- 1National Institute of Oceanography, Israel Oceanographic and Limnological Research, Haifa, Israel
- 2Institute of Marine Science, University of California, Santa Cruz, Santa Cruz, CA, United States
The atmosphere plays a fundamental role in transporting airborne prokaryotes across the oceans and land. Despite the harsh atmospheric conditions, a considerable fraction of the airborne prokaryotic microorganisms survive the journey and remain viable upon deposition, and can affect the receiving environment. Here, we provide the first estimate of potential viability proxy for airborne prokaryotic cells at the Southeastern Mediterranean coast in 22 events during 2015, representing marine and terrestrial air-mass trajectories and a significant dust storm event. This was assessed using sequence amplicons of the small subunit ribosomal RNA gene (SSU rRNA) jointly with other complementary measurements. To estimate the relative viability in our dataset we used the ratio between the abundance of the bacterial SSU rRNA transcripts in a given sampling date and the lowest measured value (23.7.2015) as a measure of a relative viability proxy. The abundance of prokaryotes SSU rRNA transcripts ranged from ∼500 to 11,000 copies m3, with ∼2-fold higher relative viability proxy in marine-origin aerosols than predominantly terrestrial atmospheric trajectories. The relative viability proxy of prokaryotes was low during the peak of an intense and prolonged dust storm, and increased by ∼1.5-fold in the subsequent days representing background conditions (<1700 ng Al m−3). Furthermore, we show that anthropogenic/toxic trace-metals (Cu/Al, Pb/Al) negatively correlates with potentially viable airborne prokaryotes in marine trajectory aerosols, whereas mineral dust load (Al, Fe proxy) positively affect their potential viability proxy. This may suggest that airborne prokaryotes associated to marine trajectories benefit from a particle-associate lifestyle, enabling relatively higher humidity and supply of nutrients attributed to mineral dust particles.
Introduction
Aerosol deposition provide external nutrients that contribute to phytoplankton/bacterial growth and activity in the surface layers of the ocean (Moore et al., 2009; Tsagaraki et al., 2017). Experimental results, however, show that aerosol addition do not consistently induce the expected nutrient fertilization effect on phytoplankton/bacteria (Hill et al., 2010; Guieu et al., 2014; Torfstein et al., 2017). Several processes may contribute to this lack of response, including differences in the chemical composition of aerosols and associated release of macro and micro-nutrients (Moore et al., 2013) and toxic elements (Paytan et al., 2009; Després et al., 2012; Jordi et al., 2012). Specifically, variability in the ambient natural assemblages of organisms at different locations and during different seasons may also result in variable responses to atmospheric deposition (Gallisai et al., 2014). Transport and deposition of dust during storms, or the frequency and intensity of the pulses of deposition, may also contribute to the observed variability in ecosystem responses (Giovagnetti et al., 2013). Another factor that may contribute to the observed variability, which has been far less investigated, is the supply of airborne microorganisms upon aerosols deposition to the surface water (Polymenakou, 2012; Peter et al., 2014; Rahav et al., 2016a), resulting in unique interaction with the in situ microbial communities (Mescioglu et al., 2019b; Fragola et al., 2021; Peng et al., 2021).
Airborne prokaryotic microorganisms were often neglected as a microbial habitat and a significant ecological vector (Kellogg and Griffin, 2006; Polymenakou, 2012; Mayol et al., 2014). This is partly due to the assumption that most microorganisms in aerosols die while in the atmosphere, before their deposition, as a result of inhospitable conditions including exposure to solar UV-radiation, desiccation, and/or due to low nutrients availability or high levels of toxic constituents (Griffin, 2007). Yet, many bacterial phylum can form spores that protect the cells from desiccation, heat, acidity, radiation, and nutrient-poor conditions (Tang, 2009). Moreover, many bacteria are highly pigmented, which may help shield the cells from damaging radiation levels (Kellogg et al., 2004; Fahlgren et al., 2010). Clouds, fog, smoke and desert dust particles can also shed airborne bacteria from damaging UV radiation (Hara and Zhang, 2012). Evidently, atmospheric aerosols contain bacteria, archaea, algae, viruses, fungi, and pollen originating from land (Kellogg and Griffin, 2006; Griffin et al., 2007) or the ocean (Prospero et al., 2005; Flores et al., 2020). Airborne microorganisms were identified in almost every aerosol sample collected over aquatic and terrestrial environments (Leck and Bigg, 2005; Favet et al., 2013; Yahya et al., 2019), usually ranging from ∼103 to ∼107 cells per m3 of air (Mayol et al., 2017; Rahav et al., 2019; Alsante et al., 2021), and were shown to contribute to bacterial production, primary production, N2 fixation and viral infections (Peter et al., 2014; Rahav et al., 2018; Rahav et al., 2020). Understanding the parameters that determine the survival of airborne microorganisms is of great ecological significance and may shed light on microbial dispersion throughout the world’s oceans.
The Southeastern Mediterranean Sea is a semi-enclosed oligotrophic basin (Siokou-Frangou et al., 2010; Sisma-Ventura et al., 2021), subjected to relatively high input of aerosols originated from neighboring deserts (Herut et al., 2002; Guieu et al., 2014). The aerosols could have different atmospheric transport routes dominated by marine and/or terrestrial paths (Rahav et al., 2019). Previous indirect evidences suggest that airborne microbes can become active following deposition in Southeastern Mediterranean seawater (e.g., Rahav et al., 2018), highlighting the need to quantify their viability and better understand their biochemical role.
Here, we proxy the potential viable airborne prokaryotic microbes (bacteria and archaea) in naturally occurring aerosols and during an extreme dust event in the Southeastern Mediterranean coastal Sea in 22 events over 2015. We hypothesized that terrestrial air-mass trajectories or dust storm events will have relatively lower viable prokaryotic microorganisms upon deposition in seawater. Contrary, marine air-mass trajectories, which likely contain a larger fraction of sea-spray derived prokaryotes, will be more protected from the hostile atmospheric conditions and thus have a relatively higher viable airborne cells.
Material and methods
Bioaerosols collection
Aerosols were collected onto sterile 0.22-μm polycarbonate filters (PALL) using a custom-made high-volume sampler with a collection rate of 40 m3 h−1 (Figure 1). Aerosols were collected over a few hours (4–6 h) to minimize prokaryotes RNA degradation, accumulate sufficient amount of genetic material and prevent prokaryotic cell damage caused by prolonged filtration (Hu et al., 2017). The sampler was placed ∼22 m above sea level at the shoreline of the Southeastern Mediterranean Sea (Lat. 32.28ʺN, Lon. 34.9ʺE). A total of 22 sampling events were undertaken between February and December 2015 thus covering different seasonal conditions. Three filters were collected in parallel; one for RNA extractions, the second for airborne prokaryotes abundance measurements, and the third as an unused blank placed on the aerosol sampler without any pumping. For some samples (n = 14), bacterial production measurements were also undertaken from a fourth filter pumped in parallel.
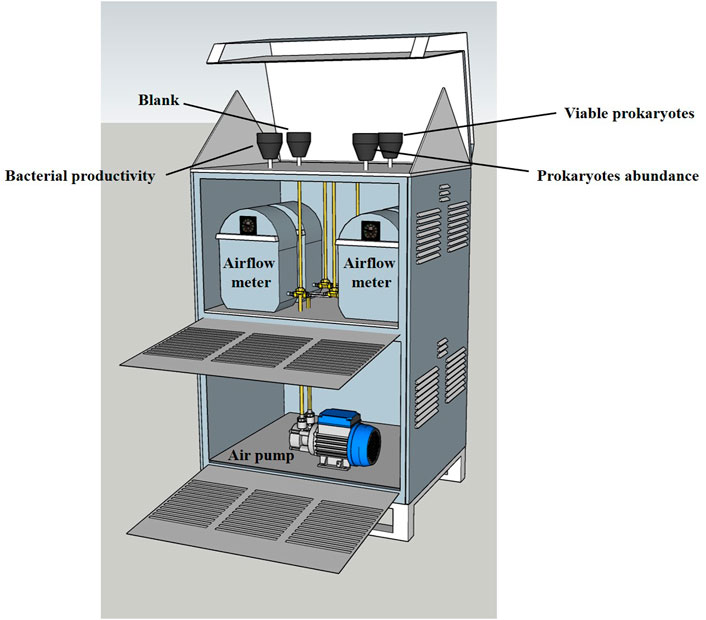
FIGURE 1. An illustration showing the custom-made high-volume sampler (40 m3 h−1, 4–6 h in each sampling event) and the experimental setup used to collect aerosols. The sampler was located ∼22 m above sea level at the Southeastern Mediterranean coast. The collected filters were later processed for measuring the potential viability proxy of airborne prokaryotes and their abundance (n = 22). Blank filters placed on the filter holder without pumping was also collected and analyzed in each sampling event. Filters were collected throughout 2015.
Trace-metals and PM2.5 measurements
Total suspended particles in air (TSP) were sampled in parallel at the same location using a high-volume sampler at a constant flow rate of 60 m3 h−1. After collection, a subsample of the Whatman 41 filters was dried in a desiccator for 24 h before being reweighed. Al, Fe, Pb, and Cu were measured after total digestion with hydrogen fluoride (Herut et al., 2001) following the procedure of ASTM (1983) by an atomic absorption spectrometer Agilent 280FS AA and graphite furnace Agilent 240Z AA.
Suspended particulate matter smaller than 2.5 μm (PM2.5) were collected every 5 min using a low-volume sampler (Thermo Scientific Ambient Particulate Monitor, TEOM® 1400ab; Precision: ±1.5 μg m3 (1-h average).
Air mass backward trajectories
Air mass backward trajectory analyses arriving at 10–250 m altitudes were computed using the HYSPLIT model (http://ready.arl.noaa.gov/HYSPLIT_traj.php). The GDAS 0.5-degree meteorology data was used to run the backward trajectories using a vertical velocity motion. The aerosols were classified to terrestrial or marine origin based on their main route 3 days prior their arrival to the Southeastern Mediterranean coast (Figure 2).
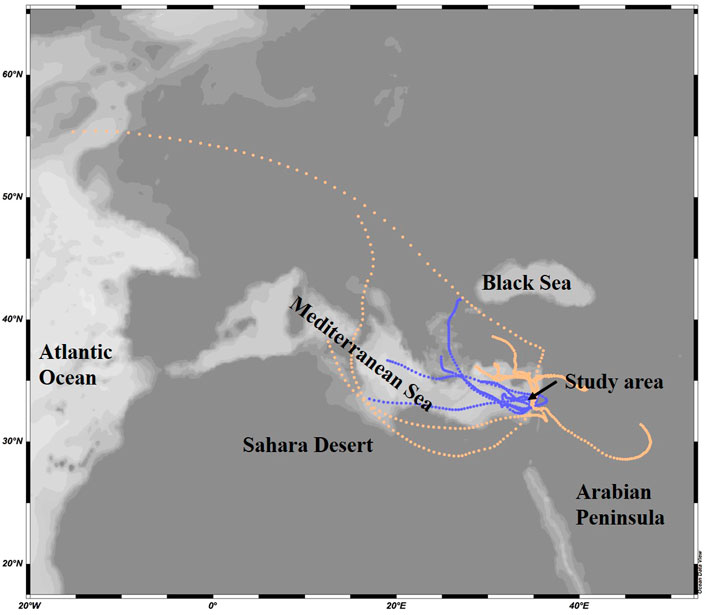
FIGURE 2. Representative marine (blue) and terrestrial (peach) origin of air mass trajectories arriving the Southeastern Mediterranean Sea at 10 m altitude in 2015. The trajectories show the 3-days atmospheric route prior collection.
Airborne prokaryotes abundance
One filter from each event was suspended in 0.22-μm filtered Southeastern Mediterranean costal seawater containing microscopy-grade glutaraldehyde solution (Sigma-Aldrich G7651, final concentration 0.2%). Then, the tube was sonicated for 1 min in a bath sonicator (Symphony) and mixed vigorously for additional 2 min to remove all prokaryotic cells from the filter. Before analyses, triplicate 100 μl aliquots were stained with SYTO9 (1:105 v:v) for 10 min in the dark, and heterotrophic prokaryotes cells were counted using an Attune®Acoustic Focusing Flow Cytometer (Applied Biosystems) equipped with 488 and 405-nm lasers at a rate of 25 μl min−1 using a discrimination threshold of green fluorescence and forward-scatter. Additional unstained triplicate samples were also run for cyanobacterial abundance determination using the orange and red fluorescence, side-scatter and on forward-scatter discriminations. The total airborne cell count is set as the sum of heterotrophic prokaryotes and cyanobacteria. Size beads of ∼1 μm (Polysciences) were run with blank seawater every five samples.
Molecular extraction and SSU rRNA gene copy number
Collected aerosols on filters were placed in an RNA-later solution (Thermo-Fisher AM7023) and snap-frozen in liquid nitrogen and stored in −80°C until they were processed within a few weeks/months. RNA was extracted from the filters using the mirVana RNA isolation kit (Ambion) as instructed by the manufacture. A high capacity cDNA reverse transcription kit (Applied Biosystems) was used to generate cDNA molecules. The small subunit ribosomal RNA (SSU rRNA) gene copy number was determined using a SYBR green-based quantitative real-time PCR (Applied Biosystems) analyses with the broadly conserved bacterial primers 331F (TCCTACGGGAGGCAGCAGT) and 518R (ATTACCGCGGCTGCTGG). Previous studies showed the validity of these primers for RT-qPCR assays (Bräuer et al., 2011; Gat et al., 2017).
Evaluating the relative viability-proxy of airborne bacteria
Estimate of the “relative viability proxy” of airborne microbial cells was defined here as the ratio between the abundance of the SSU rRNA gene copy number in a given sampling date and the value for 23 July 2015 that represent reference conditions with the lowest levels of total prokaryotes cells and Al per m3 air (Supplementary Table S1). We based our proxy on the assumption that the physiological state of prokaryotes is regulated by the expression of ribosomal RNA (Klumpp and Hwa, 2009). Note, however, this approach assumes that the average rRNA copies per airborne bacterial cell between samples/sampling dates is similar.
Results
Aerosols deposition in the Southeastern Mediterranean coastal seawater during the year 2015 ranged from 2 mg m−2 d−1 to as high as 209 mg m−2 d−1 during a dust storm in early September 2015, thus exceeding the previously reported maximal daily average flux of ∼140 mg m−2 d−1 in this area (Lawrence and Neff, 2009). Airborne prokaryotes abundance ranged from 800 to 21,000 cells m−3, with the highest values measured in early September 2015 during an intense dust storm arriving from the North-East (Supplementary Table S1 and discussion below). The abundance of bacterial SSU rRNA transcripts ranged from 524 to 10,985 copies m3 (Figure 3A). We used the abundance of bacterial SSU rRNA transcripts in 23 of July 2015 as a reference (baseline), representing the lowest ranked dataset value and particulate Al levels in air (see “Material and Methods”). We thus evaluate the change/enrichment as a “relative viability proxy,” which ranges from 1 (reference) to ∼21 (Figure 3B). A significantly higher relative viability proxy was found in marine-origin aerosols as compared to aerosols associated with a dominant terrestrial trajectory (Figure 3B, t-test, p = 0.03). The average enrichment in marine-origin aerosols was 13.4 ± 3.7 as compared to 8.6 ± 2.8.
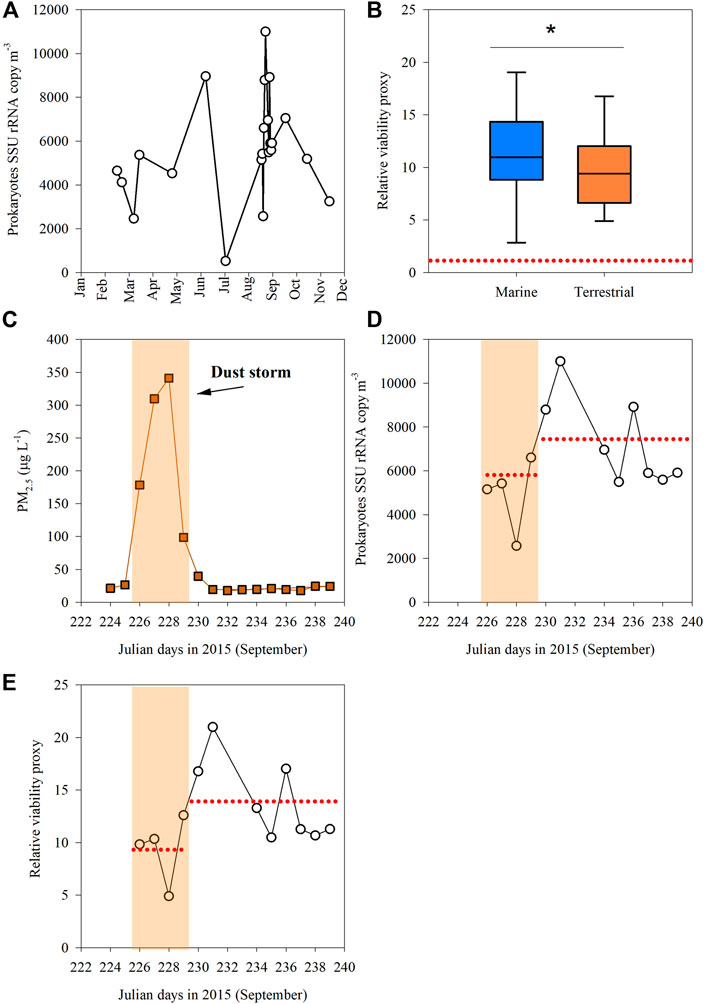
FIGURE 3. (A) Small subunit ribosomal RNA gene (SSU rRNA) copies per cubic meter of air determined by RT-qPCR analysis; (B) The “relative viability proxy” of airborne prokaryotic cells in respect to their atmospheric path using air-mass backward trajectory analyses. (C) The temporal dynamics of PM2.5 through 2015; (D) SSU rRNA gene copies during a storm (8–12 September 2015) and 8 days afterwards (13–21 September 2015); and (E) The “relative viability proxy” for airborne prokaryotes during the dust-storm event and following days. The dash red line in panel (B) signifies the relative baseline conditions with the lowest levels of total prokaryotes cells and Al per m3 air (23 July 2015). The dash red line in panels (D,E) show the averaged value in a given period. The yellowish background signifies the dust storm duration.
Throughout the dust storm event (Julian day 226–229), both the Al (∼13,000–69,000 ng m−3, Supplementary Table S1) and the PM2.5 (∼200–350 μg L−1) levels were significantly higher compared to the lower levels of Al (<1700 ng m−3) and PM2.5 (<40 μg L−1) measured in the following 8 days (t-test, p < 0.001, Figure 3C, Supplementary Table S1) and are considered similar to background conditions in this marine area (Herut et al., 1999). The abundance of bacterial SSU rRNA transcripts during the storm ranged from 5,150 to 8,790 copies m3 and increased to as high as ∼11,000 copies m3 the following days after the storm had settled (Figure 3D). The concurrent relative viability proxy for the airborne prokaryotes during the dust storm was ∼9 ± 3, significantly lower than afterwards, ∼14 ± 4 (t-test, p = 0.03, Figure 3E) or from the annual average of ∼12 ± 4 (t-test, p = 0.04).
An agglomerative hierarchical clustering (AHC) analysis, which considers the prokaryotic cell abundance and RNA transcripts, showed that the marine-origin samples significantly differ from aerosols of a dominant terrestrial trajectory (Figure 4A). In the marine-origin aerosols a significant negative correlation was calculated between Pb/Al or Cu/Al and the relative potential viability of airborne prokaryotes, while in the terrestrial-origin aerosols a less clear trend was observed (Supplementary Figure S1). Fe or Al (proxy for mineral dust particles) were positively correlated in both marine (Figures 4B–D; p < 0.05) and terrestrial-origin aerosols, noting the limited number of observations.
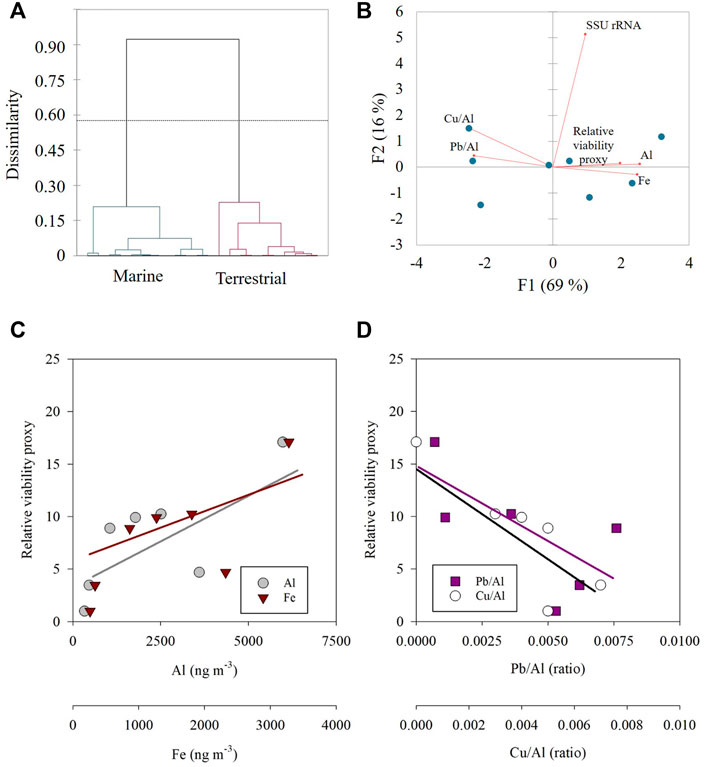
FIGURE 4. (A) An agglomerative hierarchical clustering (AHC) dendrogram showing the dissimilarities (Euclidean distance) between aerosols with marine (green) and terrestrial (red) origin; (B) Principle component analysis (PCA) of the marine-origin trajectories; (C,D) The relationship between the relative potentially viable airborne prokaryotes and Al (grey), Fe (dark red), Pb/Al (dark pink) and Cu/Al (white) in marine-origin aerosols [corresponds to panel (B)].
A limited number of aerosol samples were also collected and analyzed for airborne prokaryotes genetic diversity; one during the intense dust storm (8 September 2015, Julian day 226) and the other under background conditions a few days afterwards (20 September 2015, Julian day 238). The prokaryotic microbial beta-diversity was overall similar between the “dust” and the “background” filters (Supplementary Figure S2). Proteobacteria, Actinobacteria and Firmicutes were the most dominant bacterial phyla (Supplementary Figure S2A). The most dominant prokaryote within the phylum Proteobacteria was alpha-proteobacteria (∼80% of ASVs) followed by beta-proteobacteria (∼10%), gamma-proteobacteria (∼5%), and delta-proteobacteria (∼3%) (Supplementary Figure S2B). Within the order taxonomic level, in the dust filters bacillales, frankiales, and Cytophagales were the most abundant; ∼10% of ASVs each. Clostridia and Chromatiales were present in the dust filters but not in the background, comprising 2%–3% each. In the background filter bacillales comprised ∼20% of the ASVs, which is double than found in the dust filter. Within the family taxonomic level, bacillaceae comprised ∼23% of all ASVs in the background filter, while only ∼4% in the background. Rhodobacteraceae comprised ∼7% in the background and ∼4% in the dust filters, and the geodermatophilaceae relative ASV abundance was 5-fold higher in the dust (∼5%) than the background (∼1%) filters. Other differences in microbial beta diversity between filters were minor and insignificant.
Discussion
Aerosol’s origin and trace-metals affect airborne prokaryotic viability proxy
The physiological state of prokaryotes is indirectly regulated by ribosomal RNA transcription (Klumpp and Hwa, 2009), implying the more SSU rRNA gene copies per cell, the more active they are. Based on this assumption, our results suggest that some airborne prokaryotes indeed remain viable during atmospheric transport (Figures 3A,B), in agreement with previous reports (Womack et al., 2010; DeLeon-Rodriguez et al., 2013). A higher relative viability proxy for airborne prokaryotes was found in marine-origin aerosols, suggesting that humidity in the air is an important factor contributing to their ability to survive in the atmosphere (Leck and Bigg, 2005). Indeed, airborne prokaryotes with marine origin are often embedded in transparent gel-like polymers which protect them from desiccation, and provide them with sustaining nutrients (Aller et al., 2005; Leck and Bigg, 2005; Cunliffe and Murrell, 2009). In agreement, a recent study reported on high concentrations of transparent exopolymer particles in aerosol particles at the tropical Atlantic Ocean (Pinxteren et al., 2022). Moreover, a high percent of airborne prokaryotes in cloud-water/precipitation remain viable during atmospheric transport (Hill et al., 2007; Murata and Zhang, 2016; Stopelli et al., 2017).
We observed that in marine aerosols the relative viability proxy of airborne prokaryotes is affected by certain trace metal concentrations (Figures 4B–D). The negative effect of Cu/Al and Pb/Al (Figure 4D) was attributed to the potential toxicity of these anthropogenic trace metals. The positive relationships between the relative viability proxy and Fe or Al concentrations in air, as proxies for mineral dust content (Figure 4C, Supplementary Figure S1), may be linked to the release of some associated nutrients or the positive role of the particle’s micro-environment. This suggest that airborne prokaryotes may benefit from a particle–associated lifestyle through attachment to particles, especially under humid (marine) conditions. Indeed, large aggregates/particles were shown to protect airborne microbes through shielding from UV, especially over water which also reduces humidity stress (Dowd and Maier, 2000). Moreover, it is possible that the particle-associated microbes utilize micro and macronutrient directly from the particle surface, thereby can remain active and possibly grow. Furthermore, respiration of particle-associated airborne microbes can create a low oxygen micro-zones, as often found on large marine particles (Klawonn et al., 2015), thus “enable”/“ease” the survival of anoxic bacteria during atmospheric transport which is essentially aerial. Indeed, oxygen-sensitive prokaryotes (facultative or obligate) are routinely found on dust samples (Katra et al., 2014; Gat et al., 2017). For example, bacillaceae (order: Bacillales, Phylum: Firmicutes), which comprised 10%–20% of the ASV’s on an order level (Supplementary Figure S2A) and 4%–23% in a family taxonomic level (Supplementary Figure S2C), are considered facultatively anaerobic. While we do not have a direct/specific quantification on their viability, it is reasonable to assume that some of them survive in the atmosphere, especially given that many of them form endospores (Secaira-Morocho et al., 2020). Currently, we cannot state which bacterial phylotypes remained viable during atmospheric transport and were actively growing or involved in specific cellular pathways. This question could be investigated by sequencing efforts of the airborne ribosomal 16S RNA transcripts as well as other specific genes of interest (Alsante et al., 2021). One such gene could be nifH as airborne diazotrophs are routinely found in dust samples (Foster et al., 2009), which, in turn, may fix N2 upon deposition in seawater (Rahav et al., 2018).
Viability proxy of airborne prokaryotes during an extreme dust storm event
The dust storm that arrived at the Southeastern Mediterranean Sea in early September 2015 (starting 7 September 2015) was substantial and relatively prolonged (Rahav et al., 2016b). Estimated increase in inorganic nutrients to the surface seawater were ∼185 nM NO3+NO2 and ∼1.5 nM PO4 (Rahav et al., 2016b), which are up to 50% higher than the typically reported values in this system during the summer (Kress et al., 2019). The concurrent in-situ chlorophyll-a concentration and primary productivity rates increased only moderately a few days after aerosol deposition (Rahav et al., 2016b), suggesting the % of viable airborne bacteria, who can become active upon deposition (Rahav et al., 2018), was relatively low in this specific and unique event. Our results, based on the relative viability proxy analysis, overall agrees with this notation that airborne prokaryotes during the dust storm were “less” active than in “typical” (clear-sky) days. The low levels of the relatively viability proxy during the dust event may be attributed to anthropogenic inhibitors carried by the dust, mainly metals and organic pollutants. Thus, transport time, the presence of pollutants and the chemical reactions on surface particles during the dust event probably impacted the microbial viability, yet further research is needed to assess how physical and chemical microhabitats impacts microorganism functionality (Alsante et al., 2021). Moreover, given the acidic conditions in the atmosphere and clouds (Pye et al., 2020), trace-metals leachability and thus potential toxicity may increase (Koçak et al., 2007). It is therefore not surprising that the relative viability proxy for airborne prokaryotes and potentially toxic trace metals display a negative relationship (Figure 4D). Marine trajectory aerosols, with potentially higher pH (seawater are typically basic, pH = ∼8.15), will likely result in lower trace-metals leachability and thus lower toxicity to airborne prokaryotes, resulting in their higher relative viability (Figure 3B).
In addition to bacillaceae (see above), frankiales and Cytophagales were the most dominant bacterial groups in the dust samples (Supplementary Figure S2). Frankiales (Phylum: Actinobacteria) has high G-C content in their DNA, enabling them to survive (and likely also to remain active) in a wide range of niches, including the atmosphere (Kellogg and Griffin, 2006). Another abundant prokaryote in air is cytophagaceae (order: Cytophagales, phylum: Bacteroidota). Members of this bacterial order are well-known remineralizers of organic matter, and are widely dispersed in top-soils, freshwater and surface seawater (Sun et al., 2018; Rubin-Blum et al., 2022). While we cannot say the percent of viable cells within these bacterial phylotypes, in light of their cellular characteristics it is reasonable to hypothesize they are also the dominant groups among the viable cells.
Techniques to assess airborne microbial viability—The plot thickens
The relatively few aero-microbiology studies over the ocean are mostly based on genetic diversity (Mayol et al., 2017; Mescioglu et al., 2019a), culture or spore-counting techniques (Griffin et al., 2001; Womack et al., 2010; Fernandez et al., 2019), microscopic approaches (DeLeon-Rodriguez et al., 2013), measuring specific metabolic/catabolic microbial processes such as bacterial productivity, N2 fixation and amino-acid synthesis (Rahav et al., 2018; Ruiz-Jimenez et al., 2021), and/or by looking at airborne cell’s RNA expression (DeLeon-Rodriguez et al., 2013; Ruiz-Jimenez et al., 2021 and this study). Each approach has its pros and cons (reviewed in Alsante et al., 2021), but neither of them directly quantifies how many airborne microbes remain viable during their atmospheric transport and which taxa are more likely to survive. Here, we used rRNA transcripts quantification as a proxy for a relative consideration of this scientific challenge throughout a representative year in a coastal marine area subjected to high aerosol deposition (Herut et al., 2016). While we cannot directly calculate the actual % viability of the airborne prokaryotic cells, our result provides the first relative change in the potential viability of airborne prokaryotes during an extreme dust storm event and the days afterwards presenting background conditions, and thus a relative estimate of the potential viability at the Southeastern Mediterranean Sea. We warrant that complimentary approaches such as “live-dead” staining of airborne prokaryotes (e.g., based on membrane integrity or other redox-based vitality approaches), and/or collection of airborne cells into sterile seawater containing RNA-later preservation solution, are required to better quantify % viable cells. Moreover, comparing sequences of 16S rRNA gene (DNA level) and transcript (RNA level) may provide valuable information on the prokaryotes identity and which phylotypes remained viable during atmospheric transport. Understanding the factors that influence airborne bacterial abundance and viability in the atmosphere is an important missing component for further insight into microbial biogeography, connectivity/spreading and diversity (Hervas et al., 2009; Fröhlich-Nowoisky et al., 2016). Such information can also benefit the parameterization of model simulations of bacterial emissions and dispersal (Burrows et al., 2013). Moreover, climate change and desertification projections suggest an increase in dust loads and storm events boosting transoceanic/marine transport, and therefore increasing the ecological significance of airborne prokaryotic microorganisms. We note that much further studies are needed to understand the factors and mechanisms impacting bio-aerosols viability.
Data availability statement
The datasets presented in this study can be found in online repositories. The names of the repository/repositories and accession number(s) can be found below: https://www.ncbi.nlm.nih.gov/, RX272470.
Author contributions
Conceived and designed the experiment: ER. Performed the experiment: ER and BH. Analyzed the data: BH, AP, and ER. Contributed reagents/materials/analysis tools: BH and ER. Wrote the paper: AP, BH, and ER.
Funding
This study was supported by the Israel Science Foundation (grant #1211/17) to BH and ER and by the NSF-OCE (grant #0850467) to AP.
Acknowledgments
The authors gratefully acknowledge Galit Ovadia and Ania Vichik for their help with the RT-qPCR and DNA extraction analyses. We also thank Bella Ben-David from the “Town Association for Environmental Protection (Carmel-Sharon)” for providing the PM2.5 data. The authors gratefully acknowledge the NOAA Air Resources Laboratory (ARL) for the provision of the HYSPLIT transport and dispersion model used in this publication. We also acknowledge the TRACOMED project to BH and ER.
Conflict of interest
The authors declare that the research was conducted in the absence of any commercial or financial relationships that could be construed as a potential conflict of interest.
Publisher’s note
All claims expressed in this article are solely those of the authors and do not necessarily represent those of their affiliated organizations, or those of the publisher, the editors and the reviewers. Any product that may be evaluated in this article, or claim that may be made by its manufacturer, is not guaranteed or endorsed by the publisher.
Supplementary material
The Supplementary Material for this article can be found online at: https://www.frontiersin.org/articles/10.3389/fenvs.2022.900977/full#supplementary-material
References
Aller, J. Y., Kuznetsova, M. R., Jahns, C. J., and Kemp, P. F. (2005). The sea surface microlayer as a source of viral and bacterial enrichment in marine aerosols. J. Aerosol Sci. 36, 801–812. doi:10.1016/j.jaerosci.2004.10.012
Alsante, A. N., Thornton, D. C. O., and Brooks, S. D. (2021). Ocean aerobiology. Front. Microbiol. 12, 764178. doi:10.3389/fmicb.2021.764178
Bräuer, S. L., Adams, C., Kranzler, K., Murphy, D., Xu, M., Zuber, P., et al. (2011). Culturable Rhodobacter and Shewanella species are abundant in estuarine turbidity maxima of the Columbia River. Environ. Microbiol. 13, 589–603. doi:10.1111/j.1462-2920.2010.02360.x
Burrows, S. M., Hoose, C., Poschl, U., and Lawrence, M. G. (2013). Ice nuclei in marine air: Biogenic particles or dust. Atmos. Chem. Phys. 13, 245–267. doi:10.5194/acp-13-245-2013
Cunliffe, M., and Murrell, J. C. (2009). The sea-surface microlayer is a gelatinous biofilm. ISME J. 3, 1001–1003. doi:10.1038/ismej.2009.69
DeLeon-Rodriguez, N., Lathem, T. L., Rodriguez-R, L. M., Barazesh, J. M., Anderson, B. E., Beyersdorf, A. J., et al. (2013). Microbiome of the upper troposphere: Species composition and prevalence, effects of tropical storms, and atmospheric implications. Proc. Natl. Acad. Sci. U. S. A. 110, 2575–2580. doi:10.1073/pnas.1212089110
Després, V. R., Alex Huffman, J., Burrows, S. M., Hoose, C., Safatov, A. S., Buryak, G., et al. (2012). Primary biological aerosol particles in the atmosphere: A review. Tellus B Chem. Phys. Meteorology 64, 15598. doi:10.3402/tellusb.v64i0.15598
Dowd, S., and Maier, R. (2000). Aeromicrobiology. in Environmental microbiology. Editors S. Dowd, and R. Maier. 1st ed. (San Diego: Academic Press).
Fahlgren, C., Hagstrom, A., Nilsson, D., and Zweifel, U. L. (2010). Annual variations in the diversity, viability, and origin of airborne bacteria. Appl. Environ. Microbiol. 76, 3015–3025. doi:10.1128/AEM.02092-09
Favet, J., Lapanje, A., Giongo, A., Kennedy, S., Aung, Y.-Y., Cattaneo, A., et al. (2013). Microbial hitchhikers on intercontinental dust: Catching a lift in Chad. ISME J. 7, 850–867. doi:10.1038/ismej.2012.152
Fernandez, M. O., Thomas, R. J., Garton, N. J., Hudson, A., Haddrell, A., Reid, J. P., et al. (2019). Assessing the airborne survival of bacteria in populations of aerosol droplets with a novel technology. J. R. Soc. Interface 16, 20180779. doi:10.1098/rsif.2018.0779
Flores, J. M., Bourdin, G., Kostinski, A., Boss, E., Sullivan, M. B., Gorsky, G., et al. (2020). Diel cycle of sea spray aerosol concentration over vast areas of the tropical Pacific Ocean and the Caribbean Sea. Res. Sq., 1–25. doi:10.21203/rs.3.rs-74373/v1
Foster, R. A., Paytan, A., and Zehr, J. P. (2009). Seasonality of N2 fixation and nifH gene diversity in the gulf of aqaba (red sea). Limnol. Oceanogr. 54, 219–233. doi:10.4319/lo.2009.54.1.0219
Fragola, M., Perrone, M. R., Alifano, P., Talà, A., and Romano, S. (2021). Seasonal variability of the airborne eukaryotic community structure at a coastal site of the central mediterranean. Toxins (Basel) 13, 518. doi:10.3390/toxins13080518
Fröhlich-Nowoisky, J., Kampf, C. J., Weber, B., Huffman, J. A., Pöhlker, C., Andreae, M. O., et al. (2016). Bioaerosols in the earth system: Climate, health, and ecosystem interactions. Atmos. Res. 182, 346–376. doi:10.1016/j.atmosres.2016.07.018
Gallisai, R., Peters, F., Volpe, G., Basart, S., and Baldasano, J. M. (2014). Saharan dust deposition may affect phytoplankton growth in the Mediterranean sea at ecological time scales. PLoS One 9, e110762. doi:10.1371/journal.pone.0110762
Gat, D., Mazar, Y., Cytryn, E., and Rudich, Y. (2017). Origin-dependent variations in the atmospheric microbiome community in Eastern Mediterranean dust storms. Environ. Sci. Technol. 51, 6709–6718. doi:10.1021/acs.est.7b00362
Giovagnetti, V., Brunet, C., Conversano, F., Tramontano, F., Obernosterer, I., Ridame, C., et al. (2013). Assessing the role of dust deposition on phytoplankton ecophysiology and succession in a low-nutrient low-chlorophyll ecosystem: A mesocosm experiment in the mediterranean sea. Biogeosciences 10, 2973–2991. doi:10.5194/bg-10-2973-2013
Griffin, D. W., Garrison, V. H., R, H. J., and Shinn, E. A. (2001). African desert dust in the Caribbean atmosphere: Microbiology and public health. Aerobiol. (Bologna) 17, 203–213. doi:10.1023/a:1011868218901
Griffin, D. W., Kubilay, N., Koçak, M., Gray, M. a., Borden, T. C., Shinn, E. a., et al. (2007). Airborne desert dust and aeromicrobiology over the Turkish Mediterranean coastline. Atmos. Environ. X. 41, 4050–4062. doi:10.1016/j.atmosenv.2007.01.023
Griffin, D. W. (2007). Atmospheric movement of microorganisms in clouds of desert dust and implications for human health. Clin. Microbiol. Rev. 20, 459–477. doi:10.1128/CMR.00039-06
Guieu, C., Aumont, O., Paytan, A., Bopp, L., Law, C. S., Mahowald, N., et al. (2014). The significance of the episodic nature of atmospheric deposition to Low Nutrient Low Chlorophyll regions. Glob. Biogeochem. Cycles 28, 1179–1198. doi:10.1002/2014gb004852
Hara, K., and Zhang, D. (2012). Bacterial abundance and viability in long-range transported dust. Atmos. Environ. X. 47, 20–25. doi:10.1016/j.atmosenv.2011.11.050
Herut, B., Krom, M. D. D., Pan, G., and Mortimer, R. (1999). Atmospheric input of nitrogen and phosphorus to the Southeast Mediterranean: Sources, fluxes, and possible impact. Limnol. Oceanogr. 44, 1683–1692. doi:10.4319/lo.1999.44.7.1683
Herut, B., Collier, R., and Krom, M. D. (2002). The role of dust in supplying nitrogen and phosphorus to the southeast Mediterranean. Limnol. Oceanogr. 47, 870–878. doi:10.4319/lo.2002.47.3.0870
Herut, B., Rahav, E., Tsagaraki, T. M., Giannakourou, A., Tsiola, A., Psarra, S., et al. (2016). The potential impact of Saharan dust and polluted aerosols on microbial populations in the east Mediterranean Sea, an overview of a mesocosm experimental approach. Front. Mar. Sci. 3, 226. doi:10.3389/fmars.2016.00226
Herut, B., Nimmo, M., Medway, A., Chester, R., and Krom, M. D. (2001). Dry atmospheric inputs of trace metals at the Mediterranean coast of Israel (SE Mediterranean): Sources and fluxes. Atmos. Environ. 35, 803–813. doi:10.1016/S1352-2310(00)00216-8
Hervas, A., Camarero, L., Reche, I., and Casamayor, E. O. (2009). Viability and potential for immigration of airborne bacteria from Africa that reach high mountain lakes in Europe. Environ. Microbiol. 11, 1612–1623. doi:10.1111/j.1462-2920.2009.01926.x
Hill, K. A., Shepson, P. B., Galbavy, E. S., Anastasio, C., Kourtev, P. S., Konopka, A., et al. (2007). Processing of atmospheric nitrogen by clouds above a forest environment. J. Geophys. Res. 112, D11. doi:10.1029/2006JD008002
Hill, P. G., Zubkov, M. V., and Purdie, D. A. (2010). Differential responses of Prochlorococcus and SAR11-dominated bacterioplankton groups to atmospheric dust inputs in the tropical Northeast Atlantic Ocean. FEMS Microbiol. Lett. 306, 82–89. doi:10.1111/j.1574-6968.2010.01940.x
Hu, W., Murata, K., Fukuyama, S., Kawai, Y., Oka, E., Uematsu, M., et al. (2017). Concentration and viability of airborne bacteria over the Kuroshio extension region in the northwestern Pacific ocean: Data from three cruises. J. Geophys. Res. Atmos. 122 (12), 892–912. doi:10.1002/2017JD027287
Jordi, A., Basterretxea, G., Tovar-Sánchez, A., Alastuey, A., and Querol, X. (2012). Copper aerosols inhibit phytoplankton growth in the Mediterranean Sea. Proc. Natl. Acad. Sci. U. S. A. 109, 21246–21249. doi:10.1073/pnas.1207567110
Katra, I., Arotsker, L., Krasnov, H., Zaritsky, A., Kushmaro, A., Ben-Dov, E., et al. (2014). Richness and diversity in dust stormborne biomes at the southeast mediterranean. Sci. Rep. 4, 5265. doi:10.1038/srep05265
Kellogg, C., and Griffin, D. W. (2006). Aerobiology and the global transport of desert dust. Trends Ecol. Evol. 21, 638–644. doi:10.1016/j.tree.2006.07.004
Kellogg, C., Griffin, D. W., Garrison, V. H., Peak, K. K., Royall, N., Smith, R., et al. (2004). Characterization of aerosolized bacteria and fungi from desert dust events in Mali, West Africa. Aerobiol. (Bologna) 20, 99–110. doi:10.1023/b:aero.0000032947.88335.bb
Klawonn, I., Bonaglia, S., Brüchert, V., and Ploug, H. (2015). Aerobic and anaerobic nitrogen transformation processes in N2-fixing cyanobacterial aggregates. ISME J. 9, 1456–1466. doi:10.1038/ismej.2014.232
Klumpp, S., and Hwa, T. (2009). Traffic patrol in the transcription of ribosomal RNA. RNA Biol. 6, 392–394. doi:10.4161/rna.6.4.8952
Koçak, M., Kubilay, N., Herut, B., and Nimmo, M. (2007). Trace metal solid state speciation in aerosols of the northern Levantine Basin, East Mediterranean. J. Atmos. Chem. 56, 239–257. doi:10.1007/s10874-006-9053-7
Kress, N., Rahav, E., Silverman, J., and Herut, B. (2019). Environmental status of Israel’s Mediterranean coastal waters: Setting reference conditions and thresholds for nutrients, chlorophyll-a and suspended particulate matter. Mar. Pollut. Bull. 141, 612–620. doi:10.1016/j.marpolbul.2019.02.070
Lawrence, C. R., and Neff, J. C. (2009). The contemporary physical and chemical flux of aeolian dust: A synthesis of direct measurements of dust deposition. Chem. Geol. 267, 46–63. doi:10.1016/j.chemgeo.2009.02.005
Leck, C., and Bigg, E. K. (2005). Source and evolution of the marine aerosol - a new perspective. Geophys. Res. Lett. 32, 1–4. doi:10.1029/2005GL023651
Mayol, E., JimÃnez, M. A., Herndl, G. J., Duarte, C. M., and Arrieta, J. M. (2014). Resolving the abundance and air-sea fluxes of airborne microorganisms in the North Atlantic Ocean. Front. Microbiol. 5, 557. doi:10.3389/fmicb.2014.00557
Mayol, E., Arrieta, J. M., Jiménez, M. A., Martínez-Asensio, A., Garcias-Bonet, N., Dachs, J., et al. (2017). Long-range transport of airborne microbes over the global tropical and subtropical ocean. Nat. Commun. 8, 201. doi:10.1038/s41467-017-00110-9
Mescioglu, E., Rahav, E., Belkin, N., Xian, P., Eizenga, J. M., Vichik, A., et al. (2019a). Aerosol microbiome over the Mediterranean Sea diversity and abundance. Atmos. (Basel) 10, 440. doi:10.3390/atmos10080440
Mescioglu, E., Rahav, E., Frada, M. J., Rosenfeld, S., Raveh, O., Galletti, Y., et al. (2019b). Dust-associated airborne microbes affect primary and bacterial production rates, and eukaryotes diversity in the Northern Red Sea: A mesocosm approach. Atmos. (Basel) 10, 358. doi:10.3390/atmos10070358
Moore, M. C., Mills, M. M., Achterberg, E. P., Geider, R. J., LaRoche, J., Lucas, M. I., et al. (2009). Large-scale distribution of Atlantic nitrogen fixation controlled by iron availability. Nat. Geosci. 2, 867–871. doi:10.1038/ngeo667
Moore, C. M., Mills, M. M., Arrigo, K. R., Berman-Frank, I., Bopp, L., Boyd, P. W., et al. (2013). Processes and patterns of oceanic nutrient limitation. Nat. Geosci. 6, 701–710. doi:10.1038/ngeo1765
Murata, K., and Zhang, D. (2016). Concentration of bacterial aerosols in response to synoptic weather and land-sea breeze at a seaside site downwind of the Asian continent. JGR. Atmos. 121, 11636–11647. doi:10.1002/2016JD025028
Paytan, A., Mackey, K. R. M., Chen, Y., Lima, I. D., Doney, S. C., Mahowald, N., et al. (2009). Toxicity of atmospheric aerosols on marine phytoplankton. Proc. Natl. Acad. Sci. U. S. A. 106, 4601–4605. doi:10.1073/pnas.0811486106
Peng, X., Gat, D., Paytan, A., and Rudich, Y. (2021). The response of airborne mycobiome to dust storms in the eastern mediterranean. J. Fungi (Basel). 7, 802. doi:10.3390/jof7100802
Peter, H., Hörtnagl, P., Reche, I., and Sommaruga, R. (2014). Bacterial diversity and composition during rain events with and without Saharan dust influence reaching a high mountain lake in the Alps. Environ. Microbiol. Rep. 6, 618–624. doi:10.1111/1758-2229.12175
Pinxteren, M. V., Robinson, T., Zeppenfeld, S., Gong, X., Bahlmann, E., Fomba, K. W., et al. (2022). High number concentrations of transparent exopolymer particles in ambient aerosol particles and cloud water – a case study at the tropical Atlantic Ocean. Atmos. Chem. Phys. 22, 5725–5742. doi:10.5194/acp-22-5725-2022
Polymenakou, P. N. (2012). Atmosphere: A source of pathogenic or beneficial microbes? Atmos. (Basel) 3, 87–102. doi:10.3390/atmos3010087
Prospero, J. M., Blades, E., Mathison, G., and Naidu, R. (2005). Interhemispheric transport of viable fungi and bacteria from Africa to the Caribbean with soil dust. Aerobiol. (Bologna) 21, 1–19. doi:10.1007/s10453-004-5872-7
Pye, H. O. T., Nenes, A., Alexander, B., Ault, A. P., Barth, M. C., Clegg, S. L., et al. (2020). The acidity of atmospheric particles and clouds. Atmos Chem Phys 20, 4809. doi:10.5194/acp-20-4809-2020
Rahav, E., Ovadia, G., Paytan, A., and Herut, B. (2016a). Contribution of airborne microbes to bacterial production and N2 fixation in seawater upon aerosol deposition. Geophys. Res. Lett. 43, 719–727. doi:10.1002/2015GL066898
Rahav, E., Paytan, A., Chien, C.-T., Ovadia, G., Katz, T., Herut, B., et al. (2016b). The impact of atmospheric dry deposition associated microbes on the southeastern mediterranean sea surface water following an intense dust storm. Front. Mar. Sci. 3, 127. doi:10.3389/fmars.2016.00127
Rahav, E., Paytan, A., Mescioglu, E., Galletti, Y., Rosenfeld, S., Raveh, O., et al. (2018). Airborne microbes contribute to N2 fixation in surface water of the Northern Red Sea. Geophys. Res. Lett. 45, 6186. doi:10.1029/2018GL077132
Rahav, E., Belkin, N., Paytan, A., and Herut, B. (2019). The relationship between air-mass trajectories and the abundance of dust-borne prokaryotes at the SE Mediterranean sea. Atmos. (Basel) 10, 280. doi:10.3390/atmos10050280
Rahav, E., Paytan, A., Mescioglu, E., Bar-zeev, E., Mart, F., Xian, P., et al. (2020). Bio-aerosols negatively affect Prochlorococcus in oligotrophic aerosol-rich marine regions. Atmos. (Basel) 11. 540. doi:10.3390/atmos11050540
Rubin-Blum, M., Sisma-Ventura, G., Yudkovski, Y., Belkin, N., Kanari, M., Herut, B., et al. (2022). Diversity, activity, and abundance of benthic microbes in the Southeastern Mediterranean Sea. FEMS Microbiol. Ecol. 98, fiac009. doi:10.1093/femsec/fiac009
Ruiz-Jimenez, J., Okuljar, M., Sietiö, O. M., Demaria, G., Liangsupree, T., Zagatti, E., et al. (2021). Determination of free amino acids, saccharides, and selected microbes in biogenic atmospheric aerosols - seasonal variations, particle size distribution, chemical and microbial relations. Atmos. Chem. Phys. 21, 8775–8790. doi:10.5194/acp-21-8775-2021
Secaira-Morocho, H., Castillo, J. A., and Driks, A. (2020). Diversity and evolutionary dynamics of spore-coat proteins in spore-forming species of Bacillales. Microb. Genom. 6, mgen000451. doi:10.1099/mgen.0.000451
Siokou-Frangou, I., Christaki, U., Mazzocchi, M. G., Montresor, M., Ribera d’Alcalá, M., Vaqué, D., et al. (2010). Plankton in the open Mediterranean sea: A review. Biogeosciences 7, 1543–1586. doi:10.5194/bg-7-1543-2010
Sisma-Ventura, G., Kress, N., Silverman, J., Gertner, Y., Ozer, T., Biton, E., et al. (2021). Post-eastern mediterranean transient oxygen decline in the deep waters of the Southeast Mediterranean sea supports weakening of ventilation rates. Front. Mar. Sci. 7, 598686. doi:10.3389/fmars.2020.598686
Stopelli, E., Conen, F., Guilbaud, C., Zopfi, J., Alewell, C., Morris, C. E., et al. (2017). Ice nucleators, bacterial cells and Pseudomonas syringae in precipitation at Jungfraujoch. Biogeosciences 14, 1189–1196. doi:10.5194/bg-14-1189-2017
Sun, Y., Xu, S., Zheng, D., Li, J., Tian, H., Wang, Y., et al. (2018). Effects of haze pollution on microbial community changes and correlation with chemical components in atmospheric particulate matter. Sci. Total Environ. 637–638, 507–516. doi:10.1016/j.scitotenv.2018.04.203
Tang, J. W. (2009). The effect of environmental parameters on the survival of airborne infectious agents. J. R. Soc. Interface 6, S737–S746. doi:10.1098/rsif.2009.0227.focus
Torfstein, A., Teutsch, N., Tirosh, O., Shaked, Y., Rivlin, T., Zipori, A., et al. (2017). Chemical characterization of atmospheric dust from a weekly time series in the north Red Sea between 2006 and 2010. Geochim. Cosmochim. Acta 211, 373–393. doi:10.1016/j.gca.2017.06.007
Tsagaraki, T. M., Herut, B., Rahav, E., Berman Frank, I. R., Tsiola, A., Tsapakis, M., et al. (2017). Atmospheric deposition effects on plankton communities in the eastern mediterranean: A mesocosm experimental approach. Front. Mar. Sci. 4, 1–17. doi:10.3389/fmars.2017.00210
Womack, A. M., Bohannan, B. J. M., and Green, J. L. (2010). Biodiversity and biogeography of the atmosphere. Phil. Trans. R. Soc. B 365, 3645–3653. doi:10.1098/rstb.2010.0283
Keywords: viable airborne prokaryotes, aerosols, trace-metals, Southeastern Mediterranean, dust
Citation: Rahav E, Paytan A and Herut B (2022) Relative viability proxy of airborne prokaryotic microorganisms at the Southeastern Mediterranean coastal Sea. Front. Environ. Sci. 10:900977. doi: 10.3389/fenvs.2022.900977
Received: 21 March 2022; Accepted: 11 July 2022;
Published: 22 July 2022.
Edited by:
Uma Das, Indian Institute of Information Technology Kalyani, IndiaReviewed by:
Kento Tominaga, The University of Tokyo, JapanAngelina Lo Giudice, Institute of Polar Sciences (CNR), Italy
Copyright © 2022 Rahav, Paytan and Herut. This is an open-access article distributed under the terms of the Creative Commons Attribution License (CC BY). The use, distribution or reproduction in other forums is permitted, provided the original author(s) and the copyright owner(s) are credited and that the original publication in this journal is cited, in accordance with accepted academic practice. No use, distribution or reproduction is permitted which does not comply with these terms.
*Correspondence: E. Rahav, ZXlhbC5yYWhhdkBvY2Vhbi5vcmcuaWw=