- 1College of Architecture and Environment, Sichuan University, Chengdu, China
- 2State Key Laboratory for Structural Chemistry of Unstable and Stable Species, CAS Research/Education Center for Excellence in Molecular Sciences, Beijing National Laboratory for Molecular Sciences, Institute of Chemistry, Chinese Academy of Sciences, Beijing, China
- 3University of Chinese Academy of Sciences, Beijing, China
- 4The Institute of Chemical Physics, School of Chemistry and Chemical Engineering, Beijing Institute of Technology, Beijing, China
Coal-derived fly ash is a major aerosol composition in the atmosphere and presents a major challenge in understanding the atmospheric environment. In this study, the heterogeneous uptake of hydrogen peroxide and sulfur dioxide on coal fly ash was investigated using a Knudsen cell reactor coupled with a quadrupole mass spectrometer. The uptake coefficients were measured as a function of the initial mixing ratio of H2O2 and SO2 from 10 to 60 ppbv, and the temperature dependence of the uptake coefficients was also carried out over a range from 253 to 313 K. The mixing ratio of H2O2 and SO2 showed little effect on the uptake coefficients for these heterogeneous processes. As a function of temperature, the initial uptake coefficients of H2O2 and SO2 on coal fly ash were (0.48–1.65) × 10−4 and (1.50–8.54) × 10−5, respectively, which decreased with an increase in temperature. The steady-state uptake coefficients of H2O2 on coal fly ash were in the range of 2.46 × 10−5 to 4.84 × 10−5, which increased with an increase in temperature. Furthermore, we examined the synergistic effects between SO2 and H2O2 in their reaction on coal fly ash and found the existence of gaseous H2O2 appeared to enhance the ability of SO2 uptake on coal fly ash. Therefore, this finding suggests that the oxidation of SO2 on the surface of coal fly ash by gaseous H2O2 would promote SO2 consumption and transformation.
Introduction
Coal is one of the most abundant, widely distributed, and economical energy resources in the world. Globally, coal-based energy is a major power source, especially in some developing countries. Power plants generate about 600–800 million tons of fly ash every year, and previous estimates indicated that 25%–60% total ash was derived from coal burning (Asokan et al., 2005; Jayaranjan et al., 2014; Kizgut et al., 2010; Wang et al., 2005). China is one of the leading countries in coal production and consumption, resulting in an annual coal-derived fly ash of 150 million tons (Cao et al., 2008). Coal fly ash is commonly considered as “non-hazardous” waste, and it is easily disposed in ash dumps either by using the wet or dry method (Lopez-Anton et al., 2007). Nowadays, coal fly ash has been reused and applied as a base of heterogenous catalysts in chemical synthesis because of its special characteristics (Hadiyanto et al., 2016; Jayaranjan et al., 2014). However, coal fly ashes usually contain heavy metals and even polycyclic aromatic hydrocarbons (PAHs) (Popovic et al., 2001). Previous studies had verified that coal fly ash was a source of metal particles in the atmosphere (Chen et al., 2012; Li et al., 2017) and can enhanced ice nucleation activity (Umo et al., 2019). Furthermore, the trace heavy metals from fly ash can be leached out under acidic conditions and can contaminate the surrounding soils, surface water, and ground water sources. Such contamination of heavy metals can pollute the environment and even enter the food chain leading to genotoxic effects on DNA (Chakraborty and Mukherjee, 2009). Therefore, coal fly ash effects to the environment and human health can be long-lasting since large amount of proportion emitted into the atmosphere and remained in the environment for a long time (Twardowska and Szczepanska, 2002). Large quantity of coal fly ash means considerable reactive surface that could mediate heterogeneous reactions between different trace gases and subsequently influence the balance of atmospheric species, such as mineral dust (Tang et al., 2018).
Hydrogen peroxide (H2O2) is a stable oxidant in the atmosphere and plays important roles in the oxidation chemistry of both liquid and aerosol phases (Liu et al., 2020; Xuan et al., 2020). Gas phase H2O2 has been observed by many field measurements (Bales et al., 1995; Jacobi et al., 2002; Hua et al., 2008; Wang et al., 2016; Qin et al., 2018; Ye et al., 2018; Ye et al., 2021). In particular, the mixing ratios of H2O2 in the atmosphere was observed to be 0.9 ppbv during moderately polluted periods (Ye et al., 2018) and can reach up to 11.3 ppbv in China at summer time (Wang et al., 2014). A Box model simulation based on known gas phase chemical mechanisms and kinetics has found the modeling results could only well reproduce the daily variation trend of H2O2, but both the H2O2 peak values in the daytime and minimal values at night were significantly overestimated comparing with field observation (H2O2 modeled/H2O2 measured = 1.8), indicating that the sink for H2O2 might be largely underestimated (Ye et al., 2021). Field studies have also shown that ambient aerosol reactions through heterogeneous processes may be an important sink for gaseous H2O2 (de Reus et al., 2005; He et al., 2010; Ye et al., 2018). Therefore, the quantitative experimental data for the removal of H2O2 through heterogeneous reactions are needed to improve the parameters used for model simulation and help to reduce the discrepancies between fields measured and modeled H2O2 concentration. Several laboratory studies have investigated the kinetics and mechanisms of H2O2 interactions with mineral aerosol surfaces (Pradhan et al., 2010a; Pradhan et al., 2010b; Wang et al., 2011; Zhao et al., 2011; Romanias et al., 2012; 2013; Zhou et al., 2012; El Zein et al., 2013; Zhao et al., 2013; El Zein et al., 2014; Wu et al., 2015; Zhou et al., 2016). In addition, mineral aerosols led to faster SO2 oxidation through heterogenic reaction on the surface of mineral aerosols to produce sulfate (Huang et al., 2014) and sulfate formation through manganese-catalyzed oxidation of SO2 on aerosol surfaces has been established (Wang et al., 2021). Meanwhile, the heterogeneous oxidation of sulfur dioxide on mineral oxide in the presence of hydrogen peroxide showed the importance for understanding the role of H2O2 as part of heterogeneous reaction of SO2 and sulfate formation in the atmosphere (Huang et al., 2016; Ye et al., 2018). Hydrogen peroxide is also an aqueous phase oxidant of S(IV) to S(VI) (i.e., sulfite to sulfate) and plays an important role in the acidification of cloud and rain. Studies on SO2 + H2O2 reaction have been conducted on aqueous surfaces in liquid clouds (Caffrey et al., 2001; Clegg and Abbatt, 2001) and even deliquesced aerosol particles (Liu et al., 2020). Meanwhile, the uptake of SO2 on mineral oxides and the catalytic oxidation process from S(IV) to S(VI) in metal-containing aqueous have also been investigated (Gankanda et al., 2016; Maters et al., 2017). The uptake of gas-phase SO2 and H2O2 by ice surfaces and mineral oxide (alpha-Al2O3) have been well documented (Clegg and Abbatt, 2001; Huang et al., 2016). However, the understanding of the synergy between hydrogen peroxide and sulfur dioxide on solid surface is limited.
Coal fly ash usually comes from the same as some common gas pollutants (such as SO2 and CO), the heterogeneous reaction of gas-phase pollutants and reactive species on coal fly ash may be important in atmospheric processing. Many studies have verified that heterogeneous reactions were vital pathways for pollutants transformation and secondary aerosol formation. Yet, research on heterogenous reactions on coal fly ash were quite limited comparing to mineral dust and most of the previous work was concerned with single reactant and performed at room temperature. In this study, the quantitative experimental data on the removal of H2O2 and SO2 due to heterogeneous reactions on coal fly ash were derived. Furthermore, assessment of the temperature effects from these reactions was carried out because temperature effect is an important part of atmosphere science. This work presents a detailed investigation on the uptake coefficients of SO2 and H2O2 on coal fly ash in the temperature range of 253 K–313 K, which represents the general atmospheric thermal environment. The temperature dependence and atmospheric implication of these reactions were also discussed in the following sections. Meanwhile, we also explored synergistic reaction mechanism of H2O2 and SO2 on coal fly ash as a function of relative concentration of H2O2 heterogenous reaction on secondary aerosol formation in dry condition. The results would provide useful information for understanding the mechanism of uptake and decomposition of H2O2 and SO2 on coal fly ash as both processes are critical properties for evaluating aerosol’s environmental and climate impacts. There is still a considerably large gap between laboratory work and modeling studies used to explain field measurements and predict future changes (Tang et al., 2017), the experimentally determined uptake coefficients from this work will enhances the understanding of field measurements and improve predictions on future changes (Qin et al., 2018).
Materials and Methods
Materials
This experiment used coal fly ash generated from coal-fired power plant without further treatment. The surface area of the powder sample was measured by using the multipoint Brunauer–Emmett–Teller (BET) analysis method with a BET apparatus (autosorb-iQ, Quantachrome Instruments, United States). The measured surface area was 1.796 m2g−1. X-ray diffraction (XRD) experiment for this powder sample was performed via a Rigaku D/max-2500 diffractometer (Japan) with Cu–K radiation at 50 kV and 100 mA. Scans from 2.6° to 50° (2θ) at a rate of 1° (2θ) per minute were carried out. The obtained XRD pattern was processed using JADE software and is shown in Supplementary Figure S1. The major peaks were indexed to permit recognition of the coal fly ash, and the results showed that the main component of this coal fly ash was aluminosilicate (mullite and corundum), aluminum, and silicon were the main component elements, such as the results derived by Koukouzas et al. (2006); Skvara et al. (2009). Figure 1 displays the scanning electron micrograph (S-4800, Hitachi, Japan) of this coal fly ash. The particle size distribution of this coal fly ash was between 5 and 100 μm in the coarse particle mode.
The gaseous sulfur dioxide (99.9%, Beijing Huayuan Gas Chemical Industry Co., Ltd.,) was used as reactant gas without further purification. The high weight percentage hydrogen peroxide solution (∼95 wt%) was produced by rotary evaporating aqueous solutions of hydrogen peroxide (35 wt%, Alfa Aesar, China) and subsequently concentrated by bubbling dry nitrogen. The weight concentration of purified H2O2 was greater than 95% as described by Zhou et al. (2012)
Experimental System and Method for Determining Uptake Coefficient
The uptake coefficients of H2O2 and SO2 on coal fly ash samples were carried out by using a Knudsen cell system combining with an electron ionization (EI) quadrupole mass spectrometer (QMS, Hiden, HAL 3F 501, United Kingdom), as illustrated in Figure 2. The experimental set up and the procedure used has been described in detail in previous publications (Wang et al., 2011; Zhou et al., 2012). In brief, the reactor of the Knudsen cell system consists of a chamber with four isolated sample compartments for parallel experiments and a small escape aperture linking to the QMS detector. To ensure that the mean free path of the molecules exceeded the dimensions of the Knudsen cell, the pressure was maintained low in the reaction chamber (∼10–3 Pa). A vacuum gauge was applied to monitor the pressure in the chamber. Before the reactant gas was injected into the chamber, the pressure was kept stable and the pressure variation due to adding gas was used to calculate the partial pressure of reactant in the chamber. Prior to the experiments, the reactor chamber was passivated with reactant gases for more than 30 min until a steady state of the QMS signal was established, while the coal fly ash samples were isolated from the gas by the sample cover.
The EI-QMS was used to detect reactants and products of these heterogeneous reactions. The measured mass charge ratio (m/z) signals varied linearly with the concentrations of the compounds. The specific masses monitored to detect H2O2 and SO2 on coal fly ash samples were m/z = 34 (H2O2+) and 64 (SO2+), respectively. A blank experiment was performed first to make sure the sample holders and covers were passivated by the gas phase reactants passing through the reactor. No obvious reactants signals consume were observed during blank experiments. The powder samples with known masses were evenly tiled on the bottom of the holder by dispersing with deionized water and drying first. For the temperature effect experiments, a circulator was applied to adjust the temperature from 253 to 313 K for the Teflon-coated metal sample holders and for the chamber. During each experiment, the temperature variability was maintained within 1 K.
The Knudsen cell heterogeneous kinetics experiments were performed following our previous works (Zhou et al., 2014; Zhou et al., 2016). For all the kinetic experiments, the temperature of reaction chamber was kept at a stable value first, and the samples were kept isolated from the gas reagent. Then, the system was passivated with gas reactant until the signal of gas reactant reached a steady state. After that, one sample was exposed to gas reactant by quickly opening the holder cover. We found the signal of reactant parent ion monitored at m/z = 34 (H2O2+) or 64 (SO2+) dropped below its original value suddenly. An observed uptake coefficient, γobs, can be derived from the following equation (Usher et al., 2002):
Here, Ah represents the effective area of the escape hole between the Knudsen cell chamber and the QMS detector; in this study, Ah is 0.211 mm2; As represents the geometric area of the sample holder, which is 5.3 cm2 in our system; I0 and I are the signal intensities detected by QMS when sample holder was covered and exposed. The parameters of this system could be found in our previous work (Zhou et al., 2016) and also summarized in Supplementary Table S1.
Results and Discussion
Kinetics of Hydrogen Peroxide and Sulfur Dioxide Uptake on Coal Fly Ash at Room Temperature (298 K)
In this work, the measurements of initial and steady state uptake coefficients at 298 K for H2O2 and SO2 on coal fly ash were conducted separately.
During the experiments, we found that H2O2 was first taken up on the surface of the fly ash, and after a certain period, the reactant signal (H2O2+, m/z = 34) became balanced and a steady state uptake process could be measured. The uptake phenomenon of SO2 on the surface of coal fly ash was a slightly different from those of H2O2: only the initial uptake was obvious, while the steady state uptake was quite small (<8 × 10−5); this steady state uptake was similar to that of Chinese Inner Mongolia desert dust, which was <5 × 10−5 (Zhou et al., 2014). Besides each gas phase reactant, some possible products, including O2 (m/z = 32), CO2 (m/z = 44). were also monitored by QMS synchronously, while there was no remarkable undulation during the uptake experiments such as catalytic oxidation reactions.
According to Eq. 1, uptake coefficients were derived by assuming the gas-surface collision only on the top layer of the solid reactants. To test if all the surface area of coal fly ash samples was involved in the uptake processes, the uptake coefficients γobs were calculated using the geometric surface area of the sample versus a series of sample masses. As illustrated in Figure 3, the observed uptake values grew linearly with increasing sample mass, which indicated that reactions were not only on the top layer of the sample surface, but the total surface areas of particles could also participate in the reactions. The observed mass dependence phenomena supported that the diffusion of the reactants can go through the underlying layers of these samples. In this work, the experiments were carried out by using the sample masses in the linear range to make sure the entire surface areas of the coal fly ash were accessible to the reactants (H2O2 and SO2). BET surface areas of each sample were treated as the entire sample participates during the reactions (Underwood et al., 2000).
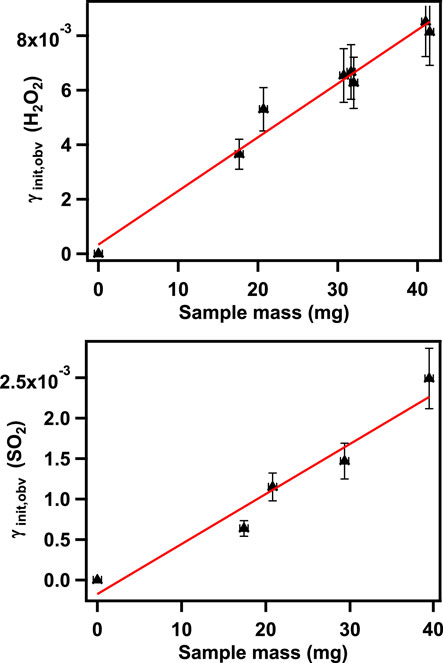
FIGURE 3. Linear mass-dependent regions of the observed uptake coefficient for H2O2 (upper panel) and SO2 (lower panel) on coal fly ash.
The plots in Figure 3 show the region where the observed uptake coefficients (γobs) are linearly dependent on the mass of samples. From the plot, a mass independent uptake coefficient can be derived as follows:
Here, ABET represents the surface area of the coal fly ash powder sample, the BET area of the powder times the sample mass is taken to calculate this value. The γBET values obtained from each experiment are given in Table 1 (298 K).
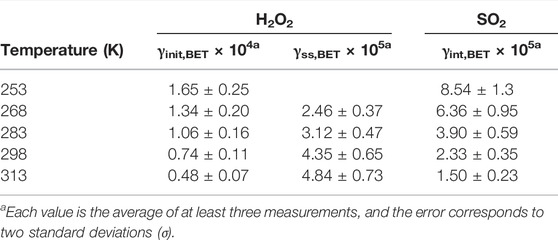
TABLE 1. Summary of the uptake coefficients of H2O2 and SO2 on coal fly ash at different temperatures.
Because the uptake processes on aerosol surfaces might be subjected to the pressure, namely, the mixing ratios of reactants, influence from H2O2/SO2 mixing ratio to the uptake coefficient was considered in this work by varying the initial mixing ratio of H2O2/SO2 from 10 to 60 ppbv, respectively. The uptake experiments of H2O2/SO2 on coal fly ash showed similar phenomena. This means that there was limited dependence of initial uptake coefficient on different mixing ratios of H2O2 or SO2 in this range.
The lack of dependence on mixing ratio observed in this study were similar with previous work conducted on mineral dust samples (El Zein et al., 2014; Zhou et al., 2016). Therefore, according to the results obtained from the experimental measurements, the H2O2 heterogeneous uptake on coal fly ash was assumed to be passing through physical adsorption and heterogeneous reactions, while SO2 heterogeneous uptake on coal fly ash was through physical adsorption at room temperature.
Synergistic Effects of the H2O2 and SO2 Uptake Process on Coal Fly Ash
In this study, we first measured the uptake coefficient of H2O2 on coal fly ash samples with a constant mixing ratio of H2O2 and SO2 mixing ratio of 0–52.8 ppbv. The initial uptake coefficients of H2O2 showed little difference between measuring with only H2O2 and with both the reactive gases (H2O2 and SO2) present (The relative deviation was less than 10%.). This phenomenon likely since the physisorption rate was much faster than the rate of chemical transformation. The main processes of initial uptake were only related to physisorption independent of the active sites for adsorption seemed to be independent. As illustrated in Figure 4, no apparent growth in steady state uptake coefficients of hydrogen peroxide on coal fly was shown with increasing of SO2 mixing rations in the reaction chamber. It was expected that on these coal fly ash samples, SO2 did not influent the reactive site for H2O2 uptake regularly. Further investigation would need to evaluate the effects of SO2 on coal fly ash. We next measured the uptake coefficient of SO2 on coal fly ash samples when the mixing ratio of SO2 was kept constant and the H2O2 mixing ratio ranged from 0 ppbv to around 40 ppbv. The initial uptake coefficients of SO2 were independent of the H2O2 mixing ratio. As mentioned above, this process was also dominated by the physisorption. However, hydrogen peroxide can easily increase the measured steady state uptake coefficients of SO2, as shown in Figure 5. It was implied that the absorbed hydrogen peroxide can provide a new reactive surface for SO2 uptake.
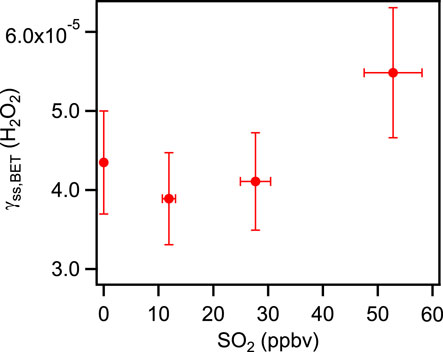
FIGURE 4. Dependence of the steady state uptake coefficients of H2O2 on different mixing ratios of SO2.
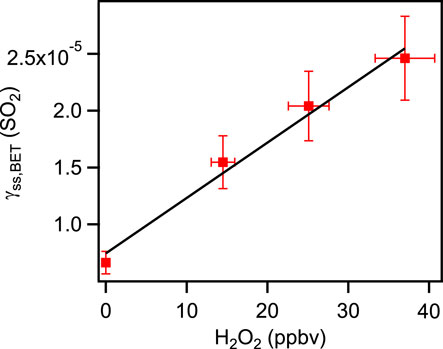
FIGURE 5. Dependence of the steady state uptake coefficients of SO2 on different mixing ratios of H2O2.
In this study, although Knudsen cell reactor was kept in a low pressure, the experiments were performed in an almost dry condition, there would still be some adsorbed water present on the surface of the coal fly ash samples. The adsorbed water present on the surface could be in the form of O2- or OH as water-related-reactive sites, but could not been quantified. The sulfate or bisulfate formation on the coal fly ash should be consistent with the reaction of gas-phase SO2 with reactive surface (Usher et al., 2002).
In previous study, the oxidation of SO2 through uptake process was proposed a two steps mechanism. The first step was a reversible adsorption of SO2 onto the surface, and the followed step was an irreversible reaction by which adsorbed SO2 was oxidized to be sulfate (Ullerstam et al., 2002). So, the H2O2 may have the reactivity to oxidize adsorbed SO2 on the surface of coal fly ash samples via the following reactions:
Our result suggested that H2O2 uptake affects mineral surface-active site and promotes the further reactions.
Temperature Effects on Uptake Kinetics of Hydrogen Peroxide and Sulfur Dioxide on Coal Fly Ash
The temperature dependence of the uptake coefficients for heterogeneous reactions on coal fly ash was investigated for the temperature ranging from 253K to 313 K with this temperature-controllable Knudsen cell system. The results are presented in Table 1.
The experimental results showed that the initial uptake coefficients of gas phase H2O2 and SO2 on coal fly ash decreased evidently with increasing temperature. This trend of the initial uptake coefficients with temperature well match with the physisorption principle (Zhou et al., 2012). A similar temperature effect of initial uptake process had also been derived on some real mineral dust and typical mineral oxides (Zhou et al., 2012; Romanias et al., 2013; El Zein et al., 2014; Zhou et al., 2016). The steady state uptake coefficients of H2O2 on coal fly ash samples performed contrary phenomena. The γss,BET values increased with the temperature increasing. At 253 K, the uptake phenomenon was too weak and generated large error for the steady state uptake coefficient calculation, so this value was not given in this work. The main component of coal fly ash was aluminosilicate. In our previous work, the interactions of H2O2 with Arizona test dust, Inner Mongolia desert dust and Xinjiang sierozem over the temperature range from 268 to 320 K have been investigated (Zhou et al., 2016). The certified chemical compositions of these mineral dust were also SiO2 and Al2O3, but the temperature effects of their steady state uptake coefficients on mineral dust samples showed different phenomena, which may relate to the differences of their trace species. Therefore, the temperature dependence of steady state uptake coefficients indicating that the structure and the component of the solid samples may impact the uptake processes.
Now that the initial uptake coefficients showed negative correlation to the temperature, the changes of enthalpy (ΔHobs) and entropy (ΔSobs) for H2O2 and SO2 adsorption on coal fly ash and the reactive energy (Ea) for H2O2 uptake on coal fly ash could be derived from the following Eqs 3, 4 (Jayne et al., 1990):
From the plot on the left side of Eq. 3 versus inverse temperature, as shown in Figures 6, 7, the enthalpy (ΔHobs) and entropy (ΔSobs) were determined to be −(13.27 ± 1.62) KJ mol−1 and −(124.0 ± 5.8) J K mol−1 for H2O2 initial uptake process; −(19.57 ± 1.56) KJ mol−1 and −(154.3 ± 5.6) J K mol−1 for SO2 initial uptake process. The reactive energy (Ea) for H2O2 uptake on coal fly ash was (11.01 ± 1.36) KJ mol−1. Normally, the activation energy of a reaction exit in the atmosphere is larger than 20 KJ mol−1, the importance of this reaction is negligible (Smith, 2003; Liu et al., 2008). This reveals that the heterogeneous uptake processes of H2O2 on coal fly ash are significant and should not be neglected.
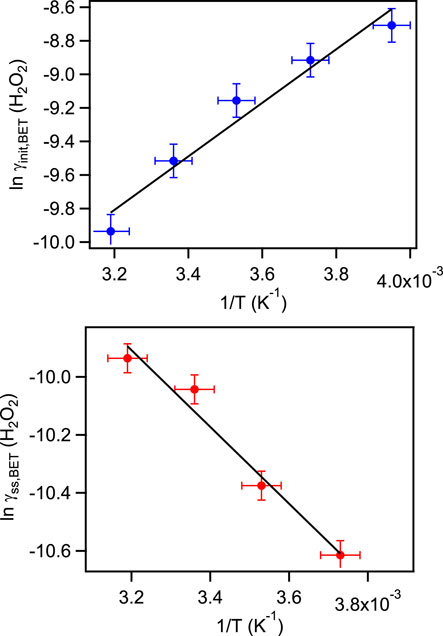
FIGURE 6. Relationship between the initial uptake coefficients (upper panel) and steady state uptake coefficients (lower panel) of sulfur dioxide on coal fly and temperature.
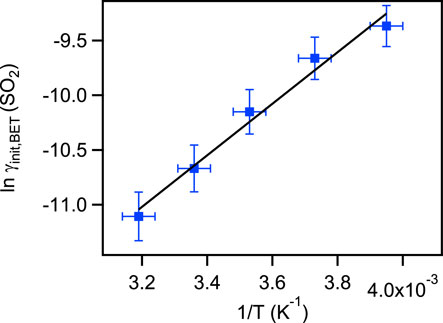
FIGURE 7. Relationship between the initial uptake coefficients of sulfur dioxide on two coal fly and temperature.
The mechanism of H2O2 uptake on an oxide surface at low relative humidity (RH) condition are presented as below (Lin and Gurol, 1998; Pradhan et al., 2010a; Zhao et al., 2011). Herein, S represents the surface of solid sample.
Based on the study results, we could suggest that the initial uptake coefficients of H2O2 and SO2 on coal fly ash are analogous to the other mineral aerosols, which can provide active surface for sinking of H2O2 and SO2, especially at low temperature.
Conclusion and Atmospheric Implication
Coal fly ash is as an important anthropogenic mineral aerosol because it exits in large quantity and it has critical impact on air contamination and climate changes in the atmosphere. It is a crucial component of particulate matter, which can provide reactive surfaces for sinking different kinds of chemical species in the troposphere. In this work, heterogeneous uptakes of H2O2 and SO2 on coal fly ash sample in the temperature range from 253 K to 313 K were performed in Knudsen cell reactor coupled with QMS detector. The reactant concentration used in this work was close to ambient levels. The uptake coefficients of H2O2 and SO2 on coal fly ash showed little dependence on the mixing ratio of H2O2 and SO2 from 10 to 60 ppbv. The initial uptake coefficients of H2O2 and SO2 are temperature dependence, the values decreased with the increasing of temperature for these uptake processes. When the reaction temperature increased, the steady state uptake coefficients of H2O2 increased and the gap between steady state uptake coefficients and initial uptake coefficients became smaller. Based on the temperature dependence of these uptake coefficients, the enthalpy (ΔHobs), entropy (ΔSobs) and reactive energy (Ea) for coal fly ash had also been calculated.
In this work, the synergistic effects of between SO2 and H2O2 in their reaction on coal fly ash were investigated. We noted that the existence of gas phase H2O2 could increase the ability for SO2 uptake on coal fly ash, suggesting that the gaseous H2O2 could potentially improve sulfate formation in atmosphere gas phase through heterogeneous reactions. Our result suggested that the interaction between coal fly ash and H2O2 can dramatically influence the mixing ratio of H2O2 in the atmosphere and the effects of oxidizing SO2 by gaseous H2O2 on coal fly ash should also be considered in future atmospheric studies.
Data Availability Statement
The original contributions presented in the study are included in the article/Supplementary Material; further inquiries can be directed to the corresponding author.
Author Contributions
LZ performed the research, analyzed data, and wrote the manuscript; DK performed XRD experiments and revised the manuscript; TL and YZ contributed coal fly ash and revised the manuscript; YG, MG and FY revised the manuscript; WW designed the research and revised the manuscript.
Funding
This work was supported financially by the National Natural Science Foundation of China (Grant Nos. 42175133, 42175124, 41875162, 91844301, and 21806169).
Conflict of Interest
The authors declare that the research was conducted in the absence of any commercial or financial relationships that could be construed as a potential conflict of interest.
Publisher’s Note
All claims expressed in this article are solely those of the authors and do not necessarily represent those of their affiliated organizations, or those of the publisher, the editors, and the reviewers. Any product that may be evaluated in this article, or claim that may be made by its manufacturer, is not guaranteed or endorsed by the publisher.
Supplementary Material
The Supplementary Material for this article can be found online at: https://www.frontiersin.org/articles/10.3389/fenvs.2022.876289/full#supplementary-material
References
Asokan, P., Saxena, M., and Asolekar, S. R. (2005). Coal Combustion Residues-Environmental Implications and Recycling Potentials. Resour. Conservation Recycl. 43 (3), 239–262. doi:10.1016/j.resconrec.2004.06.003
Bales, R. C., Losleben, M. V., McConnell, J. R., Fuhrer, K., and Neftel, A. (1995). H2O2 in Snow, Air and Open Pore Space in Firn at Summit, Greenland. Geophys. Res. Lett. 22 (10), 1261–1264. doi:10.1029/95gl01110
Caffrey, P., Hoppel, W., Frick, G., Pasternack, L., Fitzgerald, J., Hegg, D., et al. (2001). In-cloud Oxidation of SO2 by O-3 and H2O2: Cloud Chamber Measurements and Modeling of Particle Growth. J. Geophys. Res. 106 (D21), 27587–27601. doi:10.1029/2000jd900844
Cao, D.-z., Selic, E., and Herbell, J.-D. (2008). Utilization of Fly Ash from Coal-Fired Power Plants in China. J. Zhejiang Univ. Sci. A 9 (5), 681–687. doi:10.1631/jzus.A072163
Chakraborty, R., and Mukherjee, A. (2009). Mutagenicity and Genotoxicity of Coal Fly Ash Water Leachate. Ecotoxicol. Environ. Saf. 72 (3), 838–842. doi:10.1016/j.ecoenv.2008.09.023
Chen, H., Laskin, A., Baltrusaitis, J., Gorski, C. A., Scherer, M. M., and Grassian, V. H. (2012). Coal Fly Ash as a Source of Iron in Atmospheric Dust. Environ. Sci. Technol. 46 (4), 2112–2120. doi:10.1021/es204102f
Clegg, S. M., and Abbatt, J. P. D. (2001). Oxidation of SO2 by H2O2 on Ice Surfaces at 228 K: A Sink for SO2 in Ice Clouds. Atmos. Chem. Phys. 1, 73–78. doi:10.5194/acp-1-73-2001
de Reus, M., Fischer, H., Sander, R., Gros, V., Kormann, R., Salisbury, G., et al. (2005). Observations and Model Calculations of Trace Gas Scavenging in a Dense Saharan Dust Plume during MINATROC. Atmos. Chem. Phys. 5, 1787–1803. doi:10.5194/acp-5-1787-2005
El Zein, A., Bedjanian, Y., and Romanias, M. N. (2013). Kinetics and Products of HONO Interaction with TiO2 Surface Under UV Irradiation. Atmos. Environ. 67, 203–210. doi:10.1016/j.atmosenv.2012.11.016
Gankanda, A., Coddens, E. M., Zhang, Y., Cwiertny, D. M., and Grassian, V. H. (2016). Sulfate Formation Catalyzed by Coal Fly Ash, Mineral Dust and Iron(III) Oxide: Variable Influence of Temperature and Light. Environ. Sci. Process. Impacts. 18 (12), 1484–1491. doi:10.1039/c6em00430j
Hadiyanto, H., Lestari, S. P., Abdullah, A., Widayat, W., and Sutanto, H. (2016). The Development of Fly Ash-Supported CaO Derived from Mollusk Shell of Anadara Granosa and Paphia Undulata as Heterogeneous CaO Catalyst in Biodiesel Synthesis. Int. J. Energy Environ. Eng. 7 (3), 297–305. doi:10.1007/s40095-016-0212-6
He, S. Z., Chen, Z. M., Zhang, X., Zhao, Y., Huang, D. M., Zhao, J. N., et al. (2010). Measurement of Atmospheric Hydrogen Peroxide and Organic Peroxides in Beijing before and during the 2008 Olympic Games: Chemical and Physical Factors Influencing Their Concentrations. J. Geophys. Res. 115, D17307. doi:10.1029/2009jd013544
Hua, W., Chen, Z. M., Jie, C. Y., Kondo, Y., Hofzumahaus, A., Takegawa, N., et al. (2008). Atmospheric Hydrogen Peroxide and Organic Hydroperoxides during PRIDE-PRD'06, China: Their Concentration, Formation Mechanism and Contribution to Secondary Aerosols. Atmos. Chem. Phys. 8 (22), 6755–6773. doi:10.5194/acp-8-6755-2008
Huang, L., Zhao, Y., Li, H., and Chen, Z. (2016). Hydrogen Peroxide Maintains the Heterogeneous Reaction of Sulfur Dioxide on Mineral Dust Proxy Particles. Atmos. Environ. 141, 552–559. doi:10.1016/j.atmosenv.2016.07.035
Huang, X., Song, Y., Zhao, C., Li, M., Zhu, T., Zhang, Q., et al. (2014). Pathways of Sulfate Enhancement by Natural and Anthropogenic Mineral Aerosols in China. J. Geophys. Res. Atmos. 119 (24), 14165–14179. doi:10.1002/2014jd022301
Jacobi, H. W., Frey, M. M., Hutterli, M. A., Bales, R. C., Schrems, O., Cullen, N. J., et al. (2002). Measurements of Hydrogen Peroxide and Formaldehyde Exchange between the Atmosphere and Surface Snow at Summit, Greenland. Atmos. Environ. 36 (15-16), 2619–2628. doi:10.1016/s1352-2310(02)00106-1
Jayaranjan, M. L. D., van Hullebusch, E. D., and Annachhatre, A. P. (2014). Reuse Options for Coal Fired Power Plant Bottom Ash and Fly Ash. Rev. Environ. Sci. Biotechnol. 13 (4), 467–486. doi:10.1007/s11157-014-9336-4
Jayne, J. T., Gardner, J. A., Davidovits, P., Worsnop, D. R., Zahniser, M. S., and Kolb, C. E. (1990). The Effect of H2O2 Content on the Uptake of SO2(g) by Aqueous Droplets. J. Geophys. Res. 95 (D12), 20559–20563. doi:10.1029/JD095iD12p20559
Kizgut, S., Cuhadaroglu, D., and Samanli, S. (2010). Stirred Grinding of Coal Bottom Ash to Be Evaluated as a Cement Additive. Energy Sources, Part A Recovery, Util. Environ. Eff. 32 (16), 1529–1539. doi:10.1080/15567030902780378
Koukouzas, N., Zeng, R., Perdikatsis, V., Xu, W., and Kakaras, E. (2006). Mineralogy and Geochemistry of Greek and Chinese Coal Fly Ash. Fuel 85 (16), 2301–2309. doi:10.1016/j.fuel.2006.02.019
Li, R., Yang, X., Fu, H., Hu, Q., Zhang, L., and Chen, J. (2017). Characterization of Typical Metal Particles during Haze Episodes in Shanghai, China. Chemosphere 181, 259–269. doi:10.1016/j.chemosphere.2017.03.140
Lin, S.-S., and Gurol, M. D. (1998). Catalytic Decomposition of Hydrogen Peroxide on Iron Oxide: Kinetics, Mechanism, and Implications. Environ. Sci. Technol. 32 (10), 1417–1423. doi:10.1021/es970648k
Liu, T., Clegg, S. L., and Abbatt, J. P. D. (2020). Fast Oxidation of Sulfur Dioxide by Hydrogen Peroxide in Deliquesced Aerosol Particles. Proc. Natl. Acad. Sci. U.S.A. 117 (3), 1354–1359. doi:10.1073/pnas.1916401117
Liu, Y., Gibson, E. R., Cain, J. P., Wang, H., Grassian, V. H., and Laskin, A. (2008). Kinetics of Heterogeneous Reaction of CaCO3 Particles with Gaseous HNO3 over a Wide Range of Humidity. J. Phys. Chem. A 112 (7), 1561–1571. doi:10.1021/jp076169h
López-Antón, M. A., Díaz-Somoano, M., Fierro, J. L. G., and Martínez-Tarazona, M. R. (2007). Retention of Arsenic and Selenium Compounds Present in Coal Combustion and Gasification Flue Gases Using Activated Carbons. Fuel Process. Technol. 88 (8), 799–805. doi:10.1016/j.fuproc.2007.03.005
Maters, E. C., Delmelle, P., Rossi, M. J., and Ayris, P. M. (2017). Reactive Uptake of Sulfur Dioxide and Ozone on Volcanic Glass and Ash at Ambient Temperature. J. Geophys. Res. Atmos. 122 (18), 10077–10088. doi:10.1002/2017JD026993
Popovic, A., Djordjevic, D., and Polic, P. (2001). Trace and Major Element Pollution Originating from Coal Ash Suspension and Transport Processes. Environ. Int. 26 (4), 251–255. doi:10.1016/S0160-4120(00)00114-8
Pradhan, M., Kalberer, M., Griffiths, P. T., Braban, C. F., Pope, F. D., Cox, R. A., et al. (2010b). Uptake of Gaseous Hydrogen Peroxide by Submicrometer Titanium Dioxide Aerosol as a Function of Relative Humidity. Environ. Sci. Technol. 44 (4), 1360–1365. doi:10.1021/es902916f
Pradhan, M., Kyriakou, G., Archibald, A. T., Papageorgiou, A. C., Kalberer, M., and Lambert, R. M. (2010a). Heterogeneous Uptake of Gaseous Hydrogen Peroxide by Gobi and Saharan Dust Aerosols: A Potential Missing Sink for H2O2 in the Troposphere. Atmos. Chem. Phys. 10 (15), 7127–7136. doi:10.5194/acp-10-7127-2010
Qin, M., Chen, Z., Shen, H., Li, H., Wu, H., and Wang, Y. (2018). Impacts of Heterogeneous Reactions to Atmospheric Peroxides: Observations and Budget Analysis Study. Atmos. Environ. 183, 144–153. doi:10.1016/j.atmosenv.2018.04.005
Romanias, M. N., El Zein, A., and Bedjanian, Y. (2012). Heterogeneous Interaction of H2O2 with TiO2 Surface under Dark and UV Light Irradiation Conditions. J. Phys. Chem. A 116 (31), 8191–8200. doi:10.1021/jp305366v
Romanias, M. N., El Zein, A., and Bedjanian, Y. (2013). Uptake of Hydrogen Peroxide on the Surface of Al2O3 and Fe2O3. Atmos. Environ. 77, 1–8. doi:10.1016/j.atmosenv.2013.04.065
Škvára, F., Kopecký, L., Šmilauer, V., and Bittnar, Z. (2009). Material and Structural Characterization of Alkali Activated Low-Calcium Brown Coal Fly Ash. J. Hazard. Mater. 168 (2-3), 711–720. doi:10.1016/j.jhazmat.2009.02.089
Smith, I. W. M. (2003). Laboratory Studies of Atmospheric Reactions at Low Temperatures. Chem. Rev. 103 (12), 4549–4564. doi:10.1021/cr020512r
Tang, M., Huang, X., Lu, K., Ge, M., Li, Y., Cheng, P., et al. (2017). Heterogeneous Reactions of Mineral Dust Aerosol: Implications for Tropospheric Oxidation Capacity. Atmos. Chem. Phys. 17 (19), 11727–11777. doi:10.5194/acp-17-11727-2017
Tang, S., Ma, L., Luo, M., Zhang, Z., Cao, X., Huang, Z., et al. (2018). Heterogeneous Reaction of Cl2 and NO2 on γ-Al2O3: A Potential Formation Pathway of Secondary Aerosols. Atmos. Environ. 188, 25–33. doi:10.1016/j.atmosenv.2018.06.005
Twardowska, I., and Szczepanska, J. (2002). Solid Waste: Terminological and Long-Term Environmental Risk Assessment Problems Exemplified in a Power Plant Fly Ash Study. Sci. Total Environ. 285 (1-3), 29–51. doi:10.1016/s0048-9697(01)00893-2
Ullerstam, M., Vogt, R., Langer, S., and Ljungström, E. (2002). The Kinetics and Mechanism of SO2 Oxidation by O3 on Mineral Dust. Phys. Chem. Chem. Phys. 4 (19), 4694–4699. doi:10.1039/b203529b
Umo, N. S., Wagner, R., Ullrich, R., Kiselev, A., Saathoff, H., Weidler, P. G., et al. (2019). Enhanced Ice Nucleation Activity of Coal Fly Ash Aerosol Particles Initiated by Ice-Filled Pores. Atmos. Chem. Phys. 19 (13), 8783–8800. doi:10.5194/acp-19-8783-2019
Underwood, G. M., Li, P., Usher, C. R., and Grassian, V. H. (2000). Determining Accurate Kinetic Parameters of Potentially Important Heterogeneous Atmospheric Reactions on Solid Particle Surfaces with a Knudsen Cell Reactor. J. Phys. Chem. A 104 (4), 819–829. doi:10.1021/jp9930292
Usher, C. R., Al-Hosney, H., Carlos-Cuellar, S., and Grassian, V. H. (2002). A Laboratory Study of the Heterogeneous Uptake and Oxidation of Sulfur Dioxide on Mineral Dust Particles. J.-Geophys.-Res. 107 (D23), 4713. doi:10.1029/2002jd002051
Wang, S., Boyjoo, Y., Choueib, A., and Zhu, Z. H. (2005). Removal of Dyes from Aqueous Solution Using Fly Ash and Red Mud. Water Res. 39 (1), 129–138. doi:10.1016/j.watres.2004.09.011
Wang, W.-g., Ge, M.-f., and Sun, Q. (2011). Heterogeneous Uptake of Hydrogen Peroxide on Mineral Oxides. Chin. J. Chem. Phys. 24 (5), 515–520. doi:10.1088/1674-0068/24/05/515-520
Wang, W., Liu, M., Wang, T., Song, Y., Zhou, L., Cao, J., et al. (2021). Sulfate Formation Is Dominated by Manganese-Catalyzed Oxidation of SO2 on Aerosol Surfaces during Haze Events. Nat. Commun. 12 (1), 1993. doi:10.1038/s41467-021-22091-6
Wang, Y., Chen, Z., Wu, Q., Liang, H., Huang, L., Li, H., et al. (2016). Observation of Atmospheric Peroxides during Wangdu Campaign 2014 at a Rural Site in the North China Plain. Atmos. Chem. Phys. 16 (17), 10985–11000. doi:10.5194/acp-16-10985-2016
Wang, Y., Zhang, Q., Jiang, J., Zhou, W., Wang, B., He, K., et al. (2014). Enhanced Sulfate Formation during China's Severe Winter Haze Episode in January 2013 Missing from Current Models. J. Geophys. Res. Atmos. 119 (17), 10425–10440. doi:10.1002/2013jd021426
Wu, Q. Q., Huang, L. B., Liang, H., Zhao, Y., Huang, D., and Chen, Z. M. (2015). Heterogeneous Reaction of Peroxyacetic Acid and Hydrogen Peroxide on Ambient Aerosol Particles under Dry and Humid Conditions: Kinetics, Mechanism and Implications. Atmos. Chem. Phys. 15 (12), 6851–6866. doi:10.5194/acp-15-6851-2015
Xuan, X., Chen, Z., Gong, Y., Shen, H., and Chen, S. (2020). Partitioning of Hydrogen Peroxide in Gas-Liquid and Gas-Aerosol Phases. Atmos. Chem. Phys. 20 (9), 5513–5526. doi:10.5194/acp-20-5513-2020
Ye, C., Liu, P., Ma, Z., Xue, C., Zhang, C., Zhang, Y., et al. (2018). High H2O2 Concentrations Observed during Haze Periods during the Winter in Beijing: Importance of H2O2 Oxidation in Sulfate Formation. Environ. Sci. Technol. Lett. 5, 757–763. doi:10.1021/acs.estlett.8b00579
Ye, C., Xue, C., Zhang, C., Ma, Z., Liu, P., Zhang, Y., et al. (2021). Atmospheric Hydrogen Peroxide (H2O2) at the Foot and Summit of Mt. Tai: Variations, Sources and Sinks, and Implications for Ozone Formation Chemistry. JGR Atmos. 126 (15), e2020jd033975. doi:10.1029/2020jd033975
Zein, A. E., Romanias, M. N., and Bedjanian, Y. (2014). Heterogeneous Interaction of H2O2 with Arizona Test Dust. J. Phys. Chem. A 118 (2), 441–448. doi:10.1021/jp409946j
Zhao, Y., Chen, Z., Shen, X., and Huang, D. (2013). Heterogeneous Reactions of Gaseous Hydrogen Peroxide on Pristine and Acidic Gas-Processed Calcium Carbonate Particles: Effects of Relative Humidity and Surface Coverage of Coating. Atmos. Environ. 67, 63–72. doi:10.1016/j.atmosenv.2012.10.055
Zhao, Y., Chen, Z., Shen, X., and Zhang, X. (2011). Kinetics and Mechanisms of Heterogeneous Reaction of Gaseous Hydrogen Peroxide on Mineral Oxide Particles. Environ. Sci. Technol. 45 (8), 3317–3324. doi:10.1021/es104107c
Zhou, L., Wang, W.-G., and Ge, M.-F. (2012). Temperature Dependence of Heterogeneous Uptake of Hydrogen Peroxide on Silicon Dioxide and Calcium Carbonate. J. Phys. Chem. A 116 (30), 7959–7964. doi:10.1021/jp304446y
Zhou, L., Wang, W., Gai, Y., and Ge, M. (2014). Knudsen Cell and Smog Chamber Study of the Heterogeneous Uptake of Sulfur Dioxide on Chinese Mineral Dust. J. Environ. Sci. 26 (12), 2423–2433. doi:10.1016/j.jes.2014.04.005
Keywords: heterogeneous uptake, hydrogen peroxide, sulfur dioxide, coal fly ash, uptake coefficients, synergistic reaction
Citation: Zhou L, Lei T, Kang D, Guo Y, Zhang Y, Yang F, Ge M and Wang W (2022) Kinetics of Heterogeneous Reaction of H2O2 and SO2 on Coal Fly Ash: Temperature Effect and Their Synergistic Effects. Front. Environ. Sci. 10:876289. doi: 10.3389/fenvs.2022.876289
Received: 15 February 2022; Accepted: 05 May 2022;
Published: 15 June 2022.
Edited by:
Bin Yuan, Jinan University, ChinaReviewed by:
Jia Guo, Research Center for Eco-Environmental Sciences (CAS), ChinaChong Han, Northeastern University, China
Copyright © 2022 Zhou, Lei, Kang, Guo, Zhang, Yang, Ge and Wang. This is an open-access article distributed under the terms of the Creative Commons Attribution License (CC BY). The use, distribution or reproduction in other forums is permitted, provided the original author(s) and the copyright owner(s) are credited and that the original publication in this journal is cited, in accordance with accepted academic practice. No use, distribution or reproduction is permitted which does not comply with these terms.
*Correspondence: Weigang Wang, d2FuZ3dnQGljY2FzLmFjLmNu