical normalized isotherms implied that the outer surface of SiO2 shell might be entirely covered at the 1.54% (wt.) MnO2 deposition, which was in good agreement with the XPS results.
MnO2 is used as an effective adsorbent for Pb(II) removal in wastewater. Wan et al. (2018) found that the maximum adsorption capacity of biochar-supported hydrated manganese oxide for Pb(II) was 23.8 mg g−1 at a pH of 4.5. Wang S. et al. (2015) investigated Pb(II) adsorption on MnO2-modified biochar acquired an adsorption capacity of 47.1 mg g−1 at an equilibrium concentration of 40 mg L−1. Note that the adsorption capacity usually increases with the equilibrium concentration of the adsorbate, and these high adsorption capacities reported were obtained at relatively high Pb(II) equilibrium concentrations. In the current research, the maximum adsorption of Pb(II) on Fe3O4@SiO2-MnO2(1.54) was 33.6 mg g−1 Fe3O4@SiO2-MnO2 at an equilibrium concentration of 24.0 mg L−1 and pH of 4.0, showing that the uptake of Pb(II) at low Pb(II) concentrations with the synthesized sorbents was much higher than the reported results and, therefore, a higher efficiency of Pb(II) enrichment was achieved under acidic conditions.
Adsorption Kinetics
The kinetics of Pb(II) adsorption on Fe3O4@SiO2-MnO2(1.54) with different initial concentrations are depicted in Figure 7A. In roughly 30 min, equilibrium was reached at both initial concentrations of 10.0 and 25.0 mg L−1 of Pb(II) on the adsorbent. Adsorption kinetics can be better understood using three kinetic models (Yang et al., 2018): the pseudo-first-order model (Eq. 5), the pseudo-second-order model (Eq. 6), and the Weber-Morris model (Eq. 7).
where the qt (mg g−1) is the adsorption amount at time t (min), k1 (min−1) is the pseudo-first-order rate constant, k2 (g mg−1 min−1) is pseudo-second-order rate constant, kd and I denote the diffusion constant and the thickness of the boundary layer, respectively.
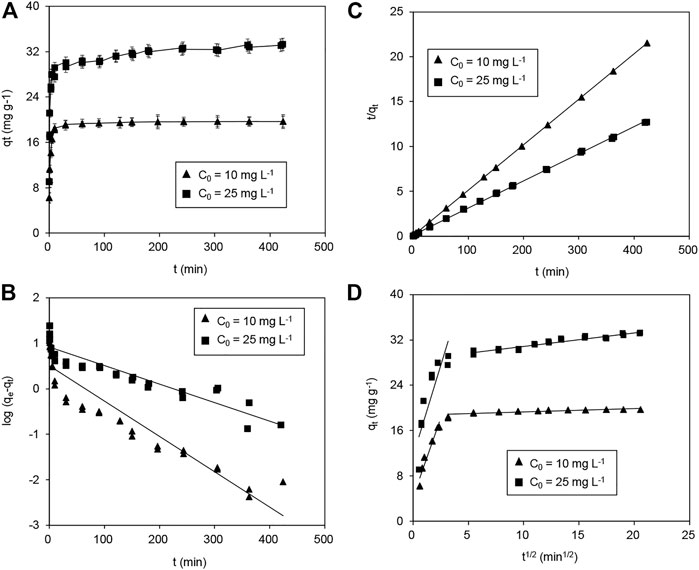
FIGURE 7. (A) Adsorption of Pb(II) with various initial concentrations on Fe3O4@SiO2-MnO2(1.54) as a function of the adsorption time, and fitting of Pb(II) adsorption kinetics using the (B) pseudo-first-order model, (C) pseudo-second-order model and (D) Weber-Morris model. (C0 (Pb(II) = 10.0 mg L−1 and 25.0 mg L−1, respectively, adsorbent dosage = 0.5 g L−1, Temperature = 25°C, pH = 4.0 ± 0.2).
Figures 7B,C show change curves of log(qe - qt) with t and t/qt with t; Table 3 contains the fitting parameters. The kinetic results for Pb(II) adsorption onto Fe3O4@SiO2-MnO2(1.54) fit this pseudo-second-order kinetic model very well, showing that Pb(II) adsorption is pseudo-second-order and chemisorption-limited (Panahandeh et al., 2021). Pb(II) adsorbed over Fe3O4@SiO2-MnO2(1.54) was shown to adhere to pseudo-second-order kinetics when the slopes of the t/qt against t plots were used. The projected q′cal significant values were very close to the experimental values. There were slower adsorption rates with higher initial Pb(II) concentrations for the Pb(II) adsorption rate constants at 10.0 and 25.0 mg L−1 starting concentrations of Pb(II).

TABLE 3. Fitting parameters of Pb(II) adsorption kinetics on Fe3O4@SiO2-MnO2(1.54) using pseudo-first-order, pseudo-second-order, and Weber-Morris models.
The fitted results of Pb(II) adsorption using Weber-Morris model are shown in Figure 7D, and the parameters that best fit the data are listed in Table 3. The plots display multilinearity with different initial Pb(II) concentrations, indicating that more than two procedures are involved in the adsorption. In Figure 7D, the Weber-Morris plots had two linear portions. The first is assigned to the fast adsorption of Pb(II), and the other to the function of the adsorption equilibrium. According to the model, if the adsorption process is mainly controlled by intraparticle diffusion, the qt - t1/2 plot will be a straight line passing through the origin (Yang et al., 2018). However, the first portion did not pass through initially indicate that external diffusion played a significant role in the adsorption procedure. Additionally, the intercepts decreased at high initial concentrations, suggesting that the diffusion was not substantially affected by the mass transfer resistance. With initial Pb(II) concentrations of 10.0 and 25.0 mg L−1, respectively, the kd were 5.5 and 7.5 mg g−1 min−1/2, indicating a higher diffusion constant at a higher initial concentration. This is likely due to the increasing diffusion driving force caused by the increasing concentration gradient.
Impact of Solution Chemistry on Pb(II) adsorption
The impact of the solution pH on Pb(II) adsorption by Fe3O4@SiO2-MnO2(1.54) is presented in Figure 8. The Pb(II) adsorption amount monotonically increased from 0.2 to 48.8 mg g−1 in the pH range from 2.0 to 8.0; subsequently, it remained constant. The pH effect on Pb(II) adsorption could be explained by the electrostatic interaction between the adsorbent and Pb(II), and the precipitation of Pb(II) at high pH conditions (>6.0) (Zhang et al., 2017b). Notably, SiO2 based shell were not stable against alkaline condition. Although the Pb(II) adsorption capacity of the Fe3O4@SiO2-MnO2(1.54) was relatively high under high pH conditions, each adsorption performance test in this study was conducted at pH = 4.0 ± 0.2. Generally, the Fe3O4@SiO2-MnO2(1.54) surface was actively charged at solution pH values under the IEP of 1.4; electrostatic repulsive interaction occurred between Pb(II) and the protonated hydroxyl groups (Wang et al., 2020). In contrast, the adsorption sites were negatively charged at solution pH values above the IEP of 1.4, leading to an electrostatic attraction between deprotonated Mn-OH groups and Pb(II) (Zhang et al., 2020b), enhancing the Pb(II) adsorption.
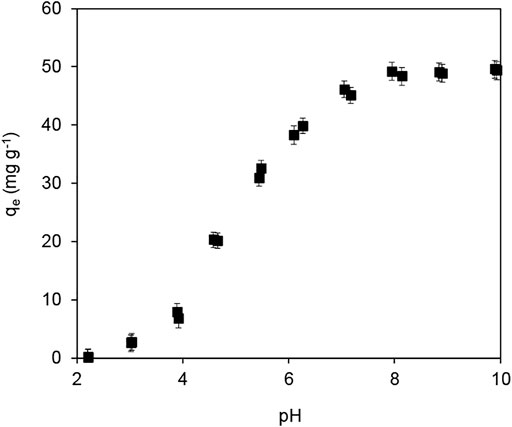
FIGURE 8. Effect of the solution pH on Pb(II) adsorption by Fe3O4@SiO2-MnO2(1.54). (C0 (Pb(II) = 25.0 mg L−1, adsorbent dosage = 0.5 g L−1, Temperature = 25°C).
Alkaline/Earth metal ions in natural water and wastewater may compete against Pb(II) for available adsorption sites of sorbents. The impacts of co-existing Na+ and Ca2+ on Pb(II) adsorption are shown in Figure 9A. These electrolytes resulted in an increase in the Pb(II) adsorption, and Ca2+ had a more significant impact on adsorption than Na+ did, suggesting that Pb(II) adsorption was controlled mainly by a surface complexation model (Zhang et al., 2020b). Notably, the effects of co-existing Na+ and Ca2+ on Pb(II) adsorption revealed the presence of a preferable adsorbent, which exhibited great potential for Pb(II) treatment in the presence of co-existing cations at high concentrations.
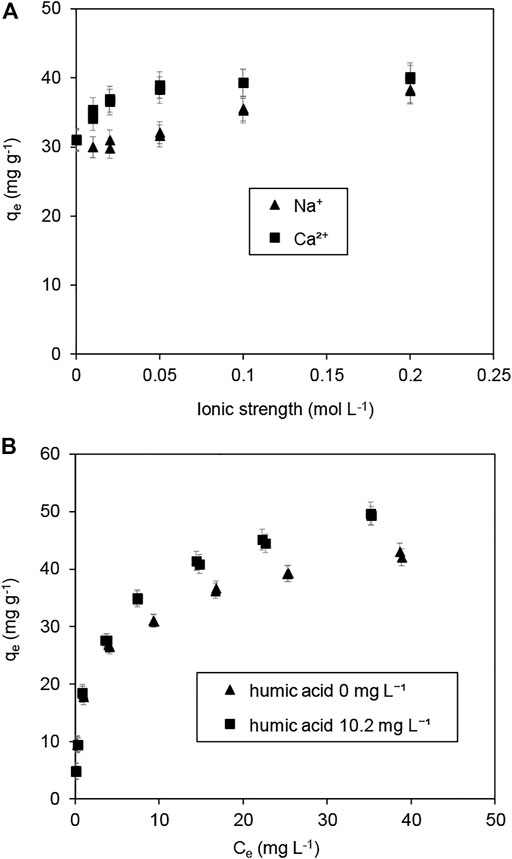
FIGURE 9. (A) Effect of co-existing ions on Pb(II) adsorption over Fe3O4@SiO2-MnO2(1.54), and (B) adsorption isotherms of Pb(II) on Fe3O4@SiO2-MnO2(1.54) in the absence and presence of dissolved humic acid. (Adsorbent dosage = 0.5 g L−1, Temperature = 25°C, pH = 4.0 ± 0.2).
In aquatic systems, dissolved humic acid may have an effect on the adsorption of Pb(II) (Esfandiar et al., 2022). Figure 9B depicts the adsorption isotherms of Pb(II) on Fe3O4@SiO2-MnO2(1.54) in the absence and presence of dissolved humic acid at a concentration of 10.2 mg L−1. The results indicate the active effect of dissolved humic acid on Pb(II) adsorption at higher equilibrium Pb(II) concentrations under the tested conditions. This positive effect is attributed to the formation of weakly adsorbing complexes between Pb(II) and humic acid (Zhang et al., 2017a).
Regeneration and Reuse of the Adsorbent
Based on the finding that Fe3O4@SiO2-MnO2 had a poor adsorption capacity at low pH values, the Pb(II)-saturated adsorbent was thought to be regenerated through the acid treatment. The adsorbent was reused for eight adsorption-regeneration periods and the results are displayed in Figure 10. The adsorption capacity reduced from 31.4 to 25.6 mg g−1 during the initial four adsorption-desorption cycles, likely because of partial active species loss during cycle processes or the incomplete desorption of Pb(II) from the surface of Fe3O4@SiO2-MnO2(1.54). After four cycles, the Fe3O4@SiO2-MnO2(1.54) adsorption capacity remained constant, and the sorbent could be efficiently separated from the solution within 10 s by an external magnetic field, indicating that the sorbent was stable and recyclable.
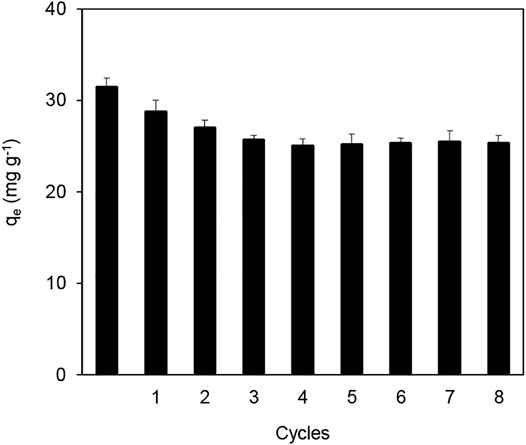
FIGURE 10. Pb(II) adsorption by virgin and regenerated Fe3O4@SiO2-MnO2(1.54) adsorbent within eight adsorption-desorption cycles. (C0 (Pb(II) = 25.0 mg L−1, adsorbent dosage = 0.5 g L−1, Temperature = 25°C, Pb(II) adsorption pH = 4.0 ± 0.2).
Implication for Real-World Application
Efficient removal of Pb(II) in wastewater and rapid separation of adsorbents have always been the difficulties in the practical application of adsorbents. The as-prepared Fe3O4@SiO2-MnO2 showed high adsorption capacity and good stability for Pb(II) adsorptive removal from acidic Pb(II)-contaminated water, suggesting potential application in wastewater treatment field. Additionally, after adsorbing Pb(II) in wastewater the effective separation and recovery of Fe3O4@SiO2-MnO2 adsorbent could be accomplished within seconds with an external magnetic field. Furthermore, the Fe3O4@SiO2-MnO2 adsorbent could be rapidly redispersed while the magnetic field was removed. This means that only one external magnetic field can be used to quickly and efficiently realize the adsorption and desorption of Pb(II) by the adsorbent, which is beneficial to reduce the cost of adsorptive removal of Pb(II) in wastewater.
Conclusion
In this research, magnetic core-shell sorbents of Fe3O4@SiO2-MnO2 with various MnO2 deposition contents were synthesized through deposition-precipitation and used for Pb(II) adsorption. The characterization results revealed that the Fe3O4 core was coated by a rough silica shell and a relatively loose MnO2 deposition. Accordingly, Fe3O4@SiO2-MnO2 demonstrated increased Pb(II) adsorption in comparison to Fe3O4@SiO2, and the Pb(II) adsorption amount was positively related to the MnO2 deposition level. Fe3O4@SiO2-MnO2 could be easily divided and recovered using an outer magnetic component. The sorbent exhibited stable adsorption and regeneration performance after four consecutive adsorption-desorption periods. This adsorption was contained by surface complexation and slightly enhanced by dissolved humic acid and co-existing cations. These findings highlight that Fe3O4@SiO2-MnO2 is a promising adsorbent for use in the removal of Pb(II) from water and wastewater.
Data Availability Statement
The original contributions presented in the study are included in the article/Supplementary Material, further inquiries can be directed to the corresponding author.
Author Contributions
HZ contributed to data curation, methodology, writing-original draft, and writing-review and editing; SC contributed to data curation and investigation; YS and JW designed all experiments and revised and examined the manuscript; XQ contributed to review and editing; YY contributed to review and editing. All authors have read and agreed to the published version of the manuscript.
Fundings
This work was supported by the National Natural Science Foundation of China (31872179, 31901447, 41701093), the Key R&D Plan Program of Jiangsu Province (BE2020319), the Innovation and Entrepreneurship Program of Jiangsu Province (JSSCBS20211062), the Excellent Doctor in Lvyangjinfeng of Yangzhou City (YZLYJFJH2021YXBS155), and the Blue Project of Yangzhou University.
Conflict of Interest
The authors declare that the research was conducted in the absence of any commercial or financial relationships that could be construed as a potential conflict of interest.
Publisher’s Note
All claims expressed in this article are solely those of the authors and do not necessarily represent those of their affiliated organizations, or those of the publisher, the editors and the reviewers. Any product that may be evaluated in this article, or claim that may be made by its manufacturer, is not guaranteed or endorsed by the publisher.
References
Bandar, S., Anbia, M., and Salehi, S. (2021). Comparison of MnO2 Modified and Unmodified Magnetic Fe3O4 Nanoparticle Adsorbents and Their Potential to Remove Iron and Manganese from Aqueous Media. J. Alloys Compd. 851, 156822. doi:10.1016/j.jallcom.2020.156822
Chen, Y., Wang, M., Hu, Y., and Han, J. (2018). Poly(2-Aminothiophenol)/MnO2 Hierarchical Nanocables as Efficient Adsorbents towards Heavy Metal Ions. Mater. Chem. Phys. 214, 172–179. doi:10.1016/j.matchemphys.2018.04.076
Esfandiar, N., Suri, R., and Mckenzie, E. R. (2022). Competitive Sorption of Cd, Cr, Cu, Ni, Pb and Zn from Stormwater Runoff by Five Low-Cost Sorbents; Effects of Co-Contaminants, Humic Acid, Salinity and pH. J. Hazard. Mater. 423, 126938. doi:10.1016/j.jhazmat.2021.126938
Faheem, , Yu, H. X., Liu, J., Shen, J. Y., Sun, X. Y., Li, J. S., et al. (2016). Preparation of MnOx-Loaded Biochar for Pb2+ Removal: Adsorption Performance and Possible Mechanism. J. Taiwan Inst. Chem. Eng. 66, 313–320. doi:10.1016/j.jtice.2016.07.010
Ghaedi, S., Seifpanahi-Shabani, K., and Sillanpää, M. (2022). Waste-to-resource: New Application of Modified Mine Silicate Waste to Removal Pb2+ Ion and Methylene Blue Dye, Adsorption Properties, Mechanism of Action and Recycling. Chemosphere 292, 133412. doi:10.1016/j.chemosphere.2021.133412
Gugushe, A. S., Mpupa, A., Munonde, T. S., Nyaba, L., and Nomngongo, P. N. (2021). Adsorptive Removal of Cd, Cu, Ni and Mn from Environmental Samples Using Fe3O4-ZrO2@APS Nanocomposite: Kinetic and Equilibrium Isotherm Studies. Molecules 26, 3209. doi:10.3390/molecules26113209
Hamid, S. A., Azha, S. F., Sellaoui, L., Bonilla-Petriciolet, A., and Ismail, S. (2020). Adsorption of Copper(II) Cation on Polysulfone/zeolite Blend Sheet Membrane: Synthesis, Characterization, Experiments and Adsorption Modelling. Colloids Surfaces A Physicochem. Eng. Aspects 601, 124980. doi:10.1016/j.colsurfa.2020.124980
Huang, S., Gu, L., Zhu, N., Feng, K., Yuan, H., Lou, Z., et al. (2014). Heavy Metal Recovery from Electroplating Wastewater by Synthesis of Mixed-Fe3O4@SiO2/metal Oxide Magnetite Photocatalysts. Green Chem. 16, 2696–2705. doi:10.1039/C3GC42496K
Hussain, T., Hussain, A. I., Chatha, S. A. S., Ali, A., Rizwan, M., Ali, S., et al. (2021). Synthesis and Characterization of Na-Zeolites from Textile Waste Ash and its Application for Removal of Lead (Pb) from Wastewater. Int. J. Environ. Res. Public Health 18, 3373. doi:10.3390/ijerph18073373
Li, Q., Fu, L., Wang, Z., Li, A., Shuang, C., and Gao, C. (2017). Synthesis and Characterization of a Novel Magnetic Cation Exchange Resin and its Application for Efficient Removal of Cu2+ and Ni2+ from Aqueous Solutions. J. Clean. Prod. 165, 801–810. doi:10.1016/j.jclepro.2017.06.150
Li, W., Li, Y., Liu, J., Chao, S., Yang, T., Li, L., et al. (2021). A Novel Hollow carbon@MnO2 Electrospun Nanofiber Adsorbent for Efficient Removal of Pb2+ in Wastewater. Chem. Res. Chin. Univ. 37, 496–504. doi:10.1007/s40242-021-1085-7
Li, M., Kuang, S., Kang, Y., Ma, H., Dong, J., and Guo, Z. (2022). Recent Advances in Application of Iron-Manganese Oxide Nanomaterials for Removal of Heavy Metals in the Aquatic Environment. Sci. Total Environ. 819, 153157. doi:10.1016/j.scitotenv.2022.153157
Liu, X., Bai, X., Dong, L., Liang, J., Jin, Y., Wei, Y., et al. (2018). Composting Enhances the Removal of Lead Ions in Aqueous Solution by Spent Mushroom Substrate: Biosorption and Precipitation. J. Clean. Prod. 200, 1–11. doi:10.1016/j.jclepro.2018.07.182
McBride, M. B. (1997). A Critique of Diffuse Double Layer Models Applied to Colloid and Surface Chemistry. Clays Clay Minerals 45, 598–608. doi:10.1346/CCMN.1997.0450412
Minale, M., Gu, Z., Guadie, A., Li, Y., Wang, Y., Meng, Y., et al. (2021). Hydrous Manganese Dioxide Modified Ploy(sodium Acrylate) Hydrogel Composite as a Novel Adsorbent for Enhanced Removal of Tetracycline and Lead from Water. Chemosphere 272, 129902. doi:10.1016/j.chemosphere.2021.129902
Panahandeh, A., Parvareh, A., and Moraveji, M. K. (2021). Synthesis and Characterization of γ-MnO2/chitosan/Fe3O4 Cross-Linked with EDTA and the Study of its Efficiency for the Elimination of Zinc(II) and Lead(II) from Wastewater. Environ. Sci. Pollut. Res. 28, 9235–9254. doi:10.1007/s11356-020-11359-x
Shi, Y., Han, Z., Yang, J., and Meng, Q. (2021). Influence of the Hollowness and Size Distribution on the Magnetic Properties of Fe3O4 Nanospheres. Langmuir 37, 9605–9612. doi:10.1021/acs.langmuir.1c01498
Vishnu, D., and Dhandapani, B. (2020). Integration of Cynodon Dactylon and Muraya Koenigii Plant Extracts in Amino-Functionalised Silica-Coated Magnetic Nanoparticles as an Effective Sorbent for the Removal of Chromium(VI) Metal Pollutants. IET Nanobiotechnol. 14, 449–456. doi:10.1049/iet-nbt.2019.0313
Wan, S., Wu, J., Zhou, S., Wang, R., Gao, B., and He, F. (2018). Enhanced Lead and Cadmium Removal Using Biochar-Supported Hydrated Manganese Oxide (HMO) Nanoparticles: Behavior and Mechanism. Sci. Total Environ. 616-617, 1298–1306. doi:10.1016/j.scitotenv.2017.10.188
Wan, S., Qiu, L., Li, Y., Sun, J., Gao, B., He, F., et al. (2020). Accelerated Antimony and Copper Removal by Manganese Oxide Embedded in Biochar with Enlarged Pore Structure. Chem. Eng. J. 402, 126021. doi:10.1016/j.cej.2020.126021
Wang, W., Zhou, J., Wei, D., Wan, H., Zheng, S., Xu, Z., et al. (2013). ZrO2-functionalized Magnetic Mesoporous SiO2 as Effective Phosphate Adsorbent. J. Colloid Interface Sci. 407, 442–449. doi:10.1016/j.jcis.2013.06.053
Wang, S., Gao, B., Li, Y., Mosa, A., Zimmerman, A. R., Ma, L. Q., et al. (2015a). Manganese Oxide-Modified Biochars: Preparation, Characterization, and Sorption of Arsenate and Lead. Bioresour. Technol. 181, 13–17. doi:10.1016/j.biortech.2015.01.044
Wang, W., Zhang, H., Zhang, L., Wan, H., Zheng, S., and Xu, Z. (2015b). Adsorptive Removal of Phosphate by Magnetic Fe3O4@C@ZrO2. Colloids Surfaces A Physicochem. Eng. Aspects 469, 100–106. doi:10.1016/j.colsurfa.2015.01.002
Wang, B., Yu, J., Liao, H., Zhu, W., Ding, P., and Zhou, J. (2020). Adsorption of Lead(II) from Aqueous Solution with High Efficiency by Hydrothermal Biochar Derived from Honey. Int. J. Environ. Res. Public Health 17, 3441. doi:10.3390/ijerph17103441
Xia, W., and Liu, Y. (2021). Preparation of MnO2 Modified Magnetic Graphitic Carbon Nitride Composite and its Adsorption toward Pb(II) in Waste Water. Water Pract. Technol. 16, 1498–1509. doi:10.2166/wpt.2021.059
Xu, W., Song, Y., Dai, K., Sun, S., Liu, G., and Yao, J. (2018). Novel Ternary Nanohybrids of Tetraethylenepentamine and Graphene Oxide Decorated with MnFe2O4 Magnetic Nanoparticles for the Adsorption of Pb(II). J. Hazard. Mater. 358, 337–345. doi:10.1016/j.jhazmat.2018.06.071
Yadav, P., Farnood, R., and Kumar, V. (2021). HMO-incorporated Electrospun Nanofiber Recyclable Membranes: Characterization and Adsorptive Performance for Pb(II) and As(V). J. Environ. Chem. Eng. 9, 106507. doi:10.1016/j.jece.2021.106507
Yang, Y., Wang, J., Qian, X., Shan, Y., and Zhang, H. (2018). Aminopropyl-functionalized Mesoporous Carbon (APTMS-CMK-3) as Effective Phosphate Adsorbent. Appl. Surf. Sci. 427, 206–214. doi:10.1016/j.apsusc.2017.08.213
Yin, H., Liu, F., Feng, X., Liu, M., Tan, W., and Qiu, G. (2011). Co2+-exchange Mechanism of Birnessite and its Application for the Removal of Pb2+ and As(III). J. Hazard. Mater. 196, 318–326. doi:10.1016/j.jhazmat.2011.09.027
Zhang, L., Yang, X., and Wu, Y. (2015). Synthesis of Fe3O4@SiO2@MnO2 Composite Magnetic Submicrospheres as Adsorbent for Methyl Orange Decolouration. Micro & Nano Lett. 10, 12–15. doi:10.1049/mnl.2014.0555
Zhang, H., Gu, L., Zhang, L., Zheng, S., Wan, H., Sun, J., et al. (2017a). Removal of Aqueous Pb(II) by Adsorption on Al2O3-Pillared Layered MnO2. Appl. Surf. Sci. 406, 330–338. doi:10.1016/j.apsusc.2017.02.011
Zhang, H., Wu, A., Fu, H., Zhang, L., Liu, H., Zheng, S., et al. (2017b). Efficient Removal of Pb(II) Ions Using Manganese Oxides: the Role of Crystal Structure. RSC Adv. 7, 41228–41240. doi:10.1039/C7RA05955H
Zhang, H., Xu, F., Xue, J., Chen, S., Wang, J., and Yang, Y. (2020a). Enhanced Removal of Heavy Metal Ions from Aqueous Solution Using Manganese Dioxide-Loaded Biochar: Behaviour and Mechanism. Sci. Rep. 10, 6067. doi:10.1038/s41598-020-63000-z
Zhang, H., Yang, Y., Yuan, L., Liu, G., Shan, Y., Qian, X., et al. (2020b). Improving the Lead Adsorption Performance of Mesoporous MnO2 by Plasma Surface Modification. Dwt 189, 283–295. doi:10.5004/dwt.2020.25607
Zhu, J., Baig, S. A., Sheng, T., Lou, Z., Wang, Z., and Xu, X. (2015). Fe3O4 and MnO2 Assembled on Honeycomb Briquette Cinders (HBC) for Arsenic Removal from Aqueous Solutions. J. Hazard. Mater. 286, 220–228. doi:10.1016/j.jhazmat.2015.01.004
Keywords: lead removal, adsorption, Fe3O4@SiO2, MnO2 grafting, water treatment
Citation: Zhang H, Chen S, Shan Y, Qian X, Yang Y and Wang J (2022) Highly Effective Lead Ion Adsorption by Manganese-Dioxide-Supported Core-Shell Structured Magnetite. Front. Environ. Sci. 10:925205. doi: 10.3389/fenvs.2022.925205
Received: 21 April 2022; Accepted: 12 May 2022;
Published: 25 May 2022.
Edited by:
Shihai Deng, Xi’an Jiaotong University, ChinaCopyright © 2022 Zhang, Chen, Shan, Qian, Yang and Wang. This is an open-access article distributed under the terms of the Creative Commons Attribution License (CC BY). The use, distribution or reproduction in other forums is permitted, provided the original author(s) and the copyright owner(s) are credited and that the original publication in this journal is cited, in accordance with accepted academic practice. No use, distribution or reproduction is permitted which does not comply with these terms.
*Correspondence: Juanjuan Wang, d2FuZ2p1YW5qdWFuQHl6dS5lZHUuY24=