- 1Institute of Oceanography, College of Geography and Oceanography, Minjiang University, Fuzhou, China
- 2Department of Civil and Resource Engineering, Faculty of Engineering, Dalhousie University, Halifax, NS, Canada
- 3School of Marine Science, Sun Yat-sen University, Zhuhai Campus, Zhuhai, China
- 4Pearl River Estuary Marine Ecosystem Research Station, Ministry of Education, Zhuhai, China
- 5Department of Engineering, Faculty of Agriculture, Dalhousie University, Truro, NS, Canada
Bitumen, an unconventional crude oil, has received much attention with the increasing consumption and the shrinking storage of conventional crude oils. Bitumen is highly viscous and, thus, is commonly diluted for transportation purposes. Spills of diluted bitumen could occur during the transportation from reservoirs to refineries via pipeline, rail, and marine vessels. Although some laboratory and numerical modeling studies have been contributed to study the spill of diluted bitumen from different aspects, there is no systematic review in the field yet. Therefore, this study first conducted a review on different types of diluted bitumen based on their physicochemical properties, followed by their weathering processes including spreading, evaporation, emulsification, photooxidation, biodegradation, and sinking. Second, the numerical modeling on the fate and behavior of spilled diluted bitumen was summarized and analyzed. Finally, the techniques for spilled oil recovery were discussed, as well as the disposal/treatment of oily waste. Currently, a rare attempt has been made to turn the recovered oily waste into wealth (reutilization/valorization of oily waste). Using the recovered oily waste as the feedstock/processing medium for an emerging thermochemical conversion technique (hydrothermal liquefaction of biomass for crude bio-oil production) is highly recommended. Overall, this article summarized the state-of-the-art knowledge of the spill of diluted bitumen, with the hope to create a deep and systematic understanding on the spill of diluted bitumen for researchers, relevant companies, and decision makers.
1 Introduction
Conventional fossil fuel is crucial for industrialization and the development of our society. Although conventional oil is still the primary energy supply worldwide, its reservoir is rapidly shrinking. Oil industries and governments are therefore actively seeking unconventional oil resources, such as the bitumen extracted from oil sands. Bitumen deposits have been found in Canada, Kazakhstan, Russia, and Venezuela. Canada occupied almost 70% of bitumen reservoirs in the world, making it the world’s third-largest oil reserve country. However, the bitumen extracted from oil sands is usually not able to be transported via pipeline due to its high viscosity and density. Thus, it is necessary to reduce the bitumen’s viscosity and density to meet the requirements for pipeline transportation. The dilution of bitumen based on different diluents (e.g., natural-gas condensate or synthetic crude) is the most commonly used technique to decrease oil viscosity in the petroleum industry, yielding the diluted bitumen (King, Robinson, Boufadel, & Lee, 2014). About 95% of bitumen and other heavy oils are transported to coastal ports by pipeline in Canada and then shipped to global markets by marine tankers (Martínez-Boza et al., 2011; King, Robinson, Boufadel, & Lee, 2014). The growing volume of diluted bitumen transportation has led to an increasing potential for spills. For instance, the pipeline operated by Kinder Morgan released about 224 m3 of diluted bitumen into the Burrard Inlet, British Columbia, Canada, in 2007 (Environment Canada, 2013); the Enbridge Line 6B pipeline leaked about 3,320–3,790 m3 of diluted bitumen into the Kalamazoo River in July 2010 (Dollhopf et al., 2014; Swarthout et al., 2016); the Enbridge’s Athabasca pipeline released about 230 m3 of diluted bitumen into the Red Deer River on 18 June 2012 (Brown, 2012). Accurate prediction of the spilled diluted bitumen’s transport and behavior is of importance to local authorities and oil companies for quick and efficient response to diluted bitumen spills. Some studies have been carried out regarding the physicochemical properties of diluted bitumen and the investigation/simulation of its weathering processes (Yang et al., 2011; Banerjee, 2012a; Environment Canada, 2013; Menzies and Thomas, 2014; Tsaprailis et al., 2014; Dew et al., 2015; Fingas, 2015; Yarranton et al., 2015; Hounjet and Dettman, 2016; Wu et al., 2016; Sereneo, 2017; Ortmann et al., 2020; Li et al., 2022). For instance, Menzies and Thomas (2014) investigated the physical properties of diluted bitumen and compared them with the conventional crude oils. Environmental Canada (2013) conducted a series of experiments to study the weathering processes of diluted bitumen. Li et al. (2022) developed a model that can simulate the weathering processes of diluted bitumen. However, no review effort has been made to summarize the corresponding findings and advances, in particular, compared with heavy crude oil that also has a high viscosity, and its spill modeling has been well developed.
Furthermore, many attempts have been made for the emergency response to spilled oil and the treatment of oily waste, to minimize the negative impact on the environment, ecology, and socioeconomics. The oil spill response technologies can be generally classified into three categories: physical, chemical, and biological methods. The physical method mainly refers to using booms, skimmers, and sorbents to recover the spilled oil on the water surface. Chemical and biological methods can be used in conjunction with physical means for further cleanup. The recovered oily waste is typically disposed of by landfill/incineration. In addition, the oily wastewater with high salinity is treated with a biological membrane reactor to meet the wastewater discharge requirement. Although some reviews have been conducted on the conventional oil spill emergency response and oily wastewater treatment, none has been found for diluted bitumen (Dave and Ghaly, 2011; Han et al., 2019; Dhaka and Chattopadhyay, 2021).
It is also worthwhile mentioning that the concept of waste to wealth is gaining momentum worldwide (Clark, 2017; Xu et al., 2019). How to reutilize/convert the collected oily wastes into high-value products is of significant research and industry interests. Currently, many biowaste conversion technologies have been developed to valorize various wastes. Unfortunately, no review is conducted on the suitability of these conversion techniques for oily waste recovery.
Therefore, this study is aiming at providing reviews on physicochemical properties, weathering processes, and numerical simulation of diluted bitumen and its spills, in comparison to heavy crude oils. In addition, the emergency response and recovery/reutilization of spilled oils are reviewed and discussed. Correspondingly, this review is organized as follows: 1) the physical and chemical properties of diluted bitumen products compared with conventional oils are presented in Section 2; 2) the weathering processes of diluted bitumen and its toxicity to aquatic organisms are summarized and compared with that of heavy crude oils in Section 3; 3) the emergency response technologies including oil spill modeling and oil spill countermeasures are reviewed in Section 4; 4) the valorization of collected oily wastes is reviewed in Section 5, focusing on the reutilization of oily waste and the suitable biowaste conversion technology; and 5) current challenges in the field and corresponding future works are presented and discussed in Section 6, and the conclusions are presented in Section 7.
2 Physical and Chemical Properties
Bitumen products are produced by surface mining/in situ recovery from oil sands. Bitumen is hard to flow naturally due to its high density, high viscosity, and high metal concentration (Humphries, 2007). Usually, bitumen products are upgraded and/or blended with diluents to reduce their viscosity (no more than 350 cSt) to meet the pipeline transportation requirement (Table 1).
Bitumen can be blended with condensate and/or synthetic crude oil to generate different products called dilbit, synbit, and syndilbit. Condensate is a light oil product typically produced from a gas well, while synthetic crude oil is produced by upgrading bitumen. As shown in Figure 1, diluted bitumen products are classified by their composition. Dilbit contains 20–30% condensate and 70–80% bitumen, while synbit is blended with 50% synthetic crude with 50% bitumen (Crosby et al., 2013). Syndilbit is a mixture of 75% bitumen, 16% condensates, and 19% synthetic crude oils (Fekete, 2006).
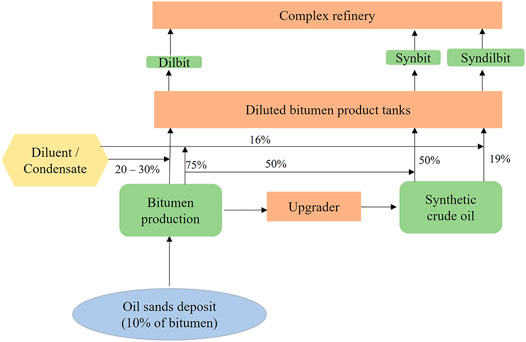
FIGURE 1. Products of diluted bitumen derived from oil sands (Fekete, 2006; Crosby et al., 2013).
Petroleum products are usually classified based on their American Petroleum Institute (API) gravity (or density) and viscosity. As shown in Table 2, the API gravities of bitumen and extra-heavy crude oil are generally less than 10°, while those of heavy crudes are between 10 and 22.3°. Furthermore, the viscosity of bitumen (>10,000 cSt) is higher than all the heavy and extra-heavy crudes (100–10,000 cSt) (Fingas, 2015; Oil Sands Magazine, 2020). The analogously high viscosity of bitumen, heavy crudes, and extra-heavy crudes are due to their high asphaltenes compositions (Menzies and Thomas, 2014). As for the diluted bitumen, the API gravity and viscosity of dilbit, synbit, and syndilbit are 20–22°, 19–21° and 19–22° and 350, 128 and176 cSt (at 15°C), respectively (Banerjee, 2012b; Oil Sands Magazine, 2020). It is worth noting that the oil viscosity is sensitive to temperature. As shown in Figure 2, with the increase in temperature, the viscosity of light crude appears to insignificantly decrease. In contrast, the viscosities of both dilbit and heavy crudes are significantly decreasing with temperature increasing from 10 to 45°C (Tsaprailis et al., 2014).
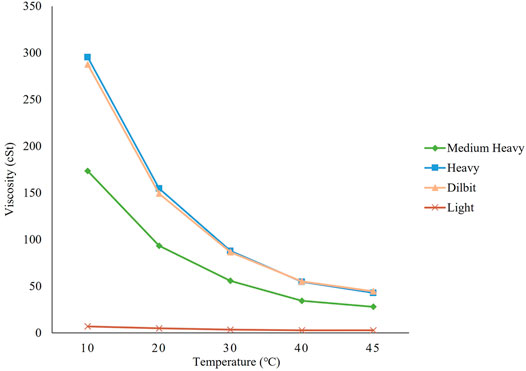
FIGURE 2. The relationship between temperature and viscosity of conventional crudes and dilbit. Adapted from Tsaprailis et al. (2014) (Tsaprailis et al., 2014).
The chemical properties of dilbit, synbit, syndilbit, light crude, medium crude, and heavy crude are presented in Table 3. The mean values and standard deviations of chemical properties for each product produced in the recent 5 years (2017–2022) were included. The light ends are referring to propane, butanes, pentanes, hexanes, heptanes, octanes, nonanes, and decanes. Dilbit contains a larger percentage of light ends than synbit, mainly due to condensate being rich in light-end compounds compared to synthetic crude oil. The micro carbon residual (MCR) is an indicator of the tendency to form carbon deposits under high-temperature conditions in an inert atmosphere. In general, dilbit has a comparable MCR with synbit and syndilbit (around 10%). BTEX (benzene, toluene, ethylbenzene, and xylene) are commonly existing compounds in oils. They are crucial for achieving satisfactory oil properties (especially for oil lubricity), even though they only account for a small percentage (Yang et al., 2019b). The contents of BTEX and sulfur in dilbit are slightly higher than in synbit and syndilbit as shown in Table 3.
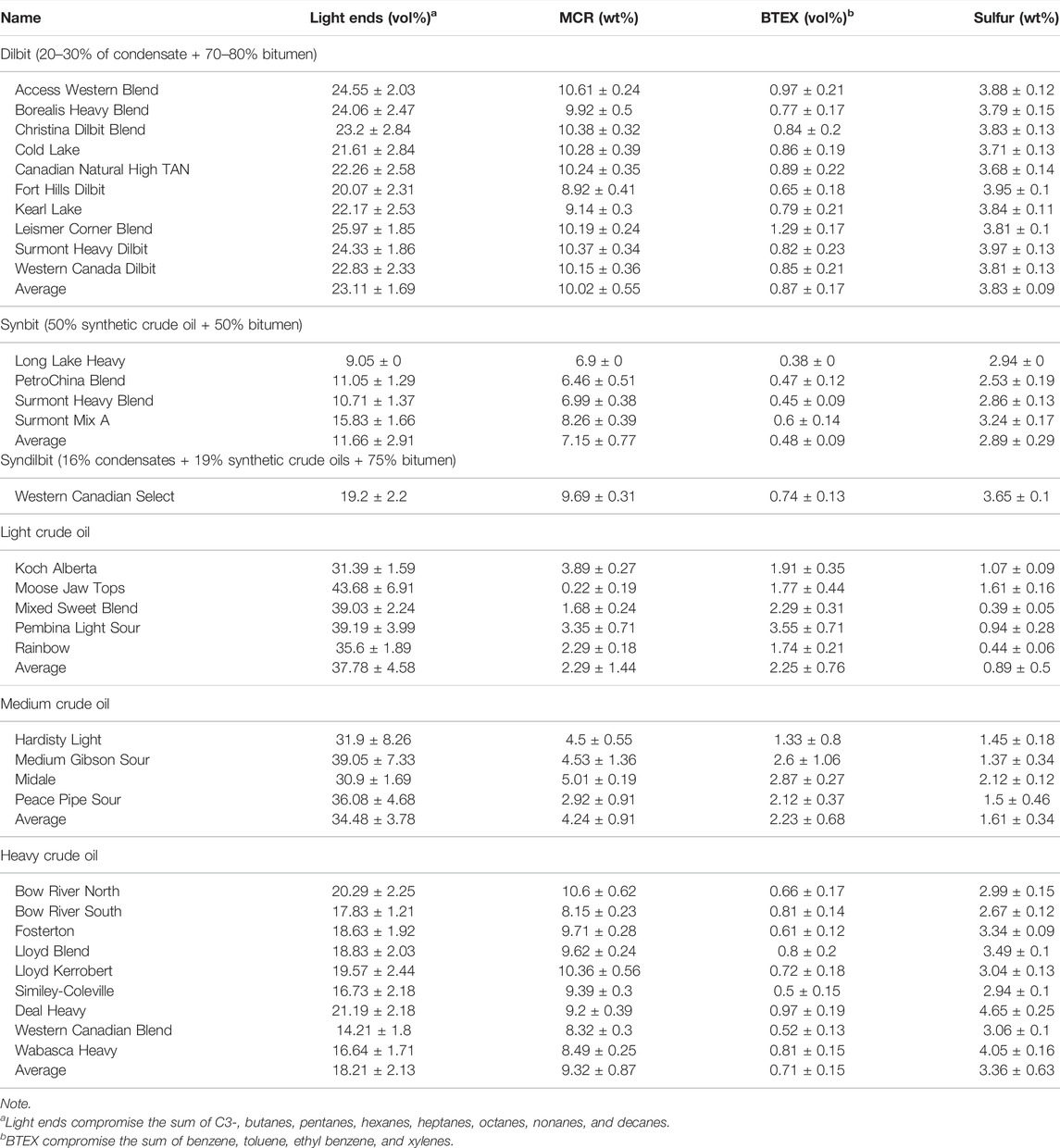
TABLE 3. Chemical properties for diluted bitumen products made in Alberta (CrudeMonitor, 2022).
As per comparisons, the chemical properties of light, medium, and heavy crude oils are also provided in Table 3. It can be clearly observed that both light and medium crude oil have higher contents of light ends and BTEX, but lower contents of MCR and sulfur, compared to dilbit and synbit. Nevertheless, diluted bitumen products are similar to heavy crude oil in chemical properties, including the percentage of light ends, BTEX, MCR, and sulfur content. Especially, the dilbit product has a very similar chemical composition to the heavy crude oils, with almost identical percentages of light ends (∼20 vol%), BTEX (∼1 vol%), MCR (∼10 wt%), and sulfur (∼3 wt%).
Furthermore, another group of volatile components, polycyclic aromatic hydrocarbons (PAHs), is associated with chronic toxicity in aquatic species. Usually, the concentration of PAHs in diluted bitumen is usually lower than that in conventional crudes (Yang et al., 2011; Zhou et al., 2015). In addition, dilbit contains more PAHs than synbit (Sereneo, 2017).
3 Weathering Processes
Weathering is a very important process that could change the physical and chemical properties of oil after a spill happened. The oil weathering processes are usually referring to the spreading, oil evaporation, oil emulsification, natural dispersion, dissolution, photooxidation, biodegradation, sedimentation, etc. (Fingas, 2011). Each process happens at different times along with varied rates as shown in Figure 3 (Lee et al., 2015). For example, evaporation occurs right after the spill, and it is a relatively short process because it happens only when an oil slick is present on the water surface. In contrast, emulsification starts at a relatively later stage, and it is a long-term process (Lee et al., 2015).
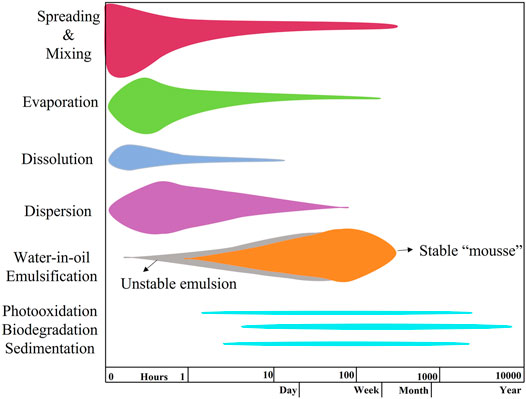
FIGURE 3. The weathering process overtime after the oil is released to the surface of the water (Lee et al., 2015).
To study the weathering processes of bitumen, various analytical methods have been developed based on chromatographic and spectroscopic instruments. A gas chromatography-mass detector (GC-MS) is commonly applied to acquire the chemical composition of bitumen, such as long-chain saturated hydrocarbons, polyaromatic hydrocarbons, etc. A gas chromatography-flame ionized detector (GC-FID) is usually used to quantify the amount of each type of hydrocarbon in bitumen (King et al., 2014; Yang et al., 2018b). For instance, King et al. (2014) used both GC-MS and GC-FID to study the influence of weathering processes on bitumen’s chemical composition in the marine environment.
In addition to chromatographic methods, spectroscopic methods are also frequently applied. Rajib et al. (2021) investigated the chemical composition change of bitumen during oily water treatment based on Fourier transform infrared (FTIR) spectroscopy. The study indicated that water exposure can draw polar compounds to the bitumen surface, leading to a significant increase in absorbance (3,400–3,200 cm−1). In addition, Ren et al. (2009) utilized the X-ray photoelectron spectroscopy (XPS) technique to explore the effect of weathering processes on the chemical composition and wettability of bitumen by Ren et al. (2009).
3.1 Spreading
Spreading is one of the most crucial processes that can increase the contamination area (Lee et al., 2015). The spilled oil spreads quickly to form a thin layer (slick) on the water surface due to the balance between several forces (e.g., gravity, inertia forces, surface tension forces, and viscosity forces) (Iler and Hamilton, 2015). Surface and interfacial tensions are important to determine the final thickness of a spreading oil film (Environment Canada, 2013). The effect of gravity could be gradually diminished overtime, and the oil continues to spread under the effect of interfacial tension (Fay, 1969). The spreading usually decreases with time due to the processes of evaporation, dispersion, and dissolution. The spreading process stops when the thickness of the slick is about 10–3–10–2 cm (Fay, 1969; Wang et al., 2008).
The spreading rate of released oil is determined by environmental conditions (e.g., temperature, water currents, tidal streams, and wind speeds), spill type, and oil properties (Lehr, 2001; Wang et al., 2008; Dew et al., 2015; Lee et al., 2015). For example, the increased temperature can reduce the interfacial tension of oil and thus increase the oil spreading rate. Oil spreads faster in warm water than in cold water (Lee et al., 2015). Spilled oil is likely to spread rapidly in open water to cover the large areas of the water surface (Lee et al., 2015). In confined water, oil cannot spread out and lead to the high risk of long-term damage (Michel and Rutherford, 2014). If surface ice is present in the spill area, the oil spreading rate will be significantly reduced (Stantec Consulting Ltd., 2016). Moreover, Stoyanovich et al. (2021) conducted a series of in situ experiments to investigate the behavior of diluted bitumen in freshwater. They indicated that the spreading rate of diluted bitumen would be higher on low wind days than that on high wind days due to the restriction of limnocorral.
In terms of oil properties, the spreading rate relies on the concentration of low molecular weight hydrocarbons (volatile components). For example, the light crude oil contains high proportions of volatile components and thus can quickly spread on the water surface (Lee et al., 2015). The spreading rate also highly depends on the viscosity of the oil (Fingas, 2015). Oils with low viscosity usually spread quicker than those with high viscosity. Subsequently, bitumen usually spreads much slower than conventional oils due to its low percentage of volatile components and high viscosity.
3.2 Evaporation
Evaporation, which significantly contributes to the growth of density, is normally considered as one of the most important weathering processes. The amount of oil remaining on water strongly relies on evaporation. The evaporation rate is greatly dependent on the composition of volatile components in oil. The evaporation rate is usually high for the oil with a high percentage of volatile components (Fingas, 2015). After a couple of hours of evaporation, most of the light components are evaporated and the remaining light components tend to disperse into the water column. Furthermore, the remaining heavier components can combine with sediments in the water column and submerge/sink (Walker et al., 2016).
Temperature has a profound impact on the evaporation rate of spilled oil (Environment Canada, 2013; Yarranton et al., 2015; Johannessen et al., 2020; Ortmann et al., 2020). Yarranton et al. (2015) conducted several tests to investigate and compare the evaporation rate and the physical property changes of dilbit and light crudes in freshwater. The Cold Lake Winter Blend (CLWB, dilbit) and Alberta Sweet Blend (ASB, light crude oil) were selected to measure the evaporation rates at temperatures of 5°C, 15°C, and 25°C with different air flow rates. The results indicated that the highest evaporated portion of CLWB was about 22% at 25°C, which was lower than ASB (40%) under the same condition. Furthermore, the diluents could evaporate at all temperatures, while the heavier compounds started to evaporate when the temperature reached 60°C (Yarranton et al., 2015). Similar results of evaporation rate were found by Environmental Canada (2013). The IFO (intermediate fuel oil) 180 was used as a reference crude oil in pan evaporation tests. At 15°C seawater, the evaporation rate of AWB was 15.9%, followed by CLB (11.7%) and IFO 180 (0.85%) in the first 6 h. In the following 24 h, the AWB, CLB, and IFO 180 lost 18.4, 15.6, and 1.75% of their mass, respectively. The mass loss of IFO 180 was less than 5%, while the mass loss of both the AWB and the CLB was more than 20%. Moreover, the AWB appeared to have a higher evaporability than the CLB (Environment Canada, 2013). A relatively long-term (14 days) experiment was conducted by Ortmann et al. (2020) to simulate the spills of diluted bitumen products in a coastal area with different seasonal conditions. This study indicated that the warmer air temperature in summer and autumn would enhance the evaporation rate. Moreover, it was noticed that the relatively thick slicks would reduce the evaporation rate (Ortmann et al., 2020).
Niu et al. (2016) conducted stochastic modeling of oil spills in the Salish Sea and compared the evaporation rate of dilbit and conventional crudes. The results illustrated that in the same scenario for 15-day duration, light crudes represented the highest evaporation rate (34.8%), followed by dilbit (16.9%) and heavy crudes (2.4%). This result was consistent with the portions of the light end composition in these oils. Furthermore, Hospital et al. (2015) studied the dilbit spill in the Westridge Terminal, the Port Mann Bridge in the Fraser River, and the other locations (Strait of Georgia, Arachne Reef, Race Rocks, and Buoy J.) along the shipping route. The H3D model (hydrodynamic model) and the SPILLCALC model (oil trajectory and weathering model) were applied in the study for spill simulation. The results indicated that the evaporation rate of dilbit ranged from 15 to 20% in all locations along the shipping route. The evaporation rates were stable throughout the year in the Westridge Terminal (19.4% on average), while they were highest in winter (11%) in the Fraser River (10% on average). It concluded that both temperature and water system might affect the evaporation rate of dilbit (Hospital et al., 2015).
3.3 Emulsification
Emulsification is the process of one liquid dispersing into another one in the form of small droplets (Fingas, 2015). The formation of water-in-oil emulsions can change the physical properties of spilled oil, such as viscosity. As found by Fingas and Fieldhouse (2004), emulsions can be classified into four groups: stable, meso-stable, entrained, and unstable. The unstable emulsion contains only a small portion of water and has a broader range of initial properties (Fingas, 2010). As shown in Table 4, the stability of an emulsion is associated with the composition of resin and asphaltenes. Asphaltenes can stabilize water-in-oil emulsions (Czarnecki et al., 2013). However, excess resin content with an asphaltene/resin ratio higher than 0.6 can destabilize the emulsion. Viscosity also affects the formation of emulsions. The high viscosity limits dispersion and thereby restricts the amount of water coalescing (Lee et al., 2015). Salinity and pH are the other two factors causing impacts on emulsification. The emulsification rate is relatively high at low salinity and low pH (Hounjet et al., 2018). The emulsions can only be formed after evaporation which increase the asphaltene and resin compositions as well as the oil’s viscosity (Belore, 2004; Fingas, 2015). Emulsification increases the volume of the surface slick, while evaporation reduces it (Fingas, 2010). Emulsification also increases the viscosity of oil by up to 1,000 times, hindering the spreading and evaporation of oil (Fingas, 2010).
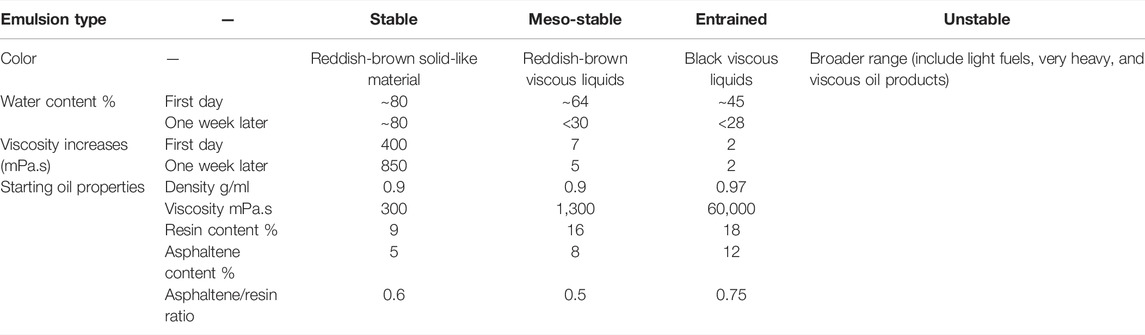
TABLE 4. Characteristics of four emulsion types (Fingas, 2010).
Hounjet and Dettman (2016) conducted lab-scale rotary agitator tests to examine the emulsion formation tendencies of diluted bitumen in both fresh and saltwater, with and without sediment conditions, respectively. The authors determined the stability of the emulsion by visual inspection of phase layer thickness. Phase layer thickness >5 mm suggested being a stable emulsion or water-entrained oil phase. Four oil samples (two dilbits, one synbit, and one conventional crude oil) were used in these tests. The results indicated that under the condition of freshwater without sediment, all of the oil samples created the thickest emulsion layers, and the tendency of emulsion formation of synbit was similar to that of dilbit. Under the condition of saltwater with sediment, tar balls were formed and sank to the bottom of the jar. The conventional crude oil formed less quantity of small tar balls than the diluted bitumen products. The quantities of tar balls are similar for different diluted bitumen products (Hounjet and Dettman, 2016). Environmental Canada (2013) tested the emulsification of fresh and weathered dilbits (CLB and AWB) in an outdoor wave tank at 8°C with seawater (3.3% NaCl). The results indicated that a meso-stable emulsion (contained 72% of water) was found in the case of fresh CLB dilbit. The weathered CLB dilbit (up to 26.5% of evaporation) and AWB dilbit (up to 25.3% of evaporation) could form entrained emulsions (Environment Canada, 2013). In addition, the experiments conducted by Hounjet et al. (2018) indicated that the water content in dilbit emulsions was significantly greater than that in light crude oil (Hounjet et al., 2018).
In general, Fingas (2015) demonstrated that bitumen and weathered dilbit did not form emulsions, while fresh- and light-weathered dilbit formed unstable or/and entrained emulsions in turbulent water condition. Lee et al. (2015) also indicated that the emulsification was not observed for weathered dilbit. These conclusions were consistent with the modeling study of the CLB spill in seawater. The modeling results illustrated that the CLB could not form stable emulsions even after evaporating 17 vol% of oil and in cold water condition (Belore, 2010).
3.4 Photooxidation and Biodegradation
Photooxidation and biodegradation are two important processes involved in the decomposition of the spilled oil (Dutta and Harayama, 2000). The photooxidation process usually occurs around oil slicks in highly oxygenated and intense solar radiation coastal waters (Lee, 2003). This process could lead to the formation of various oxygenated hydrocarbons (Lee, 2003; Fingas, 2010). Prince et al. (2003) suggested that the polar components, such as resins and asphaltenes, in petroleum products were not subject to significant photooxidation damage and were not biodegradable. Subsequently, these components were more likely to remain in the environment for a long time (Prince et al., 2003). Biodegradation is a process in which petroleum hydrocarbons are decomposed with the assistance of bacteria, fungi, and yeasts (Fingas, 2010). Most biodegradation processes happen under aerobic conditions (Atlas and Hazen, 2011). The characteristics of hydrocarbons are influential on the biodegradation rate (Fingas, 2010). For example, light crudes have higher biodegradation rates than heavy crudes due to their higher composition of resins, hopanes, polar molecules, and asphaltenes (Atlas and Hazen, 2011). Both photooxidation and biodegradation processes oxidized the hydrocarbons. To investigate the relationship between photooxidation and biodegradation, Dutta and Harayama (2000) evaluated the effects of photooxidation and biodegradation on the Arabian light crude oil and investigated the relationship between these two processes. The results indicated that 28% of crude oil seawater could be degraded within 8 weeks at 20°C, with sufficient application of nutrients to support microbial growth. Photooxidation mainly affected the aromatic compounds in the crude oil by converting them into polar substances, which increased the amount of biodegradable crude oil components.
Yang et al. (2016b) conducted an experiment to investigate the photolytic behavior of diluted bitumen and compared its photooxidation ability to conventional crudes. Two dilbit products (AWB and CLB) and the Alberta Sweet Mixed Blend #5 (ASMB #5) were exposed to natural light (in the Northern Hemisphere, Ottawa, Canada) in 3.3% NaCl salt water for 90 days in winter and summer, respectively. The results indicated that aromatic hydrocarbons, particularly PAHs and their alkylated homologs (APAHs), were the most photosensitive compounds in the sample oils. The n-alkanes were observed to be the second-fastest photo-oxidized components, followed by terpenes and steranes. The chemical structure of petroleum hydrocarbons and specific oil properties affected the photooxidation rate, in which conventional crude oils appeared at faster photolytic rates than diluted bitumen. The solar radiation and temperature could affect the photolytic behavior as well; high intense solar radiation and/or temperature could lead to high photolytic rates (Yang et al., 2016b).
The compositions of petroleum products determined the type of microbe to metabolize the oil and influenced the biodegradation rate (Westlake et al., 1974). The most biodegradable compounds were saturated hydrocarbons, especially the hydrocarbon numbers between C12 and C20 (Fingas, 2015). Dilbit products are the mixture of diluents containing hydrocarbons between C5 and C12, and bitumen that consists of hydrocarbons greater than C30. Therefore, dilbit usually does not contain hydrocarbons between 12 and 30 (Banerjee, 2012a) and is difficult to be biodegraded compared to conventional crudes. When dilbit has a similar chemical composition to conventional crudes, such as Western Canadian Select and Prudhoe Bay Crude, they appeared similar in biodegradability of alkane, polycyclic aromatic hydrocarbon (PAH), and total extractable hydrocarbon (TEH), even though the overall biodegradation rate of conventional crude oil was still higher than dilbit (Deshpande, 2016). Furthermore, temperature also affects the biodegradation rate by influencing the microbial communities and oil properties at the same time. A high temperature could promote the biodegradation process (Lee et al., 2015; Deshpande, 2016), and the optimal temperature for the maximum biodegradation rate was about 15–20°C in seawater and 20–30°C in freshwater (Lee et al., 2015).
3.5 Sinking
Oil density is essential in sinking behavior. Most conventional crude oils and diluted bitumen products are less dense than water and thus would not sink in a water environment (Short, 2013). Diluted bitumen products would not sink to the seafloor even if saturated with water because the density of raw bitumen is lower than that of seawater (Environment Canada, 2013; King et al., 2014). The experimental results also suggested that dilbit was not likely to sink in the low-energy marine environment (Lee et al., 2015). This was consistent with the modeling results from Niu et al. (2016) that most of the leaked heavy crude oil and dilbit in the Salish Sea remained on the surface and accumulated on the coastline rather than in the water column (Niu et al., 2016). Nevertheless, the spilled diluted bitumen was likely to sink under the low salinity conditions. Johannessen et al. (2020) reviewed the experimental and modeling results about diluted bitumen properties to discuss and predict the transport and fate of diluted bitumen spill on the B.C. coast. The authors indicated that the highly weathered AWB dilbit could sink in low salinity areas (Johannessen et al., 2020). In addition, the sinking ratio of diluted bitumen products was changing with seasonal conditions in saltwater. The sinking ratio was higher in summer than in winter because of the lower surface water density and oil-water interfacial tension in summer (Wu et al., 2016).
Most of the dilbit remained floating when the spill happened in freshwater, some of the dilbit stuck to the beach, and little of them stuck to the window throughout the duration of the test. No sediment was observed when cleaning the tank (Zhou et al., 2015). Furthermore, the study from Yarranton et al. (2015) indicated that the weathered dilbit could afloat on freshwater for at least 10 days. This was in agreement with experimental results from SL Ross Environmental Research Limited (2012) that the weathered dilbit continued to float on the freshwater surface during the whole experiment duration (13 days). However, it was reported that the heavier fractions in dilbit were able to sink (Swift et al., 2011). In contrast, the density of weathered heavy oils was always approaching, but not exceeding, the density of freshwater (National Academies of Sciences and Medicine, 2016).
The formation of oil–particle aggregates is another process that can affect the sinking of oil. After oil spills onto water, it can stay on the water surface or/and be broken up into oil droplets by high energy wave motion. The oil droplet can interact with suspended particles in marine environments to form oil–mineral aggregates (OMAs) or/and interact with fine sediment particles to form oil–particle aggregates (OPAs) (Niu et al., 2011; Wu et al., 2016). The OPAs/OMAs are possibly denser than water and can sink to the bottom. Recently, Ji et al. (2021) conducted a set of laboratory experiments to analyze the formation of OMAs. They indicated that the collision efficiency between the oil and the sediment with higher mixing energy (200 rpm) was three times higher than that with lower mixing energy (150 rpm). The high mixing energy leads to the rapid formation of OMAs in the first 3 hours, followed by the breakup of OMAs. The authors also indicated that the dispersed OMAs reaggregated only under low mixing energy conditions due to a large number of oil droplets on the water surface (Ji et al., 2021). Furthermore, the influence of clay types on OMA structures and settling velocities were investigated by Ye et al. (2020). The OMA droplet was produced with low stickiness kaolinite clay, and its settling velocities were much smaller than the pure kaolinite flocs. On the other hand, flack/solid OMA was generated with high stickiness Bentonite clay, resulting in greater settling velocities (by 25%) than the pure bentonite flocs (Ye et al., 2020).
The United States Environmental Protection Agency (US EPA) reported the observation of bitumen-sediment aggregates in the Kalamazoo River, which was very similar to the laboratory results. The spilled diluted bitumen formed bitumen-sediment aggregation rapidly and kept it stable over the observation period (48 h) (Lee et al., 2012). The probability of oil–particles-aggregation formation or/and sinking for diluted bitumen products depends on their chemical composition. For example, a high concentration of methylated polycyclic aromatic hydrocarbons (M-PAHs) in diluted bitumen products can lead to extreme weathering processes or/and interaction with suspended particles, increasing the oil density and ultimately reaching the point of sinking (King et al., 2014). Usually, the quantity of OMAs is positively correlated with sediment concentration and negatively correlated with particle size (Ajijolaiya et al., 2006). The sinking rate of dilbit products (e.g., AWB and CLB) highly depends on the composition of medium-to-fine sized sediment in the water column. The fresh to moderately weathered diluted bitumen products can sink in seawater when mixed with fine-size and medium-size sediments (Environment Canada, 2013). Hua et al. (2018) conducted a series of experiments to investigate the OMA size distribution for diluted bitumen, light crude, medium crude, and heavy crude oil. The dilbit OMAs could be formed in the presence of kaolinite fines with size <5.8 microns and natural sediments with a size of 2–63 microns in the high-concentration (>1,000 mg/L) experiments (Hua et al., 2018). However, the high viscous oil (after a high degree of evaporation) did not readily interact with the same size of sediments, instead forming the discrete floating tar balls (Environment Canada, 2013). Moreover, water condition is influential in the formation of OMAs as well. Dilbit released into fast-flowing and/or turbulent rivers resulted in a higher proportion of sinking than that of calm water (Dew et al., 2015). This was consistent with the experimental data from Hua et al. (2018) that dilbit OMAs were not formed in wave tank experiments with calm water conditions and low particle concentration (10–100 mg/l) but were formed in the small-scale laboratory experiments with 15 mg/L of particles (Hua et al., 2018).
3.6 Toxicity to Aquatic Organisms
Spilled oil in water conditions causes both acute and/or chronic effects on species and ecosystems (Alsaadi et al., 2018; Ruberg et al., 2021). After the oil is released, species on the shoreline may asphyxiate due to the floating oil which hinders the photosynthesis and reoxygenation processes. Birds and mammals might lose their abilities of thermal insulation and buoyancy result from oiling (Lee et al., 2015). Some chemicals in dilbit (metals, naphthenic acids PAHs, etc.) are known as toxic to fish (Kennedy, 2015). Manzetti (2012) evaluated the impacts of PAHs, aromatic amines, and nitroarenes on the Fjord ecosystems (Manzetti, 2012). The results indicated that all these compounds had the characteristics of inducing DNA damage after metabolic activation. Certain PAHs, as a probable base of their carcinogenic mechanisms, also exert hormonal structural similarities (Manzetti, 2012). The occurrence of naphthenic acids is not mutagenic or carcinogenic but are cytotoxic and suspected endocrine disruptors (Headley and McMartin, 2004).
The Royal Society of Canada Expert Panel had discussed the toxicity of crude oils, indicating that light crudes are more toxic (contain more PAHs) to aquatic species, followed by medium crudes and heavy crudes (Lee et al., 2015). To compare the toxicity of dilbit with conventional crudes, several experiments were conducted. For example, embryos of Japanese medaka (Oryzias latipes) were selected to evaluate the toxicity of fresh dilbit (i.e., AWB) (Madison et al., 2015). The fish species at the early stage are particularly vulnerable due to the inability to avoid contact with oil and high sensitivity to toxicity (Carls et al., 2000). The results indicated that exposure of AWB did not kill the fish but resulted in a high probability of BSD occurrence as well as abnormal swimming and/or non-inflation during hatching. In comparison with conventional crudes, the BSD prevalence was increased with concentration when exposed to both AWB and conventional crudes (Madison et al., 2015). Hereafter, embryos of the same species, Japanese medaka, were used to test the toxicity of CLB by Madison et al. (2015). The results illustrated that the toxicity effects of CLB were similar to AWB and conventional crudes. However, during chronic exposure, CLB was 6–10 times more toxic to hatched medaka than AWB dilbit.
Another species, zebrafish (Danio rerio), was studied to compare the toxicities of dilbit and two conventional crude oils (Medium Sour Composite and Mixed Sweet Blend) (Philibert et al., 2016). The results indicated a similar prevalence of yolk sac edema and a similar influence on continuous swimming behavior. However, the dilbit exposure showed the lowest mortality and pericardial edema. It was, therefore, concluded the toxicity of dilbit to zebrafish was less than or similar to that of conventional crudes. The authors also indicated that zebrafish were more sensitive to BTEX than to PAHs based on the prediction of lethality and pericardial edema (Philibert et al., 2016).
Oil spill effects on the aquatic ecosystem are difficult to measure, which are highly related to the location of the oil spill and its environment. For example, the total volume of oil released into freshwater is usually less than marine spills, but they may cause a more significant impact due to the insufficient volume of water to dilute and degrade the oil. Coastlines and sediments are more susceptible to contamination, and there is less time to control the spread of oil before it contaminates sensitive habitats (Lee et al., 2015).
4 Emergency Responses
4.1 Diluted Bitumen Spill Modeling
The first type of oil spill model is the oil weathering model to simulate the processes that change the oil’s behavior as well as the changes in oil properties during these processes. One of the widely used oil weathering models is the ADIOS oil weathering model, which mainly includes the evaporation, dispersion, emulsification, spreading, and sedimentation processes. This model is also capable of evaluating the effect of cleanup technologies (Lehr et al., 1992, 2002). Another commonly used model is the IKU oil weathering model, which is also known as the SINTEF OWM. The IKU model was developed based on small and mesoscale laboratory experiments and full-scale on-site tests (Daling et al., 1997; Reed et al., 2004). The main weathering processes of the IKU model include emulsification, natural dispersion, and evaporation. Furthermore, a pseudo-component approach is applied in the evaporation simulation, and Mackay’s equation is used to simulate the viscosity change (Mackay et al., 1980a, 1980b; Aamo et al., 1993).
The other types of models are trajectory models to simulate the location change of the spilled oil due to hydrodynamics/ocean dynamics and environmental conditions. The oil trajectory models can be classified into two-dimensional (2D) and three-dimensional (3D) models (Reed et al., 1999b). A 2D model can only simulate the spilled oil trajectory on the surface of the water, while a 3D model can provide the simulation of spilled oil trajectory on the water surface and in the water column. The oil spill trajectory models are usually developed based on Eulerian, Lagrangian, and Eulerian–Lagrangian methods. Currently, the Lagrangian methods are the most commonly used method for the development of 3D oil spill trajectory models (ASCE, 1996; Reed et al., 1999b; Spaulding, 2017).
The weathering and trajectory models are commonly integrated to provide a comprehensive prediction of oil spills and facilitate oil spill management. Recently, several models have been developed or integrated for oil spill transport and fate simulation, such as the Oil Spill Contingency and Response (OSCAR) model (Reed et al., 1995, 1999a, 2004; Aamo et al., 1997; Nordam et al., 2020; Barreto et al., 2021); the OILMAP™ model (Mackay et al., 1980a; Mackay, 1980; Toz and Buber, 2018; Chen, 2021; RPS-ASA, 2022); the General National Oceanic and Atmospheric Administration (NOAA) Operational Modeling Environment (GNOME) with the Automated Data Inquiry for Oil Spills (ADIOS2) (Beegle-Krause, 1999, 2001; Elizaryev et al., 2018; Torres et al., 2020); and the OpenDrift model integrated with the integrated oil spill transport and fate sub-module (OpenOil) (Dagestad et al., 2018; Röhrs et al., 2018; Keramea et al., 2022). The list of currently used oil spill models can be found in Table 5.
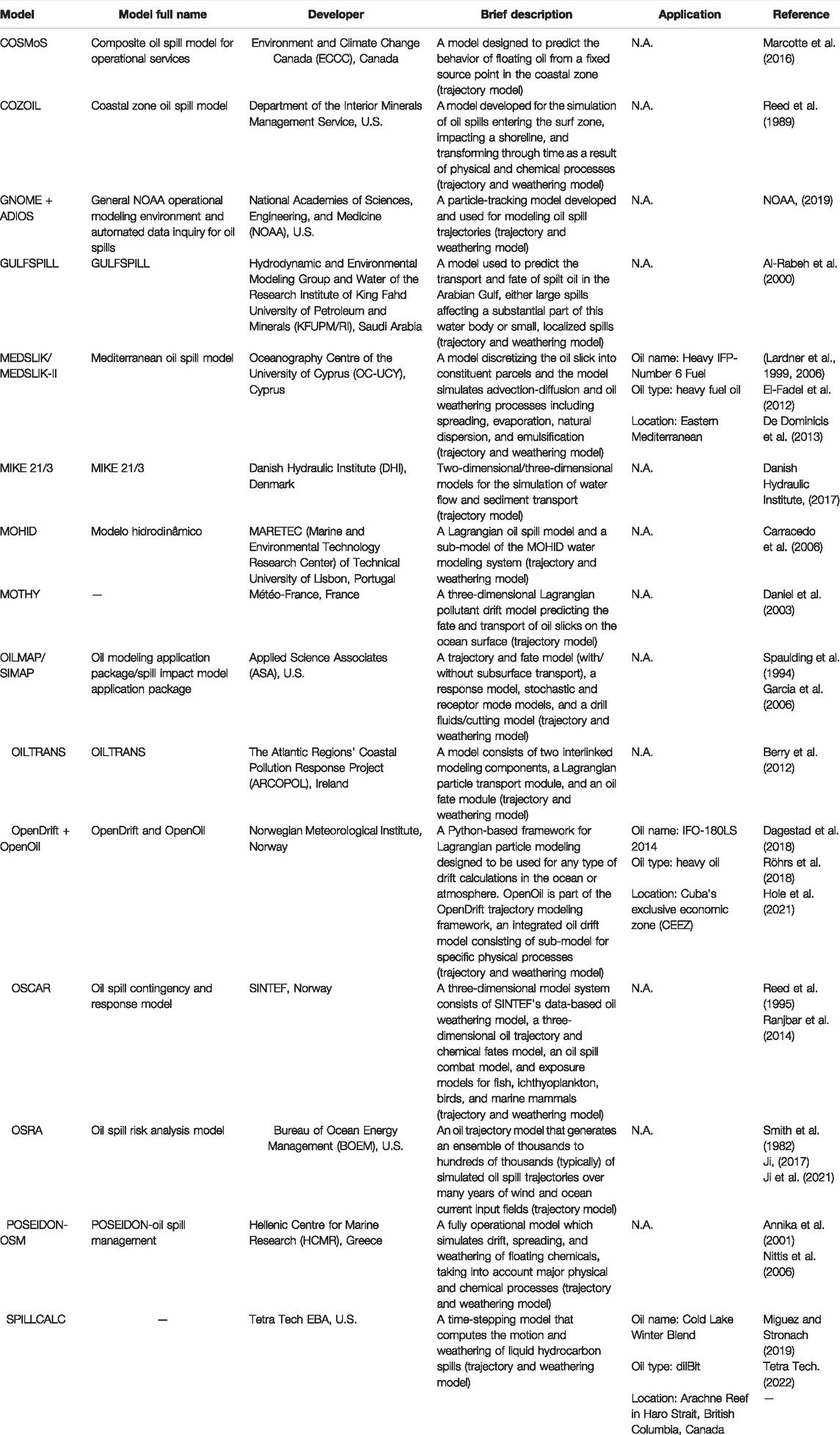
TABLE 5. Commonly used oil spill models and their application in dilbit, unconventional, or heavy oil spills.
4.2 Containment and Collection of the Spilled Oils
Based on the spilled oil modeling, further oil spill response actions such as containment/recovery are needed to deal with the spill onsite. Containment of an oil spill is the method to restrict the spread of oil or prevent the oil from spreading to a particular area (Fingas, 2010). Containment equipment can be used to concentrate the oil and allows for the subsequent recovery, removal, dispersal, etc. The floating barrier, called boom, is the key equipment to control the oil spreading and make recovery easier.
Spilled oil recovery is usually achieved by employing a mechanical skimmer and sorbent materials. Skimmers are mechanical devices designed to remove oil from the surface of the water. They vary widely in size, application, capacity, and recovery efficiency. There are different types of sorbents, including natural/organic sorbents (e.g., hay, sawdust, and straw), mineral/natural inorganic sorbents (e.g., zeolites, activated carbon, clays, and volcanic ash), and synthetic polymeric sorbents (e.g., polyethylene, polyurethane, and polyvinyl chloride) (Dhaka and Chattopadhyay, 2021). These sorbent materials appear to have distinct wetting ability, rate of retention of liquid, sorption ability, and density. It is worth noting that the synthetic polymeric sorbents are widely reported to be superior to other sorbents in terms of the oil recovery efficiency. For example, Saleem et al. (2015) utilized high-density polyethylene (HDPE) bottle to produce an oil-sorbent film and observed a high oil uptake capacity (up to 100 g/g) after floating on the oil for 5 min (Saleem et al., 2015). On this basis, Saleem and McKay (2016) investigated the selective sorption by immersing the film in the oil–water mixture and reported a high selectivity (the oil sorption capacity of 50 g/g) for the oil–water mixture that contained 1.5% oil (Saleem and McKay, 2016).
Wu et al. (2014) studied the oil sorption capacity, oil/water selectivity, and reusability of polyurethane (PU) sponges and reported that it is a promising candidate for spilled oil cleanup applications with high sorption capacity and excellent reusability (Wu et al., 2014). Sorbent for oil spill cleanup was obtained by treating PU sponges with silica sol and gasoline, and its sorption capacity for motor oil was determined to be more than 100 g/g which is 10 times higher than that of commercial polypropylene (PP) sorbent. Aboul-Gheit et al. (2006) used polyethylene (PE) and PP waste powders and sheets as oil sorbents by a simple contacting mixture of oil and fresh or seawater for a few minutes and reported that the plastic powder of PE and PP showed high sorption on light and heavy crude oil with around 90 g/g (Aboul-Gheit et al., 2006). They also claimed that compared with the natural sorbents, oil can be separated easily, and the recovery was much larger from PE and PP. Atta et al. (2013)used porous PU foams to sorb crude oil and toluene and reported that their highest sorption capacity of crude oil could be 40 g/g.
Chemical and biological methods can be used in conjunction with mechanical means for containing and cleaning oil spills. A dispersant is a commonly used chemical that helps prevent oils from reaching the shoreline and other sensitive habitats. The biological method relies on microorganisms to degrade the spilled oil molecules, which could be particularly useful to restore the environmental quality for sensitive areas such as coastlines, marshes, and wetlands (US EPA, 2018). Dispersant applications, as a non-mechanical response technique, involve the use of a variety of chemicals that are designed to promote the breakup of the oil film and disperse it into small droplets suspended in a water column. Some of the early dispersants include relatively toxic hydrocarbon solvents and are used in large doses. Today, the toxicity of dispersants is relatively low and is usually used at a dispersant-to-oil ratio of 1:20 to 1:50 or less (Fingas, 2011). By using remote sensing technology, it significantly helps to detect the thickness of oil slicks on the water surface and guides the responders to determine the amount and locations to use the dispersants (Fingas, 2010).
The process of burning floating oil at sea or in the area close to the spill site is called in situ burning (ISB). The oil must be concentrated, and an ignition source is needed to undertake the burning. Under ideal conditions, ISB can significantly reduce the amount of oil on the water surface and minimize the adverse effects of the oil in the environment (Buist et al., 1999). However, the ISB could lead to secondary fire and produce toxic smoke that impacts human health and the surrounding environment. Each countermeasure technology has its own advantages and disadvantages. Decision-makers could choose the most economical and effective methods according to the spilled oil types, environmental conditions of the oil spill site, and the analysis from oil spill modeling based on GIS and remote sensing. More detailed and systematic information on spilled oil containment and recovery can be referred to Dave and Ghaly (2011) and Dhaka and Chattopadhyay (2021).
5 Recovery/Reutilization of Oily Wastes
5.1 Disposal/Treatment of Collected Oily Waste
After the spilled oils are physically collected, different methods have been explored to dispose/treat the collected oil–water mixture. Manzetti (2012) used the biofilm-membrane bioreactor to treat the high-salinity oily wastewater and proved the feasibility of using salt-adapted microorganism for degrading the pollutants (Mancini et al., 2012). Cappello et al. (2016) obtained forty-two strains during the microbiological screening of a membrane bioreactor system. All bacterial strains were grown in 1% diesel oil for one week. The strains of BS, PG-12, and PG-11 exhibited the highest levels of diesel biodegradation (82, 71, and 68%, respectively). This observation confirmed the high activity of bacteria related to the genera Alcanivorax, Rheinheimera, Rhodococcus, and Muricauda and underlined the possible application of these bacteria in the remediation of saline oily wastewater. Tanudjaja et al. (2019) summarized the sources, compositions, and regulations related to the practical implementation of membranes for the treatment of oily wastewater. Different commercially available membranes, membrane modules, operation modes, and their economics were elaborately discussed as well.
Recently, the management framework for treating the spilled oil has been explored. Saleem et al. (2022) presented a novel life cycle assessment (LCA)-based framework for low-impact offshore oil spill response waste (OSRW) management. The factorial design considered all possible combinations of OSRW quantities, compositions, and treatment/disposal technologies, and chemical demulsification exhibited the highest total impacts before of its high marine ecotoxicity and human toxicity. Chen et al. (2021) combined an inexact inventory theory-based optimization model (ITOM) with the economic order quantity model for an oily waste management system, which could provide the optimal solutions for batch size and order cycle for treatment facilities. The most appropriate facilities and optimal waste allocation scheme under uncertainties can be selected to achieve the minimum total system cost.
Jamaly et al. (2015) reviewed the oily wastewater treatment methods, including electrochemical treatment, membrane filtration, biological treatment or hybrid technologies (biological membrane reactor), use of biosurfactants, treatment via vacuum ultraviolet radiation, and destabilization of emulsions using zeolites and other natural minerals. They pointed out that there is an increasing need to devise new sustainable approaches for oily wastewater treatment that will be geared toward economic savings and environmental preservation. Biological processes are time-consuming and require highly expensive enzymes. Furthermore, more attention should be focused on extremely toxic contaminants that may be present in oily wastewater such as radionuclides, persistent organic pollutants, and polyaromatic hydrocarbons.
5.2 Waste to Wealth
Although many attempts have been made to treat the oily waste, mostly high salinity oily wastewater by membrane technologies (Tanudjaja et al., 2019), rare effort has been contributed to reutilize the collected oil–water mixture or the oil-containing sorbent materials. There are different biowaste conversion technologies that most aim to produce biofuels. Thus, this section will briefly introduce biowaste conversion technologies, followed by evaluating which one is most suitable for valorizing recovered oily waste into value-added products.
5.2.1 Biowaste Conversion Technologies
Different biowaste conversion technologies have their own target products, suitable feedstocks, and process requirements as presented in Table 6. The physical method refers to the densification for pellet/briquette production, in which lignocellulosic feedstocks in the form of particles/chips are concentrated to pellets/briquettes by machines. The main purpose of densification is to increase bulk density for transportation and energy density for utilization (Thompson and Rough, 2021). Transesterification is used to covert non-edible vegetable oils into biodiesel with the assistance of alcohol and acid/basic catalysts (Veljković et al., 2012). The biological methods mainly refer to the fermentation for bioethanol production and anaerobic digestion for biomethane production (Heimann, 2016). During the fermentation process, glucose from lignocellulosic biomass is broken down by yeast into bioethanol. As for the anaerobic digestion, the organic matters are decomposed by anaerobic bacteria in an oxygen-starved environment to produce biomethane and other gases (e.g., CO2, NH3).
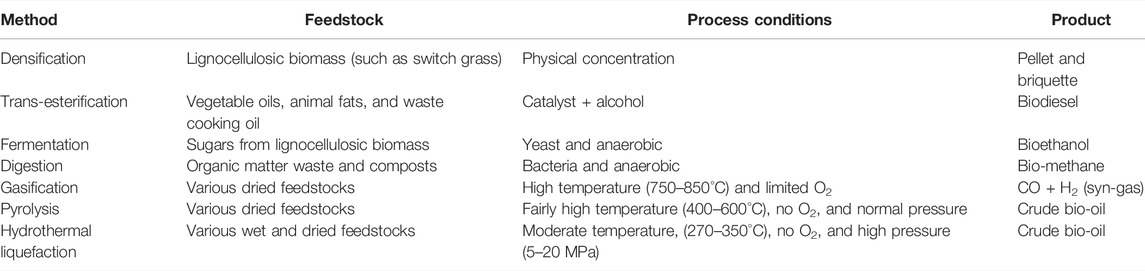
TABLE 6. Biowaste conversion technologies based on feedstock types, process requirements, and target products.
The thermochemical method for biomass valorization includes gasification, pyrolysis, and hydrothermal liquefaction (HTL) (Das et al., 2021). Gasification requires a high temperature (750–850°C) to decompose various dried feedstocks into syn-gas (H2 and CO mixture) with limited oxygen supply, and the obtained syn-gas can be further synthesized into biomethane and other value-added chemicals. The target product from pyrolysis is crude bio-oil (biocrude), in which the feedstocks are rapidly decomposed into biocrude at a temperature between 400 and 600°C in the absence of O2. It is worthwhile mentioning that pyrolysis requires the dried feedstock to obtain high-quality biocrude. Hydrothermal liquefaction (HTL) is an emerging thermochemical conversion technique that can directly process wet feedstocks into crude bio-oil (biocrude) by using subcritical water (temperature of 280–350°C and pressure of 5–20 MPa).
5.2.2 Suitability of Hydrothermal Liquefaction
The recovered oil–water mixture from the oil spill is not suitable for densification, transesterification, and fermentation simply because the oil–water mixture cannot be used as their feedstocks. Gasification and pyrolysis require dried feedstocks to achieve a high-quality target product, preventing the oil–water mixture from being used as their feedstock. Although digestion is suitable for a broad spectrum of organic matters and oil–water mixture from oil spill recovery can be considered as one of them, the presence of polyaromatic hydrocarbons and other toxic compounds in petroleum oils is highly possible to poison/deactivate the bacteria, leading to the failure of the whole digestion system. HTL can process wet feedstocks and is a thermochemical process that does not involve biological species (no poisoning issue); thus, it is the most suitable conversion technology for reutilizing the oil–water mixture from the oil spill.
HTL of biomass for biocrude production has recently attracted much research attention, mainly because HTL utilizes water as a green solvent and the energy-intensive pre-drying of biomass is not required. HTL takes advantage of subcritical water’s physicochemical properties, such as low dielectric constant and high permeability. HTL processing time is usually around 5–50 min and biomass loading is around 5–20 wt% (Peterson et al., 2008). There are four products, including biocrude (target product), hydro-char, water-soluble chemicals (aqueous phase), and gaseous products (mainly CO2). The obtained biocrude can be blended with petroleum crude oil and upgraded into gasoline, diesel, and even aviation fuel.
The HTL technique has been studied by many researchers from different perspectives, for instance, the influence of biomass compositions, reaction temperature, time, and biomass loading on the biocrude yield/quality (Yang et al., 2016a). Catalytic HTL has been investigated as well; the addition of homogenous catalysts (e.g., ethanol, HCl, and KOH) and heterogeneous catalysts (e.g., Pt/C and HZM) could improve biocrude yield/quality (Yang et al., 2019c). It is well known that biomass is composed of lipid, protein, cellulose, hemicellulose, and lignin, and some researchers employed biomass model components to explore the biocrude formation mechanism (Yang et al., 2018a). In addition to using biomass model components, kinetics tools were applied to elucidate the reaction pathways during the HTL process. The developed kinetics equations based on HTL temperature and time can be used to predict the biocrude yield as well (Sheehan and Savage, 2017). A statistical method (mixture design) has been implemented to build biocrude prediction equation, which could include a broader spectrum of process variables compared to the kinetics method (Yang et al., 2019a). In order to increase the economic viability, some researchers attempted to use the aqueous phase for microalgae cultivation or recycle the aqueous phase back to the HTL process with the aim to increase biocrude yield/quality (Chen et al., 2022). Recently, the aqueous phase has been reported to exhibit anti-bacteria/virus activities (Yu et al., 2022). A process intensification technique, microwave irradiation, was also applied for HTL biocrude production (Yang et al., 2020b). The feasibility of the continuous HTL process has been validated (Elliott et al., 2013). Various techno-economic analyses and life cycle assessments have been carried out as well (Chen and Quinn, 2021).
Although progress has been made on HTL of biocrude, there are still two main problems: 1) organic solvents are commonly used to recover the biocrude after HTL conversion, which largely reduces the environmental friendliness; 2) most HTL studies used freshwater as processing medium, making HTL technique greatly reliable on the scarce freshwater resource. Using the collected oil–water mixture from oil spill as an HTL processing medium can readily solve the aforementioned problems: 1) the crude heavy oil in the collected mixture can serve as the organic solvent to dissolve the produced biocrude, thus preventing the use of organic solvent for biocrude recovery; 2) the presence of seawater in the collected solution can lower the amount of freshwater required for HTL of biomass, therefore, reduce HTL reliance on the freshwater resource. It is notable that seawater-based HTL has been proved to be feasible (Yang et al., 2020a). Therefore, HTL is a suitable biowaste conversion technology for reutilizing the recovered oil–water mixture from oil spills.
6 Future Works
Although the physicochemical properties of different diluted bitumen products have been well documented, more research efforts are required to better understand the weathering processes of diluted bitumen. For example, the spreading of diluted bitumen has been barely investigated. In addition, the experimental observations for the emulsification process were inconsistent in different studies. As for the numerical simulation, current oil spill models are mainly developed for conventional oil spill simulation. They may not be capable of simulating diluted bitumen spills due to significant differences in the physicochemical properties and behaviors of diluted bitumen and conventional oils. As indicated in Table 5, the applications of current oil spill models in diluted bitumen spill simulation are very limited. Models aiming at diluted bitumen spill simulation may be needed, especially for the diluted bitumen weathering simulation. Recently, a diluted bitumen weathering model (DBWM) has been developed and validated with meso-scale experimental data to provide a reasonable simulation of diluted bitumen weathering (Li et al., 2022). Nevertheless, an integrated model with diluted bitumen spill trajectory and weathering simulation will be further needed.
How to efficiently reutilize the recovered oily waste is of significant importance for oil spill responses; unfortunately, extremely limited efforts have been contributed to this subject. When the boom and skimmer are employed for oil spill cleanup, the obtained high-salinity oil–water mixture can serve as the processing medium for biomass hydrothermal liquefaction, which could not only solve the organic usage problem but also decrease the reliance on freshwater. The use of synthetic polymeric sorbents (e.g., polyethylene and polyurethane) has been reported to be superior to other inorganic sorbents mainly due to their high oil uptake capacities (as high as 100–150 g/g) and satisfactory buoyant ability that allows for efficient collection. However, the disposal of such plastic materials containing oil is a profound challenge.
It is interesting to note that HTL has recently been employed to dispose of plastics and exhibited promising potential. For instance, Pedersen and Conti (2017) conducted hydrothermal processing of nine different types of plastics and reported high monomer yields (Pedersen and Conti, 2017). Belden et al. (2021) studied the thermodynamic feasibility and subsequent effects of hydrothermally converting ocean plastics into fuel and reported that HTL of ocean plastics is the most promising method to enable ship self-powered cleanup (Belden et al., 2021). In addition, Seshasayee and Savage (2021) conducted co-liquefaction of plastics and biomolecules (e.g., cellulose, starch, and lignin) in both subcritical (300, 350°C) and supercritical (400, 425°C) water and reported that the interactions not only increase the oil yield but also enable HTL to proceed at lower temperatures (Seshasayee and Savage, 2021). The lowest temperature (300°C) witnessed the highest energy recovery (45%) in the oil. As these cases clearly demonstrated that HTL could serve as a promising technique to dispose of the plastic sorbents for oil spill cleanup. Direct processing of the plastic sorbents containing spilled oil in subcritical water conditions is highly recommended in future work.
7 Conclusion
Bitumen can be blended with condensate and/or synthetic crude oil to generate dilbit, synbit, and syndilbit. Dilbit is mostly comparable with heavy crude oil based on their physicochemical properties, such as API gravity, viscosity, light ends (vol%), and MCR (wt%).
Weathering processes are closely related to the temperature, hydrodynamic condition of the spill site, type of oil, etc. To date, a rare study has been conducted to study the spreading of diluted bitumen. Dilbit evaporation rate was around 15–20%, which was slower than light crude oil mainly due to fewer amounts of low molecular weight hydrocarbons in dilbit. The observations for dilbit emulsification were not consistent between studies, and thus more research efforts are required in future works. The photooxidation and biodegradation were highly dependent on the oil chemical compositions, in which diluted bitumen products exhibited faster photolytic rates and biodegradation rates. As for the sinking, dilbit was not likely to sink under the low-energy marine environment. Nevertheless, highly weathered dilbit could sink in the low salinity water environment. In contrast, the density of weathered heavy oils is always approaching, but not exceeding, the density of freshwater. The spilled diluted bitumen might interact with particles to form oil–particle aggregates and, subsequently, sink into the water column. Compared to heavy crude oil, diluted bitumen products have been proved to appear similar toxicity to aquatic organisms.
Although some experimental studies have been conducted to explore the weathering processes of diluted bitumen products, limited efforts have been contributed to numerically simulate the diluted bitumen’s weathering processes and trajectories. Different containment technologies have been developed to restrict the spread of spilled oil, including physical recovery by boom/skimmer/sorbents, chemical dispersion, and in-suit burning. The disposal/treatment of collected oily wastewater remains a profound challenge, and membrane technologies were most commonly implemented to treat the oily wastewater. Unfortunately, a rare attempt has been made to turn the oily waste into value-added products. Among different biowaste conversion technologies, hydrothermal liquefaction was considered to be the most suitable technology for valorizing the collected oily wastes.
Author Contributions
The diluted bitumen’s properties, weathering processes, and numerical modeling parts were written by XZ, PL, and HN; the spill oil collection and treatment were written by ZZ and XL; the reutilization part was written by QH and JY; and this work was constructed and revised by HN and JY.
Funding
This research was funded by the Key Area Research and Development Program of Guangdong Province (project number 2020B1111350003); the financial support is from Minjiang University; Fujian Education Department Young and Middle-Aged Scholar Funding (JAT200447); Marine Environment Observation Prediction and Response Work (MEOPAR, Project 2–02–03–037.3); and Multi-Partner Research Initiative (MPRI, Project 6.02).
Conflict of Interest
The authors declare that the research was conducted in the absence of any commercial or financial relationships that could be construed as a potential conflict of interest.
Publisher’s Note
All claims expressed in this article are solely those of the authors and do not necessarily represent those of their affiliated organizations, or those of the publisher, the editors, and the reviewers. Any product that may be evaluated in this article, or claim that may be made by its manufacturer, is not guaranteed or endorsed by the publisher.
References
Aamo, O. M., Reed, M., and Daling, P. S. (1993). A Laboratory-Based Weathering Model: PC Version for Coupling to Transport Models. Available at: http://inis.iaea.org/Search/search.aspx?orig_q=RN:25063976 (Accessed November 23, 2018).
Aamo, O. M., Reed, M., and Downing, K. (1997). Oil Spill Contingency and Response (Oscar) Model System: Sensitivity Studies. Int. Oil Spill Conf. Proc. 1997, 429–438. doi:10.7901/2169-3358-1997-1-429
Aboul-Gheit, A. K., Khalil, F. H., and Abdel-Moghny, T. (2006). Adsorption of Spilled Oil from Seawater by Waste Plastic. Oil Gas Sci. Technol. - Rev. IFP 61, 259–268. doi:10.2516/ogst:2006019x
Ajijolaiya, L. O., Hill, P. S., Khelifa, A., Islam, R. M., and Lee, K. (2006). Laboratory Investigation of the Effects of Mineral Size and Concentration on the Formation of Oil-Mineral Aggregates. Mar. Pollut. Bull. 52, 920–927. doi:10.1016/j.marpolbul.2005.12.006
Al-Rabeh, A. H., Lardner, R. W., and Gunay, N. (2000). Gulfspill Version 2.0: a Software Package for Oil Spills in the Arabian Gulf. Environ. Model. Softw. 15, 425–442. doi:10.1016/S1364-8152(00)00013-X
Alsaadi, F., Hodson, P. V., and Langlois, V. S. (2018). An Embryonic Field of Study: The Aquatic Fate and Toxicity of Diluted Bitumen. Bull. Environ. Contam. Toxicol. 100, 8–13. doi:10.1007/s00128-017-2239-7
American Society of Civil Engineers (ASCE) (1996). State-of-the-Art Review of Modeling Transport and Fate of Oil Spills. J. Hydraulic Eng. 122122, 59411–59609. doi:10.1061/(asce)0733-9429(1996)122:11(594)
Annika, P., George, T., George, P., Konstantinos, N., Costas, D., and Koutitas, C. (2001). The Poseidon Operational Tool for the Prediction of Floating Pollutant Transport. Mar. Pollut. Bull. 43, 270–278. doi:10.1016/S0025-326X(01)00080-7
Atlas, R. M., and Hazen, T. C. (2011). Oil Biodegradation and Bioremediation: a Tale of the Two Worst Spills in US History. Environ. Sci. Technol. 45, 6709–6715. doi:10.1021/es2013227
Atta, A. M., Brostow, W., Datashvili, T., El-Ghazawy, R. A., Lobland, H. E. H., Hasan, A.-R. M., et al. (2013). Porous Polyurethane Foams Based on Recycled Poly(ethylene Terephthalate) for Oil Sorption. Polym. Int. 62, 116–126. doi:10.1002/pi.4325
Banerjee, D. K. (2012a). “Chapter 2 the Chemistry of Bitumen,” in Oil Sands, Heavy Oil & Bitumen: From Recovery to Refinery (Tulsa, OK: PennWell Corporation).
Banerjee, D. K. (2012b). “Chapter 6 Transportation of Heavy Oil/bitumen,” in Oil Sands, Heavy Oil & Bitumen: From Recovery to Refinery (Tulsa, OKPennWell Corporation).
Barreto, F. T. C., Dammann, D. O., Tessarolo, L. F., Skancke, J., Keghouche, I., Innocentini, V., et al. (2021). Comparison of the Coupled Model for Oil Spill Prediction (CMOP) and the Oil Spill Contingency and Response Model (OSCAR) during the DeepSpill Field Experiment. Ocean Coast. Manag. 204, 105552. doi:10.1016/j.ocecoaman.2021.105552
Beegle-Krause, C. J. (1999). “GNOME: NOAA's Next-Generation Spill Trajectory Model,” in Oceans ’99. MTS/IEEE. Riding the Crest into the 21st Century. Conference and Exhibition. Conference Proceedings (IEEE Cat. No.99CH37008) (IEEE), 1262–1266. doi:10.1109/OCEANS.1999.800172
Beegle-Krause, J. (2001). “General Noaa Oil Modeling Environment (Gnome): A New Spill Trajectory Model,” in International Oil Spill Conference Proceedings 2001 (IEEE), 865–871. doi:10.7901/2169-3358-2001-2-865
Belden, E. R., Kazantzis, N. K., Reddy, C. M., Kite-Powell, H., Timko, M. T., Italiani, E., et al. (2021). Thermodynamic Feasibility of Shipboard Conversion of Marine Plastics to Blue Diesel for Self-Powered Ocean Cleanup. Proc. Natl. Acad. Sci. U.S.A. 118, e2107250118. doi:10.1073/pnas.2107250118
Belore, R. (2004). Analysis of IFO-180 and IFO-380 Oil Properties for Dispersant Window of Opportunity. Technical report submitted to US dept. Interior.
Belore, R. (2010). Properties and Fate of Hydrocarbons Associated with Hypothetical Spills at the Marine Terminal and in the Confined Channel Assessment Area. Calgary, Alberta: Technical Data Report. SL Ross.
Berry, A., Dabrowski, T., and Lyons, K. (2012). The Oil Spill Model OILTRANS and its Application to the Celtic Sea. Mar. Pollut. Bull. 64, 2489–2501. doi:10.1016/j.marpolbul.2012.07.036
Buist, I., McCourt, J., Potter, S., Ross, S., and Trudel, K. (1999). In Situ Burning. Pure Appl. Chem. 71, 43–65. doi:10.1351/pac199971010043
Cappello, S., Volta, A., Santisi, S., Morici, C., Mancini, G., Quatrini, P., et al. (2016). Oil-degrading Bacteria from a Membrane Bioreactor (BF-MBR) System for Treatment of Saline Oily Waste: Isolation, Identification and Characterization of the Biotechnological Potential. Int. Biodeterior. Biodegrad. 110, 235–244. doi:10.1016/j.ibiod.2015.12.028
Carls, M. G., Hose, J. E., Thomas, R. E., and Rice, S. D. (2000). Exposure of Pacific Herring to Weathered Crude Oil: Assessing Effects on Ova. Environ. Toxicol. Chem. 19, 1649–1659. doi:10.1002/etc.5620190624
Carracedo, P., Torres-López, S., Barreiro, M., Montero, P., Balseiro, C. F., Penabad, E., et al. (2006). Improvement of Pollutant Drift Forecast System Applied to the Prestige Oil Spills in Galicia Coast (NW of Spain): Development of an Operational System. Mar. Pollut. Bull. 53, 350–360. doi:10.1016/j.marpolbul.2005.11.014
Chen, J., Zhang, J., Pan, W., An, G., Deng, Y., Li, Y., et al. (2022). A Novel Strategy to Simultaneously Enhance Bio-Oil Yield and Nutrient Recovery in Sequential Hydrothermal Liquefaction of High Protein Microalgae. Energy Convers. Manag. 255, 115330. doi:10.1016/j.enconman.2022.115330
Chen, P. H., and Quinn, J. C. (2021). Microalgae to Biofuels through Hydrothermal Liquefaction: Open-Source Techno-Economic Analysis and Life Cycle Assessment. Appl. Energy 289, 116613. doi:10.1016/j.apenergy.2021.116613
Chen, Y. (2021). Development of an Oil Spill Model Adaptable to Exposure and Submergence Conversion of Tidal Flats: A Case Study in the Changjiang Estuary. Mar. Pollut. Bull. 171, 112715. doi:10.1016/j.marpolbul.2021.112715
Chen, Z., An, C., Chen, X., Taylor, E., Bagchi, A., and Tian, X. (2021). Inexact Inventory-Theory-Based Optimization of Oily Waste Management System in Shoreline Spill Response. Sci. Total Environ. 777, 146078. doi:10.1016/j.scitotenv.2021.146078
Clark, J. H. (2017). From Waste to Wealth Using Green Chemistry: The Way to Long Term Stability. Curr. Opin. Green Sustain. Chem. 8, 10–13. doi:10.1016/j.cogsc.2017.07.008
Crosby, S., Fay, R., Groark, C., Smith, J. R., Sullivan, T., Pavia, R., et al. (2013). Transporting Alberta Oil Sands Products: Defining the Issues and Assessing the Risks. Alberta: NOAA/Office of Response and Restoration.
Crude Quality Inc. (2015). Canadian Crude Quick Reference Guide. Available at: https://www.crudemonitor.ca/tools/Quick_Reference_Guide.pdf.
CrudeMonitor (2022). CrudeMonitor.ca. Available at: https://www.crudemonitor.ca/home.php?PHPSESSID=04905d53884bc900d39c181cd04275b2 (Accessed March 18, 2022).
Czarnecki, J., Tchoukov, P., Dabros, T., and Xu, Z. (2013). Role of Asphaltenes in Stabilisation of Water in Crude Oil Emulsions. Can. J. Chem. Eng. 91, 1365–1371. doi:10.1002/cjce.21835
Dagestad, K.-F., Röhrs, J., Breivik, Ø., and Ådlandsvik, B. (2018). OpenDrift v1.0: a Generic Framework for Trajectory Modelling. Geosci. Model Dev. 11, 1405–1420. doi:10.5194/gmd-11-1405-2018
Daling, P. S., Aamo, O. M., Lewis, A., and Strøm-Kristiansen, T. (1997). “Sintef/iku Oil-Weathering Model: Predicting Oils’ Properties at Sea,” in International Oil Spill Conference Proceedings 1997, 297–307. doi:10.7901/2169-3358-1997-1-297
Daniel, P., Marty, F., Josse, P., Skandrani, C., and Benshila, R. (2003). Improvement of Drift Calculation in Mothy Operational Oil Spill Prediction System. Int. Oil Spill Conf. Proc. 2003, 1067–1072. doi:10.7901/2169-3358-2003-1-1067
Danish Hydraulic Institute (DHI) (2017). DHI Oil Spill Model - Scientific Description. Available at: http://manuals.mikepoweredbydhi.help/2017/General/DHI_OilSpill_Model.pdf.
Das, P., V.P., C., Mathimani, T., and Pugazhendhi, A. (2021). A Comprehensive Review on the Factors Affecting Thermochemical Conversion Efficiency of Algal Biomass to Energy. Sci. Total Environ. 766, 144213. doi:10.1016/j.scitotenv.2020.144213
Dave, D., and Ghaly, A. E. (2011). Remediation Technologies for Marine Oil Spills: A Critical Review and Comparative Analysis. Am. J. Environ. Sci. 7, 423–440. doi:10.3844/ajessp.2011.423.440
De Dominicis, M., Pinardi, N., Zodiatis, G., and Archetti, R. (2013). MEDSLIK-II, a Lagrangian Marine Surface Oil Spill Model for Short-Term Forecasting - Part 2: Numerical Simulations and Validations. Geosci. Model Dev. 6, 1871–1888. doi:10.5194/gmd-6-1871-2013
Dew, W. A., Hontela, A., Rood, S. B., and Pyle, G. G. (2015). Biological Effects and Toxicity of Diluted Bitumen and its Constituents in Freshwater Systems. J. Appl. Toxicol. 35, 1219–1227. doi:10.1002/jat.3196
Dhaka, A., and Chattopadhyay, P. (2021). A Review on Physical Remediation Techniques for Treatment of Marine Oil Spills. J. Environ. Manag. 288, 112428. doi:10.1016/j.jenvman.2021.112428
Dollhopf, R. H., Fitzpatrick, F. A., Kimble1, J. W., Capone, D. M., Graan, T. P., Zelt, R. B., et al. (2014). “Response to Heavy, Non-Floating Oil Spilled in a Great Lakes River Environment: A Multiple-Lines-Of-Evidence Approach for Submerged Oil Assessment and Recovery,” in International Oil Spill Conference Proceedings 2014, 434–448. doi:10.7901/2169-3358-2014.1.434
Dutta, T. K., and Harayama, S. (2000). Fate of Crude Oil by the Combination of Photooxidation and Biodegradation. Environ. Sci. Technol. 34, 1500–1505. doi:10.1021/es991063o
El-Fadel, M., Abdallah, R., and Rachid, G. (2012). A Modeling Approach toward Oil Spill Management along the Eastern Mediterranean. J. Environ. Manag. 113, 93–102. doi:10.1016/j.jenvman.2012.07.035
Elizaryev, A., Maniakova, G., Longobardi, A., Elizareva, E., Gabdulkhakov, R., Nurutdinov, A., et al. (2018). Numerical Simulation of Oil Spills Based on the GNOME and ADIOS. Int. J. Eng. Technol. 7, 24–27. doi:10.14419/IJET.V7I2.23.11876
Elliott, D. C., Hart, T. R., Schmidt, A. J., Neuenschwander, G. G., Rotness, L. J., Olarte, M. V., et al. (2013). Process Development for Hydrothermal Liquefaction of Algae Feedstocks in a Continuous-Flow Reactor. Algal Res. 2, 445–454. doi:10.1016/j.algal.2013.08.005
Environment Canada (2013). Federal Government Technical Report: Properties, Composition and Marine Spill Behaviour, Fate and Transport of Two Diluted Bitumen Products from the Canadian Oil Sands. Available at: https://metchosinmarine.ca/federal-government-technical-report-properties-composition-and-marine-spill-behaviour-fate-and-transport-of-two-diluted-bitumen-products-from-the-canadian-oil-sands/.
Fay, J. A. (1969). “The Spread of Oil Slicks on a Calm Sea,” in Oil on the Sea: Proceedings of a Symposium on the Scientific and Engineering Aspects of Oil Pollution of the Sea, Sponsored by Massachusetts Institute of Technology and Woods Hole Oceanographic Institution and Held at Cambridge, Massachusetts, May 16, 1969 Ocean Technology. Editor D. P. Hoult (Boston, MA: Springer US), 53–63. doi:10.1007/978-1-4684-9019-0_5
Fekete, S. (2006). Pricing of Canadian Oil Sands Blends. Available at: https://efiling.web.commerce.state.mn.us/edockets/searchDocuments.do?method=showPoup&documentId=%7BDCA2C2F3-DE49-4A54-9CA6-9DCF72880718%7D&documentTitle=5639144 (Accessed June 6, 2022).
Fingas, M. (2015). “Diluted Bitumen (Dilbit): A Future High Risk Spilled Material,” in Proceedings of Interspill (Edmonton, Alberta, Canada: Allen Press), 24.
Fingas, M., and Fieldhouse, B. (2004). Formation of Water-In-Oil Emulsions and Application to Oil Spill Modelling. J. Hazard. Mater. 107, 37–50. doi:10.1016/j.jhazmat.2003.11.008
Fingas, M. (2011). “Introduction to Spill Modeling,” in Oil Spill Science and Technology. Editor M. Fingas (Boston: Gulf Professional Publishing), 187–200. doi:10.1016/B978-1-85617-943-0.10008-5
Garcia, M. C., Sanz-Bobi, M. A., and del Pico, J. (2006). SIMAP: Intelligent System for Predictive Maintenance. Comput. Industry 57, 552–568. doi:10.1016/j.compind.2006.02.011
Guo, K., Li, H., and Yu, Z. (2016). In-situ Heavy and Extra-heavy Oil Recovery: A Review. Fuel 185, 886–902.
Han, M., Zhang, J., Chu, W., Chen, J., and Zhou, G. (2019). Research Progress and Prospects of Marine Oily Wastewater Treatment: A Review. Water 11, 2517. doi:10.3390/w11122517
Headley, J. V., and McMartin, D. W. (2004). A Review of the Occurrence and Fate of Naphthenic Acids in Aquatic Environments. J. Environ. Sci. Health, Part A 39, 1989–2010. doi:10.1081/ese-120039370
Heimann, K. (2016). Novel Approaches to Microalgal and Cyanobacterial Cultivation for Bioenergy and Biofuel Production. Curr. Opin. Biotechnol. 38, 183–189. doi:10.1016/j.copbio.2016.02.024
Helmer Pedersen, T., and Conti, F. (2017). Improving the Circular Economy via Hydrothermal Processing of High-Density Waste Plastics. Waste Manag. 68, 24–31. doi:10.1016/j.wasman.2017.06.002
Hole, L. R., de Aguiar, V., Dagestad, K.-F., Kourafalou, V. H., Androulidakis, Y., Kang, H., et al. (2021). Long Term Simulations of Potential Oil Spills Around Cuba. Mar. Pollut. Bull. 167, 112285. doi:10.1016/j.marpolbul.2021.112285
Hospital, A., Stronach, J. A., Miguez, T., Kanjilal, B., Rogers, J., Potts, D., et al. (2015). “Diluted Bitumen Oil Spill Modelling in Support of the Environmental and Socio-Economic Assessment (ESA) for the Trans Mountain Expansion Project,” in Proceedings of the Canadian Water Resources Association—BC Conference (IEEE).
Hounjet, L. J., Stoyanov, S. R., and Chao, D. (2018). Distributions of Diluted Bitumen and Conventional Crude Oil in a Range of Water Types. Chemosphere 211, 1212–1218. doi:10.1016/j.chemosphere.2018.08.025
Hounjet, L. J., and Dettman, H. D. (2016). “Impacts of Pretreatment on Properties and Behaviour of Diluted Bitumen in Water,” in Proceedings of the Thirty-Ninth AMOP Technical Seminar on Environmental Contamination and Response (Ottawa, ON: Environment and Climate Change Canada), 472–493.
Hua, Y., Mirnaghi, F. S., Yang, Z., Hollebone, B. P., and Brown, C. E. (2018). Effect of Evaporative Weathering and Oil-Sediment Interactions on the Fate and Behavior of Diluted Bitumen in Marine Environments. Part 1. Spill-Related Properties, Oil Buoyancy, and Oil-Particulate Aggregates Characterization. Chemosphere 191, 1038–1047. doi:10.1016/j.chemosphere.2017.10.156
Humphries, M. (2007). “North American Oil Sands: History of Development, Prospects for the Future - UNT Digital Library,” in Analyst in Energy Policy Resource, Science, and Industry Division (Washington, DC: UNT Digital Library).
Iler, A. L., and Hamilton, P. D. (2015). “Detecting Oil on Water Using Polarimetric Imaging,” in Ocean Sensing and Monitoring VII (Maryland: International Society for Optics and Photonics), 94590P. doi:10.1117/12.2180169
Jamaly, S., Giwa, A., and Hasan, S. W. (2015). Recent Improvements in Oily Wastewater Treatment: Progress, Challenges, and Future Opportunities. J. Environ. Sci. 37, 15–30. doi:10.1016/j.jes.2015.04.011
Ji, W., Boufadel, M., Zhao, L., Robinson, B., King, T., An, C., et al. (2021). Formation of Oil-Particle Aggregates: Impacts of Mixing Energy and Duration. Sci. Total Environ. 795, 148781. doi:10.1016/j.scitotenv.2021.148781
Ji, Z.-G. (2017). Hydrodynamics and Water Quality: Modeling Rivers, Lakes, and Estuaries. John Wiley & Sons.
Johannessen, S. C., Greer, C. W., Hannah, C. G., King, T. L., Lee, K., Pawlowicz, R., et al. (2020). Fate of Diluted Bitumen Spilled in the Coastal Waters of British Columbia, Canada. Mar. Pollut. Bull. 150, 110691. doi:10.1016/j.marpolbul.2019.110691
Kennedy, C. J. (2015). “Multiple Effects of Oil and its Components in Fish,” in Impacts of Oil Spill Disasters on Marine Habitats and Fisheries in North America (Boca Raton, FL: Routledge), 3–34.
Keramea, P., Kokkos, N., Gikas, G., and Sylaios, G. (2022). Operational Modeling of North Aegean Oil Spills Forced by Real-Time Met-Ocean Forecasts. Jmse 10, 411. doi:10.3390/jmse10030411
King, T. L., Robinson, B., Boufadel, M., and Lee, K. (2014). Flume Tank Studies to Elucidate the Fate and Behavior of Diluted Bitumen Spilled at Sea. Mar. Pollut. Bull. 83, 32–37. doi:10.1016/j.marpolbul.2014.04.042
Lardner, R. W., Zodiatis, G., Loizides, L., and Demetropoulos, A. (1999). An Operational Oil Spill Model for the Levantine Basin (Eastern Mediterranean Sea). Available at: http://inis.iaea.org/Search/search.aspx?orig_q=RN:30046843 (Accessed November 23, 2018).
Lardner, R., Zodiatis, G., Hayes, D., and Pinardi, N. (2006). “Application of the MEDSLIK Oil Spill Model to the Lebanese Spill of July 2006,” in European Group of Experts on Satellite Monitoring of Sea Based Oil Pollution (Brussels: European Communities), 1018–5593.
Lee, K., Boufadel, M., Chen, B., Foght, J., Hodson, P., Swanson, S., et al. (2015). Expert Panel Report on the Behaviour and Environmental Impacts of Crude Oil Released into Aqueous Environments. Ottawa: Royal Society of Canada.
Lee, K., Bugden, J., Cobanli, S., King, T., McIntyre, C., Robinson, B., et al. (2012). UV-epifluorescence Microscopy Analysis of Sediments Recovered from the Kalamazoo River. Dartmouth, NS: US EPA Kalamazoo Administrative Record, 1277.
Lee, R. F. (2003). Photo-oxidation and Photo-Toxicity of Crude and Refined Oils. Spill Sci. Technol. Bull. 8, 157–162. doi:10.1016/s1353-2561(03)00015-x
Lehr, W. J. (2001). Review of Modeling Procedures for Oil Spill Weathering Behavior. Adv. Ecol. Sci. 9, 51–90.
Lehr, W., Jones, R., Evans, M., Simecek-Beatty, D., and Overstreet, R. (2002). Revisions of the ADIOS Oil Spill Model. Environ. Model. Softw. 17, 189–197. doi:10.1016/S1364-8152(01)00064-0
Lehr, W. J., Overstreet, R., Jones, R., and Watabayashi, G. (1992). ADIOS-automated Data Inquiry for Oil Spills. Available at: http://inis.iaea.org/Search/search.aspx?orig_q=RN:25009588 (Accessed November 23, 2018).
Li, P., Niu, H., Li, S., King, T. L., Zou, S., Chen, X., et al. (2022). DBWM: A Diluted Bitumen Weathering Model. Mar. Pollut. Bull. 175, 113372. doi:10.1016/j.marpolbul.2022.113372
Mackay, D., Paterson, S., and Trudel, K. (1980b). A Mathematical Model of Oil Spill Behaviour. Ottawa: Environment Canada, Environmental Protection Service, Environmental Impact Control Directorate, Environmental Emergency Branch, Research and Development Division.
Mackay, D., Buist, I., Mascarenhas, R., and Paterson, S. (1980a). “Oil Spill Processes and Models,” in Environment Canada Manuscript Report No. EE-8 (Ottawa, Ontario: Springer).
Mackay, D. (1980). “Calculation of the Evaporation Rate of Volatile Liquids,” in Proc. National Conference on Control of Hazardous Material Spills (Louisville, KY: IEEE). Available at: https://ci.nii.ac.jp/naid/10016586821/ (Accessed March 31, 2022).
Madison, B. N., Hodson, P. V., and Langlois, V. S. (2015). Diluted Bitumen Causes Deformities and Molecular Responses Indicative of Oxidative Stress in Japanese Medaka Embryos. Aquat. Toxicol. 165, 222–230. doi:10.1016/j.aquatox.2015.06.006
Mancini, G., Cappello, S., Yakimov, M. M., Polizzi, A., and Torregrossa, M. (2012). Biological Approaches to the Treatment of Saline Oily Waste (Waters) Originated from Marine Transportation. Chem. Eng. 27.
Manzetti, S. (2012). Ecotoxicity of Polycyclic Aromatic Hydrocarbons, Aromatic Amines, and Nitroarenes through Molecular Properties. Environ. Chem. Lett. 10, 349–361. doi:10.1007/s10311-012-0368-0
Marcotte, G., Bourgouin, P., Mercier, G., Gauthier, J. P., Pellerin, P., Smith, G., et al. (2016). in Canadian Oil Spill Modelling Suite: An Overview. Editor N. S. Halifax (Canada, 1026–1034.
Martínez-Boza, F. J., Martín-Alfonso, M. J., Gallegos, C., and Fernández, M. (2011). High-Pressure Behavior of Intermediate Fuel Oils. Energy & Fuels 25, 5138–5144. doi:10.1021/ef200958v
Menzies, J. R., and Thomas, R. (2014). Effects of Diluted Bitumen on Crude Oil Transmission Pipelines. Washington, DC: TR News. Available at: https://trid.trb.org/view/1308843 (Accessed October 11, 2018).
Michel, J., and Rutherford, N. (2014). Impacts, Recovery Rates, and Treatment Options for Spilled Oil in Marshes. Mar. Pollut. Bull. 82, 19–25. doi:10.1016/j.marpolbul.2014.03.030
Miguez, T., and Stronach, J. (2019). Flammability Risk Assessment for Oil Spill Response Operations. Acta Oceanol. Sin. 38, 113–119.
National Academies of Sciences, and Medicine (2016). “Introduction,” in Spills of Diluted Bitumen from Pipelines: A Comparative Study of Environmental Fate, Effects, and Response (Washington, DC: National Academies Press).
Nittis, K., Perivoliotis, L., Korres, G., Tziavos, C., and Thanos, I. (2006). Operational Monitoring and Forecasting for Marine Environmental Applications in the Aegean Sea. Environ. Model. Softw. 21, 243–257. doi:10.1016/j.envsoft.2004.04.023
Niu, H., Li, S., King, T., and Lee, K. (2016). “Stochastic Modeling of Oil Spill in the Salish Sea,” in The 26th International Ocean and Polar Engineering Conference (Rhodes: International Society of Offshore and Polar Engineers).
Niu, H., Li, Z., Lee, K., Kepkay, P., and Mullin, J. V. (2011). Modelling the Transport of Oil-Mineral-Aggregates (OMAs) in the Marine Environment and Assessment of Their Potential Risks. Environ. Model Assess. 16, 61–75. doi:10.1007/s10666-010-9228-0
NOAA (2019). GNOME Suite for Oil Spill Modeling. Office of Response and Restoration. Available at: https://response.restoration.noaa.gov/oil-and-chemical-spills/oil-spills/response-tools/gnome-suite-oil-spill-modeling.html (Accessed January 29, 2022).
Nordam, T., Lofthus, S., and Brakstad, O. G. (2020). Modelling Biodegradation of Crude Oil Components at Low Temperatures. Chemosphere 254, 126836. doi:10.1016/j.chemosphere.2020.126836
Oil Sands Magazine (2020). Products from the Oil Sands: Dilbit, Synbit & Synthetic Crude Explained. Oil Sands Magazine. Available at: http://www.oilsandsmagazine.com/technical/product-streams (Accessed March 16, 2022).
Ortmann, A. C., Cobanli, S. E., Wohlgeschaffen, G., MacDonald, J., Gladwell, A., Davis, A., et al. (2020). Measuring the Fate of Different Diluted Bitumen Products in Coastal Surface Waters. Mar. Pollut. Bull. 153, 111003. doi:10.1016/j.marpolbul.2020.111003
Peterson, A. A., Vogel, F., Lachance, R. P., Fröling, M., Antal, Jr., M. J., and Tester, J. W. (2008). Thermochemical Biofuel Production in Hydrothermal Media: A Review of Sub- and Supercritical Water Technologies. Energy Environ. Sci. 1, 32–65. doi:10.1039/b810100k
Philibert, D. A., Philibert, C. P., Lewis, C., and Tierney, K. B. (2016). Comparison of Diluted Bitumen (Dilbit) and Conventional Crude Oil Toxicity to Developing Zebrafish. Environ. Sci. Technol. 50, 6091–6098. doi:10.1021/acs.est.6b00949
Prince, R. C., Garrett, R. M., Bare, R. E., Grossman, M. J., Townsend, T., Suflita, J. M., et al. (2003). The Roles of Photooxidation and Biodegradation in Long-Term Weathering of Crude and Heavy Fuel Oils. Spill Sci. Technol. Bull. 8, 145–156. doi:10.1016/s1353-2561(03)00017-3
Rajib, A., Hung, A., and Fini, E. H. (2021). Weathering Mechanisms in Bitumen Modified with Polyphosphoric Acid. J. Mater. Civ. Eng. 33, 04021154. doi:10.1061/(asce)mt.1943-5533.0003795
Ranjbar, P., Shafieefar, M., and Rezvandoust, J. (2014). Modeling of Oil Spill and Response in Support of Decreasing Environmental Oil Effects Case Study: Blowout from Khark Subsea Pipelines (Persian Gulf). Int. J. Environ. Res. 8, 289–296.
Reed, M., Aamo, O. M., and Daling, P. S. (1995). Quantitative Analysis of Alternate Oil Spill Response Strategies Using OSCAR. Spill Sci. Technol. Bull. 2, 67–74. doi:10.1016/1353-2561(95)00020-5
Reed, M., Daling, P., Lewis, A., Ditlevsen, M. K., Brørs, B., Clark, J., et al. (2004). Modelling of Dispersant Application to Oil Spills in Shallow Coastal Waters. Environ. Model. Softw. 19, 681–690. doi:10.1016/j.envsoft.2003.08.014
Reed, M., Ekrol, N., Rye, H., and Turner, L. (1999a). Oil Spill Contingency and Response (OSCAR) Analysis in Support of Environmental Impact Assessment Offshore Namibia. Spill Sci. Technol. Bull. 5, 29–38. doi:10.1016/S1353-2561(98)00050-4
Reed, M., Gundlach, E., and Kana, T. (1989). A Coastal Zone Oil Spill Model: Development and Sensitivity Studies. Oil Chem. Pollut. 5, 411–449. doi:10.1016/S0269-8579(89)80019-X
Reed, M., Johansen, Ø., Brandvik, P. J., Daling, P., Lewis, A., Fiocco, R., et al. (1999b). Oil Spill Modeling towards the Close of the 20th Century: Overview of the State of the Art. Spill Sci. Technol. Bull. 5, 3–16. doi:10.1016/S1353-2561(98)00029-2
Ren, S., Dang-Vu, T., Zhao, H., Long, J., Xu, Z., and Masliyah, J. (2009). Effect of Weathering on Surface Characteristics of Solids and Bitumen from Oil Sands. Energy fuels. 23, 334–341. doi:10.1021/ef800635t
Röhrs, J., Dagestad, K.-F., Asbjørnsen, H., Nordam, T., Skancke, J., Jones, C. E., et al. (2018). The Effect of Vertical Mixing on the Horizontal Drift of Oil Spills. Ocean. Sci. 14, 1581–1601. doi:10.5194/os-14-1581-2018
RPS-ASA (2022). Software - SIMAPTM. Available at: http://asascience.com/software/simap/ (Accessed September 10, 2018).
Ruberg, E. J., Elliott, J. E., and Williams, T. D. (2021). Review of Petroleum Toxicity and Identifying Common Endpoints for Future Research on Diluted Bitumen Toxicity in Marine Mammals. Ecotoxicology 30, 537–551. doi:10.1007/s10646-021-02373-x
Saleem, J., and McKay, G. (2016). Waste HDPE Bottles for Selective Oil Sorption. Asia-Pac. J. Chem. Eng. 11, 642–645. doi:10.1002/apj.1989
Saleem, J., Ning, C., Barford, J., and McKay, G. (2015). Combating Oil Spill Problem Using Plastic Waste. waste Manag. 44, 34–38. doi:10.1016/j.wasman.2015.06.003
Saleem, S., Hu, G., Li, J., Hewage, K., and Sadiq, R. (2022). Evaluation of Offshore Oil Spill Response Waste Management Strategies: A Lifecycle Assessment-Based Framework. J. Hazard. Mater. 432, 128659. doi:10.1016/j.jhazmat.2022.128659
Sereneo, M. (2017). The Sublethal Effects of Chemically-Dispersed Access Western Blend and Cold Lake Blend Diluted Bitumen on Rainbow Trout Embryos. Ontario: Oncorhynchus mykiss.
Seshasayee, M. S., and Savage, P. E. (2021). Synergistic Interactions during Hydrothermal Liquefaction of Plastics and Biomolecules. Chem. Eng. J. 417, 129268. doi:10.1016/j.cej.2021.129268
Sheehan, J. D., and Savage, P. E. (2017). Modeling the Effects of Microalga Biochemical Content on the Kinetics and Biocrude Yields from Hydrothermal Liquefaction. Bioresour. Technol. 239, 144–150. doi:10.1016/j.biortech.2017.05.013
Short, J. W. (2013). Susceptibility of Diluted Bitumen Products from the Alberta Tar Sands to Sinking in Water. Alaska: JWS Consulting LLC.
S. L. Ross Environmental Research Limited (2012). Meso-scale Weathering of Cold Lake Bitumen/condensate Blend. Ottawa: SL Ross Environmental Research Ltd, 200–1140.
Smith, R. A., Slack, J. R., Wyant, T., and Lanfear, K. J. (1982). “The Oilspill Risk Analysis Model of the US Geological Survey,” in Geological Survey Professional Paper 1227 (Reston, VA, USA: US Geological Survey), 36. doi:10.3133/pp1227
Spaulding, M. L., Kolluru, V. S., Anderson, E., and Howlett, E. (1994). Application of Three-Dimensional Oil Spill Model (WOSM/OILMAP) to Hindcast the Braer Spill. Spill Sci. Technol. Bull. 1, 23–35. doi:10.1016/1353-2561(94)90005-1
Spaulding, M. L. (2017). State of the Art Review and Future Directions in Oil Spill Modeling. Mar. Pollut. Bull. 115, 7–19. doi:10.1016/j.marpolbul.2017.01.001
Stantec Consulting Ltd (2016). Ottawa River Site-specific Risk Assessment. Available at: https://archives.bape.gouv.qc.ca/sections/mandats/oleoduc_energie-est/documents/EAU15.pdf.
Stoyanovich, S., Rodríguez-Gil, J. R., Hanson, M. L., Hollebone, B. P., Orihel, D. M., Palace, V. P., et al. (2021). Simulating Diluted Bitumen Spills in Boreal Lake Limnocorrals - Part 2: Factors Affecting the Physical Characteristics and Submergence of Diluted Bitumen. Sci. Total Environ. 790, 148580. doi:10.1016/j.scitotenv.2021.148580
Swarthout, B., Reddy, C. M., Nelson, R. K., Hamilton, S. K., Aeppli, C., Valentine, D. L., et al. (2016). The Other Major 2010 Oil Spill: Oil Weathering After the Kalamazoo River Dilbit Spill. American Geophysical Union 2016. HI54B-1875
Swift, A., Casey-Lefkowitz, S., and Shope, E. (2011). Tar Sands Pipelines Safety Risks. New York, NY: Natural Resources Defense Council. Available at: https://pstrust.org/docs/TarSandsPipelineSafetyRisksFINALFINAL.pdf.pdf (Accessed March 16, 2022).
Tanudjaja, H. J., Hejase, C. A., Tarabara, V. V., Fane, A. G., and Chew, J. W. (2019). Membrane-based Separation for Oily Wastewater: A Practical Perspective. Water Res. 156, 347–365. doi:10.1016/j.watres.2019.03.021
Tetra Tech (2022). SPILLCALC Oil and Contaminant Spill Model. Available at: http://www.tetratech.com/en/projects/spillcalc-oil-and-contaminant-spill-model (Accessed September 10, 2018).
Thompson, S. O., and Rough, S. L. (2021). The Densification of Cocoa Bean Shells for Bioenergy Purposes. Biomass Bioenergy 148, 106057. doi:10.1016/j.biombioe.2021.106057
Torres, D. H. A., da Costa Dias, F., Bahiana, B. R., Haddad, A. N., Chinelli, C. K., and Soares, C. A. P. (2020). Oil Spill Simulation and Analysis of Its Behavior Under the Effect of Weathering and Chemical Dispersant: a Case Study of the Bacia de Campos-Brazil. Water Air Soil Pollut. 231, 521. doi:10.1007/s11270-020-04857-8
Toz, A. C., and Buber, M. (2018). Performance Evaluation of Oil Spill Software Systems in Early Fate and Trajectory of Oil Spill: Comparison Analysis of OILMAP and PISCES 2 in Mersin Bay Spill. Environ. Monit. Assess. 190, 551. doi:10.1007/s10661-018-6872-3
Tsaprailis, H., Zhou, J., and Geol, P. (2014). Properties of Dilbit and Conventional Crude Oils. Calgary: Alberta Innovates.
US EPA, O. (2018). Understanding Oil Spills and Oil Spill Response. Available at: https://www.epa.gov/emergency-response/understanding-oil-spills-and-oil-spill-response (Accessed November 28, 2019).
Veljković, V. B., Avramović, J. M., and Stamenković, O. S. (2012). Biodiesel Production by Ultrasound-Assisted Transesterification: State of the Art and the Perspectives. Renew. Sustain. Energy Rev. 16, 1193–1209.
Walker, A., Stern, C., Scholz, D., Nielsen, E., Csulak, F., and Gaudiosi, R. (2016). Consensus Ecological Risk Assessment of Potential Transportation-Related Bakken and Dilbit Crude Oil Spills in the Delaware Bay Watershed, USA. Jmse 4, 23. doi:10.3390/jmse4010023
Wang, S.-D., Shen, Y.-M., Guo, Y.-K., and Tang, J. (2008). Three-dimensional Numerical Simulation for Transport of Oil Spills in Seas. Ocean. Eng. 35, 503–510. doi:10.1016/j.oceaneng.2007.12.001
Westlake, D. W. S., Jobson, A., Phillippe, R., and Cook, F. D. (1974). Biodegradability and Crude Oil Composition. Can. J. Microbiol. 20, 915–928. doi:10.1139/m74-141
Wu, D., Fang, L., Qin, Y., Wu, W., Mao, C., and Zhu, H. (2014). Oil Sorbents with High Sorption Capacity, Oil/water Selectivity and Reusability for Oil Spill Cleanup. Mar. Pollut. Bull. 84, 263–267. doi:10.1016/j.marpolbul.2014.05.005
Wu, Y., Hannah, C., Law, B., King, T., and Robinson, B. (2016). “An Estimate of the Sinking Rate of Spilled Diluted Bitumen in Sediment-Laden Coastal Waters,” in Proceedings of the 39th AMOP Technical Seminar (Ottawa, ON, Canada: Environment and Climate Change Canada), 331–347.
Xu, C., Nasrollahzadeh, M., Selva, M., Issaabadi, Z., and Luque, R. (2019). Waste-to-wealth: Biowaste Valorization into Valuable Bio(nano)materials. Chem. Soc. Rev. 48, 4791–4822. doi:10.1039/C8CS00543E
Yang, C., Wang, Z., Yang, Z., Hollebone, B., Brown, C. E., Landriault, M., et al. (2011). Chemical Fingerprints of Alberta Oil Sands and Related Petroleum Products. Environ. Forensics 12, 173–188. doi:10.1080/15275922.2011.574312
Yang, J., Chen, H., Liu, Q., Zhou, N., Wu, Y., He, Q., et al. (2020a). Is it Feasible to Replace Freshwater by Seawater in Hydrothermal Liquefaction of Biomass for Biocrude Production? Fuel 282, 118870. doi:10.1016/j.fuel.2020.118870
Yang, J., He, Q., Corscadden, K., Niu, H., Lin, J., and Astatkie, T. (2019a). Advanced Models for the Prediction of Product Yield in Hydrothermal Liquefaction via a Mixture Design of Biomass Model Components Coupled with Process Variables. Appl. Energy 233-234, 906–915. doi:10.1016/j.apenergy.2018.10.035
Yang, J., He, Q., Niu, H., Corscadden, K., and Astatkie, T. (2018a). Hydrothermal Liquefaction of Biomass Model Components for Product Yield Prediction and Reaction Pathways Exploration. Appl. energy 228, 1618–1628. doi:10.1016/j.apenergy.2018.06.142
Yang, J., He, Q., Niu, H., Dalai, A., Corscadden, K., and Zhou, N. (2020b). Microwave-assisted Hydrothermal Liquefaction of Biomass Model Components and Comparison with Conventional Heating. Fuel 277, 118202. doi:10.1016/j.fuel.2020.118202
Yang, J., Xin, Z., He, Q., Corscadden, K., and Niu, H. (2019b). An Overview on Performance Characteristics of Bio-Jet Fuels. Fuel 237, 916–936. doi:10.1016/j.fuel.2018.10.079
Yang, L., Nazari, L., Yuan, Z., Corscadden, K., Xu, C., and He, Q. (2016a). Hydrothermal Liquefaction of Spent Coffee Grounds in Water Medium for Bio-Oil Production. Biomass Bioenergy 86, 191–198. doi:10.1016/j.biombioe.2016.02.005
Yang, W., Wang, Z., Song, S., Chen, H., Wang, X., Cheng, J., et al. (2019c). Understanding Catalytic Mechanisms of HZSM-5 in Hydrothermal Liquefaction of Algae through Model Components: Glucose and Glutamic Acid. Biomass Bioenergy 130, 105356. doi:10.1016/j.biombioe.2019.105356
Yang, Z., Hollebone, B. P., Brown, C. E., Yang, C., Wang, Z., Zhang, G., et al. (2016b). The Photolytic Behavior of Diluted Bitumen in Simulated Seawater by Exposed to the Natural Sunlight. Fuel 186, 128–139. doi:10.1016/j.fuel.2016.08.068
Yang, Z., Hua, Y., Mirnaghi, F., Hollebone, B. P., Jackman, P., Brown, C. E., et al. (2018b). Effect of Evaporative Weathering and Oil-Sediment Interaction on the Fate and Behavior of Diluted Bitumen in Marine Environments. Part 2. The Water Accommodated and Particle-Laden Hydrocarbon Species and Toxicity of the Aqueous Phase. Chemosphere 191, 145–155. doi:10.1016/j.chemosphere.2017.10.033
Yarranton, H., Motahhari, H. R., Schoeggl, F., and Zhou, J. (2015). Evaporative Weathering of Diluted Bitumen Films. J. Can. Petroleum Technol. 54, 223–244. doi:10.2118/174557-pa
Ye, L., Manning, A. J., and Hsu, T.-J. (2020). Oil-mineral Flocculation and Settling Velocity in Saline Water. Water Res. 173, 115569. doi:10.1016/j.watres.2020.115569
Yu, F., Zhao, W., Qin, T., Zhao, W., Chen, Y., Miao, X., et al. (2022). Biosafety of Human Environments Can Be Supported by Effective Use of Renewable Biomass. Proc. Natl. Acad. Sci. U.S.A. 119, e2106843119. doi:10.1073/pnas.2106843119
Keywords: diluted bitumen, physicochemical properties, weathering processes, oil spill response, oil spill recovery
Citation: Zhong X, Li P, Lin X, Zhao Z, He Q(, Niu H and Yang J (2022) Diluted Bitumen: Physicochemical Properties, Weathering Processes, Emergency Response, and Recovery. Front. Environ. Sci. 10:910365. doi: 10.3389/fenvs.2022.910365
Received: 01 April 2022; Accepted: 11 May 2022;
Published: 27 June 2022.
Edited by:
Jianbing Li, University of Northern British Columbia Canada, CanadaReviewed by:
Veerasingam S., Qatar University, QatarPuspa L. Adhikari, Florida Gulf Coast University, United States
Copyright © 2022 Zhong, Li, Lin, Zhao, He, Niu and Yang. This is an open-access article distributed under the terms of the Creative Commons Attribution License (CC BY). The use, distribution or reproduction in other forums is permitted, provided the original author(s) and the copyright owner(s) are credited and that the original publication in this journal is cited, in accordance with accepted academic practice. No use, distribution or reproduction is permitted which does not comply with these terms.
*Correspondence: Haibo Niu, aGFpYm8ubml1QGRhbC5jYQ==; Jie Yang, amllLnlhbmdAbWp1LmVkdS5jbg==
†These authors have contributed equally to this work and share first authorship