- 1Beijing Key Laboratory of Resource-oriented Treatment of Industrial Pollutants, School of Energy and Environmental Engineering, University of Science and Technology Beijing, Beijing, China
- 2Department of Environmental Science and Management, School of Environmental Science and Technology, Ardhi University, Dares Salaam, Tanzania
- 3Natural Resource and Environmental Studies, University of Northern BC, Vancouver, BC, Canada
- 4Environmental Education Unit, Faculty of Education, University of Botswana, Gaborone, Botswana
With the increasing demand for renewable energy and environmental protection, biogas technology has attracted considerable attention around the world. Fecal sludge (FS) is rich in organic matter, and it contains high concentrations of excreted pathogens that cause gastro-intestinal infection. In Tanzania, fecal sludge management from on-site sanitation systems poses a threat on environmental safety. This study aimed to assess the feasibility of the use of anaerobic digestion (AD) for the treatment of FS and the production of biogas as renewable energy to achieve multiple benefits in Tanzania. For the experiments, FS and food waste (FW) were used as feedstock, and rice straw-derived biochar (RSB) was added as an additive to improve biogas production. The mesophilic anaerobic digestion resulted in a methane yield of 287.5 ml/g VS for FS + FW co-digestion and 396 ml/g VS for FS + FW + RSB co-digestion. At ambient temperature (20–26°C), the system produced a methane yield of 234 ml/g VS for FS + FW co-digestion and 275 ml/g VS for FS + FW + RSB co-digestion. Three different scenarios (digester with volumes of 4, 100, and 400 m3, respectively) and strategies for FS treatment by AD in Tanzania were proposed and analyzed. These treatments can produce methane volumes of 1.95, 49.5, and 199.5 m3 with pay-back periods of 3, 5, and 15 years and net present values of + 28, +1,337, and +52,351 USD, respectively. The calculations also showed that the heat value from the produced biogas and energy needed to heat the digester at 26–37°C resulted in energy balance values of + 0.012, + 0.53, and + 2.22 GJ/day for the 4, 100, and 400 m3 digester volumes, respectively.
1 Introduction
Fecal sludge (FS), which is known as excreta, is the rejected waste from humans. Globally, innovative treatment systems are needed for safe fecal sludge management (FSM) (Bassan, 2014). In rural Tanzania, 83% of the population do not have access to basic sanitation. Communities rely on on-site sanitation such as septic tanks and pit latrines that are not safely managed and allow for fecal sludge to contaminate groundwater that is used for drinking (Mrimi et al., 2020). Approximately 90% of the Dar es Salaam inhabitants use on-site sanitation system facilities, where 60% of the inhabitants use pit latrines and 30% use septic tanks (Makoye, 2017). In comparison with the traditional disposal method such as landfilling, drying beds, and composting, anaerobic digestion (AD) of FS for biogas production is an effective treatment solution. Although AD is considered an alternative fuel production option for bioenergy production (Cheng et al., 2020), the use of FS as a substrate has shown some challenges, such as poor process stability and low methane productivity.
Anaerobic co-digestion (ACoD) is commonly used for enhancing biogas production during AD (Dhungana et al., 2022). ACoD is the process of feeding two or more kinds of organic substrates during AD. It offers a wide range of benefits compared with mono-digestion of FS, such as dilution of toxic substances, balancing of the C:N ratio, enhanced methane yield, the synergic effect of microorganisms, and nutrient balance (Hagos et al., 2017). Biogas production from co-mixed substrates has been widely studied (Yong et al., 2015). Nevertheless, the selection of appropriate co-substrates, their characterization, composition, and mixing ratio should be wisely considered.
In addition, additive materials (e.g., polyethylene, carbon, activated carbon, and polyvinyl alcohol) and conductive materials (e.g., conductive iron oxides, semi-conductive iron oxide minerals, and micrometer-sized magnetite) are used for immobilizing microorganisms and tackling inhibitions (Cai et al., 2016). However, the use of these additive media has shown some environmental and economic problems because they persist in anaerobic digestate and are mainly used as fertilizer or require some addition system for their separation from the digestate (Haider et al., 2015; Cai et al., 2016). Accordingly, environment-friendly and economic additives should be developed. Biochar, which has good adsorptive property, is an important additive for the AD process (Fagbohungbe et al., 2016; Pan et al., 2019). Recently, in Tanzania, it has been discussed on the applications of biochar in soil improvement, waste management, energy generation, and as an additive substance in AD (Hewage and Priyadarshani, 2016; Simeon, 2017). However, limited studies have used locally produced biochar as an additive in the AD process for improving the AD process of FS. Limited studies have also focused on the use of biochar in terms of biochar dose and its particle size that can be added in AD of organic matters to improve its efficiency. Therefore, this area needs to be further studied.
Millions of rural household biogas digesters that operate in developing countries in Asia and Africa are working under ambient conditions and are primarily unheated. Therefore, they experience maximum fluctuation of temperature, thus reducing biogas production (Khan and Martin, 2016; Lohani et al., 2022). A digester’s operating temperature is recommended to be in the range of 33–37 or 45–55°C during the anaerobic start-up of the digester (Arikan et al., 2015). Failure to maintain the temperature within this range will inhibit methanogenic growth, increase the start-up time, and reduce biogas production (Dev et al., 2019). In most studies, acclimated seed (inoculum), the chemical and thermal pretreatments of feeding the substrate, and the mesophilic temperature are maintained to speed up the start-up process along with the overall AD process (Martí-Herrero et al., 2015). In retrospect, in most developing nations, the anaerobic digester is operated in ambient conditions without using the acclimated inoculum before actual feeding of substrates. Hence, many complexities arise, such as prolonged reactor start-up time and poor methane yield, which have been major challenges for domestic biogas plants in developing nations. Thus, an effective strategy for FS treatment in developing nations should be studied.
This study mainly aimed to determine whether the AD technology can be used as an FS treatment option in Tanzania, which is located in east Africa. The specific objectives were as follows: 1) to determine the specific biogas production of FS under the temperature-controlled mesophilic condition without or with additives; 2) to determine the specific biogas production of FS at ambient temperature without or with additives; 3) to calculate the energy balances of different digester scales; 4) to analyze the cost-benefit of three different application scenarios in Tanzania; and 5) to propose the strategy for FS treatment in three application scenarios.
2 Materials and method
2.1 Samples
2.1.1 Fecal sludge
The experiments were implemented in both China and Tanzania. FS was obtained from a vacuum toilet at the University of Science and Technology of Beijing (USTB) in China and from dormitory septic tanks at Ardhi University in Tanzania. After collecting FS from septic tanks by using a plastic bucket, the sample was filtered to remove large particles and thus avoiding blocking of the digester inlet and outlet pipes.
2.1.2 Food waste
Food waste (FW) was collected from a restaurant USTB in China and a student canteen in Ardhi University in Tanzania. Considering that FW is mainly composed of vegetables, meat, fish, and bone materials, the samples were pre-treated manually before grinding to remove impurities such as tissue paper, plastic spoons, and straws. For the pre-treatment stage, FW was sorted and crushed into aqueous slurry. The FW sample was stored in a refrigerator at 4°C until use to avoid early decay.
2.1.3 Inoculum
In China, inoculated sludge was obtained from a pilot system fed with waste-activated sludge. The pilot-scale reactor is located in the wastewater treatment plant in Chaoyang District, Beijing China. In Tanzania, FS was used as the inoculum.
2.1.4 Rice straw-derived biochar
The rice straw-derived biochar (RSB) obtained from Henanlize Bioenergy Technology Development Company from Henan province in China was used during the experiment. The sample was oven-dried at 105°C for 24 h for physiochemical analysis. The volatile solid (VS) matter was determined as the weight loss after heating in a covered crucible at 550°C for 3 h by using the furnace. The RSB was characterized with a mean value of pH, total solid (TS), and VS. The biochar was sieved to obtain different particle sizes of 0.075, 0.15, and 0.45 mm by using a sieving machine. The characteristic of FS, FW, RSB, and the inoculum are shown in Table 1.
2.2 Experimental setup design
For the experimental run, two set-up ambient and mesophilic temperatures were used. Under the mesophilic condition at 37°C, six identical reactors made with the glass bottles having a total volume of 500 ml, including a headspace of 100 and 400 ml working volume (effective volume), were labeled as R1, R2, R3, R4, R5, and R6 and treated as the control for the co-digestion of FS and FW, while those labeled as M1, M2, M3, M4, M5, and M6 were used for co-digestion of FS, FW, and RSB at USTB China. At ambient temperature (20–26°C), nine reactors having a total volume of 1,000 ml with a working volume of 900 ml labeled as S1, S2, S3, S4, S5, S6, S7, S8, and S9 were used for the co-digestion of FS, FW, and RSB with different biochar particle sizes of 0.075, 0.45, and 1 mm. The reactors named as A1, A2, A3, and A4 were used for the co-digestion of FS and FW experiments in various ratios on a TS basis at Ardhi University, Tanzania. The reactors (R6, M6, and A5) were fed with inoculum only and were treated as a control, where the observed biogas production (volumetric test) of the experimental group subtracted the biogas production of the blank control group to obtain the net biogas production of the substrate. The reactors were operated at 37°C at USTB in China and under ambient temperature (20–26°C) at Ardhi University in Tanzania (Supplementary Figure S1 in Supporting information). The retention time was approximately 30 days.
The biochemical methane potential (BMP) reactors were shaken manually twice a day. The production of biogas and methane was measured daily, and samples were obtained regularly to determine the physical and chemical indicators such as pH and total ammonia nitrogen (TAN). Cumulative methane volume production was calculated based on the sum of the daily methane volume, as indicated in the following equation (Ripoll et al., 2020):
where Vt CH4 is the net volume of methane, Vi CH4 is the experimental volume of methane measured when the co-substrate was used, and Vcontrol is the volume of methane produced in the control (inoculum) experiment. Methane productivity (YCH4) at the base of the initial VS of the substrate used was calculated as Vt CH4 per g of initial VS (ml CH4/g VS).
2.3 Analytical method
The collected samples were brought to USTB and Ardhi University laboratories for physical and chemical analyses. TS and VS were determined according to the standard (APHA, 2017), as described by Nandi et al. (2020). The pH of the samples was measured using a Hach pH meter (HQ 30 days). The daily biogas composition (CH4, CO2, and H2S) was measured at an interval of time until 30 days by using a biogas analyzer (Geotechnical Producer Ltd., Gloucester, United Kingdom) at USTB. The water displacement method was used for biogas production measurement at Ardhi University, Tanzania, and the biogas volume was measured using a 200-ml plastic syringe. The concentrations of the TAN and chemical oxygen demand (COD) were determined using the kits and the Hach DR 2800 portable spectrophotometer, respectively (APHA, 2017).
3 Results and discussion
3.1 Biomethane potential test at the mesophilic condition in China
The different substrate mixing ratios and biochar doses for the BMP test by using mesophilic temperature at USTB are shown in Supplementary Tables S1, S2.
3.1.1 Co-digestion of FS and food waste
The cumulative value of methane production is shown in Figure 1. The mixing ratio of 75:25 (FW: FS) in R4 produced the maximum cumulative methane. The methane yields were 59, 205, 247.6, 287.5, and 199 ml/g VS in R1, R2, R3, R4, and R5, respectively. The value of the methane yield of ACoD in this study was 1.5–4.9 times larger than FS and FW mono-digestion. The findings are lower than the methane production from the previous study (410–680 ml/g VS) (Zhang et al., 2019) because of the difference in raw materials and reaction conditions. The result is also comparable with the findings of Minale and Worku (2014), in which high cumulative biogas was obtained when the easily biodegradable organic component in the sample was higher. Similar results were also obtained by Minale and Worku (2014), in which the maximum overall biogas yield was obtained by the co-digestion of potato processing wastewater and pig slurry compared with individual potato wastewater or pig slurry digestion. Afifah and Priadi (2017) found high values of methane yield at FS and FW ratios of 3:1 and 1:1 with concentrations of 300 and 560 ml/g VS. Therefore, although the study was conducted at the mesophilic condition by using a hand stirrer, the reactors can produce methane yields that were as high as previous studies with the optimum temperature.
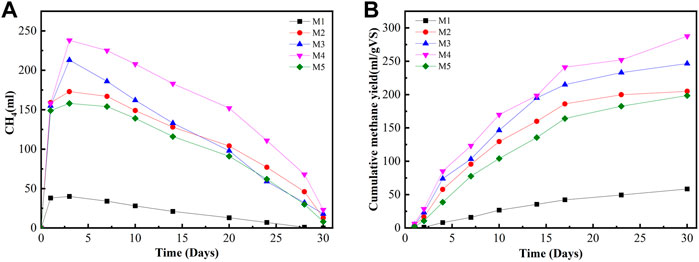
FIGURE 1. Daily methane production and cumulative methane production at different mixing ratios under mesophilic conditions. (A) Daily methane production. (B) Cumulative methane.
3.1.2 Removal of TS, VS, and COD at different mixing ratios
The characteristics of the effluent were analyzed after 30 days of digestion, and the removal efficiency was calculated. The TS of the effluent in different mixing ratios was reduced to 26.9%–66.7%. VS reduction ranged from 49.7% to 72.3%. A high TS reduction value was recorded at 100:0 (FW: FS). The high VS reduction and removal efficiency were achieved at 75:25. The higher removal efficiency of VS than the TS was a very good indication of a high uptake rate of the organic fraction of TS by methanogenic bacteria (Minale and Worku, 2014). COD reduction ranged from 41.7% to 66.9%, as shown in Table 2. Similar results were obtained by Afifah and Priadi (2017), in which the VS removal efficiency reached 92.43% at the ratio of 1:1 (FS: FW), while 79.43% at the ratio of 1:3 (FS: FW). This value was almost the same as the COD removal efficiency, which reached 87.55% at a ratio of 1:1 (FS: FW) and 72.42% at a ratio of 1:3(FS: FW).
3.1.3 Co-digestion of FS, food waste, and rice straw-derived biochar
The study also determined the AD process based on different biochar doses in digesters. The daily biogas production rate and methane contents are shown in Figure 2. It shows that the production rates vary according to different amounts of biochar addition. All biochar-supplemented groups had high cumulative methane yields and short lag phases. The cumulative methane yields at biochar doses of 25, 20, 15, 10, and 5 g were 291, 328, 396, 293, and 226 ml/g VS, which were 232, 123, 149, 5, and 27 ml/g VS more than the methane yield of reactors without biochar, respectively. In addition, cumulative methane yield had improved with the corresponding increment in biochar addition. This finding was obtained because biochar supports the rapid development of biofilms with balanced acidogenic and methanogenic bacteria through the enrichment of bacteria and methanogens, thereby enhancing their synergy and activity (Cooney et al., 2016; Soler-Cabezas et al., 2018), thus contributing to the increase of cumulative methane yields in the digesters with biochar. The difference among the five groups in terms of methane yield became increasingly large from day 5 with a prolonged digestion period. From the 10th day to the 28th day, the cumulative methane yields (CMYs) from digesters M2 (20 g biochar) and M3 (15 g biochar) were significantly higher than those of digesters M1 (25 g), M4 (10 g biochar), and M5 (5 g biochar). The maximum methane yield produced was 396 ml/g VS in reactor M3 (15 g biochar addition), as shown in Figure 2. The biochar effect in terms of higher methane yield in the present study was comparable with the results in the previous research (Vanegas and Bartlett, 2013). Additionally, Shanmugam and Horan, 2009 reported that 16.6 g/L biochar input could be optimal among different biochar loadings in the range of 8.3–33.3 g/L in a two-phase batch AD digester, and biochar dosages greater than 16.6 g/L resulted in lower cumulative methane production. CMY decreased at a higher biochar dosage, possibly because excessive biochar could not further provide enhancing effects for microbial communities in a fixed reactor space but can adsorb more methane-rich biogas because of the biochar’s high adsorption capacity (Browne et al., 2015). Biochar addition enhanced biogas production, possibly because of the ability of the biochar to adsorb ammonium ions and promote electron transfer between itself and other substrates. Sinervo (2017) reported that different types of biochar have variable ammonium ion adsorption values depending on biochar types and concentrations while using zeolite to obtain reference results.
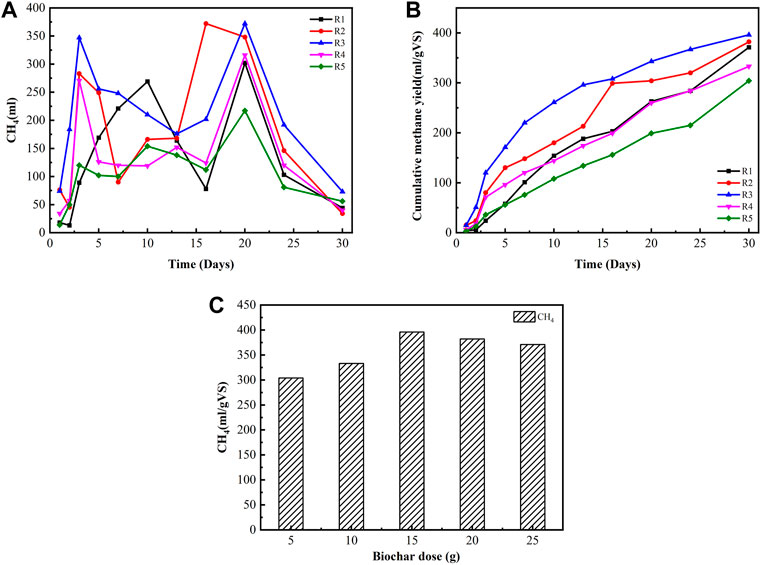
FIGURE 2. Daily methane production, cumulative methane yield, and specific methane yield production with different biochar doses under mesophilic temperature. (A) Daily methane production. (B) Cumulative methane yield. (C) Specific methane yield at different biochar doses.
3.1.4 TS, VS., chemical oxygen demand removal, and pH change at different biochar doses
The COD removal efficiency is a critical parameter, which reflects the efficiency of AD. The effluent sample was obtained from different reactors and analyzed after 30 days of the BMP test, and the removal efficiency was calculated. For mesophilic temperature, the TS removal efficiency was in the range of 69.4%–76.2%, the VS removal efficiency was 76.4%–80.5%, and the COD removal efficiency was 75.2%–78.9%, as shown in Table 3. The COD removal efficiencies were only 78.4%, 78.9%, 77.3%, 76.2%, and 75.2% at biochar doses of 25, 20, 15, 10, and 5 g, respectively. A low COD removal efficiency was observed in the reactors because excessive molasses loading may reduce the digester’s ability to decompose COD because of insufficient biochar, thereby inhibiting the activity of microorganisms that can degrade the organic matter (Cooney et al., 2016; Soler-Cabezas et al., 2018). The pH in the mesophilic temperature was higher and stable than that in the ambient temperature. Nasir et al. (2012) reported a stable and neutral pH at higher temperatures (30 and 35°C) than at lower temperatures (20 and 25°C), thus increasing the CH4 concentration. During the experiment, the methane concentration reached 72.3%, in which the pH value was stable near the neutral value (∼7.0) under the mesophilic condition.
3.2 Biomethane potential test at ambient conditions in Tanzania
The different substrate mixing ratios for the BMP test under the ambient temperature at Ardhi University, Tanzania, are presented in Supplementary Tables S3, S4.
3.2.1 Co-digestion of FS and food waste
The daily biogas production from four reactors labeled A1, A2, A3, and A4 over a period of 30 days is shown in Figure 3. The experimental results show that biogas production was slow at the beginning and after the observation of the experiment. Biogas production was generally slow within the first few days of the experiment because of the lag phase of the microbial growth, where methanogens (microbial community) become established to the medium within the digester (Simeon, 2017). The maximum values of daily biogas production were recorded on the 10th–14th before a gradual fall in the production rate was recorded for the rest of the study period. The daily biogas production decreased at the end of the experiment, possibly because of the pH drop, resulting in an increase in the concentration of ammonia nitrogen that could inhibit the process.
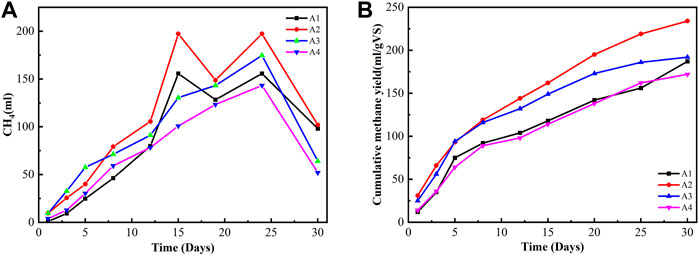
FIGURE 3. Daily methane production and cumulative methane yield in different mixing ratios under ambient temperature. (A) Daily methane production. (B) Cumulative methane yield.
The cumulative methane yields in reactors A1, A2, A3, and A4 were 187, 234, 192, and 173 ml/g VS. The substrate mixing of 1:2 (FW:FS) had high cumulative methane production. The highest total methane yield was 234 ml/g VS. The BMP results suggest that a higher proportion of FS is beneficial for methane production from a substrate mixture, possibly because of its highly biodegradable and nutritionally balanced organic matter.
3.2.2 Co-digestion of FS, food waste, and rice straw-derived biochar
Figure 4 presents the daily methane production and cumulative methane yield in different biochar doses under ambient temperature. Three different types of rice RSB doses of 20, 15, and 10 g were used in this study with different particle sizes of 0.075, 0.15, and 0.45 mm. Biogas yield increased to 275 ml/g VS when the particle size of biochar was reduced to 0.075 mm. This amount is equivalent to a 19.3% increment compared with 222 ml/gVS when the biochar particle size was 0.45 mm. Notably, the digester with 0.45 mm biochar particle size showed the lowest average methane yield among all the biochar-amended digesters, possibly because of the floating of the large particle size. He et al. (2018) found that different biochar particle sizes could exhibit significant differences in some physicochemical properties, such as elemental composition, surface functional groups, microcrystalline structure, and pore size distribution. The addition of biochar with different particle sizes could effectively tolerate high substrate loading rates and avoid the excessive accumulation of organic acids and potential digester failure. The average maximum methane contents during the 30 days of AD operation were 50.1%, 48.4%, and 45.9% for 0.075, 0.15, and 0.45 mm, respectively. The digester pH was a key indicator of the process stability for the AD operations (Mehariya et al., 2018). The variation performance of these digesters could be ascribed to the similar physicochemical properties, such as density, surface area, and pore size of the supplemented biochar with different particle sizes.
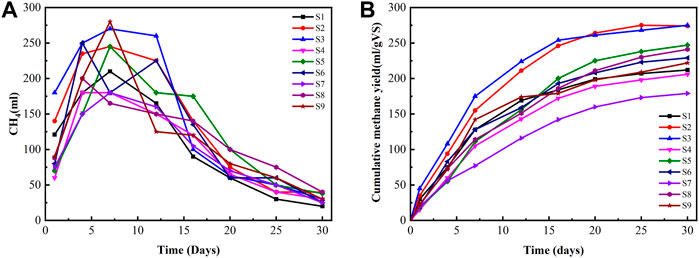
FIGURE 4. Daily methane production and cumulative methane yield in different biochar doses under ambient temperature. (A) Daily methane production. (B) Cumulative methane yield.
3.2.3 Removal efficiency of TS, VS, chemical oxygen demand, and pH fluctuation
pH and COD are two key indicators of the process stability for the AD operations (Mehariya et al., 2018). Hence, pH and COD values were examined during the AD process to understand the changing tendency of methane yields in different digesters.
The TS of the effluent in different mixing ratios was reduced to 50.9%–59.2%. VS reduction was also in the range of 50.1%–65.5%. COD reduction ranged from 59.3% to 69.6%. The maximum COD reduction was achieved in the S1, S2, and S3 digesters at a biochar size of 0.075 mm with a biochar addition dose of 10 g, in which the maximum amount of gas was produced. Recent experimental works has proven that substrates with a high COD value resulted in good performance in terms of biogas production and COD reduction (Filer et al., 2019; Bakraoui et al., 2020). The methane concentration also depends on pH. The biogas composition shifts more toward CH4 when the pH is high because of the increased alkalinity caused by the NH3 release. Nasir et al. (2012) reported a stable and neutral pH at higher temperatures (30 and 35°C) than at lower temperatures (20 and 25°C), resulting in a high CH4 concentration. Sabbir et al. (2022) reported that the average methane concentrations were 61.43%, 59.75%, and 56.3% in the autumn, late autumn, and winter, respectively, where the pH in the autumn was higher and stable than that in late autumn and winter. The removal efficiencies for TS, VS, and COD and the pH fluctuation are shown in Table 4.
3.3 Lesson learnt for biogas technology in China
The Chinese biogas industry deviates from the usual development path compared with developing countries. Tanzania can learn numerous lessons, which should be reconsidered.
3.3.1 National subsidy
The development of China’s biogas industry is successful with the aid of the government subsidy. At present, the central government policy aims to establish and subsidize thousands of bio-natural gas plant installations in rural areas to promote biogas production. The Ministry of Agriculture aims for a daily bio-methane generation of >10,000 m3 and a total digester volume of >16,000 m3. Accordingly, it provides 2,500 CNY (375 USD) subsidy per cubic meter of the methane generation capability. This subsidy is 40% of the total financial aid and is equivalent to 40 million CNY (6,000,000 USD) in subsidy by the central government of China (Zheng et al., 2020). Small-scale digesters are subsidized with 1,500 CNY (225 USD) per cubic meter of the fermentation volume for biogas power generation, including heating and cooking (domestic consumption). This value represents 35% of the total investment and is equivalent to a subsidy of 30 million (4,500,000 USD) CNY from the federal government. Approximately 386 small-scale biogas projects and 25 engineering biogas projects were subsidized in 2015, and these numbers increased by 552 in 2016. A large amount of subsidy was also provided for digestate fertilizers for the replacement of mineral fertilizers with organic fertilizers for improved vegetable and fruit production. A total of 100 demo counties were constructed in 2017 (Zheng et al., 2020).
3.3.2 Equipment and technology innovation
The industrialization level of China’s biogas industry remains high compared with that of Africa. The use of advanced equipment can lead to high biogas production and utilization efficiency. For instance, the poor pre-treatment technology of feedstock leads directly to low gas production rates (Yu et al., 2019). The key components should be feedstock crushing or chopping equipment for straw. The use of mixing devices is important to guarantee the homogenization of feedstock. Moreover, the biogas desulfurization efficiency is used in China, and solid chemical adsorption technology is employed. Regular process monitoring and control are required to provide information about general process performance and safety and recognize and respond to process instabilities/disturbance (Jimenez et al., 2015).
3.3.3 Co-digestion plant
By comparison, very few biogas plants in China adopt the co-digestion technology, although numerous laboratory studies have investigated the co-digestion technology. Co-digestion provides great benefits for China. In China’s urbanization, the amount of household garbage and other organic wastes such as food waste is expected to increase remarkably (Hagos et al., 2017). The sewage sludge production in cities reached 10.53 million tons (dry matter) in 2017 (Skovsgaard and Jacobsen, 2017). The construction of a centralized co-digestion plant can be encouraged by introducing a special subsidy for feedstock in Tanzania. A detailed category for different substrates could be built.
4 Strategy for FS management at different AD scales in Tanzania
FS is a critical issue in Tanzania to archive the targets of Sustainable Development Goal (SDG) 6, such as SDG target 6.1: By 2030, achieve universal and equitable access to safe and affordable drinking water for all. FS is mainly stored in septic tanks and pit latrines, where sludge is removed either manually or mechanically. Collecting, emptying, and transport services are provided by the government water supply and sanitation authorities and informal and unregulated private operators by using vacuum trucks. In informal settlements, FS is collected but not treated, and much of it is dumped into landfill or water bodies, such as the ocean. This practice does not promote hygiene for all and does not end open defecation. In 1975, the development of biogas in Tanzania is credited to the Small Industries Development Organization (SIDO), which installed approximately 120 anaerobic digesters of the floating drum types, mostly in schools (Mshandete and Parawira, 2009). Biogas plants with digester volumes of 8, 12, to 16 m3 were deployed. After 5 years of the program, new standardized plant sizes were added with volumes of 12, 16, 30, and 50 m3 for institutions, households, and special “toilet biogas plants” (Mshandete and Parawira, 2009), while toilet biogas plants have been widely disseminated in China (Cheng et al., 2018). An augmentation was observed in the unit cost for installing an anaerobic digester ranging from Tshs 300,000 (USD 130) at 1989 to between Tshs 4,000,000 (1,700 USD) and Tshs 7,000,000 (3,000 USD) in the 1990s. Cheng et al. (2014) found that the initial cost of biogas plants can be reduced by introducing a low-cost polythene biodigester for African countries in 1993, and these digesters use animal manure as their feedstock. Tanzania has a successful biogas program, and approximately 8,796 biogas plants have been deployed (Mshandete and Parawira, 2009; Rupf et al., 2015). This finding serves as a good basis to introduce AD technology for FS treatment. Considering the context of Tanzania in the sub-Saharan African region, the FS treatment units at three different scales that are targeted for household-level, community-scale, and large-scale digesters are proposed, and the energy balances are analyzed.
4.1 Scenarios for FS treatment by AD
4.1.1 Household biogas digester (4 m3)
Most of household biogas digesters in Tanzania are operated under ambient temperature at 15–28°C depending on seasonal variation. The calculation of energy balances shows that the energy of 0.019 GJ/d can heat the digester and increase its temperature from 26 °C to 37°C (Fuchs et al., 2018; Campello et al., 2021). Without heating the digester, it can produce a methane volume of 1.95 m3/day under ambient temperature, and this volume can generate an energy of 0.031 GJ/d. The results show that heating energy could be balanced by the produced methane gas. Therefore, the introduction of the heating unit for household digesters is not feasible. The cost of installing the 4-m3 digester in Tanzania requires approximately 764 USD, as shown in Supplementary Table S5, and this amount includes the construction and operation cost. The household FS treatment and biogas production together with FW and animal manure can upgrade biogas production. Heating cost can be avoided by burying the digester underground without special isolation and heating measures.
4.1.2 Community-scale biogas digester (100 m3)
For the community-scale digester, a volume of 100 m3 can treat the FS from 50 families. The calculation shows that an energy of 0.27 GJ/day is required to heat the digester from 26 to 37°C (Fuchs et al., 2018; Campello et al., 2021). Under ambient temperature, the biogas digester with a working volume of 100 m3 in Tanzania costs 40,825 USD, as shown in Supplementary Table S6, and this piece of equipment could provide a heating value of 0.8 GJ/day, which is enough to reach a positive energy balance if the biogas is partially used for heating the digester. For the community-scale FS digester, the isolation measure could be implemented, and the heating unit could be installed if surplus biogas production is present and professional staff for operation and maintenance is available locally.
4.1.3 Large-scale biogas digester (400 m3)
For a large-scale digester (working volume, 400 m3) with the co-substrate, the initial installation cost is 1,820,454 USD, as shown in Supplementary Table S7; this cost includes the heating, feedstock, operation, and construction cost, where the plant can produce an energy production rate of 3.2 GJ/day (Fuchs et al., 2018; Campello et al., 2021) under ambient temperature, resulting in positive energy balance. Digester heating cost is avoided using different methods which are used on energy recovery; a system with energy recovery from produced biogas means that the heat and electricity produced from the digester can be utilized and re-used for supplying heat.
4.2 Biogas production and energy balance estimation
The AD process is complicated, and it depends on various parameters, such as the composition, size of feedstock, and operational conditions.
To make an AD model simple, basic assumptions have been made as follows:
1 The amount of biogas produced can be predicted based on the experimental results;
2 The digesters work at a hydraulic retention time of 30 days;
3 During the experimental period, no heat dispassion was observed from the digesters, and the ambient temperature was in the range of 20–26°C instead of temperature control;
4 A cubic meter of methane is equivalent to 34 MJ of energy;
5 The specific heat capacity (C) for FS is 4.2 MJ ton−1°C −1; and
6 Generally, 1 kg of FS generates approximately 15 L of biogas (0.015 m3/kg).
4.2.1 Methane production calculation
4.2.1.1 Methane yield at the 400-m3 digester
Daily feedstock volume (Q) = 400/30 = 13.3 m3 day−1 = 13,300 kg/day.
Methane gas production rate = Q × Biogas yield in fresh biogas feedstock.
Methane gas production rate is 13,300 kg/day × 0.015 m3/kg = 199.5 m3/day.
4.2.1.2 Methane yield at the 100-m3 digester.
Q = 100/30 = 3.3 m3 day−1 = 3,300 kg/day.
Methane gas production rate = Q × Biogas yield in fresh biogas feedstock.
Methane gas production rate = 3,300 kg/day × 0.015 m3/kg = 49.5 m3/day.
4.2.1.3 Methane yield at the 4-m3 digester.
Q = 4/30 = 0.13 m3 day−1 = 130 kg/day.
Methane gas production rate = Q × Biogas yield in fresh biogas feedstock.
Methane gas production rate = 130 kg/day × 0.015 m3/kg = 1.95 m3/day.
4.2.2 Energy balance calculations
The energy balance in AD system describes how much energy is used in an AD plant and how much is generated. Two sources of heat are required in AD as follows:
➢ Heat loss of the digester;
➢ Heat to bring the feedstock material up to the digester temperature.
4.2.2.1 Heat loss calculations
According to Fuchs et al. (2018), the expected heat loss (HL) from the digester surface can be calculated using the following equation:
where U = overall coefficient of heat transfer, W m−2°C −1, A = surface area of the digester m2, and ΔT = temperature drop across the surface in question, °C.
According to Sapkota and Poudel (2019), the reported digester heat transfer coefficient is U = 1.7 W m−2°C−1.
(a) Heat loss for the 400-m3 digester
Working volume: 80% of total volume.
D = 1.3078 V1/3 = 8.5 m;
H = 4 × 0.3142 D3/3.14 D2 = 2.83 m;
A = V/H = 320/2.83 = 113.074 m2.
The heat loss at the digester temperature of 37°C is as follows:
HL = UA (T2–T1)
HL = 1.7 × 113.074 × (37–26) = 2,114 kW = 0.36 GJ day−1.
(b) Heat loss for the 100-m3 digester
Working volume: 80% of total volume.
D = 1.3078 V 1/3 = 4.7 m;
H = 4 × 0.3142 D3/3.14 D2 = 1.87 m;
A = V/H = 80/1.87 = 42.78 m2.
The heat loss at the digester temperature of 37°C is as follows:
HL = UA (T2-T1)
HL = 1.7 × 42.78 × (37–26) = 799.986 kW = 0.12 GJday−1.
(c) Heat loss for the 4-m3 digester
Working volume: 80% of total volume.
D = 1.3078 V 1/3 = 1.8 m;
H = 4 × 0.3142 D3/3.14 D2 = 0.72 m;
A = V/H = 3.2/0.72 = 4.4 m2.
The heat loss at a digester temperature of 37°C is as follows:
HL = UA (T2–T1)
HL = 1.7 × 4.4 × (37–26) = 82.28 kW = 0.013 GJday−1.
4.2.2.2 Heating of the feedstock
According to Fuchs et al. (2018), the feedstock added to the digester must be brought up to the operating temperature of the digester, as expressed in Eq. 4 as follows:
where C = specific heat capacity of the feedstock (MJ tonne−1°C−1), Q = volume to be added (m3/day), and ΔT = temperature difference (°C)
(a) Heat required for the 400-m3 digester
A 400-m3 digester running at 37°C with a retention time of 30 days.
Volume added (Q) = 400/30 = 13.3 m3 day−1;
Specific heat capacity (C) = 4.2 MJ ton−1°C−1;
Feedstock temperature = 26°C;
Heat required = 4.2 × 13.3 × (37–26) = 0.62 GJ day−1;
Total heat requirement = heat loss + heat for feedstock;
Total heat requirement including heat loss = 0.62 + 0.36 = 0.98 GJ day−1.
(b) Heat required for the 100-m3 digester
Volume added (Q) = 100/30 = 3.3 m3 day−1;
Specific heat capacity (C) = 4.2 MJ ton−1°C−1;
Heat required = 4.2 × 3.3 × (37–26) = 0.153 GJ day−1;
Total heat requirement including heat loss = 0.15 + 0.12 = 0.27 GJ day−1.
(c) Heat required for the 4-m3 digester
Volume added (Q) = 4/30 = 0.13 m3 day−1;
Specific heat capacity (C) = 4.2 MJ ton−1 °C −1;
Heat required = 4.2 × 0.13 × (37–26) = 0.006 GJ day−1;
Total heat requirement including heat loss = 4.2 × 0.13 × (37–26) = 0.006 + 0.013 = 0.019 GJ day−1.
4.2.3 Energy generated from the produced biogas
The energy balance of digesters in scenarios 1, 2, and 3 was determined by assuming that the biogas produced was utilized using a combined heat and power (CHP) system. The energy output from the three digestion systems was calculated as described by Campello et al. (2021).
Electricity generated from the biogas produced = Biogas produced (m3) × LHVCH4 × methane content (%) × engine efficiency.
Heat generated from biogas produced = Biogas produced (m3) × LHVCH4 × methane content (%) × engine efficiency.
The assumptions made were as follows: lower heating value (LHV) of methane of 35.59 MJ/m3 and engine efficiencies of 40% for electrical energy and 50% for heat energy.
(a) Energy generated from biogas produced at the 400-m3 digester
Heat generated from biogas produced = Biogas produced (m3) × LHVCH4 × methane content (%) × engine efficiency.
(199.5% × 35.59% × 50.4% × 40%) + (199.5% × 35.59% × 50.4% × 50%) = 3,195 MJ = 3.2 GJ.
(b)Energy generated from biogas produced at the 100-m3 digester
Heat generated from biogas produced = Biogas produced (m3) × LHVCH4 × methane content (%) × engine efficiency.
(49.5% × 35.59% × 50.4% × 40%) + (49.5% × 35.59% × 50.4% × 50%) = 800 MJ = 0.8 GJ.
(c) Energy generated from biogas produced at the 4-m3 digester
Heat generated from biogas produced = Biogas produced (m3) × LHVCH4 × methane content (%) × engine efficiency.
(1.95% × 35.59% × 50.4% × 40%) + (1.95% × 35.59% × 50.4% × 50%) = 31.23 MJ = 0.031 GJ.
The energy balance analysis of biogas produced during the whole study period from the 4, 100, and 400 m3 digesters is shown in Table 5, where the biogas produced energy outputs of 0.031, 0.8, and 3.2 GJ. After subtracting the energy input (energy required in the system) from the energy output (energy leaving the system), the energy balance values were + 0.012, + 0.53, and + 2.22 GJ for the 4, 100, and 400 m3 digesters, respectively.
4.3 Cost and benefit analysis for biogas technology dissemination in the context of Tanzania in terms of FS treatment.
4.3.1 Benefit analysis
Tanzania, which belongs to sub-Saharan African (SSA) countries, has several favorable conditions for biogas technology utilization. The country is dominated by a tropical climate with an average monthly temperature above 18°C throughout the year, which is well suited for AD (Mshandete and Parawira, 2009). Livestock keeping and on-site sanitation infrastructures are abundant to provide a significant potential for biogas production from animal excreta. The increasing prices of fossil fuels and fertilizer have helped in making biogas an attractive alternative for energy and fertilizer production in Tanzania. The increasing prices of fuel wood and other energy sources for cooking and expensive lighting costs when using kerosene have prompted interest in biogas as a cheaper, cleaner, and more convenient alternative energy source. The benefits of biogas to Tanzania are categorized in the three main pillars of sustainability, namely, environmental, social, and economic factors.
4.3.1.1 Environmental benefits
Inadequate sanitation deteriorates the environment and public health in urban areas of developing countries than in rural areas, where simple and sustainable on-site sanitation solutions can be implemented. Emptying pit latrines is a major problem in urban sanitation in low-income countries. Biogas can offer a sanitation solution in urban areas and has gained prominence in recent years. A biogas latrine is an integrated waste management system that provides a sanitation solution and energy in the form of biogas, and it reduces the collection and transportation cost of FS from an on-site system to a treatment facility (Mutai et al., 2016). Biogas generation may improve the water quality. Moreover, AD deactivates pathogens and parasites; thus, it is also quite effective in reducing the incidence of waterborne diseases. Similarly, waste collection and management are remarkably improved in areas with biogas plants. This condition leads to improvements in the environment, sanitation, and hygiene (Berhe et al., 2017). Firewood is the main energy source for cooking in Tanzania. The cutting down of trees for firewood has resulted in the rapid deterioration of forest reserves. In recent years, the abundant natural vegetation in the country has been cleared/transformed for agriculture, habitation, and firewood, thus contributing to climate change (Velempini et al., 2018). Brown (2006) added that a well-designed and installed biogas digester has several benefits; “it improves sanitation by converting generated FS into biogas; it reduces greenhouse gas (GHG) emissions; it serves time to search firewood and charcoal for cooking, preserve deforestation and natural vegetation, and it provides a high-quality organic fertilizer.” Berhe et al. (2017) viewed biogas systems as a sustainable source of energy that can provide low-cost energy without gathering wood as fuel; it can lessen the degradation of indigenous forests, reduce GHG emissions into the air, and improve the carbon sequestration of indigenous forests. Kelebe and Olorunnisola, 2016 confirmed this finding and stated that 12 rural households that substituted firewood with biogas resulted in a decrease of 50%–60% of firewood consumption. They also stated that the 9,577 domestic biogas systems installed in Ethiopia in 2014 saved approximately 2,873 ha of the forest land. Minde et al. (2013) pointed out that if 1 kg of wood is burned in traditional cookstoves, it generates approximately 318 g of C. However, if biogas is used, each household saves the consumption of 3 metric ton of firewood annually. Biogas technology for FS treatment can combat environmental challenges such as spread of diseases, eutrophication, acidification, air pollution, and climate change issues (Cheng et al., 2022).
4.3.1.2 Social benefits
FSM is not gender-neutral and could deepen gender inequalities if not handled appropriately. Interventions must be based on a good understanding of gender-specific needs and, in particular, the constraints faced by women and girls in accessing safe sanitation. The lack of access to sanitation facilities has a differential impact on gender because of expectations regarding modesty and personal security. The unavailability of good sanitation facilities can lead to psychosocial stress for girls, whereas access to good sanitation reduces child mortality and death while supporting health (Hirve et al., 2014). The major responsibility of energy needs in terms of access to wood fuel requirements lies largely with women and children who have to trek long distances to harvest them for domestic use. This process is time-consuming and tiring because the loads are usually very heavy. This cultural practice is carried out mainly by women and children, especially young girls, who spend much time to search firewood in many parts of SSA countries. Lambe et al. (2015), Minde et al. (2013), and Fullerton et al. (2008) pointed out that biomass burning releases pollutants such as carbon monoxide, methane, nitrogen oxides, benzene, formaldehyde, benzopyrene, aromatics, and particulate matter, which cause considerable damage to women and children because they are more at risk for prolonged exposure. An additional indirect benefit of the use of AD systems is a product of the biogas generated and captured from these systems. Several communities that utilize AD and capture biogas do not have access to the main electric grid because of the remoteness or the high cost. The electricity produced from biogas becomes a tremendous social benefit to communities as it can change the way communities interact. AD systems can give farmers energy independence and make them self-sufficient. Another social benefit is the creation of jobs. When farms or mills utilize workers for daily operations, the addition of an AD system can create new jobs. Learning how to operate an AD system and perform routine operations and maintenance checks also improves laborer skills and can help establish the local expertise needed for additional biomass electricity systems. Smokeless biogas is an excellent substitute for use in developing countries, especially in Tanzania, to improve the well-being of women and children. The time saved from gathering fuelwood can be used for productive ventures and provide females with an opportunity to be in school.
4.3.1.3 Economic benefits
The inappropriate FSM facilities cause economic losses because of the cost of treating illnesses that result from poor sanitation and the loss of income through reduced productivity. Emptying FS remains a challenge in many cities because low-income households cannot afford the service (Cheng et al., 2017). Enormous revenue is generated by the various service providers who are active in this segment of FSM. In Tanzania, the Ministry of Water reported the emptying charges that occasionally elevates to as high as TZS 300,000 (130 USD) per trip when servicing far distances by using a private operator. AD systems eliminate the need to transport waste to an established waste disposal facility. The use of waste onsite cuts the upfront costs of transportation and generates financial gains with the byproducts produced. The process creates a viable energy source that can be used for heating and electricity for homes or the facility itself. This biomass energy may come with a net zero cost and offset electrical costs, thus providing new forms of energy to areas that may not have access to traditional electrical energy sources or generating profit if the energy produced can be sold. The bio-slurry from biogas plants is estimated to have high a nitrogen content compared with fresh manure, thus allowing AD systems to generate commodities such as fertilizer, which can be used at the site or sold for additional income. When bio-slurry is considered as manure, the return on investment in the process can be realized within 3–4 years (Minde et al., 2013). A nationwide deployment of biogas technology for institutions will generate many jobs in the form of carpentry, masonry, and plumbing. Cost savings on fossil fuel importation will also be realized.
4.3.2 Cost analysis
The cost of a domestic biogas digester falls into two categories, namely, construction and operation. The construction cost has three major components, namely, materials (e.g., cement, sand, gravel, bricks, steel rods and wire, and coatings), excavation and construction (e.g., technician service, labor, and steel mold used to cast concrete), and gas appliance parts (e.g., pipeline and valves, gas pressure gauge, desulfurizer, gas cooker, and gas lamp).
Operational costs also have three major components, namely, (e.g., collection, preparation, and purchase), maintenance (e.g., feeding and discharge), and repair or replacement of parts (e.g., gas pressure gauges, pipeline and valves, cooker spare parts, and lamp mantles). However, major difficulties are encountered in calculating operational costs. However, the manure or other feedstock generated by individual households has no commercial value in that case, and the operation cost is 2%–10% of the construction cost (Carmatec, 2020). The calculation shows that the total construction cost of 4, 100, and 400 m3 digesters are 764, 40,825, and 1,820,454 USD, respectively.
5 Conclusion
In developing countries such as Tanzania, FSM is indeed a big challenge toward goal 6 of SDGS. Based on the laboratory tests by using mesophilic digestion at 37°C, a methane yield of 396 ml/g VS was achieved with the increment of 109 ml/g VS compared with the non-biochar addition experiment. The results showed that FS is a good raw material to be used for biogas production. The study provided a good insight into biochar-amended mesophilic AD of FS for enhanced methane production in terms of biochar dosage and particle sizes with the optimal dosage of 10–20 g/L. In comparison with the control digester without biochar amendment, average methane yields increased to 234–396 ml/gVS.
Considering the tropic climate, the laboratory test under ambient conditions in Tanzania showed that although the specific biogas production was relatively low, such digesters may have a great potential for practical applications in Tanzania because of the low capital and ease of operation. The theoretical analyses show that energy consumes large amounts of biogas when relied upon heating the digesters. However, the energy needed to optimize the reactor temperature can be balanced out by a partial amount of biogas produced. A strategy for FS treatment systems in the context of Tanzania has been proposed and developed. The practical application options for different local conditions include a household FS treatment and biogas production system (where a digester could be buried underground without special isolation and heating measures), community-scale FS treatment and a biogas production system (where isolation measures could be taken, and heating could be installed if there is surplus biogas, and professional staff for operation and maintenance is available locally), and large-scale FS treatment and biogas production equipped with modern technology and equipment for maximum biogas production. Although this study has been a successful comparative study, local biochar (man-made) and industrially produced biochar could be used for more clarification.
Data availability statement
The original contributions presented in the study are included in the article/Supplementary Material; further inquiries can be directed to the corresponding authors.
Author contributions
MK contributed to the writing of the original draft and methodology. SC contributed to the conceptualization, writing, reviewing, and editing of the manuscript. SM, SN, and KV contributed to the methodology, writing, reviewing, and editing of the manuscript. XL and KD contributed to the data curation of the study. XW contributed to visualization of the study. ZL contributed to the funding acquisition and supervision of the study. All authors approved the submitted version.
Funding
The study was supported by the National Key Research and Development Plan (2019YFC0408700), the USTB Youth Teacher International Exchange and Growth Program (QNXM20210029), and the USTB Research Center for International People-to-People Exchange in Science, Technology and Civilization (2022KFZD007, 2022KFYB020, and 2022KFYB024).
Acknowledgments
The authors would like to take this opportunity to express their sincere appreciation for the support of the China National Environmental and Energy Science and Technology International Cooperation Base and the International Scientific and Technological Cooperation and Exchange Projects of Ardhi University, Dar es Salaam, Tanzania.
Conflict of interest
The authors declare that the research was conducted in the absence of any commercial or financial relationships that could be construed as a potential conflict of interest.
Publisher’s note
All claims expressed in this article are solely those of the authors and do not necessarily represent those of their affiliated organizations, or those of the publisher, the editors, and the reviewers. Any product that may be evaluated in this article, or claim that may be made by its manufacturer, is not guaranteed or endorsed by the publisher.
Supplementary material
The Supplementary Material for this article can be found online at: https://www.frontiersin.org/articles/10.3389/fenvs.2022.911348/full#supplementary-material
References
Afifah, U., and Priadi, C. R. (2017). Biogas potential from anaerobic co-digestion of faecal sludge with food waste and garden waste. AIP Conf. Proc. 1826, 020032. doi:10.1063/1.4979248
APHA (2017). Standard methods for the examination of water and wastewater. 23rd Edition. Washington, DC: American Public Health Association APHA.
Arikan, O. A., Mulbry, W., and Lansing, S. (2015). Effect of temperature on methane production from field-scale anaerobic digesters treating dairy manure. Waste Manag. 43, 108–113. doi:10.1016/j.wasman.2015.06.005
Bassan, M. (2014). in Faecal sludge management: Systems approach for implementation and operation. Editors L. Strande, L. Ronteltap, and D. Brdjanovic (London: IWA publishing).
Bakraoui, M., Karouach, F., Ouhammou, B., Aggour, M., Essamri, A., and El Bari, H. (2020). Biogas production from recycled paper mill wastewater by UASB digester: Optimal and mesophilic conditions. Biotechnol. Rep. 25, e00402. doi:10.1016/j.btre.2019.e00402
Berhe, M., Hoag, D., Tesfay, G., and Keske, C. (2017). Factors influencing the adoption of biogas digesters in rural Ethiopia. Energy sustain. Soc. 7, 10. doi:10.1186/s13705-017-0112-5
Brown, V. J. (2006). Biogas: A bright idea for Africa. Environ. Health Perspect. 114, A300–A303. doi:10.1289/ehp.114-a300
Browne, J. D., Gilkinson, S. R., and Frost, J. P. (2015). The effects of storage time and temperature on biogas production from dairy cow slurry. Biosyst. Eng. 129, 48–56. doi:10.1016/j.biosystemseng.2014.09.008
Cai, J., He, P., Wang, Y., Shao, L., and Lu, F. (2016). Effects and optimization of the use of biochar in anaerobic digestion of food wastes. Waste Manag. Res. 34, 409–416. doi:10.1177/0734242x16634196
Campello, L. D., Barros, R. M., Tiago Filho, G. L., and dos Santos, I. F. S. (2021). Analysis of the economic viability of the use of biogas produced in wastewater treatment plants to generate electrical energy. Environ. Dev. Sustain. 23, 2614–2629. doi:10.1007/s10668-020-00689-y
Carmatec, (2020). Tanzania domestic biogas program report 2020. Dar es Salaam: Centre for Agricultural Mechanization and Rural Technology CARMATEC.
Cheng, Q., Xu, C., Huang, W., Jiang, M., Yan, J., Fan, G., et al. (2020). Improving anaerobic digestion of piggery wastewater by alleviating stress of ammonia using biochar derived from rice straw. Environ. Technol. Innovation 19, 100948. doi:10.1016/j.eti.2020.100948
Cheng, S. K., Zheng, S., Bai, X., and Zhao, S. M. Y. (2017). Assessment of two faecal sludge treatment plants in urban areas: Case study in Beijing. Int. J. Agric. Biol. Eng. 10, 237–245. doi:10.3965/j.ijabe.20171003.3067
Cheng, S., Li, Z., Mang, H. P., Huba, E. M., Gao, R., and Wang, X. (2014). Development and application of prefabricated biogas digesters in developing countries. Renew. Sustain. Energy Rev. 34, 387–400. doi:10.1016/j.rser.2014.03.035
Cheng, S., Li, Z., Uddin, S. M. N., Mang, H. P., Zhou, X., Zhang, J., et al. (2018). Toilet revolution in China. J. Environ. Manag. 216, 347–356. doi:10.1016/j.jenvman.2017.09.043
Cheng, S., Long, J., Evans, B., Zhan, Z., Li, T., Chen, C., et al. (2022). Non-negligible greenhouse gas emissions from non-sewered sanitation systems: A meta-analysis. Environ. Res. 212, 113468. doi:10.1016/j.envres.2022.113468
Cooney, M. J., Lewis, K., Harris, K., Zhang, Q., and Yan, T. (2016). Start up performance of biochar packed bed anaerobic digesters. J. Water Process Eng. 9, e7–e13. doi:10.1016/j.jwpe.2014.12.004
Dev, S., Saha, S., Kurade, M. B., Salama, E. S., El-Dalatony, M. M., Ha, G. S., et al. (2019). Perspective on anaerobic digestion for biomethanation in cold environments. Renew. Sustain. Energy Rev. 103, 85–95. doi:10.1016/j.rser.2018.12.034
Dhungana, B., Lohani, S. P., and Marsolek, M. (2022). Anaerobic Co-digestion of food waste with livestock manure at ambient temperature: A biogas based circular economy and sustainable development goals. Sustainability 14, 3307. doi:10.3390/su14063307
Fagbohungbe, M. O., Herbert, B. M., Hurst, L., Li, H., Usmani, S. Q., and Semple, K. T. (2016). Impact of biochar on the anaerobic digestion of citrus peel waste. Bioresour. Technol. 216, 142–149. doi:10.1016/j.biortech.2016.04.106
Filer, J., Chang, S., and Ding, H. (2019). Biochemical methane potential (BMP) assay method for anaerobic digestion research. Water 11. doi:10.3390/w11050921
Fuchs, W., Wang, X., Gabauer, W., Ortner, M., and Li, Z. (2018). Tackling ammonia inhibition for efficient biogas production from chicken manure: Status and technical trends in Europe and China. Renew. Sustain. Energy Rev. 97, 186–199. doi:10.1016/j.rser.2018.08.038
Fullerton, D. G., Bruce, N., and Gordon, S. B. (2008). Indoor air pollution from biomass fuel smoke is a major health concern in the developing world. Trans. R. Soc. Trop. Med. Hyg. 102, 843–851. doi:10.1016/j.trstmh.2008.05.028
Hagos, K., Zong, J., Li, D., Liu, C., and Lu, X. (2017). Anaerobic co-digestion process for biogas production: Progress, challenges and perspectives. Renew. Sustain. Energy Rev. 76, 1485–1496. doi:10.1016/j.rser.2016.11.184
Haider, M. R., Zeshan, , Yousaf, S., Malik, R. N., and Visvanathan, C. (2015). Effect of mixing ratio of food waste and rice husk co-digestion and substrate to inoculum ratio on biogas production. Bioresour Technoogy 190, 451–457. doi:10.1016/j.biortech.2015.02.105
He, P., Liu, Y., Shao, L., Zhang, H., and Lu, F. (2018). Particle size dependence of the physicochemical properties of biochar. Chemosphere 212, 385–392. doi:10.1016/j.chemosphere.2018.08.106
Hewage, S., and Priyadarshani, R. (2016). Effect of charred digestate (biochar) and digestate on soil organic carbon and nutrients in temperate bioenergy crop production systems. Hamburg, Germany: Universität Hamburg Hamburg.
Hirve, S., Lee, P., and Weiss, M. (2014). Psychosocial stress associated with sanitation practices: Experiences of women in a rural community in India. J. Water, Sanitation Hyg. Dev. 5, 115–126. doi:10.2166/washdev.2014.110
Jimenez, J., Latrille, E., Harmand, J., Robles, A., Ferrer, J., Gaida, D., et al. (2015). Instrumentation and control of anaerobic digestion processes: A review and some research challenges. Rev. Environ. Sci. Biotechnol. 14, 615–648. doi:10.1007/s11157-015-9382-6
Kelebe, H. E., and Olorunnisola, A. (2016). Biogas as an alternative energy source and a waste management strategy in Northern Ethiopia. Biofuels 7, 479–487. doi:10.1080/17597269.2016.1163211
Khan, E. U., and Martin, A. R. (2016). Review of biogas digester technology in rural Bangladesh. Renew. Sustain. Energy Rev. 62, 247–259. doi:10.1016/j.rser.2016.04.044
Lambe, F., Jürisoo, M., and Wanjiru, H. (2015). Bringing clean, safe, affordable cooking energy to households across Africa: An agenda for action. Stockholm and Nairobi, for the new climate economy: Prepared by the Stockholm Environment Institute.
Lohani, S. P., Pokhrel, D., Bhattarai, S., and Pokhrel, A. K. (2022). Technical assessment of installed domestic biogas plants in Kavre, Nepal. Renew. Energy 181, 1250–1257. doi:10.1016/j.renene.2021.09.092
Makoye, K. (2017). Tanzanian city gets new sewage scheme to curb disease, ocean pollution. Zanzibar, Tanzania: WIOMSA and UN-Habitat.
Martí-Herrero, J., Alvarez, R., Cespedes, R., Rojas, M., Conde, V., Aliaga, L., et al. (2015). Cow, sheep and llama manure at psychrophilic anaerobic co-digestion with low cost tubular digesters in cold climate and high altitude. Bioresour. Technol. 181, 238–246. doi:10.1016/j.biortech.2015.01.063
Mehariya, S., Patel, A. K., Obulisamy, P. K., Punniyakotti, E., and Wong, J. W. (2018). Co-digestion of food waste and sewage sludge for methane production: Current status and perspective. Bioresour. Technol. 265, 519–531. doi:10.1016/j.biortech.2018.04.030
Minale, M., and Worku, T. (2014). Anaerobic co-digestion of sanitary wastewater and kitchen solid waste for biogas and fertilizer production under ambient temperature: Waste generated from condominium house. Int. J. Environ. Sci. Technol. (Tehran). 11, 509–516. doi:10.1007/s13762-013-0255-7
Minde, G., Sandip, S., and Kalyanraman, V. (2013). Biogas as a sustainable alternative for current energy need of India. J. Sustain. Energy & Environ. 4, 121–132.
Mrimi, E. C., Matwewe, F. J., Kellner, C. C., and Thomas, J. M. (2020). Safe resource recovery from faecal sludge: Evidence from an innovative treatment system in rural Tanzania. Environ. Sci. Water Res. Technol. 6, 1737–1748. doi:10.1039/c9ew01097a
Mshandete, A., and Parawira, W. (2009). Biogas technology research in selected sub-Saharan African countries - a review. Afr. J. Biotechnol. 8, 116–125.
Mutai, P., Charles, N., John, B. T., and Robinah, N. (2016). Key factors affecting performance of biogas latrines in urban informal areas: Case of Kampala and Nairobi, East Africa. Afr. J. Environ. Sci. Tech. 10, 207–220. doi:10.5897/ajest2016.2108
Nandi, R., Saha, C. K., Sarker, S., Huda, M. S., and Alam, M. M. (2020). Optimization of reactor temperature for continuous anaerobic digestion of cow manure: Bangladesh perspective. Sustainability 12, 8772. doi:10.3390/su12218772
Nasir, I. M., Mohd Ghazi, T. I., and Omar, R. (2012). Anaerobic digestion technology in livestock manure treatment for biogas production: A review. Eng. Life Sci. 12, 258–269. doi:10.1002/elsc.201100150
Pan, J., Ma, J., Liu, X., Zhai, L., Ouyang, X., and Liu, H. (2019). Effects of different types of biochar on the anaerobic digestion of chicken manure. Bioresour. Technol. 275, 258–265. doi:10.1016/j.biortech.2018.12.068
Ripoll, V., Agabo-Garcia, C., Perez, M., and Solera, R. (2020). Improvement of biomethane potential of sewage sludge anaerobic co-digestion by addition of “sherry-wine” distillery wastewater. J. Clean. Prod. 251, 119667. doi:10.1016/j.jclepro.2019.119667
Rupf, G. V., Bahri, P. A., de Boer, K., and McHenry, M. P. (2015). Barriers and opportunities of biogas dissemination in Sub-Saharan Africa and lessons learned from Rwanda, Tanzania, China, India, and Nepal. Renew. Sustain. Energy Rev. 52, 468–476. doi:10.1016/j.rser.2015.07.107
Sabbir, A. S. M. Y. B., Saha, C. K., Nandi, R., Zaman, M. F. U., Alam, M. M., and Sarker, S. (2021). Effects of seasonal temperature variation on slurry temperature and biogas composition of a commercial fixed-dome anaerobic digester used in Bangladesh. Sustainability 13, 11096. doi:10.3390/su131911096
Sapkota, P., and Poudel, L. (2019). Heat exchanger for bio-digester under thermophilic range. J. Adv. Coll. Engin. Mgt. 5, 79–88. doi:10.3126/jacem.v5i0.26691
Shanmugam, P., and Horan, N. J. (2009). Optimising the biogas production from leather fleshing waste by co-digestion with MSW. Bioresour. Technol. 100, 4117–4120. doi:10.1016/j.biortech.2009.03.052
Simeon, M. I. (2017). Biogas production from the Co-digestion of cow dung and poultry droppings using a plastic cylindrical digester.
Sinervo, R. (2017). Effects of biochar addition on anaerobic digestion and comparison of different biochar qualities.
Skovsgaard, L., and Jacobsen, H. K. (2017). Economies of scale in biogas production and the significance of flexible regulation. Energy Policy 101, 77–89. doi:10.1016/j.enpol.2016.11.021
Soler-Cabezas, J. L., Mendoza-Roca, J., Vincent-Vela, M., Lujan-Facundo, M., and Pastor-Alcaniz, L. (2018). Simultaneous concentration of nutrients from anaerobically digested sludge centrate and pre-treatment of industrial effluents by forward osmosis. Sep. Purif. Technol. 193, 289–296. doi:10.1016/j.seppur.2017.10.058
Vanegas, C., and Bartlett, J. (2013). Anaerobic digestion of laminaria digitata: The effect of temperature on biogas production and composition. Waste Biomass Valorization 4, 509–515. doi:10.1007/s12649-012-9181-z
Velempini, K., Smucker, T. A., and Clem, K. R. (2018). Community-based adaptation to climate variability and change: Mapping and assessment of water resource management challenges in the north pare highlands, Tanzania. Afr. Geogr. Rev. 37, 30–48. doi:10.1080/19376812.2016.1229203
Yong, Z., Dong, Y., Zhang, X., and Tan, T. (2015). Anaerobic co-digestion of food waste and straw for biogas production. Renew. Energy 78, 527–530. doi:10.1016/j.renene.2015.01.033
Yu, Q., Liu, R., Li, K., and Ma, R. (2019). A review of crop straw pretreatment methods for biogas production by anaerobic digestion in China. Renew. Sustain. Energy Rev. 107, 51–58. doi:10.1016/j.rser.2019.02.020
Zhang, L., Guo, B., Zhang, Q., Florentino, A., Xu, R., Zhang, Y., et al. (2019). Co-digestion of blackwater with kitchen organic waste: Effects of mixing ratios and insights into microbial community. J. Clean. Prod. 236, 117703. doi:10.1016/j.jclepro.2019.117703
Keywords: anaerobic digestion, fecal sludge, co-digestion, sanitation, energy balance
Citation: Kilucha M, Cheng S, Minza S, Nasiruddin SM, Velempini K, Li X, Wang X, Mokeira Doroth K and Li Z (2022) Insights into the anaerobic digestion of fecal sludge and food waste in Tanzania. Front. Environ. Sci. 10:911348. doi: 10.3389/fenvs.2022.911348
Received: 02 April 2022; Accepted: 15 July 2022;
Published: 26 August 2022.
Edited by:
Xiaofei Tan, Hunan University, ChinaReviewed by:
Sunil Prasad Lohani, Kathmandu University, NepalYi Liu, Biogas Institute of Ministry of Agriculture (CAAS), China
Copyright © 2022 Kilucha, Cheng, Minza, Nasiruddin, Velempini, Li, Wang, Mokeira Doroth and Li. This is an open-access article distributed under the terms of the Creative Commons Attribution License (CC BY). The use, distribution or reproduction in other forums is permitted, provided the original author(s) and the copyright owner(s) are credited and that the original publication in this journal is cited, in accordance with accepted academic practice. No use, distribution or reproduction is permitted which does not comply with these terms.
*Correspondence: Shikun Cheng, Y2hlbmdzaGlrdW5AdXN0Yi5lZHUuY24=; Zifu Li, emlmdWxlZUBhbGl5dW4uY29t