- 1Department of Plant and Wildlife Sciences, Brigham Young University, Provo, UT, United States
- 2Innovative Breakthrough Energy Technology Ltd., Permafrost Carbon Feedback Action Group, Cascade Institute, Colwood, BC, Canada
- 3Division of Math and Science, Babson College, Wellesley, MA, United States
- 4Department of Natural Resources and the Environment, University of New Hampshire, Durham, NH, United States
- 5Sandia National Laboratories, Albuquerque, NM, United States
- 6School of Freshwater Sciences, University of Wisconsin-Milwaukee, Milwaukee, WI, United States
- 7Department of Physical Geography, Bolin Centre for Climate Research, Stockholm University, Stockholm, Sweden
- 8Department of Geography, Colgate University, Hamilton, NY, United States
- 9Institute of Ocean Science (IOS), Sidney, BC, Canada
- 10Department of Geography and Environmental Sciences, Northumbria University, Newcastle-Upon-Tyne, United Kingdom
- 11Woodwell Climate Research Center, Falmouth, MA, United States
- 12Department of Renewable Resources, University of Alberta, Edmonton, AB, Canada
- 13International Cryosphere Climate Initiative, Pawlet, VT, United States
- 14Rubenstein School of Environment and Natural Resources, University of Vermont, Burlington, VT, United States
- 15Wildlife Conservation Society, Arctic Beringia Program, Fairbanks, AK, United States
- 16Environmental Science Division and Climate Change Science Institute, Oak Ridge National Laboratory, Oak Ridge, TN, United States
- 17Northern Arizona University, Flagstaff, AZ, United States
- 18Department of Biological Sciences, University of Alberta, Edmonton, AB, Canada
- 19Department of Biological Sciences, The University of Alabama, Tuscaloosa, AL, United States
- 20Permafrost Research Section, Alfred Wegener Institute Helmholtz Centre for Polar and Marine Research, Potsdam, Germany
- 21Department of Geological Sciences and Bolin Centre for Climate Research, Stockholm University, Stockholm, Sweden
- 22University of Colorado Boulder, Institute of Arctic and Alpine Research (INSTAAR), Boulder, CO, United States
- 23Department of Environmental and Biological Sciences, University of Eastern Finland, Kuopio, Finland
- 24Permafrost Carbon Feedback Action Group, Cascade Institute, Colwood, BC, Canada
- 25State Key Laboratory of Vegetation and Environmental Change, Institute of Botany, Chinese Academy of Sciences, Beijing, China University of Chinese Academy of Sciences, Beijing, China
- 26Department of Earth and Environmental Sciences, Michigan State University, East Lansing, MI, United States
- 27Harvard University, Cambridge, MA, United States
Climate change is an existential threat to the vast global permafrost domain. The diverse human cultures, ecological communities, and biogeochemical cycles of this tenth of the planet depend on the persistence of frozen conditions. The complexity, immensity, and remoteness of permafrost ecosystems make it difficult to grasp how quickly things are changing and what can be done about it. Here, we summarize terrestrial and marine changes in the permafrost domain with an eye toward global policy. While many questions remain, we know that continued fossil fuel burning is incompatible with the continued existence of the permafrost domain as we know it. If we fail to protect permafrost ecosystems, the consequences for human rights, biosphere integrity, and global climate will be severe. The policy implications are clear: the faster we reduce human emissions and draw down atmospheric CO2, the more of the permafrost domain we can save. Emissions reduction targets must be strengthened and accompanied by support for local peoples to protect intact ecological communities and natural carbon sinks within the permafrost domain. Some proposed geoengineering interventions such as solar shading, surface albedo modification, and vegetation manipulations are unproven and may exacerbate environmental injustice without providing lasting protection. Conversely, astounding advances in renewable energy have reopened viable pathways to halve human greenhouse gas emissions by 2030 and effectively stop them well before 2050. We call on leaders, corporations, researchers, and citizens everywhere to acknowledge the global importance of the permafrost domain and work towards climate restoration and empowerment of Indigenous and immigrant communities in these regions.
Introduction
Though permafrost-affected regions cover only 10% of Earth’s surface, they constitute more than half of all remaining terrestrial and marine wilderness (Watson et al., 2018), making them crucial to maintaining biosphere integrity in our rapidly changing world. These regions, which we refer to as the permafrost domain (Figure 1), contain between 2.5 and 3 trillion tons of organic carbon—more than all of Earth’s other life, soil, and atmosphere combined (Hugelius et al., 2014; Abbott et al., 2016b; Sayedi et al., 2020; Mishra et al., 2021; Abbott B. W., 2022; Schuur et al., 2022). The permafrost domain is home to tens of millions of people, including diverse Indigenous and immigrant cultures that both depend on and sustain these globally-significant ecosystems (Riedlinger and Berkes, 2001; Parkinson and Berner, 2009; Pearce et al., 2009; Chapin et al., 2013; Díaz et al., 2019; Proverbs et al., 2020; Ellis et al., 2021; Mettiäinen et al., 2022). The permafrost domain’s three-fold importance—biodiversity, climate, and human peoples—means that governments, corporations, and communities within and outside of these regions must commit to preventing dangerous environmental change (Chapin and Díaz, 2020; Whyte, 2020; Chapin, 2021; Natali et al., 2021; Arctic Council, 2022).
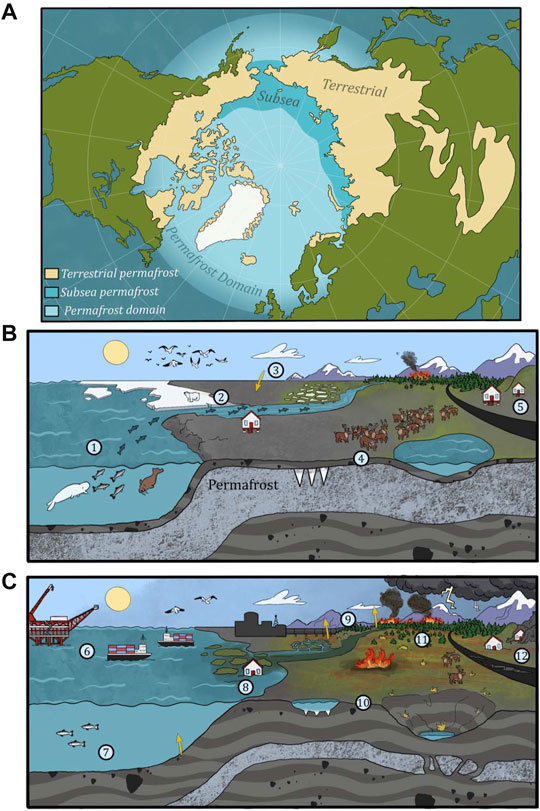
FIGURE 1. The extent and example futures of the permafrost domain (A) Our definition of the domain includes marine and terrestrial regions substantially affected by perennial frozen material, including oceans and seas above 50°N, subsea permafrost on the continental shelves, Arctic tundra, Boreal forest, and Alpine permafrost. The figure shows potential permafrost futures for (B) a rapid decarbonization scenario and (C) a continued fossil energy scenario. Panel B depicts: 1. Resilient biological communities in marine, coastal, and terrestrial environments, 2. Persistence of sea ice, especially crucial multiyear and landfast ice, 3. Maintenance of net greenhouse gas uptake in the permafrost domain, 4. Recovery of permafrost and a shallow active layer, and 5. Preservation of human cultural activities and infrastructure. Panel C depicts: 6. Expansion of fossil fuel extraction and marine navigation, 7. Disruption of food webs, migrations, and biological communities on sea and land, 8. Accelerated coastal erosion, aquatic and marine primary productivity, ocean acidification, and erosion of terrestrial material, including pollutants, 9. Transition to net release of greenhouse gases (CH4, N2O, and CO2), 10. Extensive permafrost degradation, including active-layer thickening and lake formation and draining, 11. Intensifying terrestrial disturbances including wildfire, hydrological extremes, thermokarst, vegetation shifts, and invasive species, 12. Profound disruption of human culture and infrastructure.
The permafrost domain is uniquely vulnerable to climate change because of accelerated warming and the prevalence of ice. Air temperature in the permafrost domain over land and sea has risen two to four times faster than the global mean, largely because of ice and snow loss, changes in ocean and atmospheric circulation, and the effects of ozone-depleting gases (Huang et al., 2017; Goosse et al., 2018; Mu et al., 2020a; Polvani et al., 2020; AMAP, 2021). Ice in all its forms underpins and overlays the permafrost domain, and its loss disrupts energy balance, ecosystem structure, and human activity (Bamber et al., 2018; Schuur and Mack, 2018; Bamber et al., 2019; Turetsky et al., 2020; Schmidt et al., 2021; Irrgang et al., 2022). Consequently, climate change is intensifying disturbance regimes across the permafrost domain and restructuring socioecological dynamics at continental scales (Hjort et al., 2018; Chou et al., 2021; Veraverbeke et al., 2021; Treharne et al., 2022). In many regions, these changes are progressing decades faster than expected (Farquharson et al., 2019; Angelopoulos et al., 2021; Parkinson and DiGirolamo, 2021), likely heralding the transition of the permafrost domain into unprecedented biophysical and socioecological states (Box et al., 2019; IPCC, 2019; Meredith et al., 2019; Chen et al., 2021).
Pervasive and interconnected changes in the permafrost domain are triggering complex local biogeochemical responses with global repercussions. The ongoing release of large amounts of greenhouse gas (GHG) from permafrost soils, sediments, and waterways has motivated substantial research and attracted public attention (Schuur et al., 2015; Andreassen et al., 2017; Kessler, 2017; Froitzheim et al., 2021; Treharne et al., 2022). The production and release of CO2, CH4, and N2O occur during the decomposition or combustion of organic matter in terrestrial and aquatic environments (Schädel et al., 2016; Plaza et al., 2019; Voigt et al., 2020; Abbott B. W., 2022). Additionally, CH4 can escape from subsea or subterranean fossil reserves or hydrate deposits, particularly during disturbance from permafrost thaw or fossil-fuel exploration and extraction (Walter et al., 2008; Thornton et al., 2016; Behari et al., 2020; Sayedi et al., 2020; Froitzheim et al., 2021).
The release of GHGs and loss of surface ice and snow from the permafrost domain constitute some of the largest destabilizing climate feedbacks globally (Schaefer et al., 2012; Schuur et al., 2015; Hugelius, 2022). These feedbacks are very likely to be nonlinear, with the amount of GHG production and albedo change often exponentially related to temperature (Abbott et al., 2016b; Carey et al., 2016; Turetsky et al., 2020; AMAP, 2021). The potential magnitude and timing of such amplifying permafrost climate feedbacks remain highly uncertain but could amount to several hundred gigatons (Gt) of CO2 equivalent over the next two centuries (Schuur et al., 2015, 2022; McGuire et al., 2018; Meredith et al., 2019; Sayedi et al., 2020; Canadell et al., 2021). GHG emissions from the permafrost domain are already similar to the annual emissions of Japan (Hugelius, 2022). Until recently, permafrost domain GHG release was omitted from the Earth system models (ESMs) used to predict climate change trajectories and inform international climate targets (Canadell et al., 2021; Natali et al., 2021). New ESMs are beginning to integrate permafrost carbon feedbacks, but estimates remain preliminary and lack a number of important processes (Abbott et al., 2016b; McGuire et al., 2018; Chen et al., 2020; de Vrese and Brovkin, 2021; Natali et al., 2021).
The combination of growing scientific attention and persistent socio-ecological complexity has created polarized and incomplete perceptions about permafrost in public and policymaker circles (Table 1). In this paper, we review permafrost climate responses with a focus on mitigation. The sections below highlight how climate change and other human disturbances are affecting the physical, biological, and social fabric of the permafrost domain. These examples are not comprehensive, and we remind all readers that these regions are composed of diverse ecosystems and peoples with unique histories and ecological contexts (Jorgenson et al., 2013; Chapin, 2020; Tank et al., 2020; Turetsky et al., 2020; Arctic Council, 2022).
Unstable Footing: Terrestrial Permafrost Degradation
Permanently frozen ground or permafrost has developed in cold regions primarily in the Northern Hemisphere (Figure 1). The formation and degradation of permafrost depends on complex interactions between local climate and ecosystem characteristics, especially vegetation, water, and glacial history (Shur and Jorgenson, 2007; Lindgren et al., 2018; Loranty et al., 2018). Because of unique soil processes, the permafrost domain has accumulated much of the Earth’s freshwater, carbon, nitrogen, phosphorus, and pollutants such as mercury transported from lower latitudes by the atmosphere and rivers (Fisher et al., 2012; Hugelius et al., 2014; Strauss et al., 2017; Malone et al., 2018; Schuster et al., 2018; Abbott et al., 2019; Voigt et al., 2020). For example, cold and waterlogged soils slow microbial decomposition, and periglacial processes can incorporate materials that are produced or deposited on the surface much deeper in the soil than in other regions (Lindgren et al., 2018; FAO, 2021; Finger and Rekvig, 2022).
Soils in the permafrost domain are warming worldwide (Biskaborn et al., 2019; Neumann et al., 2019; Miner et al., 2021; Smith et al., 2022) because of higher air temperature, vegetation shifts, loss of snow and ice cover, surface disturbances, and intensification of the hydrological cycle (Rawlins et al., 2010; Stevens and Latimer, 2015; Forbes et al., 2016; Egelkraut et al., 2018; Loranty et al., 2018; Kropp et al., 2020). Deeper and longer thaw stimulates microbial decomposition—the main driver of GHG release from the permafrost domain (Treat et al., 2015; Schädel et al., 2016; Natali et al., 2019; Voigt et al., 2020; Treharne et al., 2022). Nutrients, trace elements, and pollutants are also released during soil warming, affecting plant growth, microbial activity, and human health (Keuper et al., 2012; Chen et al., 2018; Hewitt et al., 2018; Carey et al., 2019; UNEP, 2019; Mu et al., 2020b; Yang et al., 2021; Basu et al., 2022). While increased nutrient availability and CO2 fertilization have long been predicted to enhance plant uptake of atmospheric CO2, observed trends of primary productivity in the permafrost domain have been mixed because of vegetation shifts, droughts, and other disturbances (Forbes et al., 2010; Hayes et al., 2011; Abbott et al., 2016b; McGuire et al., 2018; Rocha et al., 2018; Myers-Smith et al., 2020; Bruhwiler et al., 2021; Mack et al., 2021; Zhao et al., 2021; Vitali et al., 2022).
Terrestrial disturbances in the permafrost domain are intensifying, including wildfire, surface subsidence (thermokarst), and direct human disturbance from resource extraction, grazing, and infrastructure. Lengthening dry periods, vegetation shifts, and more lightning are increasing Boreal and Arctic wildfire, with effects on local habitat, air quality, and regional carbon and nutrient cycles (Masrur et al., 2018; Box et al., 2019; Rodríguez-Cardona et al., 2020; Abbott et al., 2021b; Chen et al., 2021; Mack et al., 2021). In addition to directly producing CO2 and CH4 during combustion, wildfires warm soil through loss of insulating vegetation and albedo changes. This can stimulate organic matter decomposition and hydrological export for decades after the wildfire (Grosse et al., 2011; Rocha and Shaver, 2011; Carey et al., 2019; Meredith et al., 2019; Abbott et al., 2021b; Bruhwiler et al., 2021). Permafrost wildfires are expanding northward and even burning through the winter (Holloway et al., 2020; McCarty et al., 2020; Scholten et al., 2021; Veraverbeke et al., 2021; Talucci et al., 2022).
In areas with ground ice, soil warming often triggers abrupt surface collapse, mass wasting, and coastal erosion (Kokelj and Jorgenson, 2013; Olefeldt et al., 2016; Grotheer et al., 2020; Angelopoulos et al., 2021). These thermokarst processes have a wide range of consequences depending on landscape position and soil characteristics (Mu et al., 2020a; Turetsky et al., 2020; Yang et al., 2021), including soil warming, GHG release or uptake, and delivery of sediment and solutes to aquatic ecosystems (Anthony et al., 2014; Abbott et al., 2015; Farquharson et al., 2019; Kokelj et al., 2021; Wologo et al., 2021; Yang et al., 2021). Subsidence can result in complex changes in soil moisture, affecting the type and amount of GHGs produced, further complicating estimates of permafrost climate feedbacks (Lupascu et al., 2013; Lawrence et al., 2015; Boike et al., 2016; Schuur et al., 2022). Approximately 20% of the northern permafrost region is vulnerable to thermokarst formation, which can trigger rapid GHG release and damage human infrastructure and wildlife habitat (Olefeldt et al., 2016; Hjort et al., 2018; Turetsky et al., 2020; Gao et al., 2021).
While the permafrost domain generally has low human population density, direct human impacts are extensive and growing. Fossil fuel extraction, logging, peat harvesting, and construction of roads, power lines, and buildings can cause soil warming or collapse by disturbing vegetation and modifying surface conditions, including moisture and albedo (Van Seters and Price, 2001; Kreutzweiser et al., 2008; Lamers et al., 2015; Bartsch et al., 2021; FAO, 2021; Maslakov et al., 2021). Some human activities, such as grazing, can cool or warm soils depending on the intensity of the disturbance and local ecosystem attributes including soil structure and vegetation community (Egelkraut et al., 2018; Beer et al., 2020; FAO, 2021). Many of these large-scale human activities are driven by demand for energy, fiber, and tourism from outside the permafrost domain, adding another layer to the environmental injustice of climate change (Johnson, 2010; Chapin, 2021).
Troubled Waters: Hydrochemical Disruption From Streams to Seas
Though terrestrial, freshwater, and marine environments are often considered separately, they are closely coupled climatically, biogeochemically, and culturally (Bhatt et al., 2010; Kling, 2010; Chapin et al., 2013; Forbes et al., 2016; MacDonald et al., 2021). This is especially true in the permafrost domain, where the ground is often held up by frozen water, extensive freshwater networks blur boundaries, and sea ice regulates exchange of energy and material among land, sea, and atmosphere (Campeau et al., 2019; Harms et al., 2019; IPCC, 2019; Shogren et al., 2021). Indeed, much of the marine habitat in the Arctic Ocean still carries thermal and biogeochemical legacies from when it was terrestrial during the Last Glacial Maximum (Figure 1A) (Frederick and Buffett, 2016; Overduin et al., 2019; Angelopoulos et al., 2020; Sayedi et al., 2020). Consequently, terrestrial and marine disturbances are strongly interlinked. For example, the release of sediment, organic matter, and pollutants from degrading permafrost can alter aquatic food webs, influence migrations of marine animals, and expose human communities to biomagnified pollutants (AMAP, 2017; UNEP, 2019). Likewise, loss of sea ice increases atmospheric moisture and energy availability, driving shifts in terrestrial vegetation and likelihood of extreme precipitation events (Bhatt et al., 2010; Forbes et al., 2016).
The Arctic Ocean and surrounding seas (referred to hereafter simply as the Arctic Ocean) play pivotal roles in global climate, ocean circulation, marine and terrestrial biodiversity, and international politics and commerce (Steinacher et al., 2009; Carmack et al., 2016; Chou et al., 2021; Arctic Council, 2022). Like in terrestrial permafrost regions, many changes in the Arctic Ocean are occurring decades faster than predicted (Steinacher et al., 2009; Boers and Rypdal, 2021; Parkinson and DiGirolamo, 2021). Sea ice extent and thickness have plummeted, with summer ice expected to disappear mid-century (IPCC, 2019). Freshwater inputs have increased because of glacial and icesheet melt and climbing river discharge (Bamber et al., 2018; King et al., 2020; Feng et al., 2021). At the same time, terrestrial permafrost degradation and pollution from outside the permafrost domain are substantially altering the delivery of carbon, nutrients, sediment, and pollutants via coastal collapse, river discharge, groundwater flux, and atmospheric transport (Fisher et al., 2012; Tank et al., 2016; Toohey et al., 2016; Fritz et al., 2017; Drake et al., 2018; Connolly et al., 2020; Wologo et al., 2021; Mann et al., 2022).
Coastal erosion and riverine material fluxes are changing particularly rapidly (Fritz et al., 2017; Tank et al., 2020). Coastal collapse has been supercharged by three factors: permafrost degradation, increased wave action from sea ice loss, and intrusions of saltwater (Jones et al., 2009; Lantuit et al., 2012; Berry et al., 2021; Guimond et al., 2021). Coastal erosion rates now exceed 20 m yr−1 in some areas, though differences in local conditions create high spatial variability (Lantuit et al., 2012; Günther et al., 2015; Fritz et al., 2017). Changes in riverine transport are being caused by hydrological intensification, thickening of the seasonally-thawed active layer, widespread thermokarst formation, and changes in plant uptake of water and nutrients (Toohey et al., 2016; Treat et al., 2016; Carey et al., 2019, 2020; Shogren et al., 2020; Tank et al., 2020). Interacting disturbances, such as wildfire, thermokarst formation, and extreme hydrological events can deliver large pulses of material to river networks and Arctic estuaries (Holmes et al., 2012; St. Pierre et al., 2018; Rodríguez-Cardona et al., 2020; Abbott et al., 2021b). For example, in the western Canadian Arctic, mass wasting along fluvial networks has increased two orders of magnitude from 1986–2018, creating sedimentary deposits that will cascade through rivers and lakes to the Arctic Ocean for decades to millennia (Kokelj et al., 2021). Understanding and preventing changes in water chemistry and river discharge are particularly important for the Tibetan Plateau, which provides drinking and agricultural water for 1.4 billion people (Yao et al., 2018; Mu et al., 2020b; Gao et al., 2021).
Riverine and coastal erosion is also releasing trace metals and semi-volatile contaminants from permafrost and active-layer soils (Loiko et al., 2017; Schuster et al., 2018; St. Pierre et al., 2018; Perryman et al., 2020; Basu et al., 2022). These toxic materials include mercury, organochlorines, PAHs, and other compounds that accumulate naturally or from human pollution such as coal combustion and mining (Fisher et al., 2012; AMAP, 2021). The permafrost domain’s aquatic environments are especially vulnerable to these global contaminants largely due to efficient transport pathways and bio-accumulating and biomagnifying processes (Fahnestock et al., 2019). Mercury is of special concern because one of its neurotoxic forms, methylmercury, accumulates in food webs and is found at elevated levels in wildlife and human populations across the circumpolar north (AMAP, 2011; Basu et al., 2022). Because of its strong influence on redox state and bioavailability of metals, the release of terrestrial organic matter may also foster the production and transport of methylmercury in aquatic environments (Stern et al., 2012; Abbott et al., 2016a; Fahnestock et al., 2019).
In addition to altered material flux from terrestrial environments, climate change is affecting the Arctic Ocean directly. The characteristics of the Arctic Ocean make it highly vulnerable to ocean acidification, “the other CO2 problem” (Bates and Mathis, 2009; Doney et al., 2009; AMAP, 2017). Acidic conditions decrease the availability of calcium carbonate, disrupting marine primary and secondary production (Yamamoto-Kawai et al., 2009; Denman et al., 2011). Arctic Ocean biogeochemistry is dominated by land-derived runoff, receiving ∼ 11% of global river runoff while constituting just 1% of ocean volume (McClelland et al., 2012). Consequently, the Arctic Ocean’s cold and poorly-buffered surface waters absorb large quantities of CO2 (Bates and Mathis, 2009; Bruhwiler et al., 2021). The decomposition of terrestrial organic matter adds more CO2, further decreasing pH (Alling et al., 2012; Semiletov et al., 2016; Tanski et al., 2021). Strong vertical stratification and sea ice prevent mixing within the water column and exchange with the atmosphere, resulting in rapid acidification in some of the most vulnerable compartments of the Arctic Ocean (Bates and Mathis, 2009; Yamamoto-Kawai et al., 2009; Vonk et al., 2012; Ouyang et al., 2020). The combined effects of rising CO2, increased meltwater inputs, and changes in circulation could push calcium carbonate below critical saturation thresholds in the Arctic Ocean’s surface water by the mid-21st Century (Steinacher et al., 2009; Denman et al., 2011). In combination with increasing thermal stresses, this could trigger major breakdowns in planktonic and benthic food webs (Yamamoto-Kawai et al., 2009; Denman et al., 2011; Arrigo et al., 2020). For example, the combination of increased light availability from sea ice loss and changes in water and nutrient delivery from terrestrial ecosystems is estimated to have increased primary productivity in the Arctic Ocean by roughly 60% over the last 20 years (Lewis et al., 2020; Terhaar et al., 2021). This state change could cause the loss of ecological niches and biodiversity in Arctic food webs.
Predicting and Shaping Permafrost Futures
Given the complexity of the permafrost domain and the unprecedented speed of climate change, we do not know the specific timeline and severity of disruption to its peoples, biodiversity, and biogeochemistry (Proverbs et al., 2020; Bruhwiler et al., 2021; Canadell et al., 2021; Fewster et al., 2022; Mann et al., 2022; Versen et al., 2022). For example, the most comprehensive permafrost model intercomparison project (MIP) of carbon balance estimated a range of ∼600 Gt of carbon release to ∼200 Gt of carbon uptake by the year 2300 (McGuire et al., 2018). Though the divergence of model outputs is clearly problematic, the multi-model mean of this study has become the default reference for comparison (Turetsky et al., 2020; de Vrese and Brovkin, 2021; Natali et al., 2021). These models were state-of-the art seven to 10 years ago, but there have been considerable advances in permafrost domain modeling since then (Randers and Goluke, 2020; Shu et al., 2020; Smith et al., 2021; Wiltshire et al., 2021; Chadburn et al., 2022). This emphasizes the larger problem of the continued exclusion of permafrost in Earth system models. Only 4 of 11 CMIP6 models used for IPCC AR6 included permafrost (IPCC, 2019, 2021), and only two of the 18 models in the Zero Emissions Commitment model intercomparison included permafrost dynamics (MacDougall, 2021).
Because the stakes of environmental change in the permafrost domain are so high for human and nonhuman permafrost communities, we need to use all available tools for monitoring and prediction. For example, recognizing these shortcomings of Earth system models, the IPCC AR6 WG1 estimated permafrost climate feedbacks using a range of different methods, including empirically based studies (Canadell et al., 2021). In research, management, and policymaking, we need to fully integrate traditional ecological knowledge, empirical and model-based evidence, expert assessments, and paleo studies considering the full range of socioecological consequences (Kimmerer, 2002; Sayedi et al., 2020; Chapin, 2021; Arctic Council, 2022). Specifically, we need to consider disruptions and sources of resilience that cascade across ecosystem boundaries (e.g., terrestrial, freshwater, marine) and ecological dimensions (e.g., human wellbeing, biosphere integrity, and biogeochemical cycles). Identified research and management priorities go far beyond questions of carbon balance, including: Arctic Ocean circulation, marine and coastal habitat, destabilization of organic matter and methane clathrates on the continental shelves, thermokarst formation, fire-induced thaw, nutrient interactions, peatland dynamics, and socioecological adaptation and resilience (Lewis et al., 2020; Voigt et al., 2020; FAO, 2021; Finger and Rekvig, 2022; Mettiäinen et al., 2022).
What can we do?
Persistent uncertainty about permafrost processes does not limit our ability to act now to protect the permafrost domain. Across traditional, modeled, and empirical approaches, there is consensus that the timing and degree of damage to the permafrost domain are directly associated with the amount of human-caused warming (Canadell et al., 2021; Abbott B. W., 2022; Cheng et al., 2022; Fewster et al., 2022). The question then becomes, how can we most effectively reduce anthropogenic climate change?
In very general terms, there are three non-exclusive approaches to stopping climate change: 1) Reduce human GHG emissions, 2) Protect ecosystems to sustain natural GHG sinks, and 3) Attempt to control the Earth’s energy balance through geoengineering. The first two approaches are feasible, cost-effective, and come with a wide array of co-benefits (Foley et al., 2011; Breyer et al., 2021; Chapin et al., 2022). For example, eliminating fossil fuel burning could prevent 10.2 million premature deaths each year and add US$10 trillion annually in economic benefits from improved air quality (Errigo et al., 2020; Shindell et al., 2021; Vohra et al., 2021; Abbott B., 2022). Likewise, collaboratively expanding conservation of intact ecosystems enhances biosphere integrity and can restore rights of Indigenous and immigrant peoples of the permafrost domain (Steffen et al., 2018; Watson et al., 2018; Díaz et al., 2019; Bergstrom et al., 2021; Chapin et al., 2022). Unfortunately, the third approach (geoengineering) is both less proven and more prone to unintended consequences (Lawrence et al., 2018; Zarnetske et al., 2021; Mettiäinen et al., 2022; Versen et al., 2022).
A wide suite of geoengineering interventions have been proposed in the permafrost domain, including solar radiation management (SRM), ocean brightening, artificial sea-ice creation, ocean fertilization, biomass energy with carbon capture and storage (BECCS), and biomanipulations such as tree planting and herbivore introductions (Olson, 2012; Harper et al., 2018; Whyte, 2018; Zampieri and Goessling, 2019; Beer et al., 2020; Chen et al., 2020; Zarnetske et al., 2021; Mettiäinen et al., 2022; Versen et al., 2022). While continued research into some of these interventions is merited, all have serious side effects and known ethical and practical limitations (Tuana et al., 2012; Lawrence et al., 2018; Mettiäinen et al., 2022). For example, SRM could theoretically reduce temperatures enough to protect a portion of the permafrost domain (Chen et al., 2020). However, this would not solve acidification of the Arctic Ocean and would very likely disrupt global agriculture while exacerbating the Arctic ozone hole (Tilmes et al., 2008; Proctor et al., 2018; Zarnetske et al., 2021). Likewise, converting portions of the Boreal forest for BECCS could decrease local ecosystem carbon storage while producing pollution that would harm public health and create substantial regional warming from black and brown carbon deposition (Hanssen et al., 2020; Calì Quaglia et al., 2022; Yue et al., 2022). Additionally, many of these proposed solutions may be ineffective or counterproductive in the new conditions created by anthropogenic climate change. For example, the survival of large herds of herbivores could be negatively affected by shifts in forage and extreme weather events (Forbes et al., 2016; Zarnetske et al., 2021), and carbon uptake from tree planting can be erased by temperature-induced mass mortality and an intensifying wildfire regime (Hammond et al., 2022; Talucci et al., 2022). Even if these interventions achieved their climate goals, they would threaten more than half of remaining intact ecosystems globally (Watson et al., 2018; Díaz et al., 2019).
We conclude that rapid reduction of fossil fuel emissions and empowerment of local communities are needed to conserve permafrost ecosystems. While many scientific questions remain about permafrost-climate complexities, we know that the faster the drawdown of atmospheric GHGs, the more of the permafrost domain will be preserved (Canadell et al., 2021; Abbott B. W., 2022). Because of the permafrost domain’s immensity and momentum (Schuur et al., 2015; Lindgren et al., 2018; Biskaborn et al., 2019; Sayedi et al., 2020; de Vrese and Brovkin, 2021), the choices we make over the next decade regarding GHG emissions could either open pathways towards recovery and conservation or lock us into a future of loss and degradation (Figure 1) (King et al., 2020; Ritchie et al., 2021; Abbott B., 2022). Consequently, the future of the permafrost domain depends on energy choices made far beyond its borders.
Thankfully, recent breakthroughs in renewable energy production, transmission, and storage now allow much faster decarbonization than previously believed possible (Bogdanov et al., 2021; Victoria et al., 2021; Jacobson et al., 2022). The costs of solar photovoltaics and wind turbine have plummeted 91 and 71%, respectively since 2009, now providing the cheapest and cleanest electricity ever available to humankind (Abbott et al., 2021a; Breyer et al., 2021; Abbott B., 2022). Global markets have already responded, with renewables constituting 90% of all new electricity capacity built in 2021 (IEA, 2021a) and 95% of all projected growth through 2025 (IEA, 2021b). With doubling periods of 3.7 years for wind and 1.9 years for solar, renewables could meet global electricity demand within 10 years and all primary energy demand within 25 (Rockström et al., 2017; Haegel et al., 2019; Abbott et al., 2021a; Bogdanov et al., 2021; Abbott B., 2022). Mature technologies now allow electrification of nearly the entire economy (transportation, manufacturing, agriculture, etc.), with developing technologies on track to allow full decarbonization before 2040 (Breyer et al., 2021; Jacobson et al., 2022). If the global community commits to sustaining this ongoing transition, the clean and abundant energy will also bring down costs of direct air carbon capture and storage (DACCS), one of the few scalable and sustainable geoengineering approaches (Breyer et al., 2020). The combination of abrupt global decarbonization and renewable DACCS could enable a return to Holocene-like conditions by the end of the century (Breyer et al., 2021; Abbott B., 2022).
Now that the technology and economics for rapid decarbonization and atmospheric drawdown of CO2 are in place, meeting our moral responsibility to restore the Earth’s climate is in reach. Rather than aiming for 1.5–2 °C of warming—a level of change that would cause radical transformation and widespread destruction throughout the permafrost domain (Farquharson et al., 2019; King et al., 2020; Ritchie et al., 2021)—we must aim for restoring Holocene-like climate conditions by stopping fossil fuel burning while supporting natural GHG sinks and developing negative emissions technologies (Breyer et al., 2020; Abbott B., 2022).
While pursuing abrupt GHG drawdown at global scales, we must support and empower communities in the permafrost domain who are adapting to unprecedented environmental and economic changes, and who are essential to the sustainable conservation of these ecosystems (Díaz et al., 2019; Proverbs et al., 2020; Chapin et al., 2022; Mettiäinen et al., 2022). For both practical and ethical reasons, we need to follow the guidance of the Indigenous and other local peoples with the most at stake and the deepest knowledge of the complex ecological responses across the permafrost domain (Kimmerer, 2002; Proverbs et al., 2020; Chapin, 2021). Greater recognition of forums such as the Arctic Council and Inuit Circumpolar Council could contribute to this goal (Johnson, 2010; Kristoffersen and Langhelle, 2017; Arctic Council, 2022; ICC, 2022; Koivurova and Smieszek, 2022). We call on people everywhere to share and promote the empowering and evidence-based assessment that we have the tools to reverse climate change and protect the irreplaceable permafrost domain.
Author Contributions
BA and LG conceived of the paper concept. The entire co-author team collaboratively wrote and revised the manuscript.
Funding
This work was supported by the U.S. National Science Foundation (award numbers 1916565, 1916567, 1916576, 1906381, and 1931333). VGS was supported by NGEE Arctic, a project funded by the Department of Energy’s Biological and Environmental Research Program (ORNL Contract No. DE-AC05-00OR22725 awarded to UT-Battelle, LLC). JMF was supported by the Laboratory Directed Research and Development program at Sandia National Laboratories. Sandia National Laboratories is a multimission laboratory managed and operated by National Technology and Engineering Solutions of Sandia, LLC, a wholly owned subsidiary of Honeywell International Inc., for the U.S. Department of Energy’s National Nuclear Security Administration under contract DE-NA0003525. Artwork for Figure 1 was created by Brenna Kilpatrick. We thank the participants in the Permafrost Carbon Feedback Dialogues for their ideas and feedback, including Nathan Obed, Dana Tizya-Tramm, and Elizabeth May. We dedicate this manuscript to the late RM and his family and friends.
Author Disclaimer
Any subjective views or opinions that might be expressed in the paper do not necessarily represent the views of the U.S. Department of Energy or the United States Government.
Conflict of Interest
Since 2016, MB has been the chair of Innovative Breakthrough Energy Technology Ltd., which invests in non-carbon energy generation, negative emissions technology, and climate adaptation.
The authors declare that this study received funding from Sandia, LLC. The funder was not involved in the study design, collection, analysis, interpretation of data, the writing of this article or the decision to submit it for publication.
Publisher’s Note
All claims expressed in this article are solely those of the authors and do not necessarily represent those of their affiliated organizations, or those of the publisher, the editors and the reviewers. Any product that may be evaluated in this article, or claim that may be made by its manufacturer, is not guaranteed or endorsed by the publisher.
References
Abbott, B., Chelsea, A., Nicholas, N., Peter, S., Marina, M., Sayedeh, S. S., et al. (2022a). Accelerating the Renewable Energy Revolution to Get Back to the Holocene. doi:10.13140/RG.2.2.35491.12326
Abbott, B. W., Baranov, V., Mendoza-Lera, C., Nikolakopoulou, M., Harjung, A., Kolbe, T., et al. (2016a). Using Multi-Tracer Inference to Move beyond Single-Catchment Ecohydrology. Earth-Science Rev. 160, 19–42. doi:10.1016/j.earscirev.2016.06.014
Abbott, B. W., Bishop, K., Zarnetske, J. P., Minaudo, C., Chapin, F. S., Krause, S., et al. (2019). Human Domination of the Global Water Cycle Absent from Depictions and Perceptions. Nat. Geosci. 12, 533–540. doi:10.1038/s41561-019-0374-y
Abbott, B. W., Bliss, A., Mitchell, L., Barros, L., Moyer, T., Moore, F., et al. (2021a). Clean Electrification of the U.S. Economy. Provo, Utah, USA: Brigham Young University. doi:10.13140/RG.2.2.25581.10725
Abbott, B. W., Jones, J. B., Godsey, S. E., Larouche, J. R., and Bowden, W. B. (2015). Patterns and Persistence of Hydrologic Carbon and Nutrient Export from Collapsing Upland Permafrost. Biogeosciences 12, 3725–3740. doi:10.5194/bg-12-3725-2015
Abbott, B. W., Jones, J. B., Schuur, E. A. G., Bowden, W. B., Bret-Harte, M. S., Epstein, H. E., et al. (2016b). Biomass Offsets Little or None of Permafrost Carbon Release from Soils, Streams, and Wildfire: an Expert Assessment. Environ. Res. Lett. 11, 034014. doi:10.1088/1748-9326/11/3/034014
Abbott, B. W. (2022b). “Permafrost Climate Feedbacks,” in Global Arctic: An Introduction to the Multifaceted Dynamics of the Arctic. Editors M. Finger, and G. Rekvig (Cham: Springer International Publishing), 189–209. doi:10.1007/978-3-030-81253-9_10
Abbott, B. W., Rocha, A. V., Shogren, A., Zarnetske, J. P., Iannucci, F., Bowden, W. B., et al. (2021b). Tundra Wildfire Triggers Sustained Lateral Nutrient Loss in Alaskan Arctic. Glob. Change Biol. 27, 1408–1430. doi:10.1111/gcb.15507
Alling, V., Porcelli, D., Mörth, C.-M., Anderson, L. G., Sanchez-Garcia, L., Gustafsson, Ö., et al. (2012). Degradation of Terrestrial Organic Carbon, Primary Production and Out-Gassing of CO2 in the Laptev and East Siberian Seas as Inferred from δ13C Values of DIC. Geochimica Cosmochimica Acta 95, 143–159. doi:10.1016/j.gca.2012.07.028
AMAP (2021). Arctic Climate Change Update 2021: Key Trends and Impacts. Tromsø, Norway: Arctic Monitoring and Assessment Programme (AMAP). Available at: https://www.amap.no/documents/download/6759/inline (Accessed May 15, 2022).
AMAP (2011). Snow, Water, Ice and Permafrost in the Arctic (SWIPA): Climate Change Adn the Cryosphere. Oslo, Norway: Arctic Monitoring and Assessment Programme (AMAP). Available at: http://www.amap.no/documents/doc/snow-water-ice-and-permafrost-in-the-arctic-swipa-climate-change-and-the-cryosphere/743.
Andreassen, K., Hubbard, A., Winsborrow, M., Patton, H., Vadakkepuliyambatta, S., Plaza-Faverola, A., et al. (2017). Massive Blow-Out Craters Formed by Hydrate-Controlled Methane Expulsion from the Arctic Seafloor. Science 356, 948–953. doi:10.1126/science.aal4500
Angelopoulos, M., Overduin, P. P., Jenrich, M., Nitze, I., Günther, F., Strauss, J., et al. (2021). Onshore Thermokarst Primes Subsea Permafrost Degradation. Geophys. Res. Lett. 48, e2021GL093881. doi:10.1029/2021GL093881
Angelopoulos, M., Overduin, P. P., Miesner, F., Grigoriev, M. N., and Vasiliev, A. A. (2020). Recent Advances in the Study of Arctic Submarine Permafrost. Permafr. Periglac Process 31, 442–453. doi:10.1002/ppp.2061
Anthony, K. M. W., Zimov, S. A., Grosse, G., Jones, M. C., Anthony, P. M., Iii, F. S. C., et al. (2014). A Shift of Thermokarst Lakes from Carbon Sources to Sinks during the Holocene Epoch. Nature 511, 452–456. doi:10.1038/nature13560
The Arctic Council, (2022). The Arctic Council. Norway: Arctic Council. Available at: https://arctic-council.org/ (Accessed February 20, 2022).
Arrigo, K. R., van Dijken, G. L., Cameron, M. A., van der Grient, J., Wedding, L. M., Hazen, L., et al. (2020). Synergistic Interactions Among Growing Stressors Increase Risk to an Arctic Ecosystem. Nat. Commun. 11, 6255. doi:10.1038/s41467-020-19899-z
Bamber, J. L., Oppenheimer, M., Kopp, R. E., Aspinall, W. P., and Cooke, R. M. (2019). Ice Sheet Contributions to Future Sea-Level Rise from Structured Expert Judgment. Proc. Natl. Acad. Sci. U.S.A. 116, 11195–11200. doi:10.1073/pnas.1817205116
Bamber, J. L., Tedstone, A. J., King, M. D., Howat, I. M., Enderlin, E. M., van den Broeke, M. R., et al. (2018). Land Ice Freshwater Budget of the Arctic and North Atlantic Oceans: 1. Data, Methods, and Results. J. Geophys. Res. Oceans 123, 1827–1837. doi:10.1002/2017JC013605
Bartsch, A., Pointner, G., Nitze, I., Efimova, A., Jakober, D., Ley, S., et al. (2021). Expanding Infrastructure and Growing Anthropogenic Impacts along Arctic Coasts. Environ. Res. Lett. 16, 115013. doi:10.1088/1748-9326/ac3176
Basu, N., Abass, K., Dietz, R., Krümmel, E., Rautio, A., and Weihe, P. (2022). The Impact of Mercury Contamination on Human Health in the Arctic: A State of the Science Review. Sci. Total Environ. 831, 154793. doi:10.1016/j.scitotenv.2022.154793
Bates, N. R., and Mathis, J. T. (2009). The Arctic Ocean Marine Carbon Cycle: Evaluation of Air-Sea CO<sub>2</sub> Exchanges, Ocean Acidification Impacts and Potential Feedbacks. Biogeosciences 6, 2433–2459. doi:10.5194/bg-6-2433-2009
Beer, C., Zimov, N., Olofsson, J., Porada, P., and Zimov, S. (2020). Protection of Permafrost Soils from Thawing by Increasing Herbivore Density. Sci. Rep. 10, 4170. doi:10.1038/s41598-020-60938-y
Behari, N., Sheriff, M. Z., Rahman, M. A., Nounou, M., Hassan, I., and Nounou, H. (2020). Chronic Leak Detection for Single and Multiphase Flow: A Critical Review on Onshore and Offshore Subsea and Arctic Conditions. J. Nat. Gas Sci. Eng. 81, 103460. doi:10.1016/j.jngse.2020.103460
Bergstrom, D. M., Wienecke, B. C., Hoff, J., Hughes, L., Lindenmayer, D. B., Ainsworth, T. D., et al. (2021). Combating Ecosystem Collapse from the Tropics to the Antarctic. Glob. Change Biol. 27, 1692–1703. doi:10.1111/gcb.15539
Berry, H. B., Whalen, D., and Lim, M. (2021). Long-term Ice-Rich Permafrost Coast Sensitivity to Air Temperatures and Storm Influence: Lessons from Pullen Island, Northwest Territories, Canada. Arct. Sci. 7, 723–745. doi:10.1139/as-2020-0003
Bhatt, U. S., Walker, D. A., Raynolds, M. K., Comiso, J. C., Epstein, H. E., Jia, G., et al. (2010). Circumpolar Arctic Tundra Vegetation Change Is Linked to Sea Ice Decline. Earth Interact. 14, 1–20. doi:10.1175/2010EI315.1
Biskaborn, B. K., Smith, S. L., Noetzli, J., Matthes, H., Vieira, G., Streletskiy, D. A., et al. (2019). Permafrost Is Warming at a Global Scale. Nat. Commun. 10, 264. doi:10.1038/s41467-018-08240-4
Boers, N., and Rypdal, M. (2021). Critical Slowing Down Suggests that the Western Greenland Ice Sheet Is Close to a Tipping Point. Proc. Natl. Acad. Sci. U.S.A. 118. doi:10.1073/pnas.2024192118
Bogdanov, D., Ram, M., Aghahosseini, A., Gulagi, A., Oyewo, A. S., Child, M., et al. (2021). Low-cost Renewable Electricity as the Key Driver of the Global Energy Transition towards Sustainability. Energy 227, 120467. doi:10.1016/j.energy.2021.120467
Boike, J., Grau, T., Heim, B., Günther, F., Langer, M., Muster, S., et al. (2016). Satellite-derived Changes in the Permafrost Landscape of Central Yakutia, 2000-2011: Wetting, Drying, and Fires. Glob. Planet. Change 139, 116–127. doi:10.1016/j.gloplacha.2016.01.001
Box, J. E., Colgan, W. T., Christensen, T. R., Schmidt, N. M., Lund, M., Parmentier, F.-J. W., et al. (2019). Key Indicators of Arctic Climate Change: 1971-2017. Environ. Res. Lett. 14, 045010. doi:10.1088/1748-9326/aafc1b
Breyer, C., Bogdanov, D., Khalili, S., and Keiner, D. (2021). “Solar Photovoltaics in 100% Renewable Energy Systems,”. Encyclopedia of Sustainability Science and Technology. Editor R. A. Meyers (New York, NY: Springer New York), 1–30. doi:10.1007/978-1-4939-2493-6_1071-1
Breyer, C., Fasihi, M., and Aghahosseini, A. (2020). Carbon Dioxide Direct Air Capture for Effective Climate Change Mitigation Based on Renewable Electricity: a New Type of Energy System Sector Coupling. Mitig. Adapt Strateg. Glob. Change 25, 43–65. doi:10.1007/s11027-019-9847-y
Bruhwiler, L., Parmentier, F.-J. W., Crill, P., Leonard, M., and Palmer, P. I. (2021). The Arctic Carbon Cycle and its Response to Changing Climate. Curr. Clim. Change Rep. 7, 14–34. doi:10.1007/s40641-020-00169-5
Calì Quaglia, F., Meloni, D., Muscari, G., Di Iorio, T., Ciardini, V., Pace, G., et al. (2022). On the Radiative Impact of Biomass-Burning Aerosols in the Arctic: The August 2017 Case Study. Remote Sens. 14, 313. doi:10.3390/rs14020313
Campeau, A., Bishop, K., Amvrosiadi, N., Billett, M. F., Garnett, M. H., Laudon, H., et al. (2019). Current Forest Carbon Fixation Fuels Stream CO2 Emissions. Nat. Commun. 10, 1876. doi:10.1038/s41467-019-09922-3
Canadell, J. G., Monteiro, P. M. S., Costa, M. H., Cunha, L. C. D., Cox, P. M., Eliseev, A. V., et al. (2021). Global Carbon and Other Biogeochemical Cycles and Feedbacks. Cambridge, New York: IPCC.
Carey, J. C., Abbott, B. W., and Rocha, A. V. (2019). Plant Uptake Offsets Silica Release from a Large Arctic Tundra Wildfire. Earth's Future 7, 1044–1057. doi:10.1029/2019EF001149
Carey, J. C., Gewirtzman, J., Johnston, S. E., Kurtz, A., Tang, J., Vieillard, A. M., et al. (2020). Arctic River Dissolved and Biogenic Silicon Exports-Current Conditions and Future Changes with Warming. Glob. Biogeochem. Cycles 34, e2019GB006308. doi:10.1029/2019GB006308
Carey, J. C., Tang, J., Templer, P. H., Kroeger, K. D., Crowther, T. W., Burton, A. J., et al. (2016). Temperature Response of Soil Respiration Largely Unaltered with Experimental Warming. Proc. Natl. Acad. Sci. U.S.A. 113, 13797–13802. doi:10.1073/pnas.1605365113
Carmack, E. C., Yamamoto‐Kawai, M., Haine, T. W. N., Bacon, S., Bluhm, B. A., Lique, C., et al. (2016). Freshwater and its Role in the Arctic Marine System: Sources, Disposition, Storage, Export, and Physical and Biogeochemical Consequences in the Arctic and Global Oceans. J. Geophys. Res. Biogeosci. 121, 675–717. doi:10.1002/2015JG003140
Chadburn, S. E., Burke, E. J., Gallego-Sala, A. V., Smith, N. D., Bret-Harte, M. S., Charman, D. J., et al. (2022). A New Approach to Simulate Peat Accumulation, Degradation and Stability in a Global Land Surface Scheme (JULES vn5.8_accumulate_soil) for Northern and Temperate Peatlands. Geosci. Model Dev. 15, 1633–1657. doi:10.5194/gmd-15-1633-2022
Chapin, F. S., and Díaz, S. (2020). Interactions between Changing Climate and Biodiversity: Shaping Humanity's Future. Proc. Natl. Acad. Sci. U.S.A. 117, 6295–6296. doi:10.1073/pnas.2001686117
Chapin, F. S. I. (2020). Grassroots Stewardship: Sustainability within Our Reach. Oxford University Press.
Chapin, F. S., Robards, M. D., Johnstone, J. F., Lantz, T. C., and Kokelj, S. V. (2013). “Case Study: Novel Socio-Ecological Systems in the North: Potential Pathways toward Ecological and Societal Resilience,” in Novel Ecosystems (John Wiley & Sons, Ltd), 334–344. doi:10.1002/9781118354186.ch40
Chapin, F. S., Weber, E. U., Bennett, E. M., Biggs, R., van den Bergh, J., Adger, W. N., et al. (2022). Earth Stewardship: Shaping a Sustainable Future through Interacting Policy and Norm Shifts. Ambio. 5, 1–14. doi:10.1007/s13280-022-01721-3
Chapin, III, F. S. (2021). Social and Environmental Change in the Arctic: Emerging Opportunities for Well-Being Transformations through Stewardship. E&S 26, art15. doi:10.5751/ES-12499-260315
Chen, L., Liu, L., Mao, C., Qin, S., Wang, J., Liu, F., et al. (2018). Nitrogen Availability Regulates Topsoil Carbon Dynamics after Permafrost Thaw by Altering Microbial Metabolic Efficiency. Nat. Commun. 9, 1–11. doi:10.1038/s41467-018-06232-y
Chen, Y., Liu, A., and Moore, J. C. (2020). Mitigation of Arctic Permafrost Carbon Loss through Stratospheric Aerosol Geoengineering. Nat. Commun. 11, 2430. doi:10.1038/s41467-020-16357-8
Chen, Y., Romps, D. M., Seeley, J. T., Veraverbeke, S., Riley, W. J., Mekonnen, Z. A., et al. (2021). Future Increases in Arctic Lightning and Fire Risk for Permafrost Carbon. Nat. Clim. Chang. 11, 404–410. doi:10.1038/s41558-021-01011-y
Cheng, F., Garzione, C., Li, X., Salzmann, U., Schwarz, F., Haywood, A. M., et al. (2022). Alpine Permafrost Could Account for a Quarter of Thawed Carbon Based on Plio-Pleistocene Paleoclimate Analogue. Nat. Commun. 13, 1329. doi:10.1038/s41467-022-29011-2
Chou, E., Southall, B. L., Robards, M., and Rosenbaum, H. C. (2021). International Policy, Recommendations, Actions and Mitigation Efforts of Anthropogenic Underwater Noise. Ocean Coast. Manag. 202, 105427. doi:10.1016/j.ocecoaman.2020.105427
Connolly, C. T., Cardenas, M. B., Burkart, G. A., Spencer, R. G. M., and McClelland, J. W. (2020). Groundwater as a Major Source of Dissolved Organic Matter to Arctic Coastal Waters. Nat. Commun. 11, 1479. doi:10.1038/s41467-020-15250-8
de Vrese, P., and Brovkin, V. (2021). Timescales of the Permafrost Carbon Cycle and Legacy Effects of Temperature Overshoot Scenarios. Nat. Commun. 12, 2688. doi:10.1038/s41467-021-23010-5
Denman, K., Christian, J. R., Steiner, N., Portner, H.-O., and Nojiri, Y. (2011). Potential Impacts of Future Ocean Acidification on Marine Ecosystems and Fisheries: Current Knowledge and Recommendations for Future Research. ICES J. Mar. Sci. 68, 1019–1029. doi:10.1093/icesjms/fsr074
Díaz, S., Settele, J., Brondízio, E. S., Ngo, H. T., Agard, J., Arneth, A., et al. (2019). Pervasive Human-Driven Decline of Life on Earth Points to the Need for Transformative Change. Science 366. doi:10.1126/science.aax3100
Doney, S. C., Fabry, V. J., Feely, R. A., and Kleypas, J. A. (2009). Ocean Acidification: The Other CO2 Problem. Annu. Rev. Mar. Sci. 1, 169–192. doi:10.1146/annurev.marine.010908.163834
Drake, T. W., Tank, S. E., Zhulidov, A. V., Holmes, R. M., Gurtovaya, T., and Spencer, R. G. M. (2018). Increasing Alkalinity Export from Large Russian Arctic Rivers. Environ. Sci. Technol. 52, 8302–8308. doi:10.1021/acs.est.8b01051
Egelkraut, D., Aronsson, K.-Å., Allard, A., Åkerholm, M., Stark, S., and Olofsson, J. (2018). Multiple Feedbacks Contribute to a Centennial Legacy of Reindeer on Tundra Vegetation. Ecosystems 21, 1545–1563. doi:10.1007/s10021-018-0239-z
Ellis, E. C., Gauthier, N., Klein Goldewijk, K., Bliege Bird, R., Boivin, N., Díaz, S., et al. (2021). People Have Shaped Most of Terrestrial Nature for at Least 12,000 Years. Proc. Natl. Acad. Sci. U.S.A. 118. doi:10.1073/pnas.2023483118
Errigo, I. M., Abbott, B. W., Mendoza, D. L., Chaney, R. A., Freeman, A., Glenn, J., et al. (2020). Human Health and Economic Costs of Air Pollution in Utah. Provo, Utah, USA: Brigham Young University.
Fahnestock, M. F., Bryce, J. G., McCalley, C. K., Montesdeoca, M., Bai, S., Li, Y., et al. (2019). Mercury Reallocation in Thawing Subarctic Peatlands. Geochem. Persp. Let., 33–38. doi:10.7185/geochemlet.1922
FAO (2021). Recarbonizing Global Soils – A Technical Manual of Recommended Management Practices. Rome, Italy: FAO. doi:10.4060/cb6378en
Farquharson, L. M., Romanovsky, V. E., Cable, W. L., Walker, D. A., Kokelj, S. V., and Nicolsky, D. (2019). Climate Change Drives Widespread and Rapid Thermokarst Development in Very Cold Permafrost in the Canadian High Arctic. Geophys. Res. Lett. 46, 6681–6689. doi:10.1029/2019GL082187
Feng, D., Gleason, C. J., Lin, P., Yang, X., Pan, M., and Ishitsuka, Y. (2021). Recent Changes to Arctic River Discharge. Nat. Commun. 12, 6917. doi:10.1038/s41467-021-27228-1
Fewster, R. E., Morris, P. J., Ivanovic, R. F., Swindles, G. T., Peregon, A. M., and Smith, C. J. (2022). Imminent Loss of Climate Space for Permafrost Peatlands in Europe and Western Siberia. Nat. Clim. Chang. 12, 373–379. doi:10.1038/s41558-022-01296-7
Finger, M., and Rekvig, G. (2022). Global Arctic. 1st ed. Springer International Publishing. Available at: https://link.springer.com/book/9783030812522 (Accessed February 18, 2022).
Fisher, J. A., Jacob, D. J., Soerensen, A. L., Amos, H. M., Steffen, A., and Sunderland, E. M. (2012). Riverine Source of Arctic Ocean Mercury Inferred from Atmospheric Observations. Nat. Geosci. 5, 499–504. doi:10.1038/ngeo1478
Foley, J. A., Ramankutty, N., Brauman, K. A., Cassidy, E. S., Gerber, J. S., Johnston, M., et al. (2011). Solutions for a Cultivated Planet. Nature 478, 337–342. doi:10.1038/nature10452
Forbes, B. C., Fauria, M. M., and Zetterberg, P. (2010). Russian Arctic Warming and 'greening' Are Closely Tracked by Tundra Shrub Willows. Glob. Change Biol. 16, 1542–1554. doi:10.1111/j.1365-2486.2009.02047.x
Forbes, B. C., Kumpula, T., Meschtyb, N., Laptander, R., Macias-Fauria, M., Zetterberg, P., et al. (2016). Sea Ice, Rain-On-Snow and Tundra Reindeer Nomadism in Arctic Russia. Biol. Lett. 12, 20160466. doi:10.1098/rsbl.2016.0466
Frederick, J. M., and Buffett, B. A. (2016). Submarine Groundwater Discharge as a Possible Formation Mechanism for Permafrost-Associated Gas Hydrate on the Circum-Arctic Continental Shelf. J. Geophys. Res. Solid Earth 121, 1383–1404. doi:10.1002/2015JB012627
Fritz, M., Vonk, J. E., and Lantuit, H. (2017). Collapsing Arctic Coastlines. Nat. Clim. Change 7, 6–7. doi:10.1038/nclimate3188
Froitzheim, N., Majka, J., and Zastrozhnov, D. (2021). Methane Release from Carbonate Rock Formations in the Siberian Permafrost Area during and after the 2020 Heat Wave. Proc. Natl. Acad. Sci. U.S.A. 118. doi:10.1073/pnas.2107632118
Gao, T., Zhang, Y., Kang, S., Abbott, B. W., Wang, X., Zhang, T., et al. (2021). Accelerating Permafrost Collapse on the Eastern Tibetan Plateau. Environ. Res. Lett. 16, 054023. doi:10.1088/1748-9326/abf7f0
Goosse, H., Kay, J. E., Armour, K. C., Bodas-Salcedo, A., Chepfer, H., Docquier, D., et al. (2018). Quantifying Climate Feedbacks in Polar Regions. Nat. Commun. 9, 1919. doi:10.1038/s41467-018-04173-0
Grosse, G., Harden, J., Turetsky, M., McGuire, A. D., Camill, P., Tarnocai, C., et al. (2011). Vulnerability of High-Latitude Soil Organic Carbon in North America to Disturbance. J. Geophys. Res. 116, 1–23. doi:10.1029/2010JG001507
Grotheer, H., Meyer, V., Riedel, T., Pfalz, G., Mathieu, L., Hefter, J., et al. (2020). Burial and Origin of Permafrost‐Derived Carbon in the Nearshore Zone of the Southern Canadian Beaufort Sea. Geophys. Res. Lett. 47, e2019GL085897. doi:10.1029/2019GL085897
Guimond, J. A., Mohammed, A. A., Walvoord, M. A., Bense, V. F., and Kurylyk, B. L. (2021). Saltwater Intrusion Intensifies Coastal Permafrost Thaw. Geophys. Res. Lett. 48, e2021GL094776. doi:10.1029/2021GL094776
Günther, F., Overduin, P. P., Yakshina, I. A., Opel, T., Baranskaya, A. V., and Grigoriev, M. N. (2015). Observing Muostakh Disappear: Permafrost Thaw Subsidence and Erosion of a Ground-Ice-Rich Island in Response to Arctic Summer Warming and Sea Ice Reduction. Cryosphere 9, 151–178. doi:10.5194/tc-9-151-2015
Haegel, N. M., Atwater, H., Barnes, T., Breyer, C., Burrell, A., Chiang, Y.-M., et al. (2019). Terawatt-scale Photovoltaics: Transform Global Energy. Science 364, 836–838. doi:10.1126/science.aaw1845
Hammond, W. M., Williams, A. P., Abatzoglou, J. T., Adams, H. D., Klein, T., López, R., et al. (2022). Global Field Observations of Tree Die-Off Reveal Hotter-Drought Fingerprint for Earth's Forests. Nat. Commun. 13, 1761. doi:10.1038/s41467-022-29289-2
Hanssen, S. V., Daioglou, V., Steinmann, Z. J. N., Doelman, J. C., Van Vuuren, D. P., and Huijbregts, M. a. J. (2020). The Climate Change Mitigation Potential of Bioenergy with Carbon Capture and Storage. Nat. Clim. Chang. 10, 1023–1029. doi:10.1038/s41558-020-0885-y
Harms, T. K., Cook, C. L., Wlostowski, A. N., Gooseff, M. N., and Godsey, S. E. (2019). Spiraling Down Hillslopes: Nutrient Uptake from Water Tracks in a Warming Arctic. Ecosystems 22, 1546–1560. doi:10.1007/s10021-019-00355-z
Harper, A. B., Powell, T., Cox, P. M., House, J., Huntingford, C., Lenton, T. M., et al. (2018). Land-use Emissions Play a Critical Role in Land-Based Mitigation for Paris Climate Targets. Nat. Commun. 9, 2938. doi:10.1038/s41467-018-05340-z
Hayes, D. J., McGuire, A. D., Kicklighter, D. W., Gurney, K. R., Burnside, T. J., and Melillo, J. M. (2011). Is the Northern High-Latitude Land-Based CO2 Sink Weakening? Glob. Biogeochem. Cycles 25. doi:10.1029/2010gb003813
Hewitt, R. E., Taylor, D. L., Genet, H., McGuire, A. D., and Mack, M. C. (2018). Below‐ground Plant Traits Influence Tundra Plant Acquisition of Newly Thawed Permafrost Nitrogen. J. Ecol. 107, 950–962. doi:10.1111/1365-2745.13062
Hjort, J., Karjalainen, O., Aalto, J., Westermann, S., Romanovsky, V. E., Nelson, F. E., et al. (2018). Degrading Permafrost Puts Arctic Infrastructure at Risk by Mid-century. Nat. Commun. 9, 5147. doi:10.1038/s41467-018-07557-4
Holloway, J. E., Lewkowicz, A. G., Douglas, T. A., Li, X., Turetsky, M. R., Baltzer, J. L., et al. (2020). Impact of Wildfire on Permafrost Landscapes: A Review of Recent Advances and Future Prospects. Permafr. Periglac Process 31, 371–382. doi:10.1002/ppp.2048
Holmes, R. M., McClelland, J. W., Peterson, B. J., Tank, S. E., Bulygina, E., Eglinton, T. I., et al. (2012). Seasonal and Annual Fluxes of Nutrients and Organic Matter from Large Rivers to the Arctic Ocean and Surrounding Seas. Estuaries Coasts 35, 369–382. doi:10.1007/s12237-011-9386-6
Huang, J., Zhang, X., Zhang, Q., Lin, Y., Hao, M., Luo, Y., et al. (2017). Recently Amplified Arctic Warming Has Contributed to a Continual Global Warming Trend. Nat. Clim. Change 7, 875–879. doi:10.1038/s41558-017-0009-5
Hugelius, G. (2022). Global Stocktake Submission: “Country” of Permafrost. Stockholm, Sweden: Stockholm University. Available at: https://unfccc.int/documents/461587 (Accessed May 4, 2022).
Hugelius, G., Strauss, J., Zubrzycki, S., Harden, J. W., Schuur, E. A. G., Ping, C.-L., et al. (2014). Estimated Stocks of Circumpolar Permafrost Carbon with Quantified Uncertainty Ranges and Identified Data Gaps. Biogeosciences 11, 6573–6593. doi:10.5194/bg-11-6573-2014
ICC (2022). Inuit Circumpolar Council – United Voice of the Arctic. Available at: https://www.inuitcircumpolar.com/ (Accessed February 20, 2022).
IPCC (2021). IPCC, 2021: Climate Change 2021: The Physical Science Basis. Contribution of Working Group 1 to the Sixth Assessment Report of the Intergovernmental Panel on Climate Change. Cambridge University Press. Available at: https://www.ipcc.ch/report/ar6/wg1/downloads/report/IPCC_AR6_WGI_Full_Report.pdf.
IPCC (2019). Special Report on the Ocean and Cryosphere in a Changing Climate. Available at: https://www.ipcc.ch/srocc/ (Accessed November 9, 2021).
Irrgang, A. M., Bendixen, M., Farquharson, L. M., Baranskaya, A. V., Erikson, L. H., Gibbs, A. E., et al. (2022). Drivers, Dynamics and Impacts of Changing Arctic Coasts. Nat. Rev. Earth Environ. 3, 39–54. doi:10.1038/s43017-021-00232-1
Jacobson, M. Z., von Krauland, A.-K., Coughlin, S. J., Palmer, F. C., and Smith, M. M. (2022). Zero Air Pollution and Zero Carbon from All Energy at Low Cost and without Blackouts in Variable Weather throughout the U.S. With 100% Wind-Water-Solar and Storage. Renew. Energy 184, 430–442. doi:10.1016/j.renene.2021.11.067
Johnson, L. (2010). The Fearful Symmetry of Arctic Climate Change: Accumulation by Degradation. Environ. Plan. D. 28, 828–847. doi:10.1068/d9308
Jones, B. M., Arp, C. D., Jorgenson, M. T., Hinkel, K. M., Schmutz, J. A., and Flint, P. L. (2009). Increase in the Rate and Uniformity of Coastline Erosion in Arctic Alaska. Geophys. Res. Lett. 36, a–n. doi:10.1029/2008GL036205
Jorgenson, M. T., Harden, J., Kanevskiy, M., O’Donnell, J., Wickland, K., Ewing, S., et al. (2013). Reorganization of Vegetation, Hydrology and Soil Carbon after Permafrost Degradation across Heterogeneous Boreal Landscapes. Environ. Res. Lett. 8, 035017. doi:10.1088/1748-9326/8/3/035017
Kessler, L. (2017). Estimating the Economic Impact of the Permafrost Carbon Feedback. Clim. Change Econ. 08, 1750008. doi:10.1142/S2010007817500087
Keuper, F., Bodegom, P. M., Dorrepaal, E., Weedon, J. T., Hal, J., Logtestijn, R. S. P., et al. (2012). A Frozen Feast: Thawing Permafrost Increases Plant-Available Nitrogen in Subarctic Peatlands. Glob. Change Biol. 18, 1998–2007. doi:10.1111/j.1365-2486.2012.02663.x
Kimmerer, R. W. (2002). Weaving Traditional Ecological Knowledge into Biological Education: A Call to Action. BioScience 52, 432–438. doi:10.1641/0006-3568(2002)052[0432:wtekib]2.0.co;2
King, M. D., Howat, I. M., Candela, S. G., Noh, M. J., Jeong, S., Noël, B. P. Y., et al. (2020). Dynamic Ice Loss from the Greenland Ice Sheet Driven by Sustained Glacier Retreat. Commun. Earth Environ. 1, 1–7. doi:10.1038/s43247-020-0001-2
Kling, G. (2010). “Land Water Interactions,” in Alaska’s Changing Arctic: Ecological Consequences for Tundra, Streams, and Lakes.
Koivurova, T., and Smieszek, M. (2022). “The Arctic Council at 25: Incremental Building of a More Ambitious Inter-governmental Forum,” in Global Arctic: An Introduction to the Multifaceted Dynamics of the Arctic. Editors M. Finger, and G. Rekvig (Cham: Springer International Publishing), 407–424. doi:10.1007/978-3-030-81253-9_21
Kokelj, S. V., and Jorgenson, M. T. (2013). Advances in Thermokarst Research. Permafr. Periglac. Process. 24, 108–119. doi:10.1002/ppp.1779
Kokelj, S. V., Kokoszka, J., van der Sluijs, J., Rudy, A. C. A., Tunnicliffe, J., Shakil, S., et al. (2021). Thaw-driven Mass Wasting Couples Slopes with Downstream Systems, and Effects Propagate through Arctic Drainage Networks. Cryosphere 15, 3059–3081. doi:10.5194/tc-15-3059-2021
Kreutzweiser, D. P., Hazlett, P. W., and Gunn, J. M. (2008). Logging Impacts on the Biogeochemistry of Boreal Forest Soils and Nutrient Export to Aquatic Systems: A Review. Environ. Rev. 16, 157–179. doi:10.1139/A08-006
Kristoffersen, B., and Langhelle, O. (2017). “Sustainable Development as a Global-Arctic Matter: Imaginaries and Controversies,” in Governing Arctic Change: Global Perspectives. Editors K. Keil, and S. Knecht (London: Palgrave Macmillan UK), 21–41. doi:10.1057/978-1-137-50884-3_2
Kropp, H., Loranty, M. M., Natali, S. M., Kholodov, A. L., Rocha, A. V., Myers-Smith, I., et al. (2020). Shallow Soils Are Warmer under Trees and Tall Shrubs across Arctic and Boreal Ecosystems. Environ. Res. Lett. 16, 015001. doi:10.1088/1748-9326/abc994
Lamers, L. P. M., Vile, M. A., Grootjans, A. P., Acreman, M. C., van Diggelen, R., Evans, M. G., et al. (2015). Ecological Restoration of Rich Fens in Europe and North America: from Trial and Error to an Evidence‐based Approach. Biol. Rev. 90, 182–203. doi:10.1111/brv.12102
Lantuit, H., Overduin, P. P., Couture, N., Wetterich, S., Aré, F., Atkinson, D., et al. (2012). The Arctic Coastal Dynamics Database: A New Classification Scheme and Statistics on Arctic Permafrost Coastlines. Estuaries Coasts 35, 383–400. doi:10.1007/s12237-010-9362-6
Lawrence, D. M., Koven, C. D., Swenson, S. C., Riley, W. J., and Slater, A. G. (2015). Permafrost Thaw and Resulting Soil Moisture Changes Regulate Projected High-Latitude CO 2 and CH 4 Emissions. Environ. Res. Lett. 10, 094011. doi:10.1088/1748-9326/10/9/094011
Lawrence, M. G., Schäfer, S., Muri, H., Scott, V., Oschlies, A., Vaughan, N. E., et al. (2018). Evaluating Climate Geoengineering Proposals in the Context of the Paris Agreement Temperature Goals. Nat. Commun. 9, 3734. doi:10.1038/s41467-018-05938-3
Lewis, K. M., van Dijken, G. L., and Arrigo, K. R. (2020). Changes in Phytoplankton Concentration Now Drive Increased Arctic Ocean Primary Production. Science 369, 198–202. doi:10.1126/science.aay8380
Lindgren, A., Hugelius, G., and Kuhry, P. (2018). Extensive Loss of Past Permafrost Carbon but a Net Accumulation into Present-Day Soils. Nature 560, 219–222. doi:10.1038/s41586-018-0371-0
Loiko, S. V., Pokrovsky, O. S., Raudina, T. V., Lim, A., Kolesnichenko, L. G., Shirokova, L. S., et al. (2017). Abrupt Permafrost Collapse Enhances Organic Carbon, CO 2 , Nutrient and Metal Release into Surface Waters. Chem. Geol. 471, 153–165. doi:10.1016/j.chemgeo.2017.10.002
Loranty, M. M., Abbott, B. W., Blok, D., Douglas, T. A., Epstein, H. E., Forbes, B. C., et al. (2018). Reviews and Syntheses: Changing Ecosystem Influences on Soil Thermal Regimes in Northern High-Latitude Permafrost Regions. Biogeosciences 15, 5287–5313. doi:10.5194/bg-15-5287-2018
Lupascu, M., Welker, J. M., Seibt, U., Maseyk, K., Xu, X., and Czimczik, C. I. (2013). High Arctic Wetting Reduces Permafrost Carbon Feedbacks to Climate Warming. Nat. Clim. Change 4, 51–55. doi:10.1038/nclimate2058
MacDonald, E. N., Tank, S. E., Kokelj, S. V., Froese, D. G., and Hutchins, R. H. S. (2021). Permafrost-derived Dissolved Organic Matter Composition Varies across Permafrost End-Members in the Western Canadian Arctic. Environ. Res. Lett. 16, 024036. doi:10.1088/1748-9326/abd971
MacDougall, A. H. (2021). Estimated Effect of the Permafrost Carbon Feedback on the Zero Emissions Commitment to Climate Change. Biogeosciences 18, 4937–4952. doi:10.5194/bg-18-4937-2021
Mack, M. C., Walker, X. J., Johnstone, J. F., Alexander, H. D., Melvin, A. M., Jean, M., et al. (2021). Carbon Loss from Boreal Forest Wildfires Offset by Increased Dominance of Deciduous Trees. Science 372, 280–283. doi:10.1126/science.abf3903
Malone, E. T., Abbott, B. W., Klaar, M. J., Kidd, C., Sebilo, M., Milner, A. M., et al. (2018). Decline in Ecosystem δ13C and Mid-successional Nitrogen Loss in a Two-Century Postglacial Chronosequence. Ecosystems 21, 1659–1675. doi:10.1007/s10021-018-0245-1
Mann, P. J., Strauss, J., Palmtag, J., Dowdy, K., Ogneva, O., Fuchs, M., et al. (2022). Degrading Permafrost River Catchments and Their Impact on Arctic Ocean Nearshore Processes. Ambio 51, 439–455. doi:10.1007/s13280-021-01666-z
Maslakov, A., Zotova, L., Komova, N., Grishchenko, M., Zamolodchikov, D., and Zelensky, G. (2021). Vulnerability of the Permafrost Landscapes in the Eastern Chukotka Coastal Plains to Human Impact and Climate Change. Land 10, 445. doi:10.3390/land10050445
Masrur, A., Petrov, A. N., and DeGroote, J. (2018). Circumpolar Spatio-Temporal Patterns and Contributing Climatic Factors of Wildfire Activity in the Arctic Tundra from 2001-2015. Environ. Res. Lett. 13, 014019. doi:10.1088/1748-9326/aa9a76
McCarty, J. L., Smith, T. E. L., and Turetsky, M. R. (2020). Arctic Fires Re-emerging. Nat. Geosci. 13, 658–660. doi:10.1038/s41561-020-00645-5
McClelland, J. W., Holmes, R. M., Dunton, K. H., and Macdonald, R. W. (2012). The Arctic Ocean Estuary. Estuaries Coasts 35, 353–368. doi:10.1007/s12237-010-9357-3
McGuire, A. D., Lawrence, D. M., Koven, C., Clein, J. S., Burke, E., Chen, G., et al. (2018). Dependence of the Evolution of Carbon Dynamics in the Northern Permafrost Region on the Trajectory of Climate Change. Proc. Natl. Acad. Sci. U.S.A. 115, 3882–3887. doi:10.1073/pnas.1719903115
Meredith, M., Sommerkorn, M., Cassotta, S., Derksen, C., Ekaykin, A., Hollowed, A., et al. (2019). Polar Regions. Cambridge, New York: Cambridge University Press. Available at: https://www.ipcc.ch/site/assets/uploads/sites/3/2019/11/07_SROCC_Ch03_FINAL.pdf.
Mettiäinen, I., Buck, H. J., MacMartin, D. G., and Ricke, K. L. (2022). 'Bog Here, Marshland There': Tensions in Co-producing Scientific Knowledge on Solar Geoengineering in the Arctic. Environ. Res. Lett. 17, 045001. doi:10.1088/1748-9326/ac5715
Miner, K. R., D’Andrilli, J., Mackelprang, R., Edwards, A., Malaska, M. J., Waldrop, M. P., et al. (2021). Emergent Biogeochemical Risks from Arctic Permafrost Degradation. Nat. Clim. Chang. 11, 809–819. doi:10.1038/s41558-021-01162-y
Mishra, U., Hugelius, G., Shelef, E., Yang, Y., Strauss, J., Lupachev, A., et al. (2021). Spatial Heterogeneity and Environmental Predictors of Permafrost Region Soil Organic Carbon Stocks. Sci. Adv. 7, eaaz5236. doi:10.1126/sciadv.aaz5236
Mu, C., Abbott, B. W., Norris, A. J., Mu, M., Fan, C., Chen, X., et al. (2020a). The Status and Stability of Permafrost Carbon on the Tibetan Plateau. Earth-Science Rev. 211, 103433. doi:10.1016/j.earscirev.2020.103433
Mu, C., Schuster, P. F., Abbott, B. W., Kang, S., Guo, J., Sun, S., et al. (2020b). Permafrost Degradation Enhances the Risk of Mercury Release on Qinghai-Tibetan Plateau. Sci. Total Environ. 708, 135127. doi:10.1016/j.scitotenv.2019.135127
Myers-Smith, I. H., Kerby, J. T., Phoenix, G. K., Bjerke, J. W., Epstein, H. E., Assmann, J. J., et al. (2020). Complexity Revealed in the Greening of the Arctic. Nat. Clim. Chang. 10, 106–117. doi:10.1038/s41558-019-0688-1
Natali, S. M., Holdren, J. P., Rogers, B. M., Treharne, R., Duffy, P. B., Pomerance, R., et al. (2021). Permafrost Carbon Feedbacks Threaten Global Climate Goals. Proc. Natl. Acad. Sci. U.S.A. 118. doi:10.1073/pnas.2100163118
Natali, S. M., Watts, J. D., Rogers, B. M., Potter, S., Ludwig, S. M., Selbmann, A.-K., et al. (2019). Large Loss of CO2 in Winter Observed across the Northern Permafrost Region. Nat. Clim. Chang. 9, 852–857. doi:10.1038/s41558-019-0592-8
Neumann, R. B., Moorberg, C. J., Lundquist, J. D., Turner, J. C., Waldrop, M. P., McFarland, J. W., et al. (2019). Warming Effects of Spring Rainfall Increase Methane Emissions from Thawing Permafrost. Geophys. Res. Lett. 46, 1393–1401. doi:10.1029/2018GL081274
Olefeldt, D., Goswami, S., Grosse, G., Hayes, D., Hugelius, G., Kuhry, P., et al. (2016). Circumpolar Distribution and Carbon Storage of Thermokarst Landscapes. Nat. Commun. 7, 13043. doi:10.1038/ncomms13043
Olson, R. L. (2012). Soft Geoengineering: A Gentler Approach to Addressing Climate Change. Environ. Sci. Policy Sustain. Dev. 54, 29–39. doi:10.1080/00139157.2012.711672
Ouyang, Z., Qi, D., Chen, L., Takahashi, T., Zhong, W., DeGrandpre, M. D., et al. (2020). Sea-ice Loss Amplifies Summertime Decadal CO2 Increase in the Western Arctic Ocean. Nat. Clim. Chang. 10, 678–684. doi:10.1038/s41558-020-0784-2
Overduin, P. P., Schneider von Deimling, T., Miesner, F., Grigoriev, M. N., Ruppel, C., Vasiliev, A., et al. (2019). Submarine Permafrost Map in the Arctic Modeled Using 1‐D Transient Heat Flux (SuPerMAP). J. Geophys. Res. Oceans 124, 3490–3507. doi:10.1029/2018JC014675
Parkinson, A. J., and Berner, J. (2009). Climate Change and Impacts on Human Health in the Arctic: an International Workshop on Emerging Threats and the Response of Arctic Communities to Climate Change. Int. J. Circumpolar Health 68, 84–91. doi:10.3402/ijch.v68i1.18295
Parkinson, C. L., and DiGirolamo, N. E. (2021). Sea Ice Extents Continue to Set New Records: Arctic, Antarctic, and Global Results. Remote Sens. Environ. 267, 112753. doi:10.1016/j.rse.2021.112753
Pearce, T. D., Ford, J. D., Laidler, G. J., Smit, B., Duerden, F., Allarut, M., et al. (2009). Community Collaboration and Climate Change Research in the Canadian Arctic. Polar Res. 28, 10–27. doi:10.1111/j.1751-8369.2008.00094.x
Perryman, C. R., Wirsing, J., Bennett, K. A., Brennick, O., Perry, A. L., Williamson, N., et al. (2020). Heavy Metals in the Arctic: Distribution and Enrichment of Five Metals in Alaskan Soils. PLOS ONE 15, e0233297. doi:10.1371/journal.pone.0233297
Plaza, C., Pegoraro, E., Bracho, R., Celis, G., Crummer, K. G., Hutchings, J. A., et al. (2019). Direct Observation of Permafrost Degradation and Rapid Soil Carbon Loss in Tundra. Nat. Geosci. 12, 627–631. doi:10.1038/s41561-019-0387-6
Polvani, L. M., Previdi, M., England, M. R., Chiodo, G., and Smith, K. L. (2020). Substantial Twentieth-Century Arctic Warming Caused by Ozone-Depleting Substances. Nat. Clim. Chang. 10, 130–133. doi:10.1038/s41558-019-0677-4
Proctor, J., Hsiang, S., Burney, J., Burke, M., and Schlenker, W. (2018). Estimating Global Agricultural Effects of Geoengineering Using Volcanic Eruptions. Nature 560, 480–483. doi:10.1038/s41586-018-0417-3
Proverbs, T., Lantz, T., and Gwich’in Tribal Council, (2020). Cumulative Environmental Impacts in the Gwich'in Cultural LandscapeCumulative Environmental Impacts in the Gwich’in Cultural Landscape. Sustainability 12, 4667. doi:10.3390/su12114667
Randers, J., and Goluke, U. (2020). An Earth System Model Shows Self-Sustained Thawing of Permafrost Even if All Man-Made GHG Emissions Stop in 2020. Sci. Rep. 10, 18456. doi:10.1038/s41598-020-75481-z
Rawlins, M. A., Steele, M., Holland, M. M., Adam, J. C., Cherry, J. E., Francis, J. A., et al. (2010). Analysis of the Arctic System for Freshwater Cycle Intensification: Observations and Expectations. J. Clim. 23, 5715–5737. doi:10.1175/2010JCLI3421.1
Riedlinger, D., and Berkes, F. (2001). Contributions of Traditional Knowledge to Understanding Climate Change in the Canadian Arctic. Polar Rec. 37, 315–328. doi:10.1017/S0032247400017058
Ritchie, P. D. L., Clarke, J. J., Cox, P. M., and Huntingford, C. (2021). Overshooting Tipping Point Thresholds in a Changing Climate. Nature 592, 517–523. doi:10.1038/s41586-021-03263-2
Rocha, A. V., Blakely, B., Jiang, Y., Wright, K. S., and Curasi, S. R. (2018). Is Arctic Greening Consistent with the Ecology of Tundra? Lessons from an Ecologically Informed Mass Balance Model. Environ. Res. Lett. 13, 125007. doi:10.1088/1748-9326/aaeb50
Rocha, A. V., and Shaver, G. R. (2011). Postfire Energy Exchange in Arctic Tundra: the Importance and Climatic Implications of Burn Severity. Glob. Change Biol. 17, 2831–2841. doi:10.1111/j.1365-2486.2011.02441.x
Rockström, J., Gaffney, O., Rogelj, J., Meinshausen, M., Nakicenovic, N., and Schellnhuber, H. J. (2017). A Roadmap for Rapid Decarbonization. Science 355, 1269–1271. doi:10.1126/science.aah3443
Rodríguez-Cardona, B. M., Coble, A. A., Wymore, A. S., Kolosov, R., Podgorski, D. C., Zito, P., et al. (2020). Wildfires Lead to Decreased Carbon and Increased Nitrogen Concentrations in Upland Arctic Streams. Sci. Rep. 10, 8722. doi:10.1038/s41598-020-65520-0
Sayedi, S. S., Abbott, B. W., Thornton, B. F., Frederick, J. M., Vonk, J. E., Overduin, P., et al. (2020). Subsea Permafrost Carbon Stocks and Climate Change Sensitivity Estimated by Expert Assessment. Environ. Res. Lett. 15, 124075. doi:10.1088/1748-9326/abcc29
Schädel, C., Bader, M. K.-F., Schuur, E. A. G., Biasi, C., Bracho, R., Čapek, P., et al. (2016). Potential Carbon Emissions Dominated by Carbon Dioxide from Thawed Permafrost Soils. Nat. Clim. Change 6, 950–953. doi:10.1038/nclimate3054
Schaefer, K., Lantuit, H., Romanovsky, V., Schuur, E., and Gärtner-Roer, I. (2012). Policy Implications of Warming Permafrost. Available at: http://epic.awi.de/33086/1/permafrost.pdf (Accessed March 14, 2015).
Schmidt, J. I., Hausner, V. H., and Monz, C. (2021). Building Adaptive Capacity in a Changing Arctic by Use of Technology. E&S 26, art1. doi:10.5751/ES-12605-260401
Scholten, R. C., Jandt, R., Miller, E. A., Rogers, B. M., and Veraverbeke, S. (2021). Overwintering Fires in Boreal Forests. Nature 593, 399–404. doi:10.1038/s41586-021-03437-y
Schuster, P. F., Schaefer, K. M., Aiken, G. R., Antweiler, R. C., Dewild, J. F., Gryziec, J. D., et al. (2018). Permafrost Stores a Globally Significant Amount of Mercury. Geophys. Res. Lett. 45, 1463–1471. doi:10.1002/2017GL075571
Schuur, E. A. G., Abbott, B. W., Commane, R., Ernakovich, J. G., Euskirchen, E. S., Hugelius, G., et al. (2022). Permafrost and Climate Change: Carbon Cycle Feedbacks from the Warming Arctic. Annu. Rev. Environ. Resour.
Schuur, E. A. G., and Mack, M. C. (2018). Ecological Response to Permafrost Thaw and Consequences for Local and Global Ecosystem Services. Annu. Rev. Ecol. Evol. Syst. 49, 279–301. doi:10.1146/annurev-ecolsys-121415-032349
Schuur, E. A. G., McGuire, A. D., Schädel, C., Grosse, G., Harden, J. W., Hayes, D. J., et al. (2015). Climate Change and the Permafrost Carbon Feedback. Nature 520, 171–179. doi:10.1038/nature14338
Semiletov, I., Pipko, I., Gustafsson, Ö., Anderson, L. G., Sergienko, V., Pugach, S., et al. (2016). Acidification of East Siberian Arctic Shelf Waters through Addition of Freshwater and Terrestrial Carbon. Nat. Geosci. 9, 361–365. doi:10.1038/ngeo2695
Shindell, D., Ru, M., Zhang, Y., Seltzer, K., Faluvegi, G., Nazarenko, L., et al. (2021). Temporal and Spatial Distribution of Health, Labor, and Crop Benefits of Climate Change Mitigation in the United States. Proc. Natl. Acad. Sci. U.S.A. 118. doi:10.1073/pnas.2104061118
Shogren, A. J., Zarnetske, J. P., Abbott, B. W., Iannucci, F., and Bowden, W. B. (2020). We Cannot Shrug off the Shoulder Seasons: Addressing Knowledge and Data Gaps in an Arctic Headwater. Environ. Res. Lett. 15, 104027. doi:10.1088/1748-9326/ab9d3c
Shogren, A. J., Zarnetske, J. P., Abbott, B. W., Iannucci, F., Medvedeff, A., Cairns, S., et al. (2021). Arctic Concentration-Discharge Relationships for Dissolved Organic Carbon and Nitrate Vary with Landscape and Season. Limnol. Oceanogr. 66, S197–S215. doi:10.1002/lno.11682
Shu, S., Jain, A. K., Koven, C. D., and Mishra, U. (2020). Estimation of Permafrost SOC Stock and Turnover Time Using a Land Surface Model with Vertical Heterogeneity of Permafrost Soils. Glob. Biogeochem. Cycles 34, e2020GB006585. doi:10.1029/2020GB006585
Shur, Y. L., and Jorgenson, M. T. (2007). Patterns of Permafrost Formation and Degradation in Relation to Climate and Ecosystems. Permafr. Periglac. Process. 18, 7–19. doi:10.1002/ppp.582
Smith, N. D., Chadburn, S. E., Burke, E. J., Schanke Aas, K., Althuizen, I. H. J., Boike, J., et al. (2021). Explicitly Modelling Microtopography in Permafrost Landscapes in a Land-Surface Model (JULES vn5.4_microtopography). Geosci. Model Dev. Discuss., 1–43. doi:10.5194/gmd-2021-285
Smith, S. L., O’Neill, H. B., Isaksen, K., Noetzli, J., and Romanovsky, V. E. (2022). The Changing Thermal State of Permafrost. Nat. Rev. Earth Environ. 3, 10–23. doi:10.1038/s43017-021-00240-1
Steffen, W., Rockström, J., Richardson, K., Lenton, T. M., Folke, C., Liverman, D., et al. (2018). Trajectories of the Earth System in the Anthropocene. Proc. Natl. Acad. Sci. U.S.A. 115, 8252–8259. doi:10.1073/pnas.1810141115
Steinacher, M., Joos, F., Frölicher, T. L., Plattner, G.-K., and Doney, S. C. (2009). Imminent Ocean Acidification in the Arctic Projected with the NCAR Global Coupled Carbon Cycle-Climate Model. Biogeosciences 6, 515–533. doi:10.5194/bg-6-515-2009
Stern, G. A., Macdonald, R. W., Outridge, P. M., Wilson, S., Chételat, J., Cole, A., et al. (2012). How Does Climate Change Influence Arctic Mercury? Sci. Total Environ. 414, 22–42. doi:10.1016/j.scitotenv.2011.10.039
Stevens, J. T., and Latimer, A. M. (2015). Snowpack, Fire, and Forest Disturbance: Interactions Affect Montane Invasions by Non-native Shrubs. Glob. Change Biol. 21, 2379–2393. doi:10.1111/gcb.12824
St. Pierre, K. A., Zolkos, S., Shakil, S., Tank, S. E., St. Louis, V. L. V. L., and Kokelj, S. V. (2018). Unprecedented Increases in Total and Methyl Mercury Concentrations Downstream of Retrogressive Thaw Slumps in the Western Canadian Arctic. Environ. Sci. Technol. 52, 14099–14109. doi:10.1021/acs.est.8b05348
Strauss, J., Schirrmeister, L., Grosse, G., Fortier, D., Hugelius, G., Knoblauch, C., et al. (2017). Deep Yedoma Permafrost: A Synthesis of Depositional Characteristics and Carbon Vulnerability. Earth-Science Rev. 172, 75–86. doi:10.1016/j.earscirev.2017.07.007
Talucci, A. C., Loranty, M. M., and Alexander, H. D. (2022). Siberian Taiga and Tundra Fire Regimes from 2001-2020. Environ. Res. Lett. 17, 025001. doi:10.1088/1748-9326/ac3f07
Tank, S. E., Striegl, R. G., McClelland, J. W., and Kokelj, S. V. (2016). Multi-decadal Increases in Dissolved Organic Carbon and Alkalinity Flux from the Mackenzie Drainage Basin to the Arctic Ocean. Environ. Res. Lett. 11, 054015. doi:10.1088/1748-9326/11/5/054015
Tank, S. E., Vonk, J. E., Walvoord, M. A., McClelland, J. W., Laurion, I., and Abbott, B. W. (2020). Landscape Matters: Predicting the Biogeochemical Effects of Permafrost Thaw on Aquatic Networks with a State Factor Approach. Permafr. Periglac Process 31, 358–370. doi:10.1002/ppp.2057
Tanski, G., Bröder, L., Wagner, D., Knoblauch, C., Lantuit, H., Beer, C., et al. (2021). Permafrost Carbon and CO2 Pathways Differ at Contrasting Coastal Erosion Sites in the Canadian Arctic. Front. Earth Sci. 9. doi:10.3389/feart.2021.630493
Terhaar, J., Lauerwald, R., Regnier, P., Gruber, N., and Bopp, L. (2021). Around One Third of Current Arctic Ocean Primary Production Sustained by Rivers and Coastal Erosion. Nat. Commun. 12, 169. doi:10.1038/s41467-020-20470-z
Thornton, B. F., Geibel, M. C., Crill, P. M., Humborg, C., and Mörth, C.-M. (2016). Methane Fluxes from the Sea to the Atmosphere across the Siberian Shelf Seas. Geophys. Res. Lett. 43, 5869–5877. doi:10.1002/2016GL068977
Tilmes, S., Müller, R., and Salawitch, R. (2008). The Sensitivity of Polar Ozone Depletion to Proposed Geoengineering Schemes. Science 320, 1201–1204. doi:10.1126/science.1153966
Toohey, R. C., Herman-Mercer, N. M., Schuster, P. F., Mutter, E. A., and Koch, J. C. (2016). Multidecadal Increases in the Yukon River Basin of Chemical Fluxes as Indicators of Changing Flowpaths, Groundwater, and Permafrost. Geophys. Res. Lett. 43 (12), 120–12130. doi:10.1002/2016GL070817
Treat, C. C., Wollheim, W. M., Varner, R. K., and Bowden, W. B. (2016). Longer Thaw Seasons Increase Nitrogen Availability for Leaching during Fall in Tundra Soils. Environ. Res. Lett. 11, 064013. doi:10.1088/1748-9326/11/6/064013
Treat, C., Natali, S. M., Ernakovich, J., Iversen, C. M., Lupascu, M., McGuire, A. D., et al. (2015). A Pan-Arctic Synthesis of CH4 and CO2 Production from Anoxic Soil Incubations. Glob. Change Biol. doi:10.1111/gcb.12875
Treharne, R., Rogers, B. M., Gasser, T., MacDonald, E., and Natali, S. (2022). 3. doi:10.3389/fclim.2021.716464Identifying Barriers to Estimating Carbon Release from Interacting Feedbacks in a Warming ArcticFront. Clim.
Tuana, N., Sriver, R. L., Svoboda, T., Olson, R., Irvine, P. J., Haqq-Misra, J., et al. (2012). Towards Integrated Ethical and Scientific Analysis of Geoengineering: A Research Agenda. Ethics, Policy & Environ. 15, 136–157. doi:10.1080/21550085.2012.685557
Turetsky, M. R., Abbott, B. W., Jones, M. C., Anthony, K. W., Olefeldt, D., Schuur, E. A. G., et al. (2020). Carbon Release through Abrupt Permafrost Thaw. Nat. Geosci. 13, 138–143. doi:10.1038/s41561-019-0526-0
UNEP (2019). Global Mercury Assessment 2018. Geneva, Switzerland: United Nations Environmental Progamme, Chemicals and Health Branch. Available at: https://www.unep.org/resources/publication/global-mercury-assessment-2018.
Van Seters, T. E., and Price, J. S. (2001). The Impact of Peat Harvesting and Natural Regeneration on the Water Balance of an Abandoned Cutover Bog, Quebec. Hydrol. Process. 15, 233–248. doi:10.1002/hyp.145
Veraverbeke, S., Delcourt, C. J. F., Kukavskaya, E., Mack, M., Walker, X., Hessilt, T., et al. (2021). Direct and Longer-Term Carbon Emissions from Arctic-Boreal Fires: A Short Review of Recent Advances. Curr. Opin. Environ. Sci. Health 23, 100277. doi:10.1016/j.coesh.2021.100277
Versen, J., Mnatsakanyan, Z., and Urpelainen, J. (2022). Concerns of Climate Intervention: Understanding Geoengineering Security Concerns in the Arctic and beyond. Clim. Change 171, 27. doi:10.1007/s10584-022-03345-8
Victoria, M., Haegel, N., Peters, I. M., Sinton, R., Jäger-Waldau, A., del Cañizo, C., et al. (2021). Solar Photovoltaics Is Ready to Power a Sustainable Future. Joule 5, 1041–1056. doi:10.1016/j.joule.2021.03.005
Vitali, R., Chadburn, S. E., Keuper, F., Harper, A. B., and Burke, E. J. (2022). Simulating Increased Permafrost Peatland Plant Productivity in Response to Belowground Fertilisation Using the JULES Land Surface Model. Nitrogen 3, 260–283. doi:10.3390/nitrogen3020018
Vohra, K., Vodonos, A., Schwartz, J., Marais, E. A., Sulprizio, M. P., and Mickley, L. J. (2021). Global Mortality from Outdoor Fine Particle Pollution Generated by Fossil Fuel Combustion: Results from GEOS-Chem. Environ. Res. 195, 110754. doi:10.1016/j.envres.2021.110754
Voigt, C., Marushchak, M. E., Abbott, B. W., Biasi, C., Elberling, B., Siciliano, S. D., et al. (2020). Nitrous Oxide Emissions from Permafrost-Affected Soils. Nat. Rev. Earth Environ. 1, 420–434. doi:10.1038/s43017-020-0063-9
Vonk, J. E., Sánchez-García, L., van Dongen, B. E., Alling, V., Kosmach, D., Charkin, A., et al. (2012). Activation of Old Carbon by Erosion of Coastal and Subsea Permafrost in Arctic Siberia. Nature 489, 137–140. doi:10.1038/nature11392
Walter, K. M., Chanton, J. P., Chapin, F. S., Schuur, E. A. G., and Zimov, S. A. (2008). Methane Production and Bubble Emissions from Arctic Lakes: Isotopic Implications for Source Pathways and Ages. J. Geophys. Res. 113. doi:10.1029/2007JG000569
Watson, J. E. M., Venter, O., Lee, J., Jones, K. R., Robinson, J. G., Possingham, H. P., et al. (2018). Protect the Last of the Wild. Nature 563, 27–30. doi:10.1038/d41586-018-07183-6
Whyte, K. P. (2018). Indigeneity in Geoengineering Discourses: Some Considerations. Ethics, Policy & Environ. 21, 289–307. doi:10.1080/21550085.2018.1562529
Whyte, K. (2020). Too Late for Indigenous Climate Justice: Ecological and Relational Tipping Points. WIREs Clim. Change 11, e603. doi:10.1002/wcc.603
Wiltshire, A. J., Burke, E. J., Chadburn, S. E., Jones, C. D., Cox, P. M., Davies-Barnard, T., et al. (2021). JULES-CN: a Coupled Terrestrial Carbon-Nitrogen Scheme (JULES vn5.1). Geosci. Model Dev. 14, 2161–2186. doi:10.5194/gmd-14-2161-2021
Wologo, E., Shakil, S., Zolkos, S., Textor, S., Ewing, S., Klassen, J., et al. (2021). Stream Dissolved Organic Matter in Permafrost Regions Shows Surprising Compositional Similarities but Negative Priming and Nutrient Effects. Glob. Biogeochem. Cycles 35, e2020GB006719. doi:10.1029/2020GB006719
Yamamoto-Kawai, M., McLaughlin, F. A., Carmack, E. C., Nishino, S., and Shimada, K. (2009). Aragonite Undersaturation in the Arctic Ocean: Effects of Ocean Acidification and Sea Ice Melt. Science 326, 1098–1100. doi:10.1126/science.1174190
Yang, G., Peng, Y., Abbott, B. W., Biasi, C., Wei, B., Zhang, D., et al. (2021). Phosphorus rather Than Nitrogen Regulates Ecosystem Carbon Dynamics after Permafrost Thaw. Glob. Change Biol. 27, 5818–5830. doi:10.1111/gcb.15845
Yao, T., Xue, Y., Chen, D., Chen, F., Thompson, L., Cui, P., et al. (2019). Recent Third Pole's Rapid Warming Accompanies Cryospheric Melt and Water Cycle Intensification and Interactions between Monsoon and Environment: Multidisciplinary Approach with Observations, Modeling, and Analysis. Bull. Amer. Meteor. Soc. 100, 423–444. doi:10.1175/BAMS-D-17-0057.1
Yue, S., Zhu, J., Chen, S., Xie, Q., Li, W., Li, L., et al. (2022). Brown Carbon from Biomass Burning Imposes Strong Circum-Arctic Warming. One Earth 5, 293–304. doi:10.1016/j.oneear.2022.02.006
Zampieri, L., and Goessling, H. F. (2019). Sea Ice Targeted Geoengineering Can Delay Arctic Sea Ice Decline but Not Global Warming. Earth's Future 7, 1296–1306. doi:10.1029/2019EF001230
Zarnetske, P. L., Gurevitch, J., Franklin, J., Groffman, P. M., Harrison, C. S., Hellmann, J. J., et al. (2021). Potential Ecological Impacts of Climate Intervention by Reflecting Sunlight to Cool Earth. Proc. Natl. Acad. Sci. U.S.A. 118. doi:10.1073/pnas.1921854118
Keywords: permafrost climate feedback, Arctic, Boreal, climate policy, renewable energy, ecosystem feedback, Earth stewardship, permafrost domain
Citation: Abbott BW, Brown M, Carey JC, Ernakovich J, Frederick JM, Guo L, Hugelius G, Lee RM, Loranty MM, Macdonald R, Mann PJ, Natali SM, Olefeldt D, Pearson P, Rec A, Robards M, Salmon VG, Sayedi SS, Schädel C, Schuur EAG, Shakil S, Shogren AJ, Strauss J, Tank SE, Thornton BF, Treharne R, Turetsky M, Voigt C, Wright N, Yang Y, Zarnetske JP, Zhang Q and Zolkos S (2022) We Must Stop Fossil Fuel Emissions to Protect Permafrost Ecosystems. Front. Environ. Sci. 10:889428. doi: 10.3389/fenvs.2022.889428
Received: 04 March 2022; Accepted: 23 May 2022;
Published: 29 June 2022.
Edited by:
Martin Siegert, Imperial College London, United KingdomReviewed by:
Fujun Niu, South China University of Technology, ChinaCopyright © 2022 Abbott, Brown, Carey, Ernakovich, Frederick, Guo, Hugelius, Lee, Loranty, Macdonald, Mann, Natali, Olefeldt, Pearson, Rec, Robards, Salmon, Sayedi, Schädel, Schuur, Shakil, Shogren, Strauss, Tank, Thornton, Treharne, Turetsky, Voigt, Wright, Yang, Zarnetske, Zhang and Zolkos. This is an open-access article distributed under the terms of the Creative Commons Attribution License (CC BY). The use, distribution or reproduction in other forums is permitted, provided the original author(s) and the copyright owner(s) are credited and that the original publication in this journal is cited, in accordance with accepted academic practice. No use, distribution or reproduction is permitted which does not comply with these terms.
*Correspondence: Benjamin W. Abbott, YmVuYWJib3R0QGJ5dS5lZHU=
†Deceased