- College of Land and Environment, Shenyang Agricultural University, National Engineering Research Center for Efficient Utilization of Soil and Fertilizer Resources, National Development and Reform Commission, Northeast Key Laboratory of Cultivated Land Conservation, Ministry of Agriculture and Rural Affairs, Shenyang, China
Brown earth is one of the typical soils in the dryland areas of Northeast China, and its degradation is closely related to food security in the local. Effectively preventing soil nitrogen (N) loss can promote the soil fertility supply. As the hub of nitrogen cycling, microorganisms play an important role in N transformation and accumulation. Soil aggregates are important in improving soil fertility and preventing soil degradation because they are an important index to maintain soil fertility. However, the allocation of microbial residual N and its contribution to total N in brown earth’s aggregates are still limited, especially the effects of different maize residue types’ return and soil fertility levels. Focusing on this, a 360-day laboratory incubation experiment at 25°C was carried out induced by adding maize roots and shoots into brown earth with low (L) and high (H) fertility, respectively. Randomized soil samples were taken on the incubation day of 0, 30, 60, 180, and 360, and then, they were divided into macroaggregates (>250 μm) and microaggregates (<250 μm) using a dry-sieved method. The relative contributions of fungal and bacterial residual N to soil total N were calculated by the amino sugar content to examine the accumulated differences of microbial residual N in brown earth’s aggregates with the addition of different parts of maize residues and fertility levels. The results showed that maize residue types had different effects on the fungal and bacterial residual N enrichment in soil aggregates. In macroaggregates, maize roots promoted the accumulation of fungal residual N. The fungal residual N contributions to total nitrogen with root treatments were 1.03 times more than those with shoot treatments. However, in microaggregates, the bacterial residual N contributions to total nitrogen with shoot treatments were 1.01 times more than those with root treatments. These indicated that maize roots should be more beneficial to the accumulation of bacterial residual N in microaggregates. Moreover, the high fertility soil could sequester more microbial residual N than the low fertility soil, showing the content of microbial residual N in high fertility was 1.12–1.18 times more than that in low fertility. Furthermore, the fungal residual N was more beneficially accumulated in low fertility soil. Regardless of the level of fertility, the proportion of N in total N with shoot treatment was higher than that with root treatment, indicating that the above ground maize residues could better promote the metabolic process of microorganisms than the below ground ones. These could provide a theoretical basis for studying the microbial transformation mechanism of nitrogen after maize straw returning to the field, which could be of great significance to main soil fertility.
Highlights
Microbial residual N was quantified using amino sugar analysis.
Maize residues returning could increase microbial residual N in soil aggregates.
Residue types had different effects on the accumulation of residual nitrogen in aggregates.
High fertility soil could sequester more microbial residual N than low fertility soil.
Bacterial residual N was more accumulated in high fertility soil, while fungal N in low fertility soil.
Introduction
It is an important grain production base for the dryland in Northeast China, whose area accounts for over 85% of the local region, guaranteeing national grain security (Han and Li, 2018). Brown earth (Hapli-Udic Cambisol, FAO Classification) is one of the important dryland soils of black land in Northeast China. However, for a long time, unreasonable agricultural production and management have caused serious soil degradation in the local (Liu et al., 2010). As a result, the nutrient ratio of dryland brown earth in Northeast China is unbalanced, with nitrogen loss and organic matter decreasing year by year.
Nitrogen is always considered to be the element with rapid turnover and loss in soil, and preventing its loss can promote soil fertility supplied to plants (Schlesinger et al., 1990; Wang et al., 2019). In addition, microorganisms can not only provide the driving force for nitrogen cycling and transformation but also act as the “source” and “sink” of nitrogen internal cycling (Jawson et al., 1989). Moreover, as an important indicator of soil fertility, soil aggregates contain a large amount of nitrogen and play an important role in regulating the dynamic change of soil nitrogen (Six et al., 2004). Likewise, they also influence the microenvironment in which microorganisms live to a certain extent (such as water and gas conditions and porosity), thereby affecting the microbial metabolic activities (Schutter and Dick, 2002; Ruamps et al., 2011). Thus, soil microorganisms, aggregates, and nitrogen are allowed to interact with each other, maintaining a certain balance in soil ecosystems to resist soil degradation.
For the farmland ecosystems, the addition of exogenous organic matter, such as maize (Zea mays L.) residues, would disturb this balance. Meanwhile, microbial decomposition of maize residues is an important process, showing parts of the nitrogen from maize residues would be assimilated by microorganisms, and the most of the assimilated nitrogen could be stored and accumulated in the dead microbial residual components (such as amino sugars), becoming an important reservoir of nitrogen in the soil (Engelking et al., 2007; Ding et al., 2011). Compared with the living microorganisms, the dead microbial residues may have a more lasting promoting effect on the formation and stability of aggregates to improve soil fertility (Tisdall and Oades, 1982; Chantigny et al., 1997). Therefore, understanding the distribution of the microbial residual nitrogen in soil aggregates after maize residues’ return, and further study on the role of microorganisms in nitrogen accumulation in aggregates is of great significance in the rational management of dryland nitrogen nutrients and soil fertility improvement.
As one of the major contributors to mineral nitrogen, amino sugars from microorganisms account for 5–10% of organic nitrogen (Roberts et al., 2007; Liang et al., 2019). They are the residues of microbial cell walls, relatively stable in the soil, representing the long-term remaining effects of dead microorganisms (Amelung et al., 2001). Among them, glucosamine mainly comes from fungi, while muramic acid only comes from bacteria (Joergensen, 2018; Lu et al., 2021). Due to the heterogenicity of glucosamine and muramic acid, the relative contribution of fungal and bacterial nitrogen to total nitrogen in soil aggregates can be calculated by the contents of glucosamine and muramic acid (Joergensen, 2018; Lu et al., 2021). Previous studies provide a methodological support for exploring the microbial mechanism of soil nitrogen accumulation by the combined ecosystem approaches with elemental carbon and nitrogen stoichiometry using amino sugar data published between 1996 and 2018 (Liang et al., 2019). Consequently, amino sugars were selected as a marker to study the dynamic changes of microbial residual nitrogen in soil.
Due to the different chemical compositions of carbon and nitrogen in different parts of maize residues (such as roots and shoots), they can cause microbial disturbance differences after the ones returned to the field (Abiven et al., 2005). The Microbial Efficiency-Matrix Stabilization (MEMS) framework indicated that the quality of plant litter could affect microbial biomass; high quality litters have a high microbial catabolism ratio, resulting in more microbial residues per amount of plant litter metabolism; low quality litters have a relatively low microbial biomass, resulting in fewer microbial residues per amount of plant litter metabolism (Castellano et al., 2015). Moreover, different soil fertility levels will have different effects on metabolic activities of soil microorganisms due to the different substrates in physicochemical properties and microbial community structures, resulting in the varied distributions of microbial residues in soil aggregates (An et al., 2015; Bonanomi et al., 2017; Xu et al., 2019). Some studies reported that high fertility soil had higher microbial biomass, larger aggregate ratio, enzyme activity, and different microbial community compositions than low fertility soil, which could influence the assimilation of microbial residues (Paul et al., 2002; Marschner et al., 2003; Fontaine et al., 2011; Wang et al., 2017; Xu et al., 2020).
From the previously stated facts, at present, there are few comprehensive studies on the change of residual nitrogen in soil aggregates with different fertility under the different types of maize residues by using amino sugars as microbial markers to reflect the changes of residual nitrogen. Some of them have shown that the portion of microbially derived soil organic N (SON) to total SON was higher than the portion of microbially derived SOC to total SOC, indicating the importance of microbially derived N to soil N cycling (Simpson et al., 2007; Chao Wang et al., 2020). Therefore, to examine the microbial residual nitrogen accumulation in the dryland soil aggregates, the relative contribution of fungal and bacterial-derived residual nitrogen to the total nitrogen of aggregates should be figured out; we conducted an experiment to monitor the variations of amino sugars with different maize residues’ parts (roots and shoots) added into the dryland brown earth with high and low fertility. We hypothesized that (1) maize residue types differed in the accumulation of microbial residues (fungal and bacterial residues) in different aggregates; (2) shoots should be more favorable for the accumulation of microbial residues in aggregates because the nutrient in shoots is easier to be utilized by microorganisms than that by roots; and (3) fertility level and residue types would interact with the accumulation of microbial residual nitrogen in aggregates.
Materials and Methods
Tested Materials
Tested Soil
The topsoil (0–20 cm) with high and low fertility was collected from the brown earth long-term positioning experimental station of Shenyang Agricultural University (41°49′ N, 123°34’ E, elevation of 75 m), which was established in 1987. The typical local soil is brown earth (Hapli-Udic Cambisol, FAO Classification) with loess parent material. The climate is a northern temperate continental monsoon climate with an average annual temperature of 7–8°C and an average annual rainfall of 730 mm that is concentrated in summer. Maize (Zea mays L.) is a long-term continuous cropping crop in this station. It is usually fertilized and sown in early May and harvested in late September per year. The high fertility soil (HF) was treated with a high amount of organic fertilizer (annual application of manure fertilizer was equivalent to 135 kg N hm−2, and the manure was the pig compost that contained 150 g kg−1 organic carbon, 10 g kg−1 N, 4.4 g kg−1 phosphorus (P), and 3.3 g kg−1 potassium (K) on a dry weight basis) combined with chemical N and P fertilizers (chemical fertilizers with urea N 75 kg hm−2 and P2O5 67.5 kg hm−2). The low fertility soil was the control treatment without fertilization (LF). The detailed differences between the treatments of high and low fertility soils are shown in Table 1. The collected brown earth sample was air-dried at room temperature by the combination of five random sampling points and then removed the visible roots and gravel. The soil was screened through a 2-mm sieve for the next step use. The basic physicochemical properties of the tested soil are shown in Table 1.
Tested Maize Residues
The tested maize materials were maize roots and shoots (including stem and leaf). After harvest, the maize residues collected in the field were first oven-dried at 105°C for 30 min and then oven-dried at 60°C for 8 h. After separating the roots from the shoots, they were respectively cut into 2-cm sections, ground with a grinder, and then passed through a 40-mesh sieve. The basic physicochemical properties are shown in Table 2.
Incubation Experiment
The samples of 250 g with 2 mm air-dried soil (drying weight) were weighed and placed in a 1000-ml incubation flask. The soil moisture content was adjusted to 40% of field water capacity for pre-active microorganisms, and the lid with holes (the diameter of the lid was 11 cm, and 20 pores of the same size were tied evenly) was put on the incubation flask to seal at the incubation temperature of 25oC for 7 days without light. After the pre-incubation time, the maize residue samples (roots and shoots) of 5 g (2% of air-dried soil weight) were thoroughly mixed with the pre-incubation soil, and then, the samples were sealed and placed in an incubator (25oC) for 360 days. During incubation time, the soil water content was kept at 60% of field capacity using the weighing method. There were six treatments in this incubation experiment (Figure 1): (1) low fertility brown earth with maize roots (LF + R), (2) low fertility brown earth with maize shoots (LF + S), (3) high fertility brown earth with maize roots (HF + R), (4) high fertility brown earth with maize shoots (HF + S), and (5) low (LF) or (6) high (HF) fertility brown earth without any maize residues. Each treatment was repeated three times. The samples were randomly taken on the 30, 60, 180, and 360 days after incubation. The aggregates of them were sieved at two levels (>250 μm and <250 μm) and weighed using a dry-sieving method (Dorodnikov et al., 2009; Gunina and Kuzyakov, 2014). Then, these aggregate samples were air-dried; one part was sieved through a 100-mesh sieve for soil carbon and nitrogen content determination, and the other part was extracted to determine amino sugars.
Soil Aggregates Separation
The moisture contents of fresh soil samples were uniformly regulated to about 10% using the air-dried method at 4°C. A 100 g soil sample was placed in an automatic sieving apparatus (Retsch AS 200, Germany) with a sieve hole size of 250 μm. The sample was sieved into macroaggregates (>250 μm) and microaggregates (<250 μm) with a vibration amplitude of 1.5 mm for 2 min (Dorodnikov et al., 2009; Gunina and Kuzyakov, 2014).
Soil Sample Analysis
The Contents of Soil Organic Carbon and Total Nitrogen
The soil samples were air-dried and passed through a 100-mesh sieve, and the contents of total organic carbon and total nitrogen in soil were determined using an elemental analyzer (EA, Germany).
Soil Amino Sugars
The content of amino sugars in soil samples was determined using Zhang’s method (Zhang and Amelung, 1996). The brief steps are as follows: 0.4 mg nitrogen of soil samples were placed in the hydrolyzing flask and hydrolyzed at 105oC for 8 h. The cooled samples were successively steamed, and the pH of the dissolved solution was adjusted to neutral with 0.4 mol L−1 KOH and dilute HCL. Those solutions were centrifuged (3000rpm min−1 for 10 min) and rotated; then, the supernatant (amino sugar part) obtained was transferred to a 5-ml derivative bottle for nitrogen blowing (to remove anhydrous methanol), and 1 ml DDI water and 100 μL of n-methylglucosamine (internal standard 2) were added to the dried supernatant. Then, the derivative bottles were tightened with a sealing film, and the substance was shaken in the derivative bottle well by hand. Three 5 ml derived vials were taken as standard samples, and 100 μL of cytotic acid was added to each of them. After nitrogen blowing, 100 μL of mixed standard (D-(+) -glucosamine, D-(+)–galactosamine, D-(+) -mannoamine), 100 μL of inositol, 100 μL of n-methylglucosamine, and 1 ml of DDI water were added. After sealing and shaking well, the samples were allowed for freeze drying.
The 300 μL of derivative reagents (cyanidation reaction) were added to each dried derivative bottle, followed by two water baths of duration 30 min and 60 min. During the two water baths, 1 ml of acetic anhydride and 1.5 ml of dichloromethane were added sequentially. Then, 1 ml 1 mol L−1 HCl and 3 ml DI water (1 ml per time) were used to extract the organic phase. In the final extraction, water was removed as much as possible. The extracted organic phase was dried with nitrogen at 45°C, and 200 μL ethylacetate-n-hexane mixture (1:1) liquids were added to dissolve the dry matter. Then, those were transferred into a gas chromatographic bottle with a liner for use.
Calculations
The formula for calculating the nitrogen content of microbial residues in each grain size of soil is as follows (Liang et al., 2019):
Microbial residual nitrogen content (g kg−1) = fungal residual nitrogen + bacterial residual nitrogen, where fungal residual nitrogen (g kg−1) = (glucosamine (mmol)/179.17–2 × muramic acid (mmol)/251.23) × 179.17 × 1.4 and bacterial residual nitrogen (g kg−1) = muramic acid (mmol) × 6.67.
Assuming the molar ratio of glucosamine to wall acid in bacterial cells is 2:1. Among them, 179.17 was the molecular weight of glucosamine, 251.23 was the molecular weight of muramic acid, 1.4 was the conversion coefficient of glucosamine to fungal residual nitrogen, and 6.67 was the conversion coefficient of muramic acid to bacterial residual nitrogen.
Statistics
The software of Origin 2019 and Microsoft Office Excel 2013 were used to process and plot the experimental data, and SPSS 25.0 software was used to analyze the variance of the experimental results. Repeated measurement analysis of variance was used for significance among different treatments, and the significance level was p < 0.05. Multiple linear regression analysis was conducted to partition the effects of carbon content, nitrogen content, and aggregate composition on the content of microbial, fungal, and bacterial residues of nitrogen.
Results
Contents of Glucosamine and Muramic Acid in Soil Aggregates
The contents of glucosamine and muramic acid in macro-and micro-aggregates with maize residue addition were different (Figure 2), showing the contents of glucosamine in macro- and micro-aggregates were 638.32–935.70 mg kg−1 and 550.14–969.55 mg kg−1, respectively. Moreover, the contents of muramic acid in macro- and micro-aggregates were 29.00–54.87 mg kg−1 and 28.87–68.16 mg kg−1, respectively.
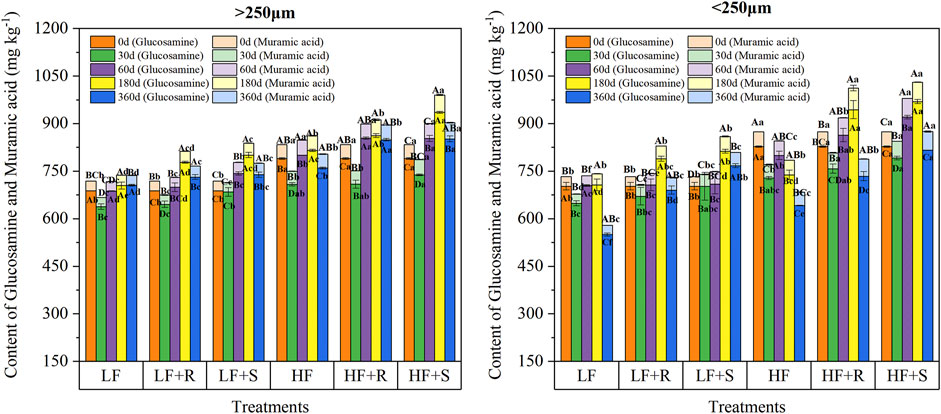
FIGURE 2. Contents of glucosamine and muramic acid in soil aggregates with different fertility levels after the addition of different parts of maize residues. Note: LF means low fertility, LF + R means low fertility + roots, and LF + S means low fertility + Shoots; HF means high fertility, HF + R means high fertility + roots, and HF + S means high fertility + Shoots. Uppercase letters represent differences between different periods in the same treatment, and lowercase letters represent differences between different treatments at the same period. Uppercase letters represent differences between different periods in the same treatment, and lowercase letters represent differences between different treatments at the same period.
Nitrogen Contents of Microbial Residues in Soil Aggregates
The soil fertility level and type of maize residues had extremely significant effects on the content of microbial, fungal, and bacterial residues of nitrogen (p < 0.001) (Figure 3, Figure 4 and Figure 5). The aggregate level had a significant effect on the content of microbial and bacterial residual nitrogen (p < 0.05). The contents of microbial and fungal residual nitrogen added with maize residues showed a decreasing trend at the early stage of incubation time in all levels of aggregates, showing the lowest on 30 days and then gradually increasing to the peak on 180 days. Those were still significantly higher than that of the control treatment on 360 days (p < 0.05). The content of microbial and fungal residual nitrogen in aggregates was higher in microaggregates than that in macroaggregates at the beginning of incubation time. Meanwhile, they were higher in macroaggregates than that in microaggregates from 180 days to the end of incubation, and the content of fungal residues of nitrogen in macroaggregates was significantly higher than that in microaggregates at the end of incubation (p = 0.001). However, the content of bacterial residual nitrogen increased briefly and then decreased in the treatment with shoots in the macroaggregates of high fertility soil. It maintained an increasing trend in the microaggregates until reaching the peak on 180 days and then gradually decreased until the end of incubation. The contents of bacterial residual nitrogen in all aggregates showed macroaggregates > microaggregates. There were significant differences on the 30, 60, and 360 days (p < 0.05).
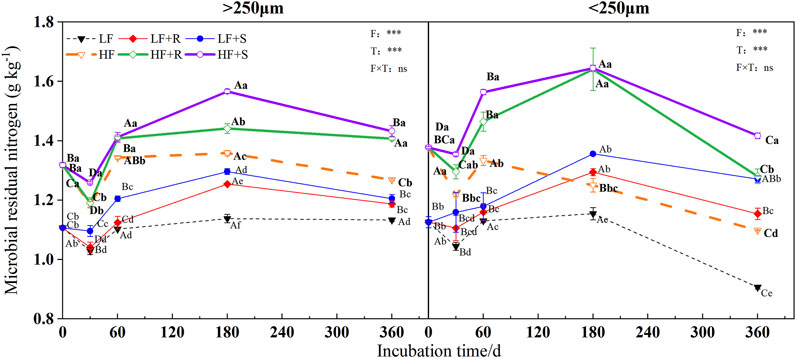
FIGURE 3. Microbial residual nitrogen in soil aggregates with different fertility levels after the addition of different parts of maize residues. Note: LF means low fertility, LF + R means low fertility + roots, and LF + S means low fertility + Shoots; HF means high fertility, HF + R means high fertility + roots, and HF + S means high fertility + Shoots. Uppercase letters represent differences between different periods in the same treatment, and lowercase letters represent differences between different treatments at the same period. For the ANOVA, F means fertility level, T means the type of maize residues, and F × T means fertility level × type of maize residues. *,**, and *** mean p < 0.05, 0.01, and 0.001, respectively. ns means no significance.
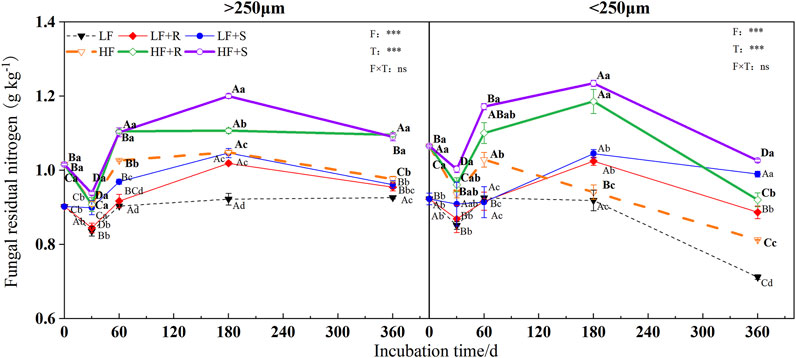
FIGURE 4. Fungal residual nitrogen in soil aggregates with different soil fertility levels after the addition of different parts of maize residues. Note: LF means low fertility, LF + R means low fertility + roots, and LF + S means low fertility + Shoots; HF means high fertility, HF + R means high fertility + roots, and HF + S means high fertility + Shoots. Uppercase letters represent differences between different periods in the same treatment, and lowercase letters represent differences between different treatments at the same period. For the ANOVA, F means fertility level, T means the type of maize residues, and F × T means fertility level × type of maize residues. *,**, and *** mean p < 0.05, 0.01, and 0.001, respectively. ns means no significance.
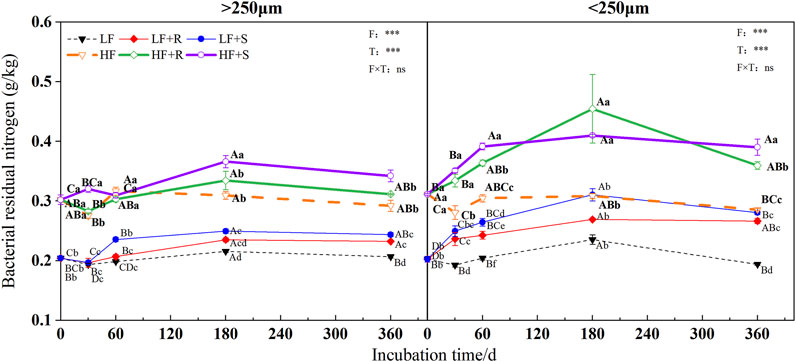
FIGURE 5. Bacterial residual nitrogen in soil aggregates with different fertility levels after the addition of different parts of maize residues. Note: LF means low fertility, LF + R means low fertility + roots, and LF + S means low fertility + Shoots; HF means high fertility, HF + R means high fertility + roots, and HF + S means high fertility + Shoots. Uppercase letters represent differences between different periods in the same treatment, and lowercase letters represent differences between different treatments at the same period. For the ANOVA, F means fertility level, T means type of maize residues, and F × T means fertility level × type of maize residues. *,**, and *** mean P<0.05, 0.01 and 0.001, respectively. ns means no significance.
After 1 year incubation, compared with the control, the addition of maize residues increased the content of microbial, fungal, and bacterial residual nitrogen in aggregates with high and low fertility levels to different degrees. The content of residual nitrogen in aggregates at all levels showed HF > LF (p < 0.05). In the macroaggregates, the contents of microbial residual nitrogen were 1.42 g kg−1 (HF) and 1.20 g kg−1 (LF). The nitrogen contents of fungal residues were 1.09 g kg−1 (HF) and 0.96 g kg−1 (LF) and those of bacterial residues were 0.33 g kg−1 (HF) and 0.25 g kg−1 (LF). In the microaggregates, the contents of microbial residual nitrogen were 1.35 g kg−1 (HF) and 1.21 g kg−1 (LF), those of fungal residual nitrogen were 0.97 g kg−1 (HF) and 0.94 g kg−1 (LF), and those of bacterial residual nitrogen were 0.37 g kg−1 (HF) and 0.27 g kg−1 (LF).
The effects of different types of maize residues on the content of microbial, fungal, and bacterial residual nitrogen in aggregates at all levels were shown by S > R at the end of incubation. In the macroaggregates, the content of microbial residual nitrogen with shoots was 1.02 times more than that with root treatment, and the content of fungal residual nitrogen with shoots was 1.00 times more than that with root treatment. In addition, the content of bacterial residual nitrogen with shoots was 1.08 times more than that with root treatment. In the microaggregates, the content of microbial residual nitrogen with shoots was 1.10 times more than that with root treatment, and the content of fungal residual nitrogen with shoots was 1.12 times more than that with root treatment. In addition, the content of bacterial residual nitrogen with shoots was 1.07 times more than that with root treatment.
Proportion of Microbial Residual Nitrogen to Total Nitrogen in Soil Aggregates
The fertility level and aggregate level significantly affected the contribution of fungal and bacterial residual nitrogen to total nitrogen (p < 0.05), and the contribution of bacterial residual nitrogen to total nitrogen was also significantly affected by the type of maize residues (p = 0.001) (Figure 6 and Figure 7). The contributions of fungal nitrogen to total nitrogen in all aggregates were shown by macroaggregates > microaggregates. Nevertheless, the contribution of bacterial residual nitrogen to total nitrogen has shown an opposite trend.
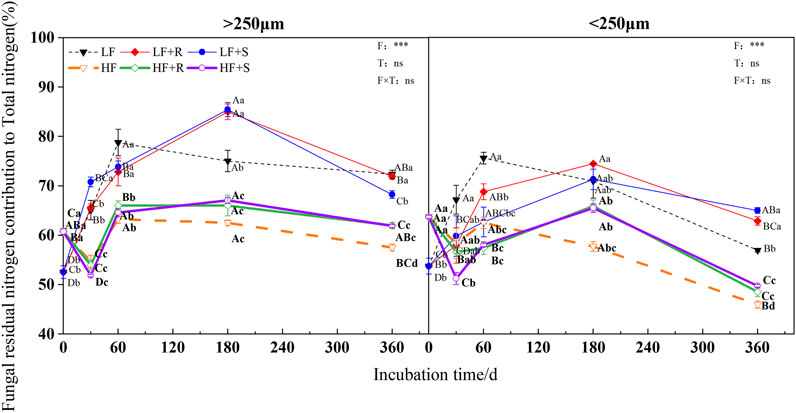
FIGURE 6. Fungal residual nitrogen contribution to total nitrogen in soil aggregates with different fertility levels after the addition of different parts of maize residues. Note: LF means low fertility, LF + R means low fertility + roots, and LF + S means low fertility + Shoots; HF means high fertility, HF + R means high fertility + roots, and HF + S means high fertility + Shoots. Uppercase letters represent differences between different periods in the same treatment, and lowercase letters represent differences between different treatments at the same period. For the ANOVA , F means fertility level, T means the type of maize residues, and F × T means fertility level × type of maize residues. *,**, and *** mean p < 0.05, 0.01, and 0.001, respectively. ns means no significance.
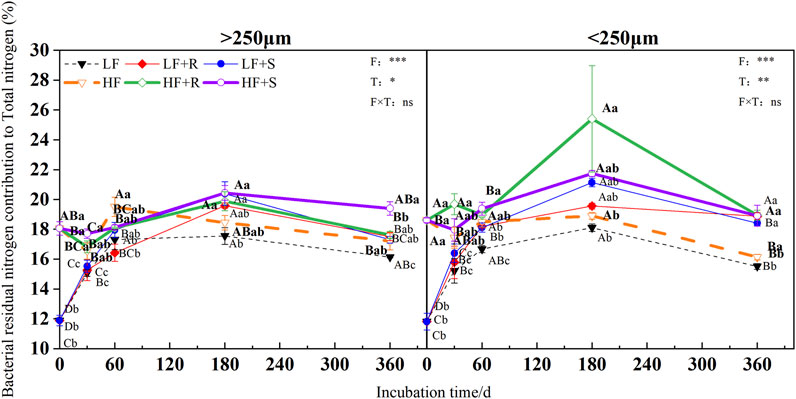
FIGURE 7. Bacterial residual nitrogen contribution to total nitrogen in soil aggregates with different fertility levels after the addition of different parts of maize residues. Note: LF means low fertility, LF + R means low fertility + roots, and LF + S means low fertility + Shoots; HF means high fertility, HF + R means high fertility + roots, and HF + S means high fertility + Shoots. Uppercase letters represent differences between different periods in the same treatment, and lowercase letters represent differences between different treatments at the same period. For the ANOVA, F means fertility level, T means the type of maize residues, and F × T means fertility level × type of maize residues. *,**, and *** mean p < 0.05, 0.01, and 0.001, respectively. ns means no significance.
At the end of incubation, compared with control treatment, the addition of maize residues increased the contribution of fungal and bacterial residual nitrogen to total nitrogen in the aggregates of high and low fertility in different degrees. The contribution of fungal residue nitrogen to total nitrogen showed LF > HF, while the contribution of bacterial residual nitrogen to total nitrogen showed inversely. In the macroaggregates, fungal residual nitrogen contributed 61.92% (HF) and 70.10% (LF) to total nitrogen, respectively, while bacterial residual nitrogen contributed 18.51% (HF) and 17.40% (LF) to total nitrogen, respectively. In the microaggregates, fungal residual nitrogen respectively contributed 49.13% (HF) and 63.92% (LF) to total nitrogen, while bacterial residual nitrogen contributed 18.93% (HF) and 18.65% (LF) to total nitrogen, respectively.
In addition, at the end of incubation, the relative contribution of nitrogen from microbial residues to total nitrogen in different aggregates was different due to the different types of maize residues. For the contribution of nitrogen from fungal residues to total nitrogen in macroaggregates, the contribution of nitrogen from roots was 1.03 times more than that from shoots. The microaggregates treated with shoots were 1.03 times more than those treated with roots. The relative contribution of bacterial residual nitrogen to total nitrogen was just the opposite. In macroaggregates, shoot treatment was 1.04 times more than that with root treatment, while in microaggregates, root treatment was 1.01 times more than that with shoot treatment.
In all treatments, the ratio of fungal residual nitrogen to bacterial residual nitrogen was showed by LF > HF (Figure 8). At the end of incubation, different types of maize residues had different effects on the ratio of fungal residual nitrogen to bacterial residual nitrogen in different aggregates, showing R > S in macroaggregates and an opposite trend in microaggregates.
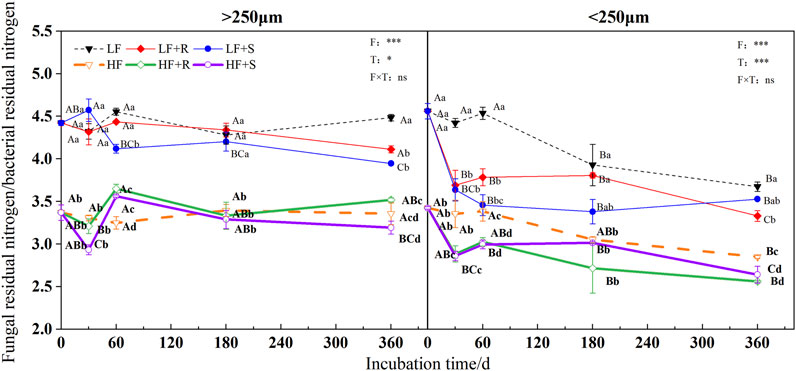
FIGURE 8. Ratio of fungal to bacterial residual nitrogen in soil aggregates with different fertility levels after the addition of different parts of maize residues. Note: LF means low fertility, LF + R means low fertility + roots, and LF + S means low fertility + Shoots; HF means high fertility, HF + R means high fertility + roots, and HF + S means high fertility + Shoots. Uppercase letters represent differences between different periods in the same treatment, and lowercase letters represent differences between different treatments at the same period. For the ANOVA, F means fertility level, T means the type of maize residues, and F × T means fertility level × type of maize residues. *,**, and *** mean p < 0.05, 0.01, and 0.001, respectively. ns means no significance.
Discussion
Effects of Maize Residues’ Parts on Microbial Residual Nitrogen in Soil Aggregates
It had been shown that microbial residues had a longer turnover time in soil than live microorganisms (Liang and Balser, 2008). The contribution of microbial nitrogen to total nitrogen might be higher than that of microbial carbon to soil organic carbon (Simpson et al., 2007; Yang Wang et al., 2020). Therefore, microbial residual nitrogen played an important role in soil nitrogen cycling. At the same time, aggregates could not only regulate the dynamic change of soil nitrogen but also provide a physical barrier to avoid nitrogen loss (Six et al., 2004). Therefore, the process of microbial nitrogen accumulation and distribution in aggregates under the different parts of maize return could be further understood by analyzing the changes of microbial residual nitrogen in aggregates of different parts of maize return.
In general, the addition of different parts of maize residues significantly increased the microbial residual nitrogen, fungal, and bacterial residual nitrogen in different levels of aggregates (Figure 2). At the end of incubation time, the content of fungal residual nitrogen in macroaggregates was higher than that in microaggregates, while the content of bacterial residual nitrogen was the opposite. This might be mainly because the pores in macroaggregates are conducive to the extension of fungal mycelia, while the higher clay content in microaggregates was more conducive to the adsorption and preservation of bacteria (Kandeler et al., 2000), which could be consistent with our hypothesis (1). Among them, the content of microbial residual nitrogen in each aggregate was affected differently by the parts of maize residues. Nevertheless, the content of microbial and fungal residual nitrogen showed a temporary decline in the early incubation time (Figure 2 and Figure 3). The main reason should be the addition of maize provided a large amount of carbon source for microorganisms; most of them were organic nutrients. There were fewer nutrients to be decomposed and utilized easily, such as mineral forms, and the effect of short-term release of nutrients was not evident (Lou et al., 2011; Han and Li, 2018). This caused the microorganisms to be in a state of nitrogen deficiency, which limited the growth and reproduction of microorganisms. At the same time, the C/N of shoots was smaller than that of roots (Table 2), which would provide more nitrogen for microbial growth. Thus, the decrease rate of the nitrogen content of microbial and fungal residues was lower than that of roots. With the gradual decomposition of maize, the nitrogen from maize was gradually released into the soil for microbial utilization. Based on the theory of nitrogen mineralization, when nitrogen is sufficient, soil microbes would propagate rapidly, while the residues could be gradually accumulated in the soil (Jun Di Li et al., 2019). Maize addition could reduce the effectiveness of nitrogen input, accelerating the mineralization of nitrogen in the soil microbial growth (Shahbaz et al., 2018). As an important source of organic matter, microbial residues could supplement the nitrogen reservoir in the soil (Liang and Balser, 2008), so the residual nitrogen content increases. The content of bacterial residual nitrogen in microaggregates did not decrease at the initial stage of incubation time (Figure 4) because the addition of maize could promote the enrichment of nitrogen in microaggregates (Lidong Li et al., 2019). In the initial stage of maize decomposition, the nutrient released rate was faster, and bacteria could use these nutrients faster than fungi (Marschner et al., 2011). Thus, the content of bacterial residual nitrogen in microaggregates at the initial stage of incubation did not show a downward trend. After 180 days, the content of microbial, fungal, and bacterial residual nitrogen decreased again because the maize entered the slow decomposition stage at the later stage of incubation. There were no other nutrients added in the experiment, resulting in the decrease of available nutrients in the soil. At this time, microbial residues, as an energy source and nitrogen source, would be given priority for decomposition and utilization (Abiven et al., 2005).
During the incubation period, shoots promoted residual nitrogen content, showing it was higher than root treatment. It was that the roots contained more lignin and other substances that were not easy to be decomposed. However, the sugars, cellulose, and hemicellulose that existed in the shoots were easily decomposed, which are easier to be used by microorganisms (Clemente et al., 2013). Therefore, nitrogen in the shoots was more involved in the growth and reproduction of microorganisms than that in the roots to form microbial biomass, even accumulated in the form of residues. These would be consistent with hypothesis (2).
Some studies had shown that the input of plant residues would lead to change the soil nutrient availability, even microbial biomass and community (Blaud et al., 2012; Lemanski and Scheu, 2014; Helfrich et al., 2015). It was reasonable to suggest that the addition of maize residues could affect the composition of microbial residues (Kandeler et al., 2000). However, in this study, the residue type only had a significant effect on bacterial residual nitrogen contribution to total nitrogen. Considering the residue type had a significant effect on the content of fungal residual nitrogen, there should be the addition of residue that could promote the accumulation of bacterial residual nitrogen better than fungal residual nitrogen. At the end of the incubation, the results of the ratio of fungal residual nitrogen to total nitrogen in macroaggregates were higher in the root treatment than those in the shoot treatment, while the ratio of bacterial residual nitrogen to total nitrogen was higher in the shoot treatment than that in the root treatment, and the opposite was in the microaggregates. These indicated that the roots were beneficial to the accumulation of fungal residues in the macroaggregates, while the shoots were beneficial to the accumulation of fungal residues in the microaggregates. Similarly, this was also proved by the different ratio of fungal and bacterial nitrogen residues in the aggregates. The results suggested that the input of different parts of maize residues had different effects on the accumulation and distribution of microbial nitrogen in aggregates, which should be consistent with the hypothesis (2). Therefore, studying the distribution of microbial residual nitrogen in aggregates by different types of maize return, understanding the effect of maize types on nitrogen microbial fixation, could provide a basis for modeling the microbial dynamics of nitrogen under the condition of maize return.
Effects of Soil Fertility Levels on Microbial Residual Nitrogen in Soil Aggregates
The soil fertility level also significantly affected the content of microbial, fungal, and bacterial residual nitrogen, showing HF > LF in this study. The low fertility soil due to the lack of nutrients restricted microbial growth, while the high fertility soil contained more available active nutrients and microbial biomass (Paul et al., 2002), and high microbial biomass would promote the generation and accumulation of microbial residues (Ding et al., 2013; Lidong Li et al., 2019). Thus, high fertility soil could maintain more residual nitrogen, which was consistent with the hypothesis (2). These indicated that high fertility soil could accumulate nitrogen more effectively by the microbial metabolic pathway than that by low fertility soil (Wang et al., 2019). In the early stage of incubation time, the content of bacterial residual nitrogen increased briefly and then decreased in the macroaggregates with high fertility soil treated by shoot addition, but this did not exist in the low fertility soil. The main reason should be high fertility soil contained more available nitrogen; in the meantime, shoots contained more easily decomposed substances and nitrogen (Ding et al., 2019). Compared with roots, shoots could replenish active nitrogen in soil during decomposition, resulting in nitrogen supply that could temporarily meet the demands of bacterial growth and metabolism. Thus, bacterial residues should be temporarily accumulated. With the continuous reproduction of bacteria, nitrogen became a key factor restricting their growth. This is the same reason why the content of microbial and fungal residual nitrogen showed a temporary decline in the early incubation time.
In addition, as a key factor regulating the growth of fungi and bacteria, soil pores provided a basis for their enrichment in aggregates with different particle sizes. Larger fungi were more likely to live in macroaggregates, while smaller individual bacteria usually lived in microaggregates (Simpson et al., 2004; Mummey et al., 2006; Strickland and Rousk, 2010). In this study, fungal residual nitrogen was more likely to be enriched in macroaggregates than bacterial residual nitrogen, while bacterial residual nitrogen was more likely to be enriched in microaggregates than fungal residual nitrogen. In addition, in this study, there was no interaction between the types of maize residues and fertility levels on microbial residual nitrogen, which was inconsistent with the hypothesis (3).
In this study, the ratio of fungal residual nitrogen to total nitrogen was higher in low fertility soil than that in high fertility, while the ratio of bacterial residual nitrogen to total nitrogen showed an opposite trend. The soil with the lower organic matter was more conducive to the survival of the fungal community than the soil with higher organic matter (Simpson et al., 2004). Compared with the high fertility brown earth, the low fertility brown earth with lower organic matter had less available active nutrients and a higher proportion of refractory substrates, which was difficult to be utilized by bacteria, leading to the dominance of fungi population (Jun Di Li et al., 2019). In addition, compared with fungi, the growth and metabolism of bacteria were more likely to be affected by the available nutrients in the environment (Helfrich et al., 2015), so the lack of phosphorus and potassium content in the low fertility brown earth would affect the proliferation of bacteria. Moreover, after plant residue addition, the soil with low nitrogen was more favorable to the growth of mycorrhizal fungi (Oates et al., 2016), while the high mycorrhizal fungi would lead to the degradation of bacterial residues (He et al., 2011; Ding et al., 2019). Our results indicated that although high fertility could improve the content of microbial residual nitrogen more than low fertility, the effects on the microbial community structure were different due to the difference in the nutrient content, resulting in different promoting effects on the accumulation of microbial residual nitrogen. Regardless of macroaggregates or microaggregates, the high fertility treatment of the microbial source of nitrogen content was higher than low fertility treatment. But because this was in favor of the existence of the fungi in low soil fertility soil, as well as considering the fungi were difficult to degrade, the maize residues returning to the field might be more meaningful for the nutrient enhancement of low fertility soil.
Conclusion
The effects of different maize parts returning to the field on the accumulation of microbial residual nitrogen in brown earth aggregates with different fertility levels were investigated by calculating the contents of amino sugars. Figure 9 showed the summarized schematic diagram for this study, indicating that the soil with shoot treatments was more likely to accumulate microbial residual nitrogen through microbial metabolic processes. Meanwhile, shoots promoted the accumulation of fungal residual nitrogen in the microaggregates, while roots were more beneficial to the accumulation of fungal residual nitrogen in the macroaggregates. High fertility soil had more microbial residual nitrogen than low fertility, and the soil with low fertility was more conducive to the accumulation of fungal residual nitrogen. Since the fungal residue was difficult to degrade, the maize returning from the soil with low fertility could be more conducive to the increase of soil nitrogen, thereby preventing the loss of nitrogen. Those results were beneficial to the construction of the microbial nitrogen accumulation cycle model on an aggregate scale and would provide a theoretical basis for the rational management of nitrogen nutrients in the dryland of brown earth to prevent soil degradation.
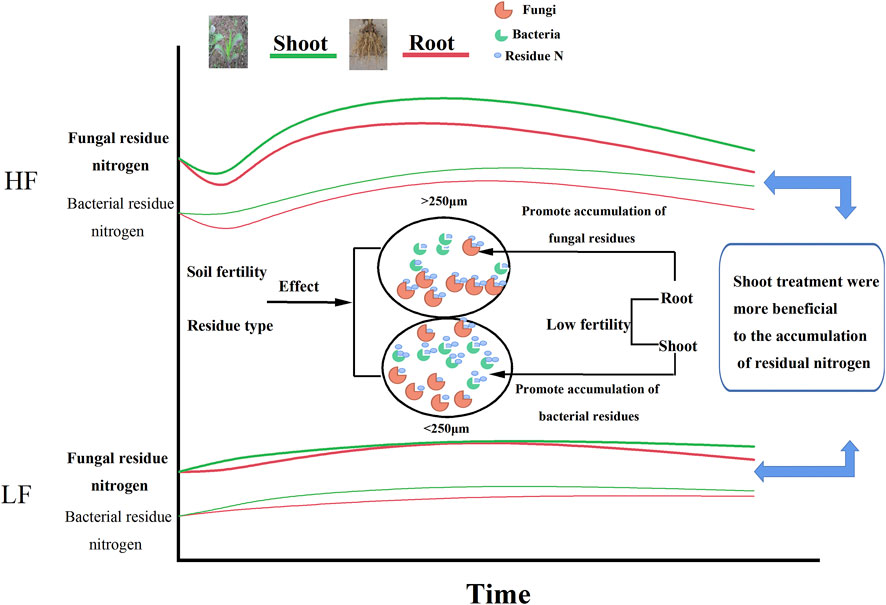
FIGURE 9. Schematic diagram of the influence of the maize residue type and soil fertility on nitrogen allocation of microbial residues in aggregates. Note: the curves represent the changes of fungal and bacterial residual N in different soil aggregates with different soil fertilities over incubation time. The black circles represent aggregates. The shapes within the circles represent microbial communities; meanwhile, the orange and green shapes inside the circles represent fungi and bacteria, respectively. The small blue circle represents microbial residual N.
Data Availability Statement
The original contributions presented in the study are included in the article/Supplementary Material, further inquiries can be directed to the corresponding author.
Author Contributions
PX wrote the manuscript as the first author. JP revised the manuscript as the corresponding author. JW and NM contributed to the experiment.
Funding
The research was supported by the National Key Research and Development Program of China and the National Natural Science Foundation of China. The project number of the National Key Research and Development Program of China is 2021YFD1500204. The project numbers of the National Natural Science Foundation of China are 41977086 and 41807086.
Conflict of Interest
The authors declare that the research was conducted in the absence of any commercial or financial relationships that could be construed as a potential conflict of interest.
Publisher’s Note
All claims expressed in this article are solely those of the authors and do not necessarily represent those of their affiliated organizations, or those of the publisher, the editors, and the reviewers. Any product that may be evaluated in this article, or claim that may be made by its manufacturer, is not guaranteed or endorsed by the publisher.
References
Abiven, S., Recous, S., Reyes, V., and Oliver, R. (2005). Mineralisation of C and N from Root, Stem and Leaf Residues in Soil and Role of Their Biochemical Quality. Biol. Fertil. Soils 42 (2), 119–128. doi:10.1007/s00374-005-0006-0
Amelung, W., Miltner, A., Zhang, X., and Zech, W. (2001). Fate of Microbial Residues during Litter Decomposition as Affected by Minerals. Soil Sci. 166 (9), 598–606. doi:10.1097/00010694-200109000-00003
An, T., Schaeffer, S., Zhuang, J., Radosevich, M., Li, S., Li, H., et al. (2015). Dynamics and Distribution of 13C-Labeled Straw Carbon by Microorganisms as Affected by Soil Fertility Levels in the Black Soil Region of Northeast China. Biol. Fertil. Soils 51 (5), 605–613. doi:10.1007/s00374-015-1006-3
Blaud, A., Lerch, T. Z., Chevallier, T., Nunan, N., Chenu, C., and Brauman, A. (2012). Dynamics of Bacterial Communities in Relation to Soil Aggregate Formation during the Decomposition of 13C-Labelled rice Straw. Appl. Soil Ecol. 53, 1–9. doi:10.1016/j.apsoil.2011.11.005
Bonanomi, G., Cesarano, G., Gaglione, S. A., Ippolito, F., Sarker, T., and Rao, M. A. (2017). Soil Fertility Promotes Decomposition Rate of Nutrient Poor, but Not Nutrient Rich Litter through Nitrogen Transfer. Plant Soil 412 (1/2), 397–411. doi:10.1007/s11104-016-3072-1
Castellano, M. J., Mueller, K. E., Olk, D. C., Sawyer, J. E., and Six, J. (2015). Integrating Plant Litter Quality, Soil Organic Matter Stabilization, and the Carbon Saturation Concept. Glob. Change Biol. 21 (9), 3200–3209. doi:10.1111/gcb.12982
Chantigny, M. H., Angers, D. A., Prévost, D., Vézina, L. P., and Chalifour, F. P. (1997). Soil Aggregation and Fungal and Bacterial Biomass under Annual and Perennial Cropping Systems. Soil Sci. Soc. America J. 61 (61), 262–267. doi:10.2136/sssaj1997.03615995006100010037x
Chao Wang, C., Wang, X., Pei, G. T., Xia, Z. W., Peng, B., Sun, L. F., et al. (2020). Stabilization of Microbial Residues in Soil Organic Matter after Two Years of Decomposition. Soil Biol. Biochem. 141, 107687. doi:10.1016/j.soilbio.2019.107687
Clemente, J. S., Simpson, M. J., Simpson, A. J., Yanni, S. F., and Whalen, J. K. (2013). Comparison of Soil Organic Matter Composition after Incubation with maize Leaves, Roots, and Stems. Geoderma 192 (1), 86–96. doi:10.1016/j.geoderma.2012.08.007
Ding, X., He, H., Zhang, B., and Zhang, X. (2011). Plant-N Incorporation into Microbial Amino Sugars as Affected by Inorganic N Addition: A Microcosm Study of 15N-Labeled maize Residue Decomposition. Soil Biol. Biochem. 43 (9), 1968–1974. doi:10.1016/j.soilbio.2011.06.012
Ding, X., Han, X., Zhang, X., Qiao, Y., and Liang, Y. (2013). Continuous Manuring Combined with Chemical Fertilizer Affects Soil Microbial Residues in a Mollisol. Biol. Fertil. Soils 49, 387–393. doi:10.1007/s00374-012-0736-8
Ding, X., Chen, S., Zhang, B., Liang, C., He, H., and Horwath, W. R. (2019). Warming Increases Microbial Residue Contribution to Soil Organic Carbon in an alpine Meadow. Soil Biol. Biochem. 135, 13–19. doi:10.1016/j.soilbio.2019.04.004
Dorodnikov, M., Blagodatskaya, E., Blagodatsky, S., Marhan, S., Fangmeier, A., and Kuzyakov, Y. (2009). Stimulation of Microbial Extracellular Enzyme Activities by Elevated CO2 depends on Soil Aggregate Size. Global Change Biol. 15 (6), 1603–1614. doi:10.1111/j.1365-2486.2009.01844.x
Engelking, B., Flessa, H., and Joergensen, R. G. (2007). Shifts in Amino Sugar and Ergosterol Contents after Addition of Sucrose and Cellulose to Soil. Soil Biol. Biochem. 39 (8), 2111–2118. doi:10.1016/j.soilbio.2007.03.020
Fontaine, S., Henault, C., Aamor, A., Bdioui, N., Bloor, J. M. G., Maire, V., et al. (2011). Fungi Mediate Long Term Sequestration of Carbon and Nitrogen in Soil through Their Priming Effect. Soil Biol. Biochem. 43 (1), 86–96. doi:10.1016/j.soilbio.2010.09.017
Gunina, A., and Kuzyakov, Y. (2014). Pathways of Litter C by Formation of Aggregates and SOM Density Fractions: Implications from 13C Natural Abundance. Soil Biol. Biochem. 71, 95–104. doi:10.1016/j.soilbio.2014.01.011
Han, X. Z., and Li, N. (2018). Research Progress of Black Soil in Northeast China. Scientia Geographica Sinica 38 (7), 1032–1041. doi:10.13249/j.cnki.sgs.2018.07.004
He, H., Zhang, W., Zhang, X., Xie, H., and Zhuang, J. (2011). Temporal Responses of Soil Microorganisms to Substrate Addition as Indicated by Amino Sugar Differentiation. Soil Biol. Biochem. 43 (6), 1155–1161. doi:10.1016/j.soilbio.2011.02.002
Helfrich, M., Ludwig, B., Thoms, C., Gleixner, G., and Flessa, H. (2015). The Role of Soil Fungi and Bacteria in Plant Litter Decomposition and Macroaggregate Formation Determined Using Phospholipid Fatty Acids. Appl. Soil Ecol. 96, 261–264. doi:10.1016/j.apsoil.2015.08.023
Jawson, M. D., Elliott, L. F., Papendick, R. I., and Campbell, G. S. (1989). The Decomposition of 14C-Labeled Wheat Straw and 15N-Labeled Microbial Material. Soil Biol. Biochem. 21 (3), 417–422. doi:10.1016/0038-0717(89)90153-3
Joergensen, R. G. (2018). Amino Sugars as Specific Indices for Fungal and Bacterial Residues in Soil. Biol. Fertil. Soils 54 (5), 559–568. doi:10.1007/s00374-018-1288-3
Jun Di Li, J. D., Zhang, Y. M., Zhao, B. H., Hu, C. S., He, H. B., Dong, W., et al. (2019). Effect of Long-Term Addition of Organic Substances on Soil Nitrogen and Amino Sugars in Particle-Size Fractions in the North China Plain. Chin. J. Eco-Agriculture 27 (04), 507–518. doi:10.13930/j.cnki.cjea.190084
Kandeler, E., Tscherko, D., Bruce, K. D., Stemmer, M., Hobbs, P. J., Bardgett, R. D., et al. (2000). Structure and Function of the Soil Microbial Community in Microhabitats of a Heavy Metal Polluted Soil. Biol. Fertil. Soils 32, 390–400. doi:10.1007/s003740000268
Lemanski, K., and Scheu, S. (2014). Incorporation of 13C Labelled Glucose into Soil Microorganisms of Grassland: Effects of Fertilizer Addition and Plant Functional Group Composition. Soil Biol. Biochem. 69, 38–45. doi:10.1016/j.soilbio.2013.10.034
Liang, C., Amelung, W., Lehmann, J., and Kästner, M. (2019). Quantitative Assessment of Microbial Necromass Contribution to Soil Organic Matter. Glob. Change Biol. 25 (11), 3578–3590. doi:10.1111/gcb.14781
Liang, C., and Balser, T. C. (2008). Preferential Sequestration of Microbial Carbon in Subsoils of a Glacial-Landscape Toposequence, Dane County, WI, USA. Geoderma 148 (1), 113–119. doi:10.1016/j.geoderma.2008.09.012
Lidong Li, L., Wilson, C. B., He, H., Zhang, X., Zhou, F., and Schaeffer, S. M. (2019). Physical, Biochemical, and Microbial Controls on Amino Sugar Accumulation in Soils under Long-Term Cover Cropping and No-Tillage Farming. Soil Biol. Biochem. 135, 369–378. doi:10.1016/j.soilbio.2019.05.017
Liu, X. B., Zhang, X. Y., Wang, Y. X., Sui, Y. Y., Zhang, S. L., Herbert, S. J., et al. (2010). Soil Degradation: a Problem Threatening the Sustainable Development of Agriculture in Northeast China. Plant Soil Environ. 56 (2), 87–97. doi:10.17221/155/2009-PSE
Lou, Y., Xu, M., Wang, W., Sun, X., and Zhao, K. (2011). Return Rate of Straw Residue Affects Soil Organic C Sequestration by Chemical Fertilization. Soil Tillage Res. 113 (1), 70–73. doi:10.1016/j.still.2011.01.007
Lu, X., Hou, E., Guo, J., Gilliam, F. S., Li, J., Tang, S., et al. (2021). Nitrogen Addition Stimulates Soil Aggregation and Enhances Carbon Storage in Terrestrial Ecosystems of China: A Meta‐analysis. Glob. Change Biol. 27 (12), 2780–2792. doi:10.1111/gcb.15604
Marschner, P., Kandeler, E., and Marschner, B. (2003). Structure and Function of the Soil Microbial Community in a Long-Term Fertilizer experiment. Soil Biol. Biochem. 35 (3), 453–461. doi:10.1016/s0038-0717(02)00297-3
Marschner, P., Umar, S., and Baumann, K. (2011). The Microbial Community Composition Changes Rapidly in the Early Stages of Decomposition of Wheat Residue. Soil Biol. Biochem. 43 (2), 445–451. doi:10.1016/j.soilbio.2010.11.015
Mummey, D., Holben, W., Six, J., and Stahl, P. (2006). Spatial Stratification of Soil Bacterial Populations in Aggregates of Diverse Soils. Microb. Ecol. 51 (3), 404–411. doi:10.1007/s00248-006-9020-5
Oates, L. G., Duncan, D. S., Sanford, G. R., Liang, C., and Jackson, R. D. (2016). Bioenergy Cropping Systems that Incorporate Native Grasses Stimulate Growth of Plant-Associated Soil Microbes in the Absence of Nitrogen Fertilization. Agric. Ecosyst. Environ. 233, 396–403. doi:10.1016/j.agee.2016.09.008
Paul, M., Andreas, F., David, D., Lucie, G., Padruot, F., and Urs, N. (2002). Soil Fertility and Biodiversity in Organic Farming. Science (New York, N.Y.). 296 (5573), 1694–1697. doi:10.1126/science.1071148
Roberts, P., Bol, R., and Jones, D. L. (2007). Free Amino Sugar Reactions in Soil in Relation to Soil Carbon and Nitrogen Cycling. Soil Biol. Biochem. 39 (12), 3081–3092. doi:10.1016/j.soilbio.2007.07.001
Ruamps, L. S., Nunan, N., and Chenu, C. (2011). Microbial Biogeography at the Soil Pore Scale. Soil Biol. Biochem. 43 (2), 280–286. doi:10.1016/j.soilbio.2010.10.010
Schlesinger, W. H., Reynolds, J. F., Cunningham, G. L., Huenneke, L. F., Jarrell, W. M., Virginia, R. A., et al. (1990). Biological Feedbacks in Global Desertification. Science 247 (4946), 1043–1048. doi:10.1126/science.247.4946.1043
Schutter, M. E., and Dick, R. P. (2002). Microbial Community Profiles and Activities Among Aggregates of Winter Fallow and Cover-Cropped Soil. Soil Sci. Soc. America J. 66 (1), 142–153. doi:10.2136/sssaj2002.0142
Shahbaz, M., Kumar, A., Kuzyakov, Y., Börjesson, G., and Blagodatskaya, E. (2018). Priming Effects Induced by Glucose and Decaying Plant Residues on SOM Decomposition: A Three-Source 13C/14C Partitioning Study. Soil Biol. Biochem. 121, 138–146. doi:10.1016/j.soilbio.2018.03.004
Simpson, R. T., Frey, S. D., Six, J., and Thiet, R. K. (2004). Preferential Accumulation of Microbial Carbon in Aggregate Structures of No‐Tillage Soils. Soil Sci. Soc. Am. J. 68 (4), 1249–1255. doi:10.2136/sssaj2004.1249
Simpson, A. J., Simpson, M. J., Smith, E., and Kelleher, B. P. (2007). Microbially Derived Inputs to Soil Organic Matter: Are Current Estimates Too Low? Environ. Sci. Technol. 41 (23), 8070–8076. doi:10.1021/es071217x
Six, J., Bossuyt, H., Degryze, S., Denef, K., and Denef, K. (2004). A History of Research on the Link between (Micro)aggregates, Soil Biota, and Soil Organic Matter Dynamics. Soil Tillage Res. 79 (1), 7–31. doi:10.1016/j.still.2004.03.008
Strickland, M. S., and Rousk, J. (2010). Considering Fungal:bacterial Dominance in Soils - Methods, Controls, and Ecosystem Implications. Soil Biol. Biochem. 42 (9), 1385–1395. doi:10.1016/j.soilbio.2010.05.007
Tisdall, J. M., and Oades, J. M. (1982). Organic Matter and Water-Stable Aggregates in Soils. Eur. J. Soil Sci. 33 (33), 141–163. doi:10.1111/j.1365-2389.1982.tb01755.x
Wang, X., Butterly, C. R., Baldock, J. A., and Tang, C. (2017). Long-term Stabilization of Crop Residues and Soil Organic Carbon Affected by Residue Quality and Initial Soil pH. Sci. Total Environ. 587-588, 502–509. doi:10.1016/j.scitotenv.2017.02.199
Wang, Y., Li, M., Pei, J., An, T., Saeed, M. F., Shan, T., et al. (2019). Dynamics of maize Straw-Derived Nitrogen in Soil Aggregates as Affected by Fertilization. J. Soils Sediments 19 (7), 2882–2890. doi:10.1007/s11368-019-02288-5
Xu, Y., Ding, F., Gao, X., Wang, Y., Li, M., and Wang, J. (2019). Mineralization of Plant Residues and Native Soil Carbon as Affected by Soil Fertility and Residue Type. J. Soils Sediments 19 (3), 1407–1415. doi:10.1007/s11368-018-2152-7
Xu, Y., Ding, X., Lal, R., Gao, X., Li, S., Sun, L., et al. (2020). Effect of Soil Fertility on the Allocation of Nitrogen Derived from Different maize Residue Parts in the Soil-Plant System. Geoderma 379, 114632. doi:10.1016/j.geoderma.2020.114632
Yang Wang, Y., Xu, Y., Pei, J., Li, M., Shan, T., Zhang, W., et al. (2020). Below Ground Residues Were More Conducive to Soil Organic Carbon Accumulation Than above Ground Ones. Appl. Soil Ecol. 148, 103509. doi:10.1016/j.apsoil.2020.103509
Keywords: maize residues, fungal residual nitrogen, bacterial residual nitrogen, soil aggregates, soil fertility
Citation: Xue P, Pei J, Ma N and Wang J (2022) Microbial Residual Nitrogen Distribution in Brown Earth’s Aggregates as Affected by Different Maize Residues and Soil Fertility Levels. Front. Environ. Sci. 10:892039. doi: 10.3389/fenvs.2022.892039
Received: 08 March 2022; Accepted: 21 March 2022;
Published: 14 April 2022.
Edited by:
Shengbo Xie, Northwest Institute of Eco-Environment and Resources (CAS), ChinaReviewed by:
Bin Zhang, Nanjing University of Information Science and Technology, ChinaHaixia Tian, Northwest A&F University, China
Copyright © 2022 Xue, Pei, Ma and Wang. This is an open-access article distributed under the terms of the Creative Commons Attribution License (CC BY). The use, distribution or reproduction in other forums is permitted, provided the original author(s) and the copyright owner(s) are credited and that the original publication in this journal is cited, in accordance with accepted academic practice. No use, distribution or reproduction is permitted which does not comply with these terms.
*Correspondence: Jiubo Pei, cGVpaml1Ym9Ac3lhdS5lZHUuY24=