- 1Institute of Environmental Science and Engineering, School of Metallurgy and Environment, Central South University, Changsha, China
- 2Chinese National Engineering Research Center for Control and Treatment of Heavy Metal Pollution, Changsha, China
Excessive levels of heavy metal(loid)s (HMs) in natural environments pose a serious threat to living beings worldwide. HM exposure causes irreversible damage to structural components and metabolic processes in living organisms, as has been observed in multiple studies on various organisms. In the natural environment, biological individuals interact with others through the food web rather than exist independently, which facilitates the transfer of HMs in the food web. However, the difference in HM toxicity among different biological species has not been elucidated. This review provides information on the speciation and migration of HMs in different environments to clarify the HM exposure routes of different biological species. The differences in the biotoxicity of HMs to different species, from microbes to humans, are emphasized. The relationship between HM toxicity and biological species is confirmed by the fact that HMs can be transferred and bioaccumulated along the food chain. Effective strategies for decreasing HMs emissions and removing HMs from the environment are briefly discussed. Finally, the limitations of the present study and future perspectives are discussed.
1 Introduction
Heavy metal(loid) (HM) pollution resulting from anthropogenic activities, such as smelting, electroplating, mining, and sewage irrigation, is a severe environmental issue worldwide (Zhang et al., 2018; Chatterjee and Abraham, 2019; Turner, 2019; Zhao et al., 2019; Yao et al., 2020). Excessive HMs in natural environments pose a threat to ecological security and health (Gress et al., 2015; Hu et al., 2016; Alvarez-Ortega et al., 2019; Giani et al., 2021). HMs such as arsenic, cadmium, chromium, lead, mercury, and zinc have been designated as priority pollutants by the United States Environmental Protection Agency (Chowdhury et al., 2016). Long-term exposure of HMs may lead to serious damage to cells and functional disorders in organisms. Therefore, the biotoxicity of HMs has attracted widespread attention.
HMs are not biodegradable, but remain in the environment for decades, even after the removal of their point sources (Gall et al., 2015), causing incalculable biological damage (Zhao et al., 2019; Zhang et al., 2019; Hausladen et al., 2018; M et al., 2012; Itabashi et al., 2019). The interactions between HMs and organisms extra- and intracellularly induce adverse effects. The chemical groups on the cell surface can bind extracellular HMs, which interfere with the cellular uptake of nutrients (Murthy et al., 2014). The interaction between HMs and the outer membrane usually causes structural damage to cells (Cao et al., 2019). In addition, severe oxidative damage may be induced by HMs when they enter cells through non-specific chemiosmotic metal uptake systems or certain transporters (Thatoi et al., 2014; Bienert and Tamas, 2018). Intracellular HMs can replace metal cofactors in the active centers of enzymes, resulting in denaturation and inactivation of these enzymes (Balistrieri et al., 2018). DNA damage may be induced by intracellular HMs by decreasing the DNA content and destroying the DNA structure (Nicolaus et al., 2016). Numerous studies have demonstrated that HMs cause serious biological toxicity through the above interaction mechanisms (Gjorgieva Ackova, 2018; Kushwaha et al., 2018). Some studies have suggested that these interactions may be affected by various environmental factors, leading to changes in HM toxicity. However, this issue has not been thoroughly discussed.
Previous studies have shown HM toxicity is partly affected by abiotic and biotic factors. Thorough studies have been conducted on the effects of abiotic factors (e.g., Eh, pH, surface charge, minerals, and organic matter content) on HM toxicity. The change of Eh and pH may interfere with the speciation of HMs (Yamaguchi et al., 2011); Minerals, such as clay minerals, can absorb HMs on their surface (Gu et al., 2019); Dissolved organic matters regulate the bioavailability of HMs (Li et al., 2021). Thus, these abiotic factors can reduce the mobility of HMs in the natural environment through adsorption, complexation, or co-precipitation to decrease the toxicity of HMs (Wang et al., 2018), and disturb the speciation of HMs to change their toxicity (Zhao et al., 2017). Biotic factors also play key roles in HM toxicity. Previous studies have focused on the toxicity of HMs to one or more classes of organisms (Voica et al., 2016; Li et al., 2019; Riyazuddin et al., 2021; Lai et al., 2022). For archaea and bacteria, HM exposure induces the adaptive expression of genes that regulate the microbial community structure and functional diversity (Stevenson et al., 2017; Xu et al., 2019). For fungi, morphological changes in mycelia are triggered by HMs, and spore formation and germination are sensitive to HMs, certain fungi can accumulate high levels of HMs in cells (Viti et al., 2014; Kapahi and Sachdeva, 2017). Plants absorb organic and inorganic HMs from the environmental media via their roots and leaves, which alter cellular ionic homeostasis and disrupt photosynthesis (Dubey et al., 2018; Di et al., 2019). For animals, including humans, exposure to HMs damages basic body organs, such as the kidney and liver, and causes serious diseases such as cancer (Rehman et al., 2021). However, these studies did not explore the toxicity of HMs in multiple biological species. Moreover, organisms interact with others through the food web in different environmental media (air, water, and soil), which accurately reflects the toxicity of HMs in the natural environment.
This review briefly explores the speciation and migration of HMs in different environmental media, and discusses up-to-date discoveries of the biological effects of HMs on different organisms, including archaea and bacteria, fungi, plants, animals, and humans, to provide a comprehensive understanding of HMs threats to the environment and health. HMs are transferred and bioaccumulated in the food chain in natural terrestrial or aqueous ecosystems, which puts predators, including humans, at risk of exposure to high concentrations of HMs. We also proposed efficient strategies for the prevention and control of HMs.
2 Heavy Metal(loid)s in Different Environmental Media
HMs enter the environment (air, water, and soil) via the deposition of atmospheric particulates, the release of metal-enriched sewage sludge and effluents, and the disposal of by-products and residues from metal mining processes (Popoola et al., 2017) (Figure 1). The source and settled environmental media of HMs determine their chemical speciation which play decisive roles in their migration and toxicity (Lee et al., 2016) (Figure 1). Atmospheric HMs flow into the soil and water through dry and wet deposition, while some of the HMs in the soil and water return into the atmosphere through evaporation. HMs circulate in different media via deposition, surface runoff, and evaporation.
2.1 Atmosphere
HMs in the atmosphere result from both natural processes (foliage emissions, volcanic activity, and water evaporation) and anthropogenic processes (primarily industrial and automotive emissions) (Table 1). Atmospheric HMs exist mostly in inorganic forms, including elemental-gaseous, oxidized-gaseous, and particulate-bound states (Lee et al., 2016). For example, gaseous elemental mercury (Hg0) and gaseous oxidized mercury (Hg2+) are often referred to as total gaseous mercury (Lee et al., 2016). Hg2+ can be secondarily formed through the oxidation of Hg0 following the formation of particulate-bound mercury. Organic species of HMs in the air are considered to be of negligible importance, except in areas of pesticide application or biotic activity (Mandal and Suzuki, 2002).
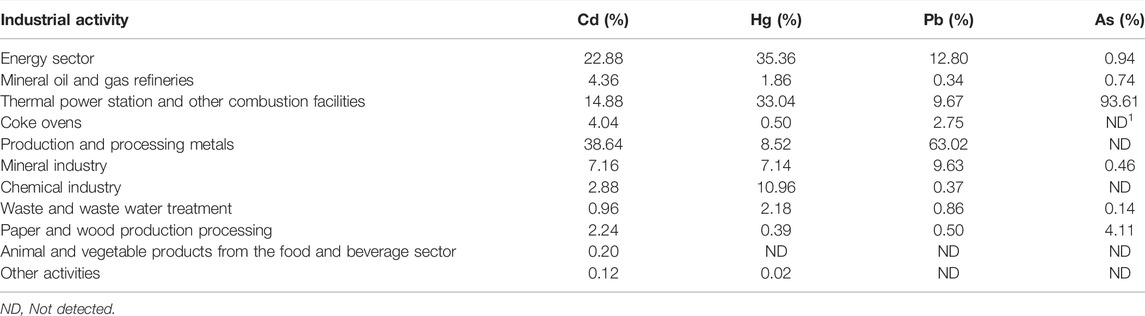
TABLE 1. HM emission from different sources into the air (Simeonov et al., 2011; Shahid et al., 2017b).
Air dispersion is an important route for the global migration of HMs. The predominance of inorganic forms of atmospheric HMs results in their high mobility. The spatial distribution of HMs in the atmosphere largely depends on the impact of current and past industrial activities as well as traffic movements in the surrounding area (Skrbic et al., 2018). In the Pearl River Delta, Guangzhou, China, a high concentration of As has been identified and attributed to the proximity to dense industrial HM point sources (Ye et al., 2021). Moreover, the content of HMs in the atmosphere varies greatly with distance from emission sources. Airborne As concentrations could exceed 1 μg/m3 near emission sources (Pringle and Jervis, 1987), ranging from 10 to 200 ng/m3 in urban areas, and lower than 10 ng/m3 in rural areas (Shahid et al., 2017b). In addition, HMs in the atmosphere can be transported over long distances owing to their relatively inert nature, low water solubility, and low deposition velocity. The levels of atmospheric HMs in European countries have increased over the last several decades (Uzu et al., 2009; Schreck et al., 2011), which might be attributed to the long-distance transport of HMs in the atmosphere. Therefore, high mobility is the most important risk posed by atmospheric HMs, leading to the continuous expansion and dispersal of pollution. Additionally, HMs are easily adsorbed onto atmospheric particles and easily dissolved in rainwater, resulting in HM enrichment and contamination of the surface environment through both dry and wet deposition. Combating atmospheric deposition should be considered an important goal for regional HM pollution management.
Owing to the invisible presence of atmospheric HMs in land surface air, they pose a great threat to the health of plants, animals, and people. HMs in ambient air can accumulate in plant leaves through foliar transfer after the deposition of atmospheric particles on leaf surfaces (Shahid et al., 2017b). Plants growing near smelters showed high foliar levels of HMs, which resulted in HMs flowing from the polluted air into the food webs, illustrating that crops in the area provide a potential pathway directly to humans, thereby threatening human health (Uhlig and Junttila, 2001). In addition, HMs in the air can easily enter the human body through ingestion, inhalation, and dermal contact (Rout et al., 2012; Cao et al., 2015). Hg0 enters the human body through the respiratory tract and accumulates in the liver, kidneys, and brain (Clarkson and Magos, 2006), resulting in serious organ damage. Compared to other exposure routes, indoor dust which acts as a carrier of inorganic and organic pollutants including HMs (Tan et al., 2016), poses the greatest threat to humans, especially children. It was reported that the level of Pb reached 639.10 μg/g in indoor dust at the location which was exposed to high traffic density and lead fuel usage (Tan et al., 2016). Some researchers have reported that the concentrations of Pb, Ni, Cd, Co, Cu, and Cr in indoor dust increase when the size of dust particles decreases (Hassan, 2012). Smaller dust particles have a larger total surface area that comes into contact with HMs in the environment, resulting in higher levels of HMs in smaller dust particles (Almeida et al., 2011).
2.2 Water and Sediments
The routes through which HMs enter an aqueous environment are diverse (Reza and Singh, 2010) (Table 2). The natural geological weathering of rocks and soil, which is directly exposed to surface waters, is usually the largest natural source (Karbassi et al., 2007). Some diffusion pathways, including surface runoff and atmospheric deposition, are also important natural sources of HMs in aqueous environments (Zahra et al., 2014). Anthropogenic sources mainly include mining, disposal of untreated and partially treated effluents, metal chelates from different industries, indiscriminate use of fertilizer, and improper waste disposal such as liquid leaching from smelting waste (Nouri et al., 2008; Malik et al., 2010). HMs discharged into aquatic systems are distributed in both the aqueous phase and sediments. Because of adsorption, hydrolysis, and co-precipitation, only a small portion of free metal ions remain in the water, while a large portion are deposited in sediments (Varol and Şen, 2012). HMs settled in sediments may be resuspended and cause secondary contamination of the aqueous phase. Therefore, the assessment of HM pollution in aquatic environments should emphasize sediment analysis (Hou et al., 2013).
HMs generally exist in a stable, single ionic state in the aqueous phase. HMs are present in various chemical forms in sediments, exhibiting different behaviors in terms of mobility, biological availability, and potential toxicity (Singh et al., 2005; He et al., 2011; Qiao et al., 2013). The diverse chemical forms in sediments are mainly attributed to the interaction between HMs and complex sediment matrices, including carbonates, Fe/Mn oxides, organic matters, sulfides, and silicates (Wang et al., 2010; Hou et al., 2013). The nature of the sediment particles and physicochemical conditions play a critical role in the geochemical fractionation and mobility of HMs (Gao and Li, 2012). In addition, biological processes change the chemical forms of HMs in sediments, resulting in their release from sediments or conversion from inorganic to organic materials, through processes such as methylation (Zhao et al., 2017). Changes in the chemical forms of HMs, mediated by sediment particles and organisms, influence their potential toxicity and threat to ecosystems (Li et al., 2007). The methylation of HMs could greatly increase their biotoxicity, as methyl-HMs would be biomagnified within aquatic food webs.
Water and sediments provide sites for biogeochemical cycling and are the base level of the food web. Thus, the aquatic environment is a key channel for biota to be exposed to HMs. Aqueous environments contaminated with HMs, particularly drinking water, pose a threat to human health. Plants are exposed to HMs in water by irrigation with HM-polluted water and root uptake of HMs from groundwater. Owing to the scarcity of freshwater sources, agricultural soils in many areas of the world are irrigated with untreated wastewater (Mustapha and Adeboye, 2014). Although wastewater contained low levels of HMs, the plants had higher values of metals due to accumulation, which threatens plant growth and crop yield. Excessive uptake of HMs in plants interferes with physiological processes, such as gaseous exchange, CO2 fixation, respiration, and nutrient absorption (Ahmed and Slima, 2018). Of these HMs, Cd is of particular concern in plants because of the high levels of Cd accumulation in leaves, which may be consumed by animals or humans (Nagajyoti et al., 2010). Additionally, HMs in the aqueous environment also accumulate in aquatic animals, such as fish and clams, some of which have been acknowledged as an integral component of a well-balanced diet. Therefore, diet is also the main route of HM exposure in the general population.
2.3 Soils
Both natural and anthropogenic activities can lead to excessive HM concentrations in the soil (Table 3). Natural weathering and erosion of crustal materials or deposition of HMs emitted into the atmosphere by volcanic activities, account for 80% of natural sources of soil HMs (Hou et al., 2017). Forest fires and biogenic sources also contribute to soil HMs (Bao et al., 2016). Natural HMs in soils exist as diverse mineral forms. However, soil HMs mainly stem from anthropogenic activities, such as traffic emissions (Eichler et al., 2015), domestic emissions, the weathering of building and pavement surfaces, sewage irrigation, fertilizer and pesticide application (Yang et al., 2013), mining, smelting, and other industrial activities (Li et al., 2015), and waste dumping and disposal (Ren et al., 2018). Compared with HMs in the atmosphere and water, soil HMs are more harmful owing to their concealment, stagnancy, and accumulation properties. Soil usually acts as both a sink and a source of a variety of pollutants and therefore has a significant influence on living organisms (Khan et al., 2010). When accumulated, HMs pose a serious threat to soil ecosystems, leading to soil degradation and a reduction in agricultural production. In addition, due to surface runoff and leaching HMs migrate underground and pollute groundwater (Guo et al., 2013). Therefore, HMs are widespread in soil system (Tahar and Keltoum, 2011).
The concentrations of soil HMs are dynamic and change with a variety of natural and anthropogenic activities, as well as the interactions of HMs with the soil matrix and biota (Xia et al., 2014). These interactions cause the absorption, leaching, and redox of HMs in the soil. Clay minerals, an important soil constituent, can absorb HMs and decrease their mobility, resulting in HM accumulation in soils (Zhang et al., 2012). These absorption and leaching processes can be affected by the soil pH, ionic strength, and the presence of competing metals (Zhang et al., 2012). The redox of HMs usually occurs in soils, especially for HMs with variable oxidation states (such as Cr). This mainly depends on soil Eh, pH, surface charge, and organic matter content (Choppala et al., 2018). Meteorology and topography may also affect the concentrations of HMs in soils and ecosystems (Shen et al., 2017). Apart from the HM concentration, the mobility and bioavailability of HMs determine the biological toxicity of HMs in soils. The mobility and bioavailability of HM fractions evaluated using sequential extraction techniques depend on their binding forms. The exchangeable fraction can be adsorbed by organisms that are enriched in the food chain. Redox-active carbonate fractions are present and are easily converted by the soil matrix or organisms to other forms. Exchangeable and carbonate fractions are the primary contributors to the bioavailability of HMs in soils (Li et al., 2015). HMs in the residual fraction are trapped within the crystalline structure of minerals and are the least mobile fraction (Zhang et al., 2017), therefore the smallest threat to biota. The addition of soil amendments, such as biochar and kaolin (Wang et al., 2018), can reduce the bioavailability of the HMs in soil by increasing the pH, adsorption, complexation, or co-precipitation. Therefore, a comprehensive understanding of the biogeochemical behavior of HMs in natural soils is crucial for assessing their environmental risks.
Soil is an important source of human HM exposure via direct (inhalation, ingestion, and dermal contact) and indirect pathways (HM-enriched crop ingestion). HMs can be strongly adsorbed by soil particles as well as plant roots, which poses a serious health risk if humans or livestock ingest polluted soils or soil particles adsorbed on the surface of leafy vegetables and root crops (Wuana and Okieimen, 2011). In addition, elevated concentrations of HMs in soils have been shown to decrease soil microbial biomass, diversity, and activities (Abdu et al., 2016). Microorganisms are regarded as the most sensitive to HM stress among all soil organisms. A few microorganisms utilize HMs as electron donors or acceptors during metabolic processes, but the survival of most microbes is threatened by HM exposure. HMs adversely affect the growth, morphology, and biochemical activities of microbial populations, ultimately resulting in decreased bacterial biomass and diversity (Chen et al., 2013). Intracellular HMs can damage cellular membranes, alter enzyme specificity, disrupt cellular functions, and damage DNA structures (Macomber and Hausinger, 2011).
3 Toxicity of Heavy Metal(loid)s to Different Organisms
HMs can be classified as essential metals required for biological physiology and function and non-essential metal (loid)s with unknown biological functions (Osman et al., 2019). Although essential metals are crucial for the structure of cell membranes, proteins, and DNA, their excess is lethal to cells (Lemire et al., 2013). Some non-essential metal(loid)s, such as Hg, Pb, and As, are extremely toxic to most organisms even at exceptionally low concentrations (Kalaivanan and Ganeshamurthy, 2016; Guo et al., 2017). Organisms have significant differences in their structure and function, resulting in diverse and distinct responses to HMs. Understanding the perturbing processes of HMs in different biological systems is crucial to facilitate the assessment of the toxicity of HMs to different prokaryotes and eukaryotes. Here, we explored the toxic effects of HMs on prokaryotes (archaea and bacteria) and eukaryotes (fungi, plants, animals, and humans). We found that the toxicity symptoms of HMs to different organisms were quite different (Table 4) and further discussed the toxic mechanisms involved.
3.1 Archaea and Bacteria
Archaea and bacteria thrive in various environments, such as soil, sediments, and wetlands, which provide excellent settings for microbe-HMs interactions. They play critical roles in the modification, activation, and distribution of HMs in the environment (Sterritt and Lester, 1980; Polizzotto et al., 2008). However, excessive levels of HMs in the environment threaten the survival of prokaryotes (Table 4). Studies of HM toxicity have been performed on single bacterial strains (Huang et al., 2019b), microbial communities (Xu et al., 2019), and microcosms (Ward et al., 2019). The underlying toxicity mechanisms of growth inhibition and functional weakening can be summarized as follows: membrane disruption, oxidative damage, protein inactivation, and genotoxicity (Figure 2).
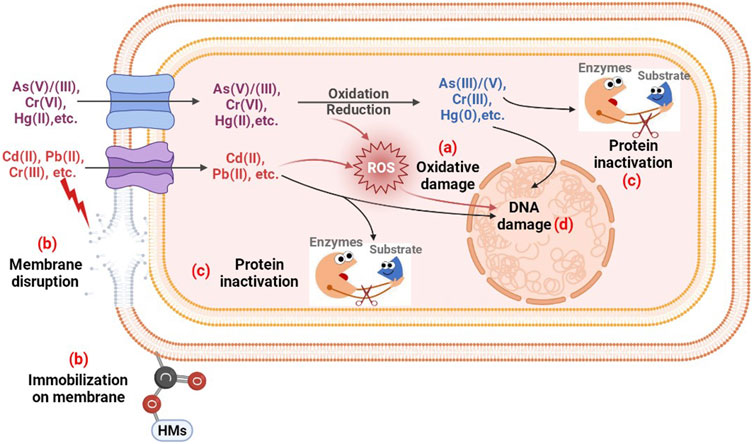
FIGURE 2. The possible toxic mechanism of HMs to prokaryotes. (A) Oxidative damage resulting from excessive ROS; (B) Structural disruption and metal ion immobilization on membranes; (C) Protein regulation; (D) DNA damage caused by oxidative stress and metal ions.
3.1.1 Oxidative Damage
The entry of HMs into microbial cells causes a series of oxidative stresses due to the over-production of reactive oxygen species (ROS) (Figure 2A). Owing to the presence of unpaired valence shell electrons, ROS are chemically highly reactive molecules and generally short-lived and unstable. This is an unavoidable consequence of aerobic metabolism (Malaviya and Singh, 2016). Certain HMs, including Cr, As, Sb, and Hg, undergo redox reactions in the presence of oxygen and induce the production of ROS directly via the Fenton-like or Haber-Weiss reactions. Other HMs without redox activity, such as Cd, Pb, Ni, and Zn, indirectly induce ROS production through their interactions with sulfur (Iannone et al., 2010). These metal cations show a high affinity for sulfur, and their interactions inhibit the activity of some enzymes depending on the thiol or cysteine groups, which facilitates the production of ROS (Steunou et al., 2020). The ROS induced by HMs include hydrogen peroxide (H2O2), superoxide anions (•O2−), singlet oxygen (½O2), hydroxyl radicals (•OH), alkoxyl (RO•), peroxyl (RO2•), organic hydroperoxide (ROOH), and fatty acid hydroperoxide (Garnier et al., 2006). Excessive ROS can lead to oxidative stress, which damages biomolecules, such as DNA, proteins, and lipids (Hu et al., 2020). Accordingly, a few antioxidant enzymes are stimulated by HMs to scavenge ROS and protect cell components. Superoxide dismutase (SOD) catalyzes the dismutation of •O2− to H2O2. Subsequently, catalase catalyzes the reduction of H2O2 to water (Yao et al., 2018). Ni2+ exposure of Burkholderia vietnamiensis and Pseudomonas putida multiplied ROS and increased the number of oxidative stress-associated defense proteins, including SOD, thioredoxin, and ferredoxin NADP reductase, as well as a thiol-specific antioxidant (Macomber and Hausinger, 2011).
3.1.2 Membrane Dysfunction
HM exposure directly destroys the integrity of cell walls and membranes. Archaea and bacteria actively alter the composition of their cell walls and membranes to protect essential components of the cells by preventing HMs from crossing cell membranes (Figure 2B). The cell wall is the first component of microbial cells that encounters metal ions. Exposure to Cr(VI) and Cu(II) resulted in extensive morphological abnormalities in Bacillus sp. S3, with the appearance of irregular cell walls and the separation of cell membranes from the cell walls (Zeng et al., 2020). Partial metals accumulate in different chemical groups on the cell membrane, such as carboxyl and phosphate groups. In P. putida CZ1, Cu2+ stress induced the synthesis of carboxyl-rich acidic polysaccharides in extracellular polymeric substances and phosphate-rich phospholipids in cell walls and cell membranes, both of which are involved in the extracellular binding of Cu (Lin et al., 2020). Carboxyl is the main functional group of Cu adsorbed by P. putida (Chen et al., 2007). Therefore, the outer membrane of cells plays a decisive role in determining the amount of metal that penetrates the cytoplasm. The transmembrane of HMs through unspecific chemiosmotic metal uptake systems usually causes structural inconsistencies in the cytoplasmic membrane (An et al., 2019). Pb entry causes cytoplasmic membrane disruption and changes the mesosomal structure (Sterritt and Lester, 1980).
3.1.3 Protein Regulation
HMs that penetrate the cell membrane can react with intracellular components and replace the metal constituents in the active centers of enzymes (Figure 2C), especially metalloproteins (Yuan et al., 2020), resulting in the denaturation and inactivation of enzymes (Zhang et al., 2016a). Mercuric reductase, MerA, from Azotobacter chroococcum contains four cysteine residues that act as a class of nickel binding sites. 100 μM Ni2+ exposure caused a 62% loss of MerA activity (Ghosh et al., 2001). In addition, HMs can bind to either the catalytic residues of non-metal enzymes to block catalysis, or the catalytic sites of enzymes to influence activity allosterically. HMs-induced oxidative stress can also affect protein function to some extent (Macomber and Hausinger, 2011). Mostly, the intracellular overloaded oxidative stress induced by HMs leads to the decrease of enzyme activities, or even inactivation (Voica et al., 2016). Not all protein activities are reduced under HM stress, the presence of HMs may stimulate the production of some enzymes to resist and detoxify HMs. The arising oxidative stress can induce the overexpression of antioxidant enzymes like glutathione transferase, SOD, and catalase to scavenge ROS (Thatoi et al., 2014). Metallothioneins show a strong affinity for metals such as Ag, Zn, Cd, Cu, and Hg. The presence of such HMs induced the intracellular synthesis and secretion of metallothionein-like proteins to immobilize HMs, which have been found in Synechococcus spp., E. coli, and P. putida (Gupta and Joia, 2016; Osman et al., 2019). For microbial survival, some oxidase or reductases for HM biotransformation, such as chromate reductase (Thatoi et al., 2014), arsenite oxidase (Yan et al., 2019), and arsenate reductase (Hu et al., 2021), may be induced to increase their activities by HMs to detoxify HMs.
3.1.4 Genotoxicity
DNA damage is a hallmark of oxidative stress (Figure 2D). HMs affect genetic material in various ways, including decreasing DNA content and damaging DNA structure (e.g., modified bases, single- and double-strand breaks, DNA-protein crosslinks, 8-hydroxyguanine, DNA demethylation and base oxidation, and sister chromatid exchange) (Shahid et al., 2017a). For example, Cd can be transported across cellular or nuclear membranes and disrupt DNA repair and replication by binding directly or indirectly to DNA or proteins (Liu et al., 2012). Cd exposure also causes ladder-like DNA degradation (Andosch et al., 2012). HMs indirectly cause DNA damage or oxidation via ROS production. The •OH radical is highly electrophilic and can oxidize DNA by reacting with the pyrimidine aromatic rings (Nzengue et al., 2011). The RO2• radicals are distributed to the hydroperoxides, which induces the protonation reaction (Shahid et al., 2017a). The purine bases of DNA are more sensitive to HM-induced ROS, particularly those with a lower oxidation potential. HMs also interfere with RNA metabolism, trigger programmed cell death, cause chromatin fragmentation, and affect genetic functioning by affecting protein structure (Behboodi and Samadi, 2004).
3.2 Fungi
Soil, water, and rich sediments are ideal for fungi growth and reproduction. However, these sites are also sinks for HMs released into the environment. Both essential and non-essential HMs in excess threaten the survival of fungi including yeasts and filamentous fungi (Miller, 1960). HMs in the natural environment may directly interact with active groups and extracellular enzymes of fungi. The HMs taken up by the fungi cause a series of physiological responses (Baldrian, 2003), which include the decline of growth and metabolic activity, morphological changes of the growing mycelium, inhibition of enzyme activity, and adverse effects on reproduction (Table 4).
3.2.1 Growth
After HMs enter the fungal cell, they affect both single reactions and complex metabolic processes. Growth inhibition is the most common and significant phenomenon associated with HM exposure. Even 0.1–0.2 mM of Cd led to severe growth inhibition of the white-rot fungus Schizophyllum commune (Lilly et al., 1992). Hg exposure decreased the growth rate of Phanerochaete chrysosporium and the protein content of the mycelia (Dhawale et al., 1996). As we know, the essential metals are relatively less toxic. The growth of P. chrysosporium was significantly limited in the presence of only 50 mg/L of Ni, Cd, and Pb, whereas the growth decrease was barely noticeable when exposed to 150 mg/L of Co and Cu, and 300 mg/L of Mn (Falih, 1998). Such a view does not apply to all fungal species, and sometimes essential metals may be more toxic than non-essential metals to certain species. In Ganoderma lucidum, the toxicity of HMs decreases in the order Hg > Cd > Cu > U > Pb > Mn = Zn (Tham et al., 1999). Similar to prokaryotes, the decrease in fungal biomass is sometimes accompanied by a prolonged lag phase, which was previously recorded in a culture of Pycnoporus cinnabarinus exposed to Hg (Mandal et al., 1998). The presence of HMs also interferes with fungal soil colonization. A significant decrease was observed in the growth of Agrocybe perfecta on a straw when exposed to Cd (Baldrian, 2003).
3.2.2 Mycelial Morphology
The decrease in fungal growth rate caused by HMs is often accompanied by morphological changes in the growing mycelium. S. commune exposure to increasing concentrations of HMs (Cd) led to an increase in aerial hyphae formation, morphological changes in hyphae, the development of loops and connective filaments, and an increase in hyphal branching (Lilly et al., 1992). The fungus Daedalea quercina cultivated in liquid media with Cd exhibited shortening and irregular appearance of surface hyphae (Gabriel et al., 1996b). Morphological changes induced by HMs are common to all groups of fungi. The addition of Cd to the culture of the ectomycorrhizal fungus Paxillus involutus increased the number of laterals per branch point but decreased the distance between branch points, increasing hyphal density (Darlington and Rauser, 1988). Fungal cultures grown in the presence of HMs can also change their discoloration. When cultivated in the presence of Cd, S. hirsutum produced a yellow-orange pigment both extracellularly and in the mycelium, Trametes versicolor produced a brown pigment (Mandal et al., 1998). When S. commune was cultivated in liquid media containing Pb, black mycelial pellets were formed, while the controls were creamy (Gabriel et al., 1994). In addition, some studies on Aspergillus have revealed the importance of mycelial morphology in HMs tolerance. The cell surface properties of Aspergillus versicolor change along with the morphology of the fungal mycelia when exposed to Cr(VI) (Das et al., 2008). The cell wall components of the mycelia possess major binding sites for Cr(VI), and thus prevent Cr(VI) transport into the cytoplasm and prevent contact between Cr(VI) and intracellular organelles.
3.2.3 Enzymatic Activities
Similar to prokaryotes, HMs are generally potent inhibitors of enzymatic reactions in fungi. Hg exerts its toxic effect mainly by binding to the -SH groups present in the active or regulatory sites of enzymes and causing their irreversible inactivation. Cu and Cd can bind to aromatic amino acid residues in the enzyme molecules, resulting in a loss of enzymatic activity. HMs also cause oxidative damage to proteins by inducing oxidative stress associated with the production of ROS such as •OH or •O2− (Stohs and Bagchi, 1995). Meanwhile, intracellular antioxidant enzymes like SOD are typically induced by HMs. In the ectomycorrhizal fungus P. involutus, Cd activates SOD, which is responsible for Cd resistance by decomposing •O2− (Jacob et al., 2001). For the lignin-degrader, white-rot fungi, most attention has been paid to metal toxicity toward extracellular enzymes (Baldrian, 2003). The extracellular enzymes produced often face independently high concentrations of HMs, and their enzymatic activities may be lost during HM exposure since they are not protected by cell-associated metal-detoxication mechanisms. In addition, HMs can get onto cells through transporters, which influence the production of enzymes at the levels of transcriptional and translational regulation.
In addition, low concentrations of essential HMs are necessary for the development of ligninolytic enzyme systems. The addition of low concentrations of Zn and Cu to the metal-free synthetic cultivation medium increased the activity of lignin peroxidase and Mn-peroxidase in P. chrysosporium, promoting the solubilization and mineralization of lignin (Singhal and Rathore, 2001). Some essential HMs, such as Mn and Cu, are directly involved in reactions catalyzed by ligninolytic enzymes. Because Mn is directly involved in the catalytic cycle of Mn-dependent peroxidase (MnP), it plays an important role in lignin degradation. Mn has been reported to play a regulatory role in the expression of MnP and the laccase enzyme during lignin degradation (Baldrian, 2003). Cu, a cofactor of the laccase enzyme, is involved in fungal lignin degradation and is present in the catalytic centers of various enzymes. Recent studies have demonstrated that Cu plays an important regulatory role in laccase production as observed in various fungal species such as Ceriporiopsis subvermispora, T. versicolor, P. ostreatus, etc. The transcription levels of laccase genes in T. versicolor increased within 15 min of Cu addition (Collins and Dobson, 1997). Supplementation with Cu significantly increased the production of all laccase isoenzymes produced by the fungus P. ostreatus (Munoz et al., 1997). In P. chrysosporium and M. quercophilus, the production of extra laccase isoenzymes, which are not present under natural conditions, was observed after Cu addition. Little is known about the effects of HMs on intracellular enzymes in fungi. The activity of ribonuclease from P. tuberregium is inhibited by Hg, Zn, Ni, Ca, and Pb (Wang et al., 1998).
3.2.4 Reproduction
HMs are harmful to the reproduction of fungi, which has been confirmed in different taxonomic groups of fungi. In saprophytic and mycorrhizal fungi, the reproductive stages of development (spore formation and germination) are much more sensitive to HMs than mycelial growth (Baldrian, 2003). In white-rot fungi, fruiting sensitivity differs between species. During a growth experiment on a straw with 0.05–1 mM of Cd, A. perfecta failed to produce fruit bodies, whereas Pleurotus ostreatus was much less sensitive and did succeed in producing fruit bodies (Gabriel et al., 1996a). Favero et al. (1990) found in another strain of P. ostreatus that sporocarp formation remained unaffected by up to 285 mg/L of Cd. Cd preferentially accumulated in the fungal caps at 56 mg/L compared to 36 mg/L when Cd preferentially accumulated in the stems. When straw was overlaid with non-sterile Cd-containing soil, A. perfecta and P. ostreatoroseus failed to produce fruiting bodies at 50 mg/L of Cd, whereas P. ostreatus produced fruit bodies even at 500 mg/L of Cd (Gabriel et al., 1996a). Approximately 15% of the HMs present in the soil were translocated into sporocarps. The overload of Cd, Hg, and Co was found to be toxic for sporocarp development of V. volvacea which is cultivated for food (Purkayastha and Mitra, 1993). The accumulation of HMs in edible fungi flows into the human body via food intake, which leads to unpredictable health risks and necessitates further study.
3.3 Plants
Plants growing in HM-contaminated soils or irrigated with HM-contaminated water show visible symptoms, including dysplasia, chlorosis, and occasionally death. HMs interfere with physiological processes, such as CO2 fixation, respiration, and nutrient absorption, even at low levels (Figure 3). Cr has been reported to affect the biochemistry and physiology of crops adversely (Zeng et al., 2011). Excessive Cd accumulation in plant tissues can result in numerous toxic morphological, physiological, and biochemical effects (Shahid et al., 2017a). Therefore, exposure to HMs may induce toxic effects on several biochemical processes in plants, mainly in plant germination, root growth and length, stem growth, and leaf development.
3.3.1 Seed Germination
HM toxicity during seed germination is one of physiological effects of HMs on plants (Singh et al., 2013). 0.5 mM in Cr(VI) almost completely inhibited seed germination of Phaseolus vulgaris (Sharma et al., 2016), similar to Triticum aestivum at 100 mg/L of Cr(VI) (Dotaniya et al., 2014). Amylase hydrolysis of starch is responsible for supplying sugars to emergent embryos. Cr and Co exposure decreased amylase activity which reduced the amount of sugar available to developing embryos, thereby inhibiting seed germination (Zeid, 2001).
3.3.2 Photosynthesis
HMs affect the photosynthetic apparatus and photosynthesis, leading to a decrease in plant growth and productivity, or even plant death. HMs induce changes in thylakoid arrangement, complete distortion of the chloroplast membrane, and affect both light and dark reactions by inhibiting the Hill reaction (Zeid, 2001). Exposure to Hg2+, Cu2+, and Cd2+ had adverse effects on the freshwater microalga, Chlorella vulgaris, including reduced photochemical quantum yield, decreased chlorophyll fluorescence ratio, minimized fluorescence yield, and non-photochemical quenching (Oh and Chan, 2015). Cr(VI) stress negatively influences photosynthesis in terms of electron transport, CO2 fixation, enzyme activity, and photophosphorylation (Shanker et al., 2005). Cr(VI) exposure induces a decrease in chlorophyll-a, chlorophyll-b, total chlorophyll, and carotenoids in Oryza sativa L. (Sharma et al., 2016). Under HM stress, electrons generated during photochemical processes may not be primarily used for carbon fixation, which leads to a reduced rate of photosynthesis. For example, electron transport can be efficiently blocked by the redox change of Cr(VI) which occurs in the Cu and Fe carriers, or the Cr(VI) binding to the heme group of cytochromes (Dixit et al., 2002). Metals such as Cr(VI) have a high oxidative potential and can reduce photosynthesis by producing ROS as an alternative sink for electrons via oxygen reduction as part of the Mehler reaction (Shahid et al., 2017a; Shahid et al., 2017b). HM-induced ROS production is an important mechanism that interferes with photosynthesis. While the chloroplast membranes are rich in polyunsaturated fatty acids which are a target of ROS-induced peroxidation, ROS induced structural alterations to the pigment-protein complexes, which occurred in three steps: 1) degradation and destabilization of the proteins of the antenna complex; 2) substitution of Mg2+ with H+ ions, resulting in pre-optimization of the chlorophylls; and 3) damage to the thylakoid membranes (Shahid et al., 2017c). Therefore, metal-induced ROS, directly and indirectly, interferes with photosynthetic machinery, resulting in reduced pigment content and plant growth (Shahid et al., 2014). Some studies have reported that Cr(VI) and Cr(III) stress might inhibit pigment biosynthesis by degrading d-aminolacvulinic acid dehydratase (Dey and Mondal, 2016), which is a key enzyme in chlorophyll biosynthesis. In addition, HMs can interfere with different steps of pigment biosynthesis, likely by competing with Fe and Mg for uptake and transport to the leaves, even depleting the chlorophyll content by replacing Mg ions from the active sites (Dey and Mondal, 2016).
3.3.3 Nutrient Uptake
HMs, which are structurally similar to other essential ions, may interfere with plant mineral nutrition in a complex manner. Several previous studies have reported that Cr(VI) and Cr(III) interfere with the uptake of essential nutrients, including Mg, P, K, Mn, Fe, Cu, and Zn in Cocos nucifera; N, P, and K in Oryza sativa; K, Fe, Mn, Mg, Ca, and P in Salsola kali; S, P, Zn, Mn, and Cu in Brassica oleracea; and Fe, Mn, Zn, and Cu by Amaranthus viridis (Shahid et al., 2017c). Therefore, the competitive binding of HMs to common carriers can decrease the uptake of many essential nutrients. Another reason for the HM-induced decrease in nutrient uptake is the decrease in the activity of plasma membrane H+ ATPase (Shanker et al., 2005). Exposure to high levels of HMs may displace essential nutrients from physiological binding sites. The antagonistic interaction between HMs and essential nutrients may be due to their interference both within the soil and inside the plant tissues. Some studies have also reported synergistic interactions between HMs and essential nutrients such as Cu, Mn, Ca, and Mg (Singh et al., 2013).
3.3.4 Plant Biomass
HMs significantly affect root growth and development of plants. The decrease in root growth has been demonstrated in P. vulgaris induced by 0.5 mM of Cr(VI) (Sharma et al., 2016). HMs also affect the number of secondary roots and the development of lateral roots. In Zea mays, compared to Cu and Fe, Cr(VI) treatment reduced root length and number of root hairs, along with causing a brownish appearance, due to a decrease in root cell division (Mallick et al., 2010). Some researchers have even reported an extension of the cell cycle and a decrease in the mitotic index of root tip cells under HM toxicity (Zou et al., 2006; Sundaramoorthy et al., 2010).
Plant stem growth is another parameter that is frequently affected by HM exposure. When Zea mays and P. vulgaris were exposed to 0.5 mM of Cr(VI) and 9 mg/L of Cr(VI), respectively, a decrease in stem growth was observed (Mallick et al., 2010; Sharma et al., 2016). This decrease in stem growth and height can be attributed to HM-reduced root growth and development, resulting in decreased water and nutrient translocation to the aboveground plant parts. Increased HM transport to shoot tissues can directly interact with sensitive plant tissues (e.g., leaves) and processes (e.g., photosynthesis), which affects the cellular metabolism of shoots, and thereby reduce plant height (Shahid et al., 2017a).
Leaf growth parameters are appropriate bioindicators of HM toxicity. HM exposure reduced the growth of leaf, and the leaves were comparatively smaller, wilted, and chlorotic compared to the control sample (Dago et al., 2014). Previous studies have reported that HMs induced leaf chlorosis and necrosis (Ropek and Para, 2003). Continuous and long-term HM application caused the old leaves to become necrotic, permanently wilted, dry, and shed. Some authors have also reported a decrease in leaf area in response to high levels of HM exposure. This can be the result of a decrease in cell division and the number of cells in the leaves due to the involvement of oxidative stress (Gallego et al., 1996). HM toxicity reduces the size and number of leaves in watermelon plants and turns them yellow and wilted due to the loss of turgor hung down from petioles (Shahid et al., 2017a).
High plant biomass is a prerequisite for high plant yields. HMs have been reported to induce noxious effects on several physiological and biochemical processes, which compromises plant yield and productivity equally (Sharma et al., 2016). When exposed to HMs in soil, the plant growth and grain yield of Triticum aestivum L. wheat significantly decreased, and Cd was the most toxic metal, followed by Cu, Ni, Zn, Pb, and Cr (Athar and Ahmad, 2002). HMs induced decreases in plant development, growth, and yield due to several factors, including reduced water and nutrient uptake, decreased cell division and cell division rate, imbalances in nutrient uptake and translocation, the inefficiency of selective inorganic nutrient uptake, enhanced production of reactive radicals and the resulting oxidative stress, substitution of essential nutrients from key molecules and ligands, and oxidative damage to sensitive plant tissues (Shanker et al., 2005; Singh et al., 2013). All these factors, separately or in combination, affect plant growth, development, and yield at the cellular, molecular, organ, and plant levels. However, which of these factors is more severely affected depends on the plant type and chemical speciation of HMs (Dago et al., 2014). In addition, the effect of HMs on plant development varies according to plant species. Generally, transgenic and hyperaccumulator plants have increased potential for selective accumulation and tolerance of HMs (Rehman et al., 2015).
3.4 Animals
The toxic effects of HMs have been studied in different animal species, and HM exposure causes a decrease in growth, development, and reproduction in animals, similar to plants. However, the primary consideration should be that HMs can accumulate in food webs (Agah et al., 2009; Wise et al., 2011; Wang et al., 2019; Zhou et al., 2019).
The aquatic environment is the largest sink of HM pollution, and numerous studies have concentrated on the toxicity of HMs to aquatic animals (Ji et al., 2015; Tan et al., 2019; Xu et al., 2021). For example, Cd tends to accumulate in mollusks at higher concentrations than in other major species groups, such as crustaceans and fish (Tan et al., 2019). When ingested by animals and humans, Cd can lead to kidney dysfunction, skeletal damage, and cancer (Satarug and Moore, 2004). According to the World Health Organization, oral ingestion of as little as 0.125 mg Cd/kg body weight may result in health issues (Cui et al., 2019). Additionally, the accumulation of HMs in aquatic animals increases with age. The contents of As, Cd, Cr, Co, Pb, Ni, Sb, and Zn in the muscles and livers of grunt, flathead, greasy grouper, tiger-tooth croaker, and silver pomfret fish species were determined (Agah et al., 2009). Almost all metals were found to accumulate in flathead, greasy grouper, and tiger-tooth fishes, whereas older fishes seemed to accumulate more (Agah et al., 2009). Animal muscle and liver tissues appear to be good bioindicators for identifying environmental exposure to metallic contaminants. Recently, Cr in the marine environment has become a concern, especially for the health of sperm whales, and recent data have shown that some whales have very high Cr skin levels. Particulate and soluble chromate induced concentration-dependent increases in cytotoxicity and genotoxicity in sperm whale skin fibroblasts, and Cr-DNA adducts induced by Cr(VI) caused a stalled replication fork and resulted in chromosomal aberrations (Wise et al., 2011). Cr(III) and Cr(VI) seemed to have no significant effect on the survival rate, hatching rate, or spontaneous movement of zebrafish. However, the malformation rate in the Cr(VI)-exposed group was increased, and both Cr(III) and Cr(VI) exposure exhibited decreasing changes in swimming distance and disturbance of sensitivity to light and darkness (Xu et al., 2021). Cr exposure significantly stimulated ROS generation, resulting in neurotoxicity in zebrafish, which is associated with metabolic disturbances. The clam, regarded as a dominant species, is widespread in estuarine and coastal waters of East Asia, where it is used as feed for crab aquaculture and acts as a potential vector for transferring contaminants to humans (Tan et al., 2019). When clams were exposed to Cd-contaminated waters, Cd tended to accumulate in clams at higher concentrations than in other species, such as crustaceans and fishes (Tan et al., 2019). With an increase in salinity in estuarine waters, Cd bioaccumulation in clams decreased, and thus Cd toxicity decreased monotonically (Tan et al., 2019).
HMs are also widely distributed in soil, resulting in bioaccumulation in the soil biota, which may ultimately affect human health (Yang et al., 2018). Earthworms are important environmental indicators of soil, and their activity may influence the species, mobility, and partitioning of HMs in soil (Sizmur et al., 2011). However, the adverse effects of HMs on earthworms are rarely discussed. Studies have shown that As(V) exposure results in a significant decline in body weight and extremely high mortality of the earthworm Metaphile sieboldi (Wang et al., 2019). It caused serious pathological damage due to arsenic uptake and accumulation through the earthworm gut and epidermal contacted with polluted soil. Meanwhile, As(V) exposure disturbs the gut microbiota, and the earthworm gut can be a reservoir of microbes capable of reducing As(V) and extruding As(III) (Wang et al., 2019). Springtails (Collembola Isotomidae) are soil organisms that have been used to study the effects of chemicals in ecotoxicity tests (van Gestel, 2012). The collembolan species Folsomia candida was used to assess the effect of major cations (Ca2+, Mg2+, Na+, K+, and H+) on Cd toxicity. Ca2+ and Mg2+ protected the springtails from Cd toxicity due to competitive absorption, while Na+, K+, and H+ had less competition with free Cd ions for binding to the uptake sites of the collembolans (Ardestani and van Gestel, 2019). These results emphasize the important role of the surrounding chemical environment in determining metal toxicity in invertebrates.
As humans are also mammals, the toxicity of HMs to humans can be predicted to some extent by studying the response of mammals. When exposed to Pb, Cd, and Hg mixtures at human environmental exposure levels, rats experienced damage to multiple organs including the brain, heart, liver, kidneys, and testicles, as well as impairments in neurobehavioral functions (Zhou et al., 2019). Statistically significant increases in liver cells CYP450 and PON1, kidney cells KIM1, and a decrease in testicle cells SDH were observed. In the brain, significant increases in oxidative stress, intracellular free Ca, and cell apoptosis were detected. Further neurobehavioral testing revealed that exposure to metal mixtures caused dose-dependent impairments in learning, memory, and sensory perception (Zhou et al., 2019). Exposure to the metal mixture also disrupted synapse remodeling, which may be associated with pathways involved in dendritic spine growth, maintenance, and elimination.
3.5 Humans
Environmental and occupational exposure to HMs poses a huge threat to human health owing to their accumulation in the food chain, direct contact through dermal exposure, and inhalation exposure (Seyfferth et al., 2019). Pb pollution was estimated to cause 0.6% of the world’s disease burden and 853,000 deaths in 2013 (Shi et al., 2019). An investigation of HM exposure in populations living in the Panasqueira mine area of central Portugal found a higher internal dose of elements such as As, Cr, Pb, Mn, Mo, and Zn in exposed individuals (Coelho et al., 2012; Coelho et al., 2014). Furthermore, HM contamination in the Panasqueira mine area had induced genotoxic damage in individuals working in the mine or living beings in the area (Coelho et al., 2013). Long-term exposure to HMs may have toxic effects on various organ systems and cause various clinical symptoms. The target of many HMs, such as As and Cd, is bone tissue which results in toxic and chronic effects (Akbal et al., 2015). Furthermore, the ingestion of Cd dust or aerosol particulate matter from soil causes chronic effects on human health, including lung cancer, pulmonary adenocarcinomas, prostatic proliferative lesions, bone fractures, kidney dysfunction, and hypertension. Chronic exposure to As dust can have adverse effects such as dermal lesions, peripheral neuropathy, skin cancer, and peripheral vascular disease (Zukowska and Biziuk, 2008). The excessive intake of Pb (PM2.5, Pb2+ ions, or organo-Pb) can damage the nervous, skeletal, circulatory, enzymatic, endocrine, and immune systems (Li et al., 2014). In general, health risks were much higher for children than for adults, suggesting that children are more vulnerable to HM pollution than adults. Therefore, children should be regarded as a priority for protection against HMs in their surroundings, especially street dust (Hou et al., 2019). Relatively low levels of HM exposure that do not greatly affect the mother may jeopardize fetal development and influence subsequent development and behavior during childhood (Horton et al., 2018; Punshon et al., 2019). In addition, As and Pb can be transported freely through the placenta (the ratio of fetal: maternal blood lead is about 0.7–0.9) and across the blood-brain barrier, as is Hg (Gundacker et al., 2010; Gundacker and Hengstschläger, 2012). Cd transfer is less distinctive but tends to accumulate in placental tissue, where it may interfere with Zn transport and affect the synthesis of endocrine hormones and cellular functions (Esteban-Vasallo et al., 2012; Chen et al., 2014). However, HMs often do not exist alone in the environment; they usually coexist in air, water, and soil and can enter the human body via a variety of routes to impact human health (Zhou et al., 2019). Pb, Cd, and Hg were measured simultaneously in blood, serum, and urine samples from the United States and Chinese populations (Wu et al., 2013; Ding et al., 2014). An observational prevalence survey in Korea showed that exposure to environmental Pb and Cd mixtures in adults and exposure to environmental Cd in adolescents may increase the risk of hearing loss (Choi and Park, 2017). In vitro studies were designed to reveal the interactions among Pb, Cd, and Hg at human environmental exposure levels in cultured cells. When individual metals were below the “no observed adverse effect” levels, the synergistic toxic effect of Pb, Cd, and Hg co-exposure was more serious than any individual metal or even the combination of two metals (Zhou et al., 2018).
At present, the cytotoxicity and genotoxicity of HMs on human cells in vivo or in vitro are mainly studied using several typical cell lines, including Jurkat cells, HepG2 cells, HeLa cells, Human hepatocarcinoma HuH-7 cells, etc. For Jurkat cells from human T-cell leukemia, both Cr(III) and Cr(VI) cause DNA damage through different mechanisms, and the effect of Cr(III) was greater than that of Cr(VI). Cr(VI) intercalates DNA and Cr(III) interfere with base-pair stacking (Table 2) (Fang et al., 2014). HeLa cells exposed to 10 μM concentrations of Cr(VI), Cr(V), or Cr(IV) displayed comet tail formation, indicating the breakage of DNA strands. Following Cr(VI) and Cr(V) exposure, and not Cr(IV), HeLa cells exhibit a significant dose-dependent decrease in DNA synthesis, representing activation of the S-phase cell cycle checkpoint (Wakeman et al., 2017). HeLa cells exposed to Cr(VI) or Cr(V) instead of Cr(IV) showed a dramatic reduction in the number of mitotic cells, indicating that Cr(VI) or Cr(V) induced G2 checkpoint activation and a lack of G2 arrest in response to Cr(IV) (Wakeman et al., 2017). It should be noted that several other cell lines, including 293T and human primary fibroblast cell lines, also show a similar reduction when exposed to Cr(VI) or Cr(V). In addition to HeLa cells, human head and neck squamous cell carcinoma or FaDu cells (pharynx epithelia), normal human lung fibroblast 19Lu cells, and mouse lung fibroblast WT13 cells treated with Cr(VI) or Cr(V) all showed ATM kinase (a DNA damage regulator) auto-phosphorylation, but cells exposed to Cr(IV) displayed no ATM phosphorylation. This suggests that the cellular DNA damage response to Cr(IV) was significantly reduced, unlike the response to Cr(V) or Cr(VI) (Wakeman et al., 2017). Different speciation of Cr shows varying cytotoxicity and genotoxicity in human cells; however, Cr(V) is the primary cytotoxic intermediate produced by the metabolism of Cr(VI). A comparison of Cr(III)-EDTA cytotoxic and genotoxic activity with Cr(VI) and Cr(III)-nitrate activity in human hepatoma (HepG2) cell lines revealed that Cr(VI) exposure resulted in a decrease in cell viability, DNA damage, and significant formation of micronuclei. Cr(III)-nitrate induced the formation of DNA strand breaks but did not affect genomic stability, while Cr(III)-EDTA complex had no influence on DNA integrity and the genomic stability of HepG2 cells (Novotnik et al., 2016). Organic HMs such as methylmercury are more biologically toxic than inorganic HMs. Methylmercury is the most toxic form of Hg, and fish contributes greatly to the Hg intake of animal and human populations. Predatory species, including swordfish, sharks, and tuna, have higher levels of mercury, and swordfish, displayed the highest content (Sevillano-Morales et al., 2015). Therefore, fish consumption may represent a risk for transferring methylmercury in humans. An investigation of the toxicity of methylmercury chloride (CH3HgCl) and methylmercury hydroxide (CH3HgOH) to the cultured neuroblastoma cell line, SH-SY5Y, was conducted (Patnaik and Padhy, 2018). The cytotoxicity and genotoxicity experiments indicated that CH3HgCl was more toxic than CH3HgOH to SH-SY5Y cells because of the more severe nuclear disruption caused by CH3HgCl.
4 Bioaccumulation of Heavy Metal(loid)s in the Food Chain
HMs remain in the environment for decades, even after the removal of their point sources, and cause long-term damage to organisms. Therefore, concerns regarding the accumulation and transfer of HMs at different levels in food chains are increasing. Many factors govern the bioaccumulation and transfer rates of metals in different ecosystems, including the properties of HMs, the response of organisms to HMs, and the structure of the food chain (Butt et al., 2018). Here, we emphasize the accumulation of HMs in the food chains of different ecosystems.
Soil, as an HM reservoir, provides a medium for HM cycling in nature (Cao et al., 2010). A portion of soil HMs enters the food chain through the producer plants (Figure 4) as the electrochemical gradient of metal ions between the plasma membrane of root cells and the soil causes their uptake in plants (Li et al., 2018). Most of the absorbed HMs are stored in the roots, and some are transferred to other plant parts; for example, hyperaccumulators accumulate HMs in their aerial parts (Cui et al., 2021). Insects occupy important positions in the food chains of most terrestrial ecosystems, as they are a protein-rich food source for numerous animal groups (Butt et al., 2018). They inadvertently absorb HMs by ingesting contaminated plants or debris. They may also absorb HMs through their exoskeletons (Hobbelen et al., 2006). HMs ingested by these insects can be transferred to their predators along the food chain (Green et al., 2010). An investigation of the accumulation of Zn, Cd, and Pb in the agricultural food chains of soil-berseem-aphid-ladybird beetles showed higher accumulation of Pb and Zn but no bioaccumulation of Cd (Butt et al., 2018). This indicated that different metals showed variable bioaccumulation rates depending on their toxicity and retention. Studies have reported that birds have a high risk of exposure to HMs through feeding on contaminated seeds and insects (Liang et al., 2016; Xia et al., 2021). This may cause the rapid transfer of metals to the local populations of animals and humans (Figure 4).
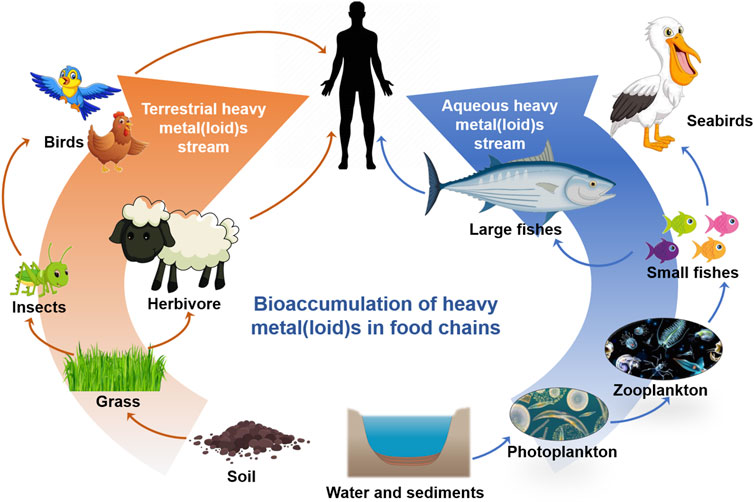
FIGURE 4. A schematic model of bioaccumulation of HMs in terrestrial and aqueous food chains. The arrow indicates the gradual enrichment of heavy metals.
HM pollution in water, including marine pollution, is a widespread environmental problem. This not only relates directly to HM exposure in humans but also to food safety. Marine organisms, such as fish, provide high-quality functional protein and polyunsaturated fatty acids omega-3, specifically eicosapentaenoic acid (EPA) and docosahexaenoic acid (DHA), which have beneficial effects on health (Matias et al., 2022). However, they can also contain contaminants such as HMs, which may represent a potential risk to human health. HMs released by natural and anthropogenic activities enter the ocean through atmospheric deposition, surface runoff, and precipitation. In marine environments, HMs can be methylated by microorganisms, bioconcentrated by phytoplankton, and bioaccumulated by organisms along the food chain (Figure 4). Among them, MeHg in prey is bioavailable for predators and is strongly retained in marine organisms once assimilated, leading to a stepwise increase in Hg concentrations along the food chain (biomagnification) and its bioaccumulation with age in organisms (Matias et al., 2022). Previous studies have demonstrated a trend of increasing Hg concentrations from producers to primary consumers to secondary consumers (Eagles-Smith et al., 2018; de Almeida Rodrigues et al., 2019). In the Southern Ocean ecosystem, the lowest Hg concentrations were observed in E. superba (0.007 ± 0.008 μg g−1), and the highest values were observed in the predator seabird Macronectes giganteus (12.090 ± 14.177 μg/g). Hg concentrations have a significant positive relationship with trophic levels, biomagnifying nearly 2 times its concentration at each level (Matias et al., 2022).
The channels of human exposure to HMs include accidental ingestion and dermal adsorption by soil and surface water. Even without direct exposure to HMs, humans can also accumulate HMs in the body through food chains, such as the intake of grains, fruits, vegetables, meats, eggs, milk and dairy products, and freshwater fish (Huang et al., 2019a). This is concerning because humans must consume a large amount of food, some of which may contain excessive HMs. Long-term exposure to HMs or accidental ingestion can cause various diseases; therefore, effective measures must be taken to prevent and limit HM distribution in the atmosphere, soil, and water.
5 Prevention and Control Strategies for Heavy Metal(loid)s
The health of humans and other organisms is threatened by anthropogenic sources of HMs, such as mining waste, automobile exhaust, and industrial effluents. Effective prevention and control of HM pollution could prevent their transfer into the environment, and thereby reduce biological exposure to HMs. Efforts should be made to reduce the concentrations of HMs in the environment to minimize their subsequent transfer to living organisms. There are three main prevention and control strategies: 1) source control is to implement cleaner production and management to reduce the discharge of HM-containing wastewater, waste gas, and waste residue; 2) process control is to cut off the channels to prevent HMs from spreading to the environment, or to disrupt the migration and transformation behaviors to eliminate HMs in the environment; 3) end treatment, once HMs enters the ecological environment, they will be treated by physical, chemical, biological, and other methods (Figure 5).
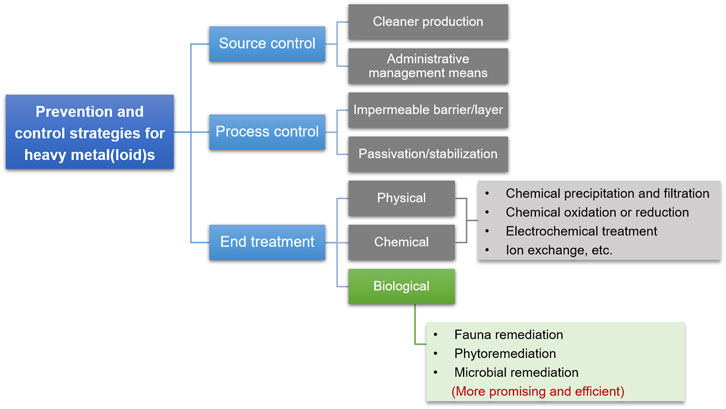
FIGURE 5. Glimpses of prevention and control strategies for HMs in the environment to mitigate health risks to organisms.
Source control is one of the most effective strategies for HM prevention and control. Cleaner production is the most common means for source control and can reduce or eliminate pollutant emissions and maximize the utilization of resources. Pollution control of coal-fired power plants includes coal washing before burning, post-combustion cleaning through selective catalytic reduction (SCR), electrostatic precipitators or fabric filters, and wet flue gas desulfurization (Zhu et al., 2016). At present this is the best available technology to control HM abatement in coal-fired power plants. Source control can diminish the discharge of HMs at the pollution source based on management, relevant departments adopting supervision, point source governance, treatment, monitoring, control, and other means. Source control measures were used to reduce the HMs in the sludge fundamentally and ensure the safety and reliability of the fertilizer made from the sewage sludge (Wenke et al., 2013). Second, effective process control can prevent HMs produced during industrial processes from entering the environment. An impermeable barrier layer is an effective and conventional strategy for controlling or minimizing the effects of solid HM-containing waste. Some special materials prepared from sewage sludge can be used as barriers for waste landfills because of their low permeability and strong retardation of HM pollutants. Zn and Cd in waste landfills are effectively retarded by compacted municipal sewage sludge owing to precipitation and adsorption (Zhang et al., 2016a). Third, the end treatment is the last barrier to avoiding direct exposure of organisms to HMs and restricts the further diffusion and migration of HMs already in the environment. Passivation and stabilization are the most common approaches to limit the further diffusion and migration of HMs in the environment and has proven an effective and economical technique. For example, Cd-contaminated water and soil were immobilized using hydroxy ferric combined acid-base modified sepiolite, which exhibited a more significant passivation effect on biologically available Cd in the soil (Xie et al., 2020). Although the source and process control of HM pollution has significantly decreased the flow of HMs into the environment, numerous HMs were already detected in the ecosystem (Singh et al., 2005; Csavina et al., 2012; Shen et al., 2017). Research on the end treatment of HMs is the most abundant and includes physical, chemical, and biological approaches. The physical and chemical processes for HM removal include chemical precipitation and filtration, chemical oxidation or reduction, electrochemical treatment, reverse osmosis, and ion exchange. The common disadvantages of physical and chemical methods are the high consumption of chemical reagents and energy, considerable costs, and secondary pollution. Therefore, bioremediation is currently the most promising and environmentally friendly method for HM end treatment. To survive in the presence of HMs, many organisms have evolved extensive networks for HM biosorption, transport, and transformation, allowing them to tolerate extreme environments. Based on these characteristics, the efficient bioremediation of HM-contaminated environments was exploited, which consists of fauna remediation such as Cd removal from biogas residues by earthworms (Sun et al., 2020), phytoremediation such as HM harvesting from soils by hyperaccumulators (Sarwar et al., 2017), and microbial remediation such as Cr(VI) and As(III) detoxification by chromate-reducing bacteria and arsenite-oxidizing bacteria (Malaviya and Singh, 2016; Ye et al., 2020).
6 Conclusion and Future Perspectives
This review underlines the issue of HM pollution worldwide and the need for immediate action. Owing to rapid industrial development, the number of HMs in the environment is still increasing, posing a serious threat to all kinds of organisms. Since all living beings are made up of same elements and substances, the toxicity of HMs to different organisms is consistent to a certain extent. At the cellular level, HM exposure causes cell membrane damage, oxidative stress, enzymatic activity change and DNA damage. At the biological individual level, HMs can lead to the inhibition of growth, the decrease of biomass, and the change of metabolic activities. Also, the reproductive organs or components, as well as the reproductive process, may be destroyed by HMs. However, there are a lot of differences in the number and function of cells from different species, so the response of organisms to HMs is quite different. Some organisms, such as archaea, bacteria, and fungi, can tolerate HMs at a certain concentration due to their own HM resistance system; certain fungi and plants can absorb and accumulate HMs in their bodies, so as to avoid the impact of HMs on their metabolism. Unfortunately, the bioaccumulation, bioadsorption, or biotransformation of HMs is limited, high level of HMs inevitably destroys these processes and cause fatal damage to organisms. In addition, the biomagnification of HMs along the food chain, which is happening all the time in the ecosystem polluted by HMs, cannot be ignored. Among all kinds of organisms, humans, which are apex predators, are at great risk of exposure to higher concentrations of HMs owing to the biomagnification of HMs along food chains. Some effective strategies preventing food from HM pollution should be implemented, including enhancing the treatment of HM pollution caused by various industries, strengthening the inspection and supervision of food safety and strictly eliminating foods with HMs, expanding the production and promotion of organic safe food with pollution-free and additive-free. However, at present, researchers pay more attention to the treatment of environmental HMs, and there are few studies to guide populations how to effectively avoid HM exposure along the food chain. Further studies on HM toxicity can focus on the influence of HMs on food safety.
In addition, a comprehensive analysis of the available literature suggests that numerous studies have concentrated on the risk of HMs in some countries or areas, rather than discussing the issue on a global scale. However, recent research has demonstrated that HMs have reached the Antarctic and Arctic regions via global water and atmospheric circulation (Marina-Montes et al., 2020; Perryman et al., 2020; Jensen et al., 2021; Matias et al., 2022). Since HMs released into the environment inevitably migrate and transform due to their physicochemical and biological properties, further research is required to assess the ecological and health risks of HMs in the natural environment on a global scale.
This review highlights the major gaps in the risk assessment of HMs and suggests that 1) the role of different industrial processes and environmental media with respect to the speciation of emitted HMs, should be explored further to clearly understand the chemical forms of HMs, thereby facilitating the development of treatment technology. 2) The database regarding human health risks due to the consumption of metal-contaminated food should be supplemented, especially for urban agriculture and aquatic products. This will help to ensure food safety. 3) Although many HM remediation technologies have been developed, efficient strategies for managing the production, usage, discharge, and recycling of HMs require further research to minimize their risk to organisms.
Author Contributions
CD contributed to the collection of the main data, the writing of the original draft, and the visualization of this paper; JC collected the partial data and wrote some sectional works; FZ, LC, and ZL contributed to the conceptualization and supervision of this paper. KZ contributed to the revision of the abstract and introduction of this paper; YS contributed to the revising and editing of the manuscript. All authors approved the submitted version.
Funding
The research is supported by the National Natural Science Foundation of China (Grant Number 2212200852) and National Key R&D Program of China (Grant Number SQ2019YFC180057).
Conflict of Interest
The authors declare that the research was conducted in the absence of any commercial or financial relationships that could be construed as a potential conflict of interest.
Publisher’s Note
All claims expressed in this article are solely those of the authors and do not necessarily represent those of their affiliated organizations, or those of the publisher, the editors and the reviewers. Any product that may be evaluated in this article, or claim that may be made by its manufacturer, is not guaranteed or endorsed by the publisher.
References
Abdu, N., Abdullahi, A. A., and Abdulkadir, A. (2016). Heavy Metals and Soil Microbes. Environ. Chem. Lett. 15 (1), 65–84. doi:10.1007/s10311-016-0587-x
Agah, H., Leermakers, M., Elskens, M., Fatemi, S. M. R., and Baeyens, W. (2009). Accumulation of Trace Metals in the Muscle and Liver Tissues of Five Fish Species from the Persian Gulf. Environ. Monit. Assess. 157 (1-4), 499–514. doi:10.1007/s10661-008-0551-8
Ahmed, D. A., and Slima, D. F. (2018). Heavy Metal Accumulation by Corchorus Olitorius L. Irrigated with Wastewater. Environ. Sci. Pollut. Res. 25 (15), 14996–15005. doi:10.1007/s11356-018-1675-1
Akbal, A., Reşorlu, H., and Savaş, Y. (2015). Toxic Effects of Heavy Metals on Bone Tissue. Tod 21 (1), 30–33. doi:10.4274/tod.31644
Almeida, S. M., Canha, N., Silva, A., Freitas, M. d. C., Pegas, P., Alves, C., et al. (2011). Children Exposure to Atmospheric Particles in Indoor of Lisbon Primary Schools. Atmos. Environ. 45 (40), 7594–7599. doi:10.1016/j.atmosenv.2010.11.052
Alvarez-Ortega, N., Caballero-Gallardo, K., and Olivero-Verbel, J. (2019). Toxicological Effects in Children Exposed to Lead: A Cross-Sectional Study at the Colombian Caribbean Coast. Environ. Int. 130, 104809. doi:10.1016/j.envint.2019.05.003
An, J., Zhang, L., Lu, X., Pelletier, D. A., Pierce, E. M., Johs, A., et al. (2019). Mercury Uptake by Desulfovibrio Desulfuricans ND132: Passive or Active? Environ. Sci. Technol. 53 (11), 6264–6272. doi:10.1021/acs.est.9b00047
Andosch, A., Affenzeller, M. J., Lütz, C., and Lütz-Meindl, U. (2012). A Freshwater Green Alga under Cadmium Stress: Ameliorating Calcium Effects on Ultrastructure and Photosynthesis in the Unicellular Model Micrasterias. J. Plant Physiology 169, 1489–1500. doi:10.1016/j.jplph.2012.06.002
Ardestani, M. M., and Van Gestel, C. A. M. (2019). The Effect of Major Cations on the Toxicity of Cadmium to Folsomia candida in a Sand-Solution Medium Analyzed by Biotic Ligand Modeling. Environ. Pollut. 246, 19–25. doi:10.1016/j.envpol.2018.11.082
Athar, R., and Ahmad, M. (2002). Heavy Metal Toxicity: Effect on Plant Growth and Metal Uptake by Wheat, and on Free Living Azotobacter. Water Air Soil Poll. 138 (1-4), 165–180. doi:10.1023/a:1015594815016
Baldrian, P. (2003). Interactions of Heavy Metals with White-Rot Fungi. Enzyme Microb. Technol. 32, 78–91. doi:10.1016/S0141-0229(02)00245-4
Balistrieri, L. S., Mebane, C. A., Cox, S. E., Puglis, H. J., Calfee, R. D., and Wang, N. (2018). Potential Toxicity of Dissolved Metal Mixtures (Cd, Cu, Pb, Zn) to Early Life Stage White Sturgeon (Acipenser transmontanus) in the Upper Columbia River, Washington, United States. Environ. Sci. Technol. 52 (17), 9793–9800. doi:10.1021/acs.est.8b02261
Bao, K.-s., Shen, J., Wang, G.-p., and Tserenpil, S. (2016). Anthropogenic, Detritic and Atmospheric Soil-Derived Sources of Lead in an Alpine Poor Fen in Northeast China. J. Mt. Sci. 13, 255–264. doi:10.1007/s11629-015-3542-6
Behboodi, B. S., and Samadi, L. (2004). Detection of Apoptotic Bodies and Oligonucleosomal DNA Fragments in Cadmium-Treated Root Apical Cells of Allium cepa Linnaeus. Plant Sci. 167, 411–416. doi:10.1016/j.plantsci.2004.04.024
Bienert, G. P., and Tamás, M. J. (2018). Editorial: Molecular Mechanisms of Metalloid Transport, Toxicity and Tolerance. Front. Cell Dev. Biol. 6, 99. doi:10.3389/fcell.2018.00099
Bugeja, R., and Shoemake, C. (2015). Heavy Metal Concentrations in Maltese Potable Water. Environments 2 (4), 186–199. doi:10.3390/environments2020186
Butt, A., Qurat-ul-Ain, A., Rehman, K., Khan, M. X., and Hesselberg, T. (2018). Bioaccumulation of Cadmium, Lead, and Zinc in Agriculture-Based Insect Food Chains. Environ. Monit. Assess. 190 (12), 698. doi:10.1007/s10661-018-7051-2
Cao, H., Chen, J., Zhang, J., Zhang, H., Qiao, L., and Men, Y. (2010). Heavy Metals in Rice and Garden Vegetables and Their Potential Health Risks to Inhabitants in the Vicinity of an Industrial Zone in Jiangsu, China. J. Environ. Sci. 22 (11), 1792–1799. doi:10.1016/S1001-0742(09)60321-1
Cao, S., Duan, X., Zhao, X., Wang, B., Ma, J., Fan, D., et al. (2015). Health Risk Assessment of Various Metal(loid)s via Multiple Exposure Pathways on Children Living Near a Typical Lead-Acid Battery Plant, China. Environ. Pollut. 200, 16–23. doi:10.1016/j.envpol.2015.02.010
Cao, X., Ma, C., Zhao, J., Musante, C., White, J. C., Wang, Z., et al. (2019). Interaction of Graphene Oxide with Co-existing Arsenite and Arsenate: Adsorption, Transformation and Combined Toxicity. Environ. Int. 131, 104992. doi:10.1016/j.envint.2019.104992
Chatterjee, A., and Abraham, J. (2019). Desorption of Heavy Metals from Metal Loaded Sorbents and E-Wastes: A Review. Biotechnol. Lett. 41 (3), 319–333. doi:10.1007/s10529-019-02650-0
Chen, L. X., Li, J. T., Chen, Y. T., Huang, L. N., Hua, Z. S., Hu, M., et al. (2013). Shifts in Microbial Community Composition and Function in the Acidification of a Lead/Zinc Mine Tailings. Environ. Microbiol. 15 (9), 2431–2444. doi:10.1111/1462-2920.12114
Chen, T., Chang, Q., Liu, J., Clevers, J. G. P. W., and Kooistra, L. (2016). Identification of Soil Heavy Metal Sources and Improvement in Spatial Mapping Based on Soil Spectral Information: A Case Study in Northwest China. Sci. Total Environ. 565, 155–164. doi:10.1016/j.scitotenv.2016.04.163
Chen, X., Shi, J., Chen, Y., Xu, X., Chen, L., Wang, H., et al. (2007). Determination of Copper Binding in Pseudomonas Putida CZ1 by Chemical Modifications and X-Ray Absorption Spectroscopy. Appl. Microbiol. Biotechnol. 74 (4), 881–889. doi:10.1007/s00253-006-0592-2
Chen, Z., Myers, R., Wei, T., Bind, E., Kassim, P., Wang, G., et al. (2014). Placental Transfer and Concentrations of Cadmium, Mercury, Lead, and Selenium in Mothers, Newborns, and Young Children. J. Expo. Sci. Environ. Epidemiol. 24 (5), 537–544. doi:10.1038/jes.2014.26
Choi, Y.-H., and Park, S. K. (2017). Environmental Exposures to Lead, Mercury, and Cadmium and Hearing Loss in Adults and Adolescents: KNHANES 2010-2012. Environ. Health Perspect. 125 (6), 067003. doi:10.1289/EHP565
Choppala, G., Kunhikrishnan, A., Seshadri, B., Park, J. H., Bush, R., and Bolan, N. (2018). Comparative Sorption of Chromium Species as Influenced by pH, Surface Charge and Organic Matter Content in Contaminated Soils. J. Geochem. Explor. 184, 255–260. doi:10.1016/j.gexplo.2016.07.012
Chowdhury, S., Mazumder, M. A. J., Al-Attas, O., and Husain, T. (2016). Heavy Metals in Drinking Water: Occurrences, Implications, and Future Needs in Developing Countries. Sci. Total Environ. 569-570, 476–488. doi:10.1016/j.scitotenv.2016.06.166
Clarkson, T. W., and Magos, L. (2006). The Toxicology of Mercury and its Chemical Compounds. Crit. Rev. Toxicol. 36 (8), 609–662. doi:10.1080/10408440600845619
Coelho, P., Costa, S., Costa, C., Silva, S., Walter, A., Ranville, J., et al. (2014). Biomonitoring of Several Toxic Metal(loid)s in Different Biological Matrices from Environmentally and Occupationally Exposed Populations from Panasqueira Mine Area, Portugal. Environ. Geochem. Health 36 (2), 255–269. doi:10.1007/s10653-013-9562-7
Coelho, P., Costa, S., Silva, S., Walter, A., Ranville, J., Sousa, A. C. A., et al. (2012). Metal(loid) Levels in Biological Matrices from Human Populations Exposed to Mining Contamination-Panasqueira Mine (Portugal). J. Toxicol. Environ. Health, Part A 75 (13-15), 893–908. doi:10.1080/15287394.2012.690705
Coelho, P., García-Lestón, J., Costa, S., Costa, C., Silva, S., Dall'armi, V., et al. (2013). Genotoxic Effect of Exposure to Metal(loid)s. A Molecular Epidemiology Survey of Populations Living and Working in Panasqueira Mine Area, Portugal. Environ. Int. 60, 163–170. doi:10.1016/j.envint.2013.08.014
Collins, P. J., and Dobson, A. (1997). Regulation of Laccase Gene Transcription in Trametes versicolor. Appl. Environ. Microbiol. 63, 3444–3450. doi:10.1128/AEM.63.9.3444-3450.1997
Csavina, J., Field, J., Taylor, M. P., Gao, S., Landázuri, A., Betterton, E. A., et al. (2012). A Review on the Importance of Metals and Metalloids in Atmospheric Dust and Aerosol from Mining Operations. Sci. Total Environ. 433, 58–73. doi:10.1016/j.scitotenv.2012.06.013
Cui, L., Noerpel, M. R., Scheckel, K. G., and Ippolito, J. A. (2019). Wheat Straw Biochar Reduces Environmental Cadmium Bioavailability. Environ. Int. 126, 69–75. doi:10.1016/j.envint.2019.02.022
Cui, X., Zhang, J., Wang, X., Pan, M., Lin, Q., Khan, K. Y., et al. (2021). A Review on the Thermal Treatment of Heavy Metal Hyperaccumulator: Fates of Heavy Metals and Generation of Products. J. Hazard. Mater. 405, 123832. doi:10.1016/j.jhazmat.2020.123832
Dago, À., González, I., Ariño, C., Martínez-Coronado, A., Higueras, P., Díaz-Cruz, J. M., et al. (2014). Evaluation of Mercury Stress in Plants from the Almadén Mining District by Analysis of Phytochelatins and Their Hg Complexes. Environ. Sci. Technol. 48 (11), 6256–6263. doi:10.1021/es405619y
Darlington, A. B., and Rauser, W. E. (1988). Cadmium Alters the Growth of the Ectomycorrhizal Fungus Paxillus Involutes: A New Growth Model Accounts for Changes in Branching. Can. J. Bot. 66 (2), 225–229. doi:10.1139/b88-038
Das, D., Salgaonkar, B. B., Mani, K., and Braganca, J. M. (2014). Cadmium Resistance in Extremely Halophilic Archaeon Haloferax Strain BBK2. Chemosphere 112, 385–392. doi:10.1016/j.chemosphere.2014.04.058
Das, S. K., Mukherjee, M., and Guha, A. K. (2008). Interaction of Chromium with Resistant Strain Aspergillus versicolor: Investigation with Atomic Force Microscopy and Other Physical Studies. Langmuir 24 (16), 8643–8650. doi:10.1021/la800958u
de Almeida Rodrigues, P., Ferrari, R. G., Dos Santos, L. N., and Conte Junior, C. A. (2019). Mercury in Aquatic Fauna Contamination: A Systematic Review on its Dynamics and Potential Health Risks. J. Environ. Sci. 84, 205–218. doi:10.1016/j.jes.2019.02.018
Dey, U., and Mondal, N. K. (2016). Ultrastructural Deformation of Plant Cell under Heavy Metal Stress in Gram Seedlings. Cogent Environ. Sci. 2 (1), 1196472. doi:10.1080/23311843.2016.1196472
Dhawale, S. S., Lane, A. C., and Dhawale, S. W. (1996). Effects of Mercury on the White Rot Fungus Phanerochaete Chrysosporium. Bull. Environ. Contam. Toxicol. 56, 825–832. doi:10.1007/s001289900120
Di, X., Beesley, L., Zhang, Z., Zhi, S., Jia, Y., and Ding, Y. (2019). Microbial Arsenic Methylation in Soil and Uptake and Metabolism of Methylated Arsenic in Plants: A Review. Ijerph 16 (24), 5012. doi:10.3390/ijerph16245012
Ding, C., Pan, Y., Zhang, A., Wu, B., Huang, H., Zhu, C., et al. (2014). Study of Distribution and Influencing Factors of Lead and Cadmium in Whole Blood and Urine Among Population in 8 Provinces in China. Chin. J. Prev. Med. 48 (2), 91–96.
Dixit, V., Pandey, V., and Shyam, R. (2002). Chromium Ions Inactivate Electron Transport and Enhance Superoxide Generationin Vivoin Pea (Pisum sativumL. Cv. Azad) Root Mitochondria. Plant Cell Environ. 25, 687–693. doi:10.1046/j.1365-3040.2002.00843.x
Dotaniya, M. L., Das, H., and Meena, V. D. (2014). Assessment of Chromium Efficacy on Germination, Root Elongation, and Coleoptile Growth of Wheat (Triticum aestivum L.) at Different Growth Periods. Environ. Monit. Assess. 186 (5), 2957–2963. doi:10.1007/s10661-013-3593-5
Dubey, S., Shri, M., Gupta, A., Rani, V., and Chakrabarty, D. (2018). Toxicity and Detoxification of Heavy Metals during Plant Growth and Metabolism. Environ. Chem. Lett. 16 (4), 1169–1192. doi:10.1007/s10311-018-0741-8
Eagles-Smith, C. A., Silbergeld, E. K., Basu, N., Bustamante, P., Diaz-Barriga, F., Hopkins, W. A., et al. (2018). Modulators of Mercury Risk to Wildlife and Humans in the Context of Rapid Global Change. Ambio 47 (2), 170–197. doi:10.1007/s13280-017-1011-x
Edgcomb, V. P., Molyneaux, S. J., Saito, M. A., Lloyd, K., Böer, S., Wirsen, C. O., et al. (2004). Sulfide Ameliorates Metal Toxicity for Deep-Sea Hydrothermal Vent Archaea. Appl. Environ. Microbiol. 70 (4), 2551–2555. doi:10.1128/AEM.70.4.2551-2555.2004
Eichler, A., Gramlich, G., Kellerhals, T., Tobler, L., and Schwikowski, M. (2015). Pb Pollution from Leaded Gasoline in South America in the Context of A 2000-Year Metallurgical History. Sci. Adv. 1 (2), e1400196. doi:10.1126/sciadv.1400196
Esteban-Vasallo, M. D., Aragonés, N., Pollan, M., López-Abente, G., and Perez-Gomez, B. (2012). Mercury, Cadmium, and Lead Levels in Human Placenta: A Systematic Review. Environ. Health Perspect. 120 (10), 1369–1377. doi:10.1289/ehp.1204952
Falih, A. M. (1998). Impact of Heavy Metals on Cellulolytic Activity of Some Soil Fungi. Kuwait. J. Sci. Eng. 25, 396–406.
Fan, X., Ding, S., Chen, M., Gao, S., Fu, Z., Gong, M., et al. (2019). Peak Chromium Pollution in Summer and Winter Caused by High Mobility of Chromium in Sediment of a Eutrophic Lake: In Situ Evidence from High Spatiotemporal Sampling. Environ. Sci. Technol. 53 (9), 4755–4764. doi:10.1021/acs.est.8b07060
Fang, Z., Zhao, M., Zhen, H., Chen, L., Shi, P., and Huang, Z. (2014). Genotoxicity of Tri- and Hexavalent Chromium Compounds In Vivo and Their Modes of Action on DNA Damage In Vitro. PLoS One 9 (8), e103194. doi:10.1371/journal.pone.0103194
Favero, N., Costa, P., and Rocco, G. P. (1990). Role of Copper in Cadmium Metabolism in the Basidiomycetes Pleurotus Ostreatus. Comp. Biochem. Physiology Part C Comp. Pharmacol. 97, 297–303. doi:10.1016/0742-8413(90)90145-Y
Fujimori, T., and Takigami, H. (2014). Pollution Distribution of Heavy Metals in Surface Soil at an Informal Electronic-Waste Recycling Site. Environ. Geochem. Health 36 (1), 159–168. doi:10.1007/s10653-013-9526-y
Gabriel, J., Capelari, M., Rychlovský, P., Krenželok, M., and Zadražil, F. (1996a). Influence of Cadmium on the Growth ofAgrocybe Perfectaand twoPleurotusspp and Translocation from Polluted Substrate and Soil to Fruitbodies. Toxicol. Environ. Chem. 56, 141–146. doi:10.1080/02772249609358356
Gabriel, J., Mokrejš, M., Bílý, J., and Rychlovský, P. (1994). Accumulation of Heavy Metals by Some Wood-Rotting Fungi. Folia Microbiol. 39, 115–118. doi:10.1007/BF02906805
Gabriel, J., ová, O. K. e., Rychlovský, P., and elok, M. K. (1996b). Accumulation and Effect of Cadmium in the Wood-Rotting Basidiomycete Daedalea Quercina. Bull. Environ. Contam. Toxicol. 57, 383–390. doi:10.1007/s001289900202
Gall, J. E., Boyd, R. S., and Rajakaruna, N. (2015). Transfer of Heavy Metals through Terrestrial Food Webs: A Review. Environ. Monit. Assess. 187 (4), 201. doi:10.1007/s10661-015-4436-3
Gallego, S. M., Benavídes, M. P., and Tomaro, M. L. (1996). Effect of Heavy Metal Ion Excess on Sunflower Leaves: Evidence for Involvement of Oxidative Stress. Plant Sci. 121 (2), 151–159. doi:10.1016/S0168-9452(96)04528-1
Gang, H., Xiao, C., Xiao, Y., Yan, W., Bai, R., Ding, R., et al. (2019). Proteomic Analysis of the Reduction and Resistance Mechanisms of Shewanella Oneidensis MR-1 under Long-Term Hexavalent Chromium Stress. Environ. Int. 127, 94–102. doi:10.1016/j.envint.2019.03.016
Gao, X., and Li, P. (2012). Concentration and Fractionation of Trace Metals in Surface Sediments of Intertidal Bohai Bay, China. Mar. Pollut. Bull. 64 (8), 1529–1536. doi:10.1016/j.marpolbul.2012.04.026
Gao, Y., Wang, Y., Qian, J., Si, W., Tan, Q., Xu, J., et al. (2020). Melatonin Enhances the Cadmium Tolerance of Mushrooms through Antioxidant-Related Metabolites and Enzymes. Food Chem. 330, 127263. doi:10.1016/j.foodchem.2020.127263
Garnier, L., Simon-Plas, F., Thuleau, P., Agnel, J.-P., Blein, J.-P., Ranjeva, R., et al. (2006). Cadmium Affects Tobacco Cells by A Series of Three Waves of Reactive Oxygen Species that Contribute to Cytotoxicity. Plant Cell Environ. 29, 1956–1969. doi:10.1111/j.1365-3040.2006.01571.x
Ghosh, S., Sadhukhan, P. C., Chaudhuri, J., Ghosh, D. K., and Mandal, A. (1999). Purification and Properties of Mercuric Reductase from Azotobacter Chroococcum. J. Appl. Microbiol. 86, 7–12. doi:10.1046/j.1365-2672.1999.00605.x
Gianì, F., Masto, R., Trovato, M. A., Malandrino, P., Russo, M., Pellegriti, G., et al. (2021). Heavy Metals in the Environment and Thyroid Cancer. Cancers 13 (16), 4052. doi:10.3390/cancers13164052
Gjorgieva Ackova, D. (2018). Heavy Metals and Their General Toxicity for Plants. Plant Sci. Today 5 (1), 14–18. doi:10.14719/pst.2018.5.1.355
Gola, D., Dey, P., Bhattacharya, A., Mishra, A., Malik, A., Namburath, M., et al. (2016). Multiple Heavy Metal Removal Using an Entomopathogenic Fungi Beauveria Bassiana. Bioresour. Technol. 218, 388–396. doi:10.1016/j.biortech.2016.06.096
Gray, J. E., Theodorakos, P. M., Fey, D. L., and Krabbenhoft, D. P. (2015). Mercury Concentrations and Distribution in Soil, Water, Mine Waste Leachates, and Air in and Around Mercury Mines in the Big Bend Region, Texas, USA. Environ. Geochem Health 37 (1), 35–48. doi:10.1007/s10653-014-9628-1
Green, I. D., Diaz, A., and Tibbett, M. (2010). Factors Affecting the Concentration in Seven-Spotted Ladybirds (Coccinella septempunctata L.) of Cd and Zn Transferred through the Food Chain. Environ. Pollut. 158 (1), 135–141. doi:10.1016/j.envpol.2009.07.032
Gress, J., De Oliveira, L. M., Da Silva, E. B., Lessl, J. M., Wilson, P. C., Townsend, T., et al. (2015). Cleaning-induced Arsenic Mobilization and Chromium Oxidation from CCA-Wood Deck: Potential Risk to Children. Environ. Int. 82, 35–40. doi:10.1016/j.envint.2015.04.012
Gu, S., Kang, X., Wang, L., Lichtfouse, E., and Wang, C. (2019). Clay Mineral Adsorbents for Heavy Metal Removal from Wastewater: A Review. Environ. Chem. Lett. 17 (2), 629–654. doi:10.1007/s10311-018-0813-9
Gundacker, C., Fröhlich, S., Graf-Rohrmeister, K., Eibenberger, B., Jessenig, V., Gicic, D., et al. (2010). Perinatal Lead and Mercury Exposure in Austria. Sci. Total Environ. 408 (23), 5744–5749. doi:10.1016/j.scitotenv.2010.07.079
Gundacker, C., and Hengstschläger, M. (2012). The Role of the Placenta in Fetal Exposure to Heavy Metals. Wien. Med. Wochenschr. 162 (9), 201–206. doi:10.1007/s10354-012-0074-3
Guo, J., Kang, Y., and Feng, Y. (2017). Bioassessment of Heavy Metal Toxicity and Enhancement of Heavy Metal Removal by Sulfate-Reducing Bacteria in the Presence of Zero Valent Iron. J. Environ. Manag. 203 (Pt 1), 278–285. doi:10.1016/j.jenvman.2017.07.075
Guo, Y., Feng, H., Chen, C., Jia, C. J., Xiong, F., and Lu, Y. (2013). Heavy Metal Concentrations in Soil and Agricultural Products Near an Industrial District. Pol. J. Environ. Stud. 22, 1357–1362.
Gupta, A., and Joia, J. (2016). Microbes as Potential Tool for Remediation of Heavy Metals: A Review. J. Microb. Biochem. Technol. 8. doi:10.4172/1948-5948.1000310
Hassan, S. K. M. (2012). Metal Concentrations and Distribution in the Household, Stairs and Entryway Dust of Some Egyptian Homes. Atmos. Environ. 54, 207–215. doi:10.1016/j.atmosenv.2012.02.013
Hassana, I. M., Omotayo, B. A., Hassana, I. M., and Omotayo, B. A. (2014). Heavy Metals Accumulation in Edible Part of Vegetables Irrigated with Untreated Municipal Wastewater in Tropical Savannah Zone, Nigeria. Afr. J. Environ. Sci. Technol. 8, 460–463. doi:10.5897/AJEST2013.1531
Hausladen, D. M., Alexander-Ozinskas, A., Mcclain, C., and Fendorf, S. (2018). Hexavalent Chromium Sources and Distribution in California Groundwater. Environ. Sci. Technol. 52 (15), 8242–8251. doi:10.1021/acs.est.7b06627
He, J., Lü, C., Fan, Q., Xue, H., and Bao, J. (2011). Distribution of AVS-SEM, Transformation Mechanism and Risk Assessment of Heavy Metals in the Nanhai Lake in China. Environ. Earth Sci. 64 (8), 2025–2037. doi:10.1007/s12665-011-1022-z
Hesterberg, D., Sayers, D. E., Zhou, W., Plummer, G. M., and Robarge, W. P. (1997). X-ray Absorption Spectroscopy of Lead and Zinc Speciation in a Contaminated Groundwater Aquifer. Environ. Sci. Technol. 31 (10), 2840–2846. doi:10.1021/es970077w
Hobbelen, P. H. F., Koolhaas, J. E., and Van Gestel, C. A. M. (2006). Bioaccumulation of Heavy Metals in the Earthworms Lumbricus Rubellus and Aporrectodea Caliginosa in Relation to Total and Available Metal Concentrations in Field Soils. Environ. Pollut. 144 (2), 639–646. doi:10.1016/j.envpol.2006.01.019
Horton, M. K., Hsu, L., Claus Henn, B., Margolis, A., Austin, C., Svensson, K., et al. (2018). Dentine Biomarkers of Prenatal and Early Childhood Exposure to Manganese, Zinc and Lead and Childhood Behavior. Environ. Int. 121 (Pt 1), 148–158. doi:10.1016/j.envint.2018.08.045
Hou, D., He, J., Lü, C., Ren, L., Fan, Q., Wang, J., et al. (2013). Distribution Characteristics and Potential Ecological Risk Assessment of Heavy Metals (Cu, Pb, Zn, Cd) in Water and Sediments from Lake Dalinouer, China. Ecotoxicol. Environ. Saf. 93, 135–144. doi:10.1016/j.ecoenv.2013.03.012
Hou, D., O'connor, D., Nathanail, P., Tian, L., and Ma, Y. (2017). Integrated GIS and Multivariate Statistical Analysis for Regional Scale Assessment of Heavy Metal Soil Contamination: A Critical Review. Environ. Pollut. 231, 1188–1200. doi:10.1016/j.envpol.2017.07.021
Hou, S., Zheng, N., Tang, L., Ji, X., Li, Y., and Hua, X. (2019). Pollution Characteristics, Sources, and Health Risk Assessment of Human Exposure to Cu, Zn, Cd and Pb Pollution in Urban Street Dust across China between 2009 and 2018. Environ. Int. 128, 430–437. doi:10.1016/j.envint.2019.04.046
Hu, L., Nie, Z., Wang, W., Zhang, D., Long, Y., and Fang, C. (2021). Arsenic Transformation Behavior Mediated by Arsenic Functional Genes in Landfills. J. Hazard. Mater. 403, 123687. doi:10.1016/j.jhazmat.2020.123687
Hu, Y., Cheng, H., and Tao, S. (2016). The Challenges and Solutions for Cadmium-Contaminated Rice in China: A Critical Review. Environ. Int. 92-93, 515–532. doi:10.1016/j.envint.2016.04.042
Hu, Y., Li, J., Lou, B., Wu, R., Wang, G., Lu, C., et al. (2020). The Role of Reactive Oxygen Species in Arsenic Toxicity. Biomolecules 10 (2), 240. doi:10.3390/biom10020240
Huang, M., Wang, X., Wang, B., Jia, S., Ye, L., Li, Z., et al. (2019a). Projections of Long-Term Human Multimedia Exposure to Metal(loid)s and the Health Risks Derived from Atmospheric Deposition: A Case Study in the Pearl River Delta Region, South China. Environ. Int. 132, 105051. doi:10.1016/j.envint.2019.105051
Huang, N., Mao, J., Zhao, Y., Hu, M., and Wang, X. (2019b). Multiple Transcriptional Mechanisms Collectively Mediate Copper Resistance in Cupriavidus Gilardii CR3. Environ. Sci. Technol. 53 (8), 4609–4618. doi:10.1021/acs.est.8b06787
Iannone, M. F., Rosales, E. P., Groppa, M. D., and Benavides, M. P. (2010). Reactive Oxygen Species Formation and Cell Death in Catalase-Deficient Tobacco Leaf Disks Exposed to Cadmium. Protoplasma 245, 15–27. doi:10.1007/s00709-009-0097-9
Islam, M. A., Karim, M. R., Higuchi, T., Sakakibara, H., and Sekine, M. (2013). Comparison of the Trace Metal Concentration of Drinking Water Supply Options in Southwest Coastal Areas of Bangladesh. Appl. Water Sci. 4 (2), 183–191. doi:10.1007/s13201-013-0140-z
Itabashi, T., Li, J., Hashimoto, Y., Ueshima, M., Sakanakura, H., Yasutaka, T., et al. (2019). Speciation and Fractionation of Soil Arsenic from Natural and Anthropogenic Sources: Chemical Extraction, Scanning Electron Microscopy, and Micro-XRF/XAFS Investigation. Environ. Sci. Technol. 53 (24), 14186–14193. doi:10.1021/acs.est.9b03864
Jacob, C., Courbot, M., Brun, A., Steinman, H. M., Jacquot, J.-P., Botton, B., et al. (2001). Molecular Cloning, Characterization and Regulation by Cadmium of a Superoxide Dismutase from the Ectomycorrhizal fungusPaxillus Involutus. Eur. J. Biochem./FEBS 268, 3223–3232. doi:10.1046/j.1432-1327.2001.02216.x
Jensen, L. T., Lanning, N. T., Marsay, C. M., Buck, C. S., Aguilar‐Islas, A. M., Rember, R., et al. (2021). Biogeochemical Cycling of Colloidal Trace Metals in the Arctic Cryosphere. J. Geophys. Res. Oceans 126 (8), e2021JC017394. doi:10.1029/2021JC017394
Ji, C., Wang, Q., Wu, H., Tan, Q., and Wang, W.-X. (2015). A Metabolomic Investigation of the Effects of Metal Pollution in Oysters Crassostrea Hongkongensis. Mar. Pollut. Bull. 90 (1-2), 317–322. doi:10.1016/j.marpolbul.2014.11.006
Kalaivanan, D., and Ganeshamurthy, A. N. (2016). “Mechanisms of Heavy Metal Toxicity in Plants,’ in Abiotic Stress Physiology of Horticultural Crops (India: Springer). doi:10.1007/978-81-322-2725-0_5
Kapahi, M., and Sachdeva, S. (2017). Mycoremediation Potential of Pleurotus Species for Heavy Metals: A Review. Bioresour. Bioprocess. 4 (1), 32. doi:10.1186/s40643-017-0162-8
Karbassi, A. R., Monavari, S. M., Nabi Bidhendi, G. R., Nouri, J., and Nematpour, K. (2007). Metal Pollution Assessment of Sediment and Water in the Shur River. Environ. Monit. Assess. 147 (1), 107–116. doi:10.1007/s10661-007-0102-8
Kelebemang, R., Dinake, P., Sehube, N., Daniel, B., Totolo, O., and Laetsang, M. (2017). Speciation and Mobility of Lead in Shooting Range Soils. Chem. Speciat. Bioavailab. 29 (1), 143–152. doi:10.1080/09542299.2017.1349552
Khan, K., Lu, Y., Khan, H., Ishtiaq, M., Khan, S., Waqas, M., et al. (2013). Heavy Metals in Agricultural Soils and Crops and Their Health Risks in Swat District, Northern Pakistan. Food Chem. Toxicol. 58, 449–458. doi:10.1016/j.fct.2013.05.014
Khan, S., Rehman, S., Zeb Khan, A., Amjad Khan, M., and Tahir Shah, M. (2010). Soil and Vegetables Enrichment with Heavy Metals from Geological Sources in Gilgit, Northern Pakistan. Ecotoxicol. Environ. Saf. 73, 1820–1827. doi:10.1016/j.ecoenv.2010.08.016
Krishna, A. K., Satyanarayanan, M., and Govil, P. K. (2009). Assessment of Heavy Metal Pollution in Water Using Multivariate Statistical Techniques in an Industrial Area: A Case Study from Patancheru, Medak District, Andhra Pradesh, India. J. Hazard. Mater. 167 (1-3), 366–373. doi:10.1016/j.jhazmat.2008.12.131
Kumari, V., Yadav, A., Haq, I., Kumar, S., Bharagava, R. N., Singh, S. K., et al. (2016). Genotoxicity Evaluation of Tannery Effluent Treated with Newly Isolated Hexavalent Chromium Reducing Bacillus Cereus. J. Environ. Manag. 183, 204–211. doi:10.1016/j.jenvman.2016.08.017
Kushwaha, A., Hans, N., Kumar, S., and Rani, R. (2018). A Critical Review on Speciation, Mobilization and Toxicity of Lead in Soil-Microbe-Plant System and Bioremediation Strategies. Ecotoxicol. Environ. Saf. 147, 1035–1045. doi:10.1016/j.ecoenv.2017.09.049
Lai, Z., He, M., Lin, C., Ouyang, W., and Liu, X. (2022). Interactions of Antimony with Biomolecules and its Effects on Human Health. Ecotoxicol. Environ. Saf. 233, 113317. doi:10.1016/j.ecoenv.2022.113317
Lee, G.-S., Kim, P.-R., Han, Y.-J., Holsen, T. M., Seo, Y.-S., and Yi, S.-M. (2016). Atmospheric Speciated Mercury Concentrations on an Island between China and Korea: Sources and Transport Pathways. Atmos. Chem. Phys. 16 (6), 4119–4133. doi:10.5194/acp-16-4119-2016
Lemire, J. A., Harrison, J. J., and Turner, R. J. (2013). Antimicrobial Activity of Metals: Mechanisms, Molecular Targets and Applications. Nat. Rev. Microbiol. 11 (6), 371–384. doi:10.1038/nrmicro3028
Li, H.-B., Li, M.-Y., Zhao, D., Li, J., Li, S.-W., Juhasz, A. L., et al. (2019). Oral Bioavailability of as, Pb, and Cd in Contaminated Soils, Dust, and Foods Based on Animal Bioassays: A Review. Environ. Sci. Technol. 53 (18), 10545–10559. doi:10.1021/acs.est.9b03567
Li, J.-t., Gurajala, H. K., Wu, L.-h., Van Der Ent, A., Qiu, R.-l., Baker, A. J. M., et al. (2018). Hyperaccumulator Plants from China: A Synthesis of the Current State of Knowledge. Environ. Sci. Technol. 52 (21), 11980–11994. doi:10.1021/acs.est.8b01060
Li, P., Lin, C., Cheng, H., Duan, X., and Lei, K. (2015). Contamination and Health Risks of Soil Heavy Metals Around a Lead/zinc Smelter in Southwestern China. Ecotoxicol. Environ. Saf. 113, 391–399. doi:10.1016/j.ecoenv.2014.12.025
Li, R.-Y., Yang, H., Zhou, Z.-G., Lü, J.-J., Shao, X.-H., and Jin, F. (2007). Fractionation of Heavy Metals in Sediments from Dianchi Lake, China. Pedosphere 17 (2), 265–272. doi:10.1016/S1002-0160(07)60033-2
Li, Y., Gong, X., Xiong, J., Sun, Y., Shu, Y., Niu, D., et al. (2021). Different Dissolved Organic Matters Regulate the Bioavailability of Heavy Metals and Rhizosphere Microbial Activity in a Plant-Wetland Soil System. J. Environ. Chem. Eng. 9 (6), 106823. doi:10.1016/j.jece.2021.106823
Li, Z., Ma, Z., Van Der Kuijp, T. J., Yuan, Z., and Huang, L. (2014). A Review of Soil Heavy Metal Pollution from Mines in China: Pollution and Health Risk Assessment. Sci. Total Environ. 468-469, 843–853. doi:10.1016/j.scitotenv.2013.08.090
Liang, J., Liu, J., Yuan, X., Zeng, G., Yuan, Y., Wu, H., et al. (2016). A Method for Heavy Metal Exposure Risk Assessment to Migratory Herbivorous Birds and Identification of Priority Pollutants/Areas in Wetlands. Environ. Sci. Pollut. Res. 23 (12), 11806–11813. doi:10.1007/s11356-016-6372-3
Lilly, W., Wallweber, J., and Lukefahr, T. A. (1992). Cadmium Absorption and its Effects on Growth and Mycelial Morphology of the Basidiomycete Fungus, Schizophyllum Commune. Microbios 72, 227–237.
Lin, H., Wang, C., Zhao, H., Chen, G., and Chen, X. (2020). A Subcellular Level Study of Copper Speciation Reveals the Synergistic Mechanism of Microbial Cells and EPS Involved in Copper Binding in Bacterial Biofilms. Environ. Pollut. 263 (Pt A), 114485. doi:10.1016/j.envpol.2020.114485
Liu, X., Lou, C., Xu, L., and Sun, L. (2012). Distribution and Bioavailability of Cadmium in Ornithogenic Coral-Sand Sediments of the Xisha Archipelago, South China Sea. Environ. Pollut. 168, 151–160. doi:10.1016/j.envpol.2012.04.018
M, A., Azlan, A., S, M., Effendi Halmi, M., and Radyaqsa, M. (2012). Heavy Metals (Mercury, Arsenic, Cadmium, Plumbum) in Selected Marine Fish and Shellfish along the Straits of Malacca. Int. Food. Res. J. 19, 135–140.
Maanan, M., Ruiz-fernández, A. C., Maanan, M., Fattal, P., Zourarah, B., and Sahabi, M. (2014). A Long-Term Record of Land Use Change Impacts on Sediments in Oualidia Lagoon, Morocco. Int. J. Sediment Res. 29 (1), 1–10. doi:10.1016/s1001-6279(14)60017-2
Maanan, M., Saddik, M., Maanan, M., Chaibi, M., Assobhei, O., and Zourarah, B. (2015). Environmental and Ecological Risk Assessment of Heavy Metals in Sediments of Nador Lagoon, Morocco. Ecol. Indic. 48, 616–626. doi:10.1016/j.ecolind.2014.09.034
Macomber, L., and Hausinger, R. P. (2011). Mechanisms of Nickel Toxicity in Microorganisms. Metallomics 3 (11), 1153–1162. doi:10.1039/c1mt00063b
Malaviya, P., and Singh, A. (2016). Bioremediation of Chromium Solutions and Chromium Containing Wastewaters. Crit. Rev. Microbiol. 42 (4), 607–633. doi:10.3109/1040841X.2014.974501
Malik, N., Biswas, A. K., Qureshi, T. A., Borana, K., and Virha, R. (2010). Bioaccumulation of Heavy Metals in Fish Tissues of a Freshwater Lake of Bhopal. Environ. Monit. Assess. 160 (1-4), 267–276. doi:10.1007/s10661-008-0693-8
Mallick, S., Sinam, G., Kumar Mishra, R., and Sinha, S. (2010). Interactive Effects of Cr and Fe Treatments on Plants Growth, Nutrition and Oxidative Status in Zea mays L. Ecotoxicol. Environ. Saf. 73 (5), 987–995. doi:10.1016/j.ecoenv.2010.03.004
Mandal, B., and Suzuki, K. T. (2002). Arsenic Round the World: A Review. Talanta 58 (1), 201–235. doi:10.1016/S0039-9140(02)00268-0
Mandal, T. K., Baldrian, P., Gabriel, J., Nerud, F., and Zadražil, F. (1998). Effect of Mercury on the Growth of Wood-rotting Basidiomycetes Pleurotus Ostreatus, Pycnoporus Cinnabarinus and Serpula lacrymans. Chemosphere 36, 435–440. doi:10.1016/S0045-6535(97)00363-9
Marina-Montes, C., Pérez-Arribas, L. V., Escudero, M., Anzano, J., and Cáceres, J. O. (2020). Heavy Metal Transport and Evolution of Atmospheric Aerosols in the Antarctic Region. Sci. Total Environ. 721, 137702. doi:10.1016/j.scitotenv.2020.137702
Matias, R. S., Guímaro, H. R., Bustamante, P., Seco, J., Chipev, N., Fragão, J., et al. (2022). Mercury Biomagnification in an Antarctic Food Web of the Antarctic Peninsula. Environ. Pollut. 304, 119199. doi:10.1016/j.envpol.2022.119199
Moberly, J. G., Staven, A., Sani, R. K., and Peyton, B. M. (2010). Influence of pH and Inorganic Phosphate on Toxicity of Zinc to Arthrobacter Sp. Isolated from Heavy-Metal-Contaminated Sediments. Environ. Sci. Technol. 44 (19), 7302–7308. doi:10.1021/es100117f
Muñoz, C., Guillén, F., Martínez, A. T., and Martínez, M. J. (1997). Induction and Characterization of Laccase in the Ligninolytic Fungus Pleurotus Eryngii. Curr. Microbiol. 34, 1–5. doi:10.1007/s002849900134
Murthy, S., Bali, G., and Sarangi, S. K. (2014). Effect of Lead on Growth, Protein and Biosorption Capacity of Bacillus Cereus Isolated from Industrial Effluent. J. Environ. Biol. 35 (2), 407–411.
Nagajyoti, P. C., Lee, K. D., and Sreekanth, T. V. M. (2010). Heavy Metals, Occurrence and Toxicity for Plants: A Review. Environ. Chem. Lett. 8 (3), 199–216. doi:10.1007/s10311-010-0297-8
Nahar, K., Ali, M. M., Khanom, A., Alam, M. K., Azad, M. A. K., and Rahman, M. M. (2020). Levels of Heavy Metal Concentrations and Their Effect on Net Nitrification Rates and Nitrifying Archaea/Bacteria in Paddy Soils of Bangladesh. Appl. Soil Ecol. 156, 103697. doi:10.1016/j.apsoil.2020.103697
Naik, U. C., Das, M. T., Sauran, S., and Thakur, I. S. (2014). Assessment of In Vitro Cyto/genotoxicity of Sequentially Treated Electroplating Effluent on the Human Hepatocarcinoma HuH-7 Cell Line. Mutat. Research/Genetic Toxicol. Environ. Mutagen. 762, 9–16. doi:10.1016/j.mrgentox.2013.12.006
Nicolaus, B., Poli, A., Di Donato, P., Romano, I., Laezza, G., Gioiello, A., et al. (2016). Pb2+ Effects on Growth, Lipids, and Protein and DNA Profiles of the Thermophilic Bacterium Thermus Thermophilus. Microorganisms 4 (4). doi:10.3390/microorganisms4040045
Nouri, J., Mahvi, A. H., Jahed, G. R., and Babaei, A. A. (2008). Regional Distribution Pattern of Groundwater Heavy Metals Resulting from Agricultural Activities. Environ. Geol. 55 (6), 1337–1343. doi:10.1007/s00254-007-1081-3
Novotnik, B., Ščančar, J., Milačič, R., Filipič, M., and Žegura, B. (2016). Cytotoxic and Genotoxic Potential of Cr(VI), Cr(III)-nitrate and Cr(III)-EDTA Complex in Human Hepatoma (HepG2) Cells. Chemosphere 154, 124–131. doi:10.1016/j.chemosphere.2016.03.118
Nzengue, Y., Candéias, S. M., Sauvaigo, S., Douki, T., Favier, A., Rachidi, W., et al. (2011). The Toxicity Redox Mechanisms of Cadmium Alone or Together with Copper and Zinc Homeostasis Alteration: Its Redox Biomarkers. J. Trace Elem. Med. Biol. 25, 171–180. doi:10.1016/j.jtemb.2011.06.002
Oh, S.-J., and Koh, S.-C. (2015). Assessment of Heavy Metal Effects on the Freshwater Microalga, Chlorella Vulgaris, by Chlorophyll Fluorescence Analysis. J. Environ. Sci. Int. 24 (12), 1591–1600. doi:10.5322/jesi.2015.24.12.1591
Osman, G. E. H., Abulreesh, H. H., Elbanna, K., Shaaban, M. R., Samreen, S., and Ahmad, I. (2019). Recent Progress in Metal-Microbe Interactions: Prospects in Bioremediation. J. Pure Appl. Microbiol. 13 (1), 13–26. doi:10.22207/jpam.13.1.02
Patnaik, R., and Padhy, R. N. (2018). Comparative Study on Toxicity of Methylmercury Chloride and Methylmercury Hydroxide to the Human Neuroblastoma Cell Line SH-Sy5y. Environ. Sci. Pollut. Res. 25 (21), 20606–20614. doi:10.1007/s11356-018-2164-2
Perryman, C. R., Wirsing, J., Bennett, K. A., Brennick, O., Perry, A. L., Williamson, N., et al. (2020). Heavy Metals in the Arctic: Distribution and Enrichment of Five Metals in Alaskan Soils. PLoS One 15 (6), e0233297. doi:10.1371/journal.pone.0233297
Polizzotto, M. L., Kocar, B. D., Benner, S. G., Sampson, M., and Fendorf, S. (2008). Near-surface Wetland Sediments as a Source of Arsenic Release to Ground Water in Asia. Nature 454 (7203), 505–508. doi:10.1038/nature07093
Popoola, L. T., Adebanjo, S. A., and Adeoye, B. K. (2017). Assessment of Atmospheric Particulate Matter and Heavy Metals: A Critical Review. Int. J. Environ. Sci. Technol. 15 (5), 935–948. doi:10.1007/s13762-017-1454-4
Pringle, T. G., and Jervis, R. E. (1987). Multi-element Correlations for Airborne Particulate Source Attribution. J. Radioanalytical Nucl. Chem. Articles 110, 321–332. doi:10.1007/BF02035524
Punshon, T., Li, Z., Jackson, B. P., Parks, W. T., Romano, M., Conway, D., et al. (2019). Placental Metal Concentrations in Relation to Placental Growth, Efficiency and Birth Weight. Environ. Int. 126, 533–542. doi:10.1016/j.envint.2019.01.063
Purkayastha, R. P., and Mitra, A. K. (1993). Metal Uptake by Mycelia during Submerged Growth and by Sporocarps of an Edible Fungus Volvariella Volvacea. Indian J. Exp. Biol. 30, 1184–1187.
Qiao, M. M., Hong-Bing, J. I., Zhu, X. F., and Chen, Y. (2013). Fraction Distribution and Risk Assessment of Heavy Metal in Sediments of Miyun Reservoir. J. Agro-Environment Sci. 33 (12), 3324–3333.
Rai, P., and Dayal, S. (2016). Evaluating Genotoxic Potential of Chromium on Pisum Sativum. Chromosome Bot. 11 (2), 44–47. doi:10.3199/iscb.11.44
Rehman, A. U., Nazir, S., Irshad, R., Tahir, K., Ur Rehman, K., Islam, R. U., et al. (2021). Toxicity of Heavy Metals in Plants and Animals and Their Uptake by Magnetic Iron Oxide Nanoparticles. J. Mol. Liq. 321, 114455. doi:10.1016/j.molliq.2020.114455
Rehman, M. Z.-u., Rizwan, M., Ghafoor, A., Naeem, A., Ali, S., Sabir, M., et al. (2015). Effect of Inorganic Amendments for In Situ Stabilization of Cadmium in Contaminated Soils and its Phyto-Availability to Wheat and Rice under Rotation. Environ. Sci. Pollut. Res. 22 (21), 16897–16906. doi:10.1007/s11356-015-4883-y
Ren, J., Chen, J., Han, L., Wang, M., Yang, B., Du, P., et al. (2018). Spatial Distribution of Heavy Metals, Salinity and Alkalinity in Soils Around Bauxite Residue Disposal Area. Sci. Total Environ. 628-629, 1200–1208. doi:10.1016/j.scitotenv.2018.02.149
Reza, R., and Singh, G. (2010). Heavy Metal Contamination and its Indexing Approach for River Water. Int. J. Environ. Sci. Technol. 7, 785–792. doi:10.1007/BF03326187
Riyazuddin, R., Nisha, N., Ejaz, B., Khan, M. I. R., Kumar, M., Ramteke, P. W., et al. (2021). A Comprehensive Review on the Heavy Metal Toxicity and Sequestration in Plants. Biomolecules 12 (1), 43. doi:10.3390/biom12010043
Ropek, A., and Para, A. (2003). The Effect of Heavy Metal Ions and Their Complexions upon Growth, Sporulation and Pathogenicity of the Entomopathogenic Fungus Paecilomyces Farinosus. Pol. J. Environ. Stud. 12 (2), 227–230.
Rout, T. K., Masto, R. E., Ram, L. C., George, J., and Padhy, P. K. (2012). Assessment of Human Health Risks from Heavy Metals in Outdoor Dust Samples in a Coal Mining Area. Environ. Geochem Health 35, 347–356. doi:10.1007/s10653-012-9499-2
Sarwar, N., Imran, M., Shaheen, M. R., Ishaque, W., Kamran, M. A., Matloob, A., et al. (2017). Phytoremediation Strategies for Soils Contaminated with Heavy Metals: Modifications and Future Perspectives. Chemosphere 171, 710–721. doi:10.1016/j.chemosphere.2016.12.116
Satarug, S., and Moore, M. R. (2004). Adverse Health Effects of Chronic Exposure to Low-Level Cadmium in Foodstuffs and Cigarette Smoke. Environ. Health Perspect. 112 (10), 1099–1103. doi:10.1289/ehp.6751
Schreck, E., Foucault, Y., Geret, F., Pradere, P., and Dumat, C. (2011). Influence of Soil Ageing on Bioavailability and Ecotoxicity of Lead Carried by Process Waste Metallic Ultrafine Particles. Chemosphere 85 (10), 1555–1562. doi:10.1016/j.chemosphere.2011.07.059
Sevillano-Morales, J. S., Cejudo-Gómez, M., Ramírez-Ojeda, A. M., Cámara Martos, F., and Moreno-Rojas, R. (2015). Risk Profile of Methylmercury in Seafood. Curr. Opin. Food Sci. 6, 53–60. doi:10.1016/j.cofs.2016.01.003
Seyfferth, A. L., Amaral, D., Limmer, M. A., and Guilherme, L. R. G. (2019). Combined Impacts of Si-Rich Rice Residues and Flooding Extent on Grain as and Cd in Rice. Environ. Int. 128, 301–309. doi:10.1016/j.envint.2019.04.060
Shahid, M., Dumat, C., Khalid, S., Niazi, N. K., and Antunes, P. M. C. (2017a). “Cadmium Bioavailability, Uptake, Toxicity and Detoxification in Soil-Plant System,” in Reviews of Environmental Contamination and Toxicology Volume 241. Editor P. de Voogt (Cham: Springer International Publishing).
Shahid, M., Dumat, C., Khalid, S., Schreck, E., Xiong, T., and Niazi, N. K. (2017b). Foliar Heavy Metal Uptake, Toxicity and Detoxification in Plants: A Comparison of Foliar and Root Metal Uptake. J. Hazard. Mater. 325, 36–58. doi:10.1016/j.jhazmat.2016.11.063
Shahid, M., Pourrut, B., Dumat, C., Nadeem, M., Aslam, M., and Pinelli, E. (2014). “Heavy-Metal-Induced Reactive Oxygen Species: Phytotoxicity and Physicochemical Changes in Plants,” in Reviews of Environmental Contamination and Toxicology Volume 232. Editor D. M. Whitacre (Cham: Springer International Publishing). doi:10.1007/978-3-319-06746-9_1
Shahid, M., Shamshad, S., Rafiq, M., Khalid, S., Bibi, I., Niazi, N. K., et al. (2017c). Chromium Speciation, Bioavailability, Uptake, Toxicity and Detoxification in Soil-Plant System: A Review. Chemosphere 178, 513–533. doi:10.1016/j.chemosphere.2017.03.074
Shanker, A., Cervantes, C., Lozatavera, H., and Avudainayagam, S. (2005). Chromium Toxicity in Plants. Environ. Int. 31 (5), 739–753. doi:10.1016/j.envint.2005.02.003
Sharma, A., Kumar, M., Chandel, M., and Kaur, S. (2012). Modulation of Chromium Trioxide Induced Genotoxicity by Methanol Extract of Leaves of <i>Trigonella Foenum-Graecum</i> L. J. Exp. Integr. Med. 2, 77. doi:10.5455/jeim.261111.or.017
Sharma, P., Kumar, A., and Bhardwaj, R. (2016). Plant Steroidal Hormone Epibrassinolide Regulate - Heavy Metal Stress Tolerance in Oryza Sativa L. By Modulating Antioxidant Defense Expression. Environ. Exp. Bot. 122, 1–9. doi:10.1016/j.envexpbot.2015.08.005
Shen, F., Liao, R., Ali, A., Mahar, A., Guo, D., Li, R., et al. (2017). Spatial Distribution and Risk Assessment of Heavy Metals in Soil Near a Pb/Zn Smelter in Feng County, China. Ecotoxicol. Environ. Saf. 139, 254–262. doi:10.1016/j.ecoenv.2017.01.044
Shi, T., Ma, J., Zhang, Y., Liu, C., Hu, Y., Gong, Y., et al. (2019). Status of Lead Accumulation in Agricultural Soils across China (1979-2016). Environ. Int. 129, 35–41. doi:10.1016/j.envint.2019.05.025
Simeonov, L. I., Kochubovski, M., and Simeonova, B. (2011). Environmental Heavy Metal Pollution and Effects on Child Mental Development. Risk Assessment and Prevention Strategies.
Singh, H. P., Mahajan, P., Kaur, S., Batish, D. R., and Kohli, R. K. (2013). Chromium Toxicity and Tolerance in Plants. Environ. Chem. Lett. 11 (3), 229–254. doi:10.1007/s10311-013-0407-5
Singh, K. P., Mohan, D., Singh, V. K., and Malik, A. (2005). Studies on Distribution and Fractionation of Heavy Metals in Gomti River Sediments-A Tributary of the Ganges, India. J. Hydrology 312 (1), 14–27. doi:10.1016/j.jhydrol.2005.01.021
Singhal, V., and Rathore, V. S. (2001). Effects of Zn2+ and Cu2+ on Growth, Lignin Degradation and Ligninolytic Enzymes in Phanerochaete Chrysosporium. World J. Microb. Biot. 17, 235–240. doi:10.1023/A:1016617025769
Sizmur, T., Palumbo-Roe, B., Watts, M. J., and Hodson, M. E. (2011). Impact of the Earthworm Lumbricus Terrestris (L.) on as, Cu, Pb and Zn Mobility and Speciation in Contaminated Soils. Environ. Pollut. 159 (3), 742–748. doi:10.1016/j.envpol.2010.11.033
Škrbić, B. D., Buljovčić, M., Jovanović, G., and Antić, I. (2018). Seasonal, Spatial Variations and Risk Assessment of Heavy Elements in Street Dust from Novi Sad, Serbia. Chemosphere 205, 452–462. doi:10.1016/j.chemosphere.2018.04.124
Sterritt, R. M., and Lester, J. N. (1980). Interactions of Heavy Metals with Bacteria. Sci. Total Environ. 14 (1), 5–17. doi:10.1016/0048-9697(80)90122-9
Steunou, A. S., Babot, M., Bourbon, M. L., Tambosi, R., Durand, A., Liotenberg, S., et al. (2020). Additive Effects of Metal Excess and Superoxide, A Highly Toxic Mixture in Bacteria. Microb. Biotechnol. 13, 1515–1529. doi:10.1111/1751-7915.13589
Stevenson, C., Hall, J. P., Harrison, E., Wood, A., and Brockhurst, M. A. (2017). Gene Mobility Promotes the Spread of Resistance in Bacterial Populations. ISME J. 11 (8), 1930–1932. doi:10.1038/ismej.2017.42
Stohs, S., and Bagchi, D. (1995). Oxidative Mechanisms in the Toxicity of Metal Ions. Free Radic. Biol. Med. 18 (2), 321–336. doi:10.1016/0891-5849(94)00159-H
Sun, F.-S., Yu, G.-H., Ning, J.-Y., Zhu, X.-D., Goodman, B. A., and Wu, J. (2020). Biological Removal of Cadmium from Biogas Residues during Vermicomposting, and the Effect of Earthworm Hydrolysates on Trichoderma Guizhouense Sporulation. Bioresour. Technol. 312, 123635. doi:10.1016/j.biortech.2020.123635
Sundaramoorthy, P., Chidambaram, A., Ganesh, K. S., Unnikannan, P., and Baskaran, L. (2010). Chromium Stress in Paddy: (I) Nutrient Status of Paddy under Chromium Stress; (Ii) Phytoremediation of Chromium by Aquatic and Terrestrial Weeds. Cr. Biol. 333 (8), 597–607. doi:10.1016/j.crvi.2010.03.002
Tahar, K., and Keltoum, B. (2011). Effects of Heavy Metals Pollution in Soil and Plant in the Industrial Area, West ALGERIA. J. Korean Chem. Soc. 55, 1018–1023. doi:10.5012/jkcs.2011.55.6.1018
Tan, Q.-G., Lu, S., Chen, R., and Peng, J. (2019). Making Acute Tests More Ecologically Relevant: Cadmium Bioaccumulation and Toxicity in an Estuarine Clam under Various Salinities Modeled in a Toxicokinetic-Toxicodynamic Framework. Environ. Sci. Technol. 53 (5), 2873–2880. doi:10.1021/acs.est.8b07095
Tan, S. Y., Praveena, S. M., Abidin, E. Z., and Cheema, M. S. (2016). A Review of Heavy Metals in Indoor Dust and its Human Health-Risk Implications. Rev. Environ. Health 31 (4), 447–456. doi:10.1515/reveh-2016-0026
Tham, L. X., Matsuhashi, S., and Kume, T. (1999). Responses of Ganoderma Lucidum to Heavy Metals. Mycoscience 40, 209–213. doi:10.1007/BF02464301
Thatoi, H., Das, S., Mishra, J., Rath, B. P., and Das, N. (2014). Bacterial Chromate Reductase, a Potential Enzyme for Bioremediation of Hexavalent Chromium: A Review. J. Environ. Manag. 146, 383–399. doi:10.1016/j.jenvman.2014.07.014
Turner, A. (2019). Heavy Metals in the Glass and Enamels of Consumer Container Bottles. Environ. Sci. Technol. 53 (14), 8398–8404. doi:10.1021/acs.est.9b01726
Uhlig, C., and Junttila, O. (2001). Airborne Heavy Metal Pollution and its Effects on Foliar Elemental Composition of Empetrum Hermaphroditum and Vaccinium Myrtillus in Sør-Varanger, Northern Norway. Environ. Pollut. 114 (3), 461–469. doi:10.1016/S0269-7491(00)00225-6
Uzu, G., Sobanska, S., Aliouane, Y., Pradere, P., and Dumat, C. (2009). Study of Lead Phytoavailability for Atmospheric Industrial Micronic and Sub-micronic Particles in Relation with Lead Speciation. Environ. Pollut. 157 (4), 1178–1185. doi:10.1016/j.envpol.2008.09.053
van Gestel, C. A. M. (2012). Soil Ecotoxicology: State of the Art and Future Directions. Zk 176, 275–296. doi:10.3897/zookeys.176.2275
Varol, M., and Şen, B. (2012). Assessment of Nutrient and Heavy Metal Contamination in Surface Water and Sediments of the Upper Tigris River, Turkey. Catena 92, 1–10. doi:10.1016/j.catena.2011.11.011
Viti, C., Marchi, E., Decorosi, F., and Giovannetti, L. (2014). Molecular Mechanisms of Cr(VI) Resistance in Bacteria and Fungi. FEMS Microbiol. Rev. 38 (4), 633–659. doi:10.1111/1574-6976.12051
Voica, D. M., Bartha, L., Banciu, H. L., and Oren, A. (2016). Heavy Metal Resistance in halophilicBacteriaandArchaea. FEMS Microbiol. Lett. 363 (14), fnw146. doi:10.1093/femsle/fnw146
Wakeman, T. P., Yang, A., Dalal, N. S., Boohaker, R. J., Zeng, Q., Ding, Q., et al. (2017). DNA Mismatch Repair Protein Mlh1 Is Required for Tetravalent Chromium Intermediate-Induced DNA Damage. Oncotarget 8, 83975–83985. doi:10.18632/oncotarget.20150
Wang, H.-T., Zhu, D., Li, G., Zheng, F., Ding, J., O’Connor, P. J., et al. (2019). Effects of Arsenic on Gut Microbiota and its Biotransformation Genes in Earthworm Metaphire Sieboldi. Environ. Sci. Technol. 53 (7), 3841–3849. doi:10.1021/acs.est.8b06695
Wang, H. X., Ng, T. B., and Ooi, V. E. C. (1998). A Ribonuclease from Sclerotia of the Edible MushroomPleurotus Tuber-Regium. Biochem. Biophysical Res. Commun. 250, 544–546. doi:10.1006/bbrc.1998.9361
Wang, R., Shafi, M., Ma, J., Zhong, B., Guo, J., Hu, X., et al. (2018). Effect of Amendments on Contaminated Soil of Multiple Heavy Metals and Accumulation of Heavy Metals in Plants. Environ. Sci. Pollut. Res. 25 (28), 28695–28704. doi:10.1007/s11356-018-2918-x
Wang, S., Jia, Y., Wang, S., Wang, X., Wang, H., Zhao, Z., et al. (2010). Fractionation of Heavy Metals in Shallow Marine Sediments from Jinzhou Bay, China. J. Environ. Sci. 22 (1), 23–31. doi:10.1016/S1001-0742(09)60070-X
Wang, X.-F., Xing, M.-L., Shen, Y., Zhu, X., and Xu, L.-H. (2006). Oral Administration of Cr(VI) Induced Oxidative Stress, DNA Damage and Apoptotic Cell Death in Mice. Toxicology 228 (1), 16–23. doi:10.1016/j.tox.2006.08.005
Wang, Y., Yang, L., Kong, L., Liu, E., Wang, L., and Zhu, J. (2015). Spatial Distribution, Ecological Risk Assessment and Source Identification for Heavy Metals in Surface Sediments from Dongping Lake, Shandong, East China. Catena 125, 200–205. doi:10.1016/j.catena.2014.10.023
Ward, C. S., Pan, J.-F., Colman, B. P., Wang, Z., Gwin, C. A., Williams, T. C., et al. (2019). Conserved Microbial Toxicity Responses for Acute and Chronic Silver Nanoparticle Treatments in Wetland Mesocosms. Environ. Sci. Technol. 53 (6), 3268–3276. doi:10.1021/acs.est.8b06654
Wenke, C., Enli, H., and Yumei, Z. (2013). Measures of Source Controlling of Heavy Metals in Sewage Sludge from Municipal Wastewater Treatment Plants. Environmental Engineering.
Wheaton, G. H., Mukherjee, A., and Kelly, R. M. (2016). Transcriptomes of the Extremely Thermoacidophilic Archaeon Metallosphaera Sedula Exposed to Metal "Shock" Reveal Generic and Specific Metal Responses. Appl. Environ. Microbiol. 82 (15), 4613–4627. doi:10.1128/AEM.01176-16
Wise, J. P., Wise, , LaCerte, C., Wise, J. P., Aboueissa, A.-M., and Aboueissa, A. M. (2011). The Genotoxicity of Particulate and Soluble Chromate in Sperm Whale (Physeter Macrocephalus) Skin Fibroblasts. Environ. Mol. Mutagen. 52 (1), 43–49. doi:10.1002/em.20579
Wu, M., Yan, C., Xu, J., Wu, W., Li, H., and Zhou, X. (2013). Umbilical Cord Blood Mercury Levels in China. J. Environ. Sci. 25 (2), 386–392. doi:10.1016/S1001-0742(12)60061-8
Wuana, R. A., and Okieimen, F. E. (2011). Heavy Metals in Contaminated Soils: A Review of Sources, Chemistry, Risks and Best Available Strategies for Remediation. ISRN Ecol. 2011, 1–20. doi:10.5402/2011/402647
Xia, P., Ma, L., Yi, Y., and Lin, T. (2021). Assessment of Heavy Metal Pollution and Exposure Risk for Migratory Birds- A Case Study of Caohai Wetland in Guizhou Plateau (China). Environ. Pollut. 275, 116564. doi:10.1016/j.envpol.2021.116564
Xia, X., Yang, Z., Cui, Y., Li, Y., Hou, Q., and Yu, T. (2014). Soil Heavy Metal Concentrations and Their Typical Input and Output Fluxes on the Southern Song-Nen Plain, Heilongjiang Province, China. J. Geochem. Explor. 139, 85–96. doi:10.1016/j.gexplo.2013.06.008
Xie, S., Wang, L., Xu, Y., Lin, D., Sun, Y., and Zheng, S. (2020). Performance and Mechanisms of Immobilization Remediation for Cd Contaminated Water and Soil by Hydroxy Ferric Combined Acid-Base Modified Sepiolite (HyFe/ABsep). Sci. Total Environ. 740, 140009. doi:10.1016/j.scitotenv.2020.140009
Xu, Y., Seshadri, B., Bolan, N., Sarkar, B., Ok, Y. S., Zhang, W., et al. (2019). Microbial Functional Diversity and Carbon Use Feedback in Soils as Affected by Heavy Metals. Environ. Int. 125, 478–488. doi:10.1016/j.envint.2019.01.071
Xu, Y., Wang, L., Zhu, J., Jiang, P., Zhang, Z., Li, L., et al. (2021). Chromium Induced Neurotoxicity by Altering Metabolism in Zebrafish Larvae. Ecotoxicol. Environ. Saf. 228, 112983. doi:10.1016/j.ecoenv.2021.112983
Yamaguchi, N., Nakamura, T., Dong, D., Takahashi, Y., Amachi, S., and Makino, T. (2011). Arsenic Release from Flooded Paddy Soils Is Influenced by Speciation, Eh, pH, and Iron Dissolution. Chemosphere 83 (7), 925–932. doi:10.1016/j.chemosphere.2011.02.044
Yan, G., Chen, X., Du, S., Deng, Z., Wang, L., and Chen, S. (2019). Genetic Mechanisms of Arsenic Detoxification and Metabolism in Bacteria. Curr. Genet. 65 (2), 329–338. doi:10.1007/s00294-018-0894-9
Yang, F., Xie, S., Wei, C., Liu, J., Zhang, H., Chen, T., et al. (2018). Arsenic Characteristics in the Terrestrial Environment in the Vicinity of the Shimen Realgar Mine, China. Sci. Total Environ. 626, 77–86. doi:10.1016/j.scitotenv.2018.01.079
Yang, S., Zhou, D., Yu, H., Wei, R., and Pan, B. (2013). Distribution and Speciation of Metals (Cu, Zn, Cd, and Pb) in Agricultural and Non-agricultural Soils Near a Stream Upriver from the Pearl River, China. Environ. Pollut. 177, 64–70. doi:10.1016/j.envpol.2013.01.044
Yao, J., Cheng, Y., Zhou, M., Zhao, S., Lin, S., Wang, X., et al. (2018). ROS Scavenging Mn3O4nanozymes Forin Vivoanti-Inflammation. Chem. Sci. 9 (11), 2927–2933. doi:10.1039/c7sc05476a
Yao, Y., Hu, L., Li, S., Zeng, Q., Zhong, H., and He, Z. (2020). Exploration on the Bioreduction Mechanisms of Cr(VI) and Hg(II) by a Newly Isolated Bacterial Strain Pseudomonas Umsongensis CY-1. Ecotoxicol. Environ. Saf. 201, 110850. doi:10.1016/j.ecoenv.2020.110850
Ye, L., Wang, L., and Jing, C. (2020). Biotransformation of Adsorbed Arsenic on Iron Minerals by Coexisting Arsenate-Reducing and Arsenite-Oxidizing Bacteria. Environ. Pollut. 256, 113471. doi:10.1016/j.envpol.2019.113471
Ye, L., Zhong, B., Huang, M., Chen, W., and Wang, X. (2021). Pollution Evaluation and Children's Multimedia Exposure of Atmospheric Arsenic Deposition in the Pearl River Delta, China. Sci. Total Environ. 787, 147629. doi:10.1016/j.scitotenv.2021.147629
Yu, R., Hu, G., Yang, Q., He, H., and Lin, C. (2016). Identification of Pb Sources Using Pb Isotopic Compositions in the Core Sediments from Western Xiamen Bay, China. Mar. Pollut. Bull. 113 (1-2), 247–252. doi:10.1016/j.marpolbul.2016.09.027
Yuan, A. T., Korkola, N. C., Wong, D. L., and Stillman, M. J. (2020). Metallothionein Cd4S11 Cluster Formation Dominates in the Protection of Carbonic Anhydrase. Metallomics 12, 767–783. doi:10.1039/D0MT00023J
Zahra, A., Hashmi, M. Z., Malik, R. N., and Ahmed, Z. (2014). Enrichment and Geo-Accumulation of Heavy Metals and Risk Assessment of Sediments of the Kurang Nallah-Feeding Tributary of the Rawal Lake Reservoir, Pakistan. Sci. Total Environ. 470-471, 925–933. doi:10.1016/j.scitotenv.2013.10.017
Zeid, I. M. (2001). Responses of Phaseolus vulgaris Chromium and Cobalt Treatments. Biol. plant. 44 (1), 111–115. doi:10.1023/A:1017934708402
Zeng, F., Zhou, W., Qiu, B., Ali, S., Wu, F., and Zhang, G. (2011). Subcellular Distribution and Chemical Forms of Chromium in Rice Plants Suffering from Different Levels of Chromium Toxicity. Z. Pflanzenernähr. Bodenk. 174 (2), 249–256. doi:10.1002/jpln.200900309
Zeng, W., Li, F., Wu, C., Yu, R., Wu, X., Shen, L., et al. (2020). Role of Extracellular Polymeric Substance (EPS) in Toxicity Response of Soil Bacteria Bacillus Sp. S3 to Multiple Heavy Metals. Bioprocess Biosyst. Eng. 43 (1), 153–167. doi:10.1007/s00449-019-02213-7
Zhang, F., Ou, X., Chen, S., Ran, C., and Quan, X. (2012). Competitive Adsorption and Desorption of Copper and Lead in Some Soil of North China. Front. Environ. Sci. Eng. 6, 484–492. doi:10.1007/s11783-012-0423-x
Zhang, G., Bai, J., Xiao, R., Zhao, Q., Jia, J., Cui, B., et al. (2017). Heavy Metal Fractions and Ecological Risk Assessment in Sediments from Urban, Rural and Reclamation-Affected Rivers of the Pearl River Estuary, China. Chemosphere 184, 278–288. doi:10.1016/j.chemosphere.2017.05.155
Zhang, H., Yang, B., Zhang, G., and Zhang, X. (2016a). Sewage Sludge as Barrier Material for Heavy Metals in Waste Landfill. Arch. Environ. Prot. 42 (2), 52–58. doi:10.1515/aep-2016-0020
Zhang, L., Lin, X., Wang, J., Jiang, F., Wei, L., Chen, G., et al. (2016b). Effects of Lead and Mercury on Sulfate-Reducing Bacterial Activity in a Biological Process for Flue Gas Desulfurization Wastewater Treatment. Sci. Rep. 6, 30455. doi:10.1038/srep30455
Zhang, L., Zhu, G., Ge, X., Xu, G., and Guan, Y. (2018). Novel Insights into Heavy Metal Pollution of Farmland Based on Reactive Heavy Metals (RHMs): Pollution Characteristics, Predictive Models, and Quantitative Source Apportionment. J. Hazard. Mater. 360, 32–42. doi:10.1016/j.jhazmat.2018.07.075
Zhang, Y., Hou, D., O’Connor, D., Shen, Z., Shi, P., Ok, Y. S., et al. (2019). Lead Contamination in Chinese Surface Soils: Source Identification, Spatial-Temporal Distribution and Associated Health Risks. Crit. Rev. Environ. Sci. Technol. 49 (15), 1386–1423. doi:10.1080/10643389.2019.1571354
Zhang, Y., Wu, D., Wang, C., Fu, X., and Wu, G. (2020). Impact of Coal Power Generation on the Characteristics and Risk of Heavy Metal Pollution in Nearby Soil. Ecosyst. Health Sustain. 6 (1), 1787092. doi:10.1080/20964129.2020.1787092
Zhao, L., Chen, H., Lu, X., Lin, H., Christensen, G. A., Pierce, E. M., et al. (2017). Contrasting Effects of Dissolved Organic Matter on Mercury Methylation by Geobacter Sulfurreducens PCA and Desulfovibrio Desulfuricans ND132. Environ. Sci. Technol. 51 (18), 10468–10475. doi:10.1021/acs.est.7b02518
Zhao, L., Hu, G., Yan, Y., Yu, R., Cui, J., Wang, X., et al. (2019). Source Apportionment of Heavy Metals in Urban Road Dust in a Continental City of Eastern China: Using Pb and Sr Isotopes Combined with Multivariate Statistical Analysis. Atmos. Environ. 201, 201–211. doi:10.1016/j.atmosenv.2018.12.050
Zhou, F., Xie, J., Zhang, S., Yin, G., Gao, Y., Zhang, Y., et al. (2018). Lead, Cadmium, Arsenic, and Mercury Combined Exposure Disrupted Synaptic Homeostasis through Activating the Snk-SPAR Pathway. Ecotoxicol. Environ. Saf. 163, 674–684. doi:10.1016/j.ecoenv.2018.07.116
Zhou, F., Yin, G., Gao, Y., Liu, D., Xie, J., Ouyang, L., et al. (2019). Toxicity Assessment Due to Prenatal and Lactational Exposure to Lead, Cadmium and Mercury Mixtures. Environ. Int. 133 (Pt B), 105192. doi:10.1016/j.envint.2019.105192
Zhu, C., Tian, H., Cheng, K., Liu, K., Wang, K., Hua, S., et al. (2016). Potentials of Whole Process Control of Heavy Metals Emissions from Coal-Fired Power Plants in China. J. Clean. Prod. 114, 343–351. doi:10.1016/j.jclepro.2015.05.008
Zou, J., Wang, M., Jiang, W., and Liu, D. (2006). Effects of Hexavalent Chromium(VI) on Root Growth and Cell Division in Root Tip Cells of Amaranthus Viridis L. Pak. J. Bot. 38.
Keywords: heavy metal(loid)s, biological toxicity, archaea and bacteria, fungi, animals, plants, human
Citation: Ding C, Chen J, Zhu F, Chai L, Lin Z, Zhang K and Shi Y (2022) Biological Toxicity of Heavy Metal(loid)s in Natural Environments: From Microbes to Humans. Front. Environ. Sci. 10:920957. doi: 10.3389/fenvs.2022.920957
Received: 15 April 2022; Accepted: 03 May 2022;
Published: 26 May 2022.
Edited by:
Wai Chin LI, The Education University of Hong Kong, Hong Kong, SAR ChinaReviewed by:
Long Zou, Jiangxi Normal University, ChinaLiyuan Ma, China University of Geosciences Wuhan, China
Pu Jia, South China Normal University, China
Copyright © 2022 Ding, Chen, Zhu, Chai, Lin, Zhang and Shi. This is an open-access article distributed under the terms of the Creative Commons Attribution License (CC BY). The use, distribution or reproduction in other forums is permitted, provided the original author(s) and the copyright owner(s) are credited and that the original publication in this journal is cited, in accordance with accepted academic practice. No use, distribution or reproduction is permitted which does not comply with these terms.
*Correspondence: Kejing Zhang, MzQyOTUyMDQzQHFxLmNvbQ==; Yan Shi, c2hpeXp5cnNAY3N1LmVkdS5jbg==